- 1Plant Immunity and Biochemistry Laboratory, Biochemistry and Molecular Biology Section, Department of Biology, Biochemistry and Natural Sciences, Universitat Jaume I, Castellón, Spain
- 2Department of Biotechnology, Ghent University, Ghent, Belgium
- 3Faculty of Sciences, Institute of Biology, University of Neuchâtel, Neuchâtel, Switzerland
- 4Department of Soil and Plant Microbiology, Estación Experimental del Zaidín (EEZ), Spanish National Research Council (CSIC), Granada, Spain
- 5Molecular Phytobacteriology Laboratory, Korea Research Institute of Bioscience and Biotechnology (KRIBB), Daejeon, Republic of Korea
- 6Section of Cell and Developmental Biology, University of California, San Diego, La Jolla, CA, United States
- 7School of Biosciences, University of Sheffield, Sheffield, United Kingdom
Abstract
Induced resistance (IR), which enables plants to increase their resilience against insect pests and microbial pathogens by promoting their own immunity, has been recognized for its value in crop protection schemes. Despite promising applications, the use of IR in crop protection has remained marginal compared with pesticides and single resistance genes. This review aims to shed light on this disparity by examining the scientific milestones achieved over the past decades for both internal (immunological) and external (ecological) IR strategies. The multifaceted advantages of IR, particularly its ability to provide broad-spectrum protection and enhance the nutritional and nutraceutical value of certain crops, are also discussed. The various obstacles hindering the widespread adoption of IR strategies are then revealed. After considering recent scientific discoveries and insights, potential solutions are proposed, including leveraging epigenetic approaches to understand the mechanisms involved in IR. By acknowledging that the future sustainability of crop protection is irreconcilable with single-use technologies, this review proposes taking advantage of the latest insights regarding the adaptive nature of the plant immune system and its ecological interactions to safely integrate IR into existing crop protection schemes. By emphasizing the need for comprehensive and holistic approaches to basic and translational research, this review sets the stage for leveraging IR alongside other strategies to foster a resilient, environmentally friendly, and economically viable future, thereby ensuring the health of crops.
Key points
- Sustainable crop protection methods, such as induced resistance (IR), are being harnessed as an alternative to the excessive use of pesticides, which can lead to increased pest resistance to pesticides and harm humans, animals, and the environment.
- IR can offer long-lasting protection to help the agricultural system mitigate the challenges posed by climate change, the emergence of new diseases, and a rapidly changing socioeconomic context.
- IR is not only active in plant defense but can also be exploited to improve the nutritional quality and health benefits of crop products.
- Maximizing the benefits of IR will require a better understanding of plant biology interactions (including the epigenetic regulation of plant defenses), IR stimuli, and the environment.
- Legislative action is important to ensure the quality and effectiveness of IR products and promote their wider adoption by farmers.
Introduction
Throughout history, plant pests and diseases have had a profound influence on human history, causing episodes of food insecurity and human suffering that have scarred our shared past. Devastating outbreaks, such as the Irish Potato Famine in the 19th century, have caused widespread hunger, economic turmoil, and mass migration with long-lasting historical consequences. Since the “green revolution” in the second half of the 20th century, our high-intensity food supply chain has become increasingly dependent on crop monocultures that are critically reliant on the excessive use of pesticides to protect against pests and diseases. However, this reliance is no longer deemed sustainable. In addition to concerns about non-target effects in the environment (1–4), the development of resistant pests, and concerns about the human health impacts of chemical residues in food products, the production and application of pesticides also generate a significant carbon footprint (5). Increasingly, such concerns have begun to influence agricultural policies globally. For example, the European Commission has proposed new policies with agricultural initiatives to reduce pesticides, such as the “European Green Deal” and “Farm to Fork Strategy” (6), which both propose a target of a 50% reduction in agrochemicals by 2030. Similar policies are being pursued in Japan, via the “Sustainable Food Systems Strategy MIDORI” (7), in the United States (8), and globally under the umbrella of the United Nations (9).
However, phasing out pesticides without replacing them with reliable alternatives can expose the food supply chain to risks that seriously affect regional food security and increase the volatility of the global food economy (10–13). Hence, there is an urgent need to identify and implement reliable alternatives to pesticides. One of the currently emerging crop protection practices identified by the European Commission is to harness strategies naturally employed by plants to resist pests and diseases (6). A plant’s immunity can be strengthened by stimulation with certain pathogens, pests, beneficial microbes, chemical agents, physical wounding, or herbivory (14). This phenomenon, known as induced resistance (IR), often provides broad-spectrum protection against a variety of pests and diseases (15, 16). IR allows plants to respond more quickly to biotic stressors (16) and can be combined with other crop protection strategies (15). IR is crucial for minimizing reliance on pesticides; moreover, in conjunction with our increasing knowledge of internal (immunological) and external (ecological) plant defense strategies (17), ample new opportunities exist to expand our current toolkit to ensure the sustainability of future plant health management. In turn, this contributes to achieving global food security and ensuring a sustainable food supply for a growing population.
How do plants defend themselves against pests and diseases?
Plants, much like all higher organisms, rely on several strategies to defend themselves against pests and pathogens (Figure 1). The first line of self-defense is controlled by the plant’s innate immune system, which operates according to a genetic blueprint that enables plants to detect signals and activate their inducible defense mechanisms. The innate immunity of plants has been studied extensively over recent decades and conceptually involves two separate layers (18, 19). The default layer, known as pattern-triggered immunity (PTI), responds to microbial molecular patterns such as flagellin, elongating factors, and danger signals and involves a variety of defense genes and mechanisms. This innate immune response provides a wide range of protection against different styles of attack. However, it has the disadvantage of being relatively weak against specialized attacks that involve PTI-suppressing virulence effectors (20). As an evolutionary response, plants can defend themselves via effector-triggered immunity (ETI), which is based on resistance (R) genes. R genes encode intracellular receptors that can activate the hypersensitivity immune response upon the direct or indirect recognition of immune-suppressive virulence effectors and are critical for so-called gene-for-gene, race-specific, or vertical resistance (21, 22). Despite the highly effective response of ETI to biotrophic parasites, its range of effectiveness is typically limited to a single species or even subspecies levels, such as pathovars. Moreover, the ongoing co-evolution by ETI-resistant attackers is selective for the emergence of new virulence effectors that are no longer recognized by existing R proteins, rendering ETI ineffective.
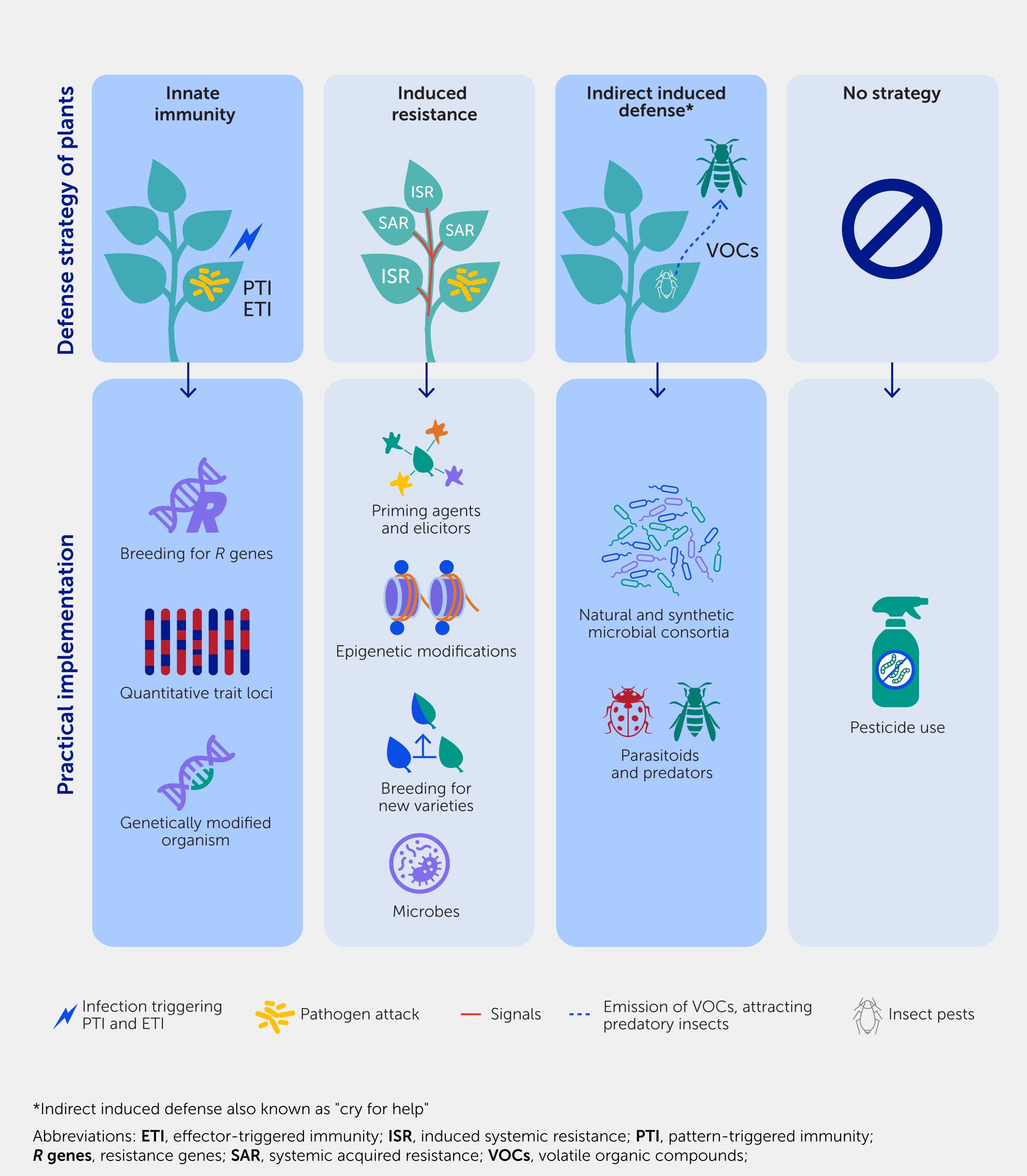
Figure 1. Defense strategies of plants and their practical implementation. Plants rely on various defense strategies for their survival when exposed to pathogens and/or pests. In the absence of an effective strategy, pesticides must be used to ensure plant survival.
While PTI and ETI are important first layers of defense, plants require additional strategies to survive in hostile environments. When plants successfully repel initial assaults by pests or diseases they develop acquired or induced resistance through the activation of their own immunity. IR often involves a form of immunological stress memory referred to as “defense priming”—a phenomenon that conditions plants for enhanced stress resilience. Stress or defense response pathways are initially only weakly activated upon exposure to the priming agent but are then more strongly or rapidly induced when the plant is later challenged by an attacker (16, 23, 24). A classic example of defense priming is systemic acquired resistance (SAR), which occurs in response to localized pathogen attacks, triggering the release of long-distance defense signals that prime distal plant parts for the efficient activation of defenses upon later attacks, leading to enhanced resistance in a phenomenon similar to vaccination in humans (14). This phenomenon involves the finely tuned regulation of phytohormone signaling, elevated levels of pattern recognition receptors or dormant defense regulatory elements, and chromatin modifications (16, 25, 26). The priming effect can be long-lasting; it can even be transmitted to the next generations (27–32). There is mounting evidence that the long-term maintenance of priming is controlled by epigenetic changes, such as the reduced DNA methylation of transposons and the trimethylation of lysine 4 of histone subunit H3 (H3K4me3) at defense gene promoters, which have also been implicated in “poised” genes (genes in a “ready to be active” state) in animals [Box 1, (26)]. However, much remains to be discovered about the mechanisms through which stress-induced epigenetic changes prime stress-specific defense genes, how these epigenetic changes are maintained over cell division and sexual reproduction, and, ultimately, how they are erased in the absence of recurrent stress.
Box 1. Epigenetics
Regulation of the timing and amplitude of gene activation is crucial for the energy-efficient and effective induction of defense upon attack. The importance of epigenetics as a major regulator of plant defense gene expression has only recently emerged. Epigenetics refers to changes in gene expression that can influence the phenotype of an organism without causing alterations in DNA sequence. Chromatin refers to the complex of nucleic acids and proteins within the nucleus of eukaryotic cells. More specifically, DNA is wrapped around an octameric complex of two sets of four histone cores to form one nucleosome. Changes to the chromatin structure, DNA, or histones are commonly considered to be “epigenetic modifications”.
Chromatin remodeling: The parts of the chromatin that are firmly packed and genetically inactive are called “heterochromatin”; they are characterized by high levels of DNA methylation (see below) and are typically inaccessible to transcription factors and the transcriptional machinery. In contrast, euchromatin refers to loosely packed chromatin and is characterized by genes that are actively transcribed or responsive to induction. Chromatin remodeling can shift the transcriptional status of protein-coding genes from being silenced to being responsive and/or active and vice versa.
Histone modification: The N-terminal tails of histones can be post-translationally modified by, for example, acetylation or methylation at lysine (and sometimes arginine or other amino acid) residues. These modifications have a strong influence on the potential activity of the associated DNA sequence. Histone acetylation is typically associated with active genes in euchromatin. Other modifications, such as SUMOylation (involving small ubiquitin-related modifier, SUMO), can also play a role in the stability and/or replacement of histones with other variants.
DNA methylation: The fifth C in the base cytosine (C) can be methylated. In plants, this can occur in CG, CHH, or CHG1 and is mediated by DOMAIN REARRANGED METHYLTRANSFERASE (DRM2). The maintenance of DNA methylation is controlled by three different proteins. CG methylation is performed by DNA METHYLTRANSFERASE1 (MET1), CHG by CHROMOMETHYLASE3 (CMT3), and CHH methylation maintenance is controlled by DRM2 and CMT2. DNA demethylation in plants is mediated by DNA glycosylases/lyases, such as REPRESSOR OF SILENCING1 (ROS1) in Arabidopsis. Active DNA methylation and demethylation dynamically regulate the global and/or locus-specific states of chromatin, which is particularly important in transposon-rich regions that require tight regulation of epigenetic control to prevent transposon re-activation or the spreading of silencing into neighboring protein-coding genes.
Chromatin remodeling and DNA methylation occur in a plant-specific process known as RNA-directed DNA methylation (RdDM), which is influenced by non-coding RNAs, which mainly consist of 21- to 24-nucleotide small interfering RNAs (siRNAs). RdDM accounts for 30% of all DNA methylation in the model plant Arabidopsis thaliana and mainly controls methylation in CHH.
Plants also employ external ecological pathways to adapt to biotic stress; this is known as “indirect induced defense” or “plants crying out for help” (33). This mechanism allows plants to recruit other organisms to combat attackers. For example, the herbivore-induced emission of volatile organic compounds (VOCs) can attract predatory or parasitic insects that attack the impeding herbivore (34). Recently, similar interactions have been discovered above and below ground, where stressed plants alter their root exudation and headspace VOC chemistry to select and/or recruit a disease-suppressive microbiome. This also activates an immune response in neighboring plants, which protects the plant via a range of direct and indirect mechanisms, including induced systemic resistance (ISR) (35–37).
The current approach to crop protection still relies largely on pesticides and selective breeding for R genes, whereas strategies such as IR remain relatively underexploited. Unlike pesticides, IR is not curative and rarely provides complete protection against pests or microbial pathogens. Moreover, IR chemicals may have associated allocation fitness costs (trade-offs), such as reduced plant growth and yield or increased susceptibility to other stresses. These reasons may contribute to IR remaining underexploited. However, when applied in the correct context, IR offers numerous benefits. In addition to its broad-spectrum effectiveness, IR can help reduce pathogen loads, synergize with other management methods, and protect single R gene strategies from co-evolving pests and disease adaptations. This can help to decrease our reliance on unsustainable pesticides (38).
In this review, we explore the potential applications of IR and discuss how it can be integrated with the use of pesticides, other crop protection methods, and breeding strategies. By examining the costs and benefits of IR, we aim to establish a realistic framework for the safe exploitation of IR within current crop protection schemes.
Significant milestones in research into induced resistance
Reports on how IR combats plant pathogens date back to the 1930s (Figure 2). Initially, these reports were descriptive, presenting instances in which IR-like phenomena were observed [reviewed in Chester (39)]. In 1961, the term SAR was introduced by Frank Ross, who reported an experiment showing that inoculating a tobacco leaf with tobacco mosaic virus (TMV) led to resistance against the same virus in distal non-inoculated leaves 1 week after the first inoculation. In the 1960s, numerous studies on the biological induction of resistance were conducted in various plant species in which resistance was triggered by pathogens such as viruses, bacteria, and fungi (40).
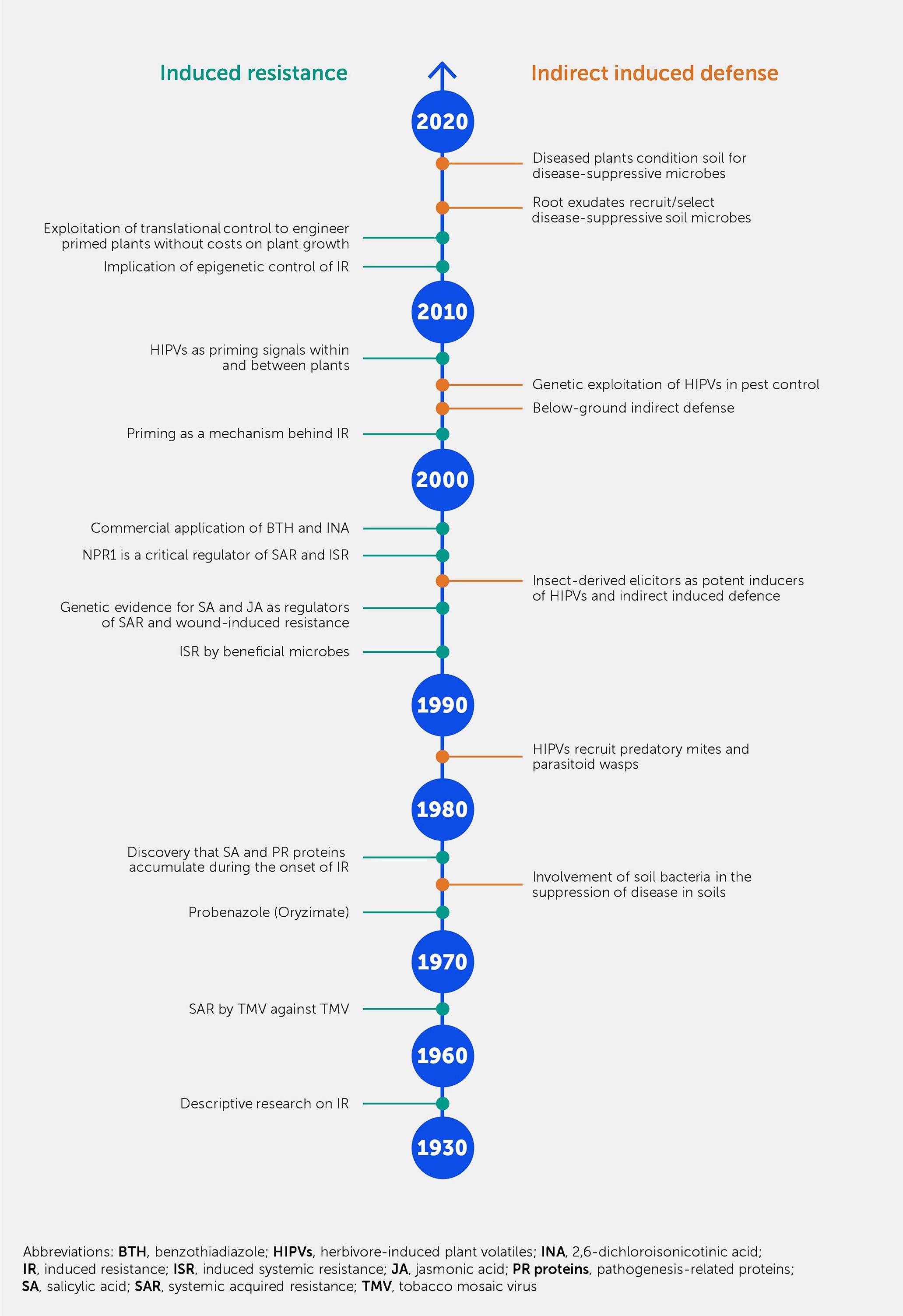
Figure 2. A not-to-scale timeline of the main events and discoveries in the field of induced resistance. The manifestation of resistance is either based on events leading to changes in the plant itself (left side of the time axis) or in the plant’s environment (right side of the time axis).
A novel aspect of IR was described in a report by White (41): it showed that infiltration of the leaves of tobacco plants with salicylic acid (SA) led to SAR against TMV and induction of pathogenesis-related (PR) proteins. This is consistent with the later-described effects of endogenous SA during plant defense (42, 43). Ultimately, this discovery allowed screening programs that identified the functional analogs of SA as IR elicitors, which yielded products such as 2,6-dichloroisonicotinic acid (INA) and acibenzolar-S-methyl (ASM; an active ingredient of the commercial product Bion) (44, 45). As early as 1975, oryzimate was used as a resistance inducer in rice seedlings (46). Transgenic plants, such as SA-degrading NahG plants, which cannot accumulate SA (47), or the Nonexpressor of pathogenesis-related genes 1 (npr1) Arabidopsis mutant (48, 49), have helped to provide a deeper mechanistic understanding of IR signaling. Similarly, the discovery of jasmonic acid (JA) as an endogenous wound-inducible IR signal against insects and necrotrophic pathogens (50, 51) led to new research into the biosynthesis and response pathways of this IR-related hormone (52). Toward the end of the 2000s, researchers independently reported long-distance signals mediating systemic IR responses, such as methyl salicylate (53), azelaic acid (54), glycerol-3-phosphate (55), dehydroabietinal (56), and N-hydroxy pipecolic acid (57) for SAR, as well as electrical signals for the systemic IR response to wounding (58).
At approximately the same time, it was discovered that prolonged maintenance of IR-related defense priming involves epigenetic control (26) (Box 1), offering a plausible mechanism for the long-term (and sometimes transgenerational) durability of IR. More recently, it was shown that IR-related priming can be genetically engineered by fusing the NPR1 gene to a pathogen-responsive untranslated open reading frame (59) or epigenetically induced by the introgression of meiotically stable regions of reduced DNA methylation (60).
In the early 1990s, it was shown that IR was developed upon recovery from biotic stress, such as localized disease or herbivory, as well as being triggered by the root colonization of beneficial plant growth-promoting rhizobacteria (PGPR) (61–63).
ISR typically operates independently of SA but requires NPR1 and the intact jasmonate and ethylene (ET) signaling pathways (64–66). Subsequently, IR induction by beneficial fungi such as mycorrhizae (67) and even whole microbiota (68, 69) has been amply documented (Box 2).
Box 2. Microbe-elicited induced resistance
Microbial induction of induced resistance (IR) in plants resembles human immunization, i.e., inoculation with vaccines containing live attenuated or killed microbial pathogens. However, the potential risks of using pathogens as triggers or IR in agriculture (uncontrolled multiplication and/or virulence increase) have increased scientists’ interest in non-pathogenic microbes. In and around the root system (the rhizosphere), various non-pathogenic microbes, including bacteria, protozoa, and fungi, thrive and interact with the root and other microbes. The ability of soil microorganisms to directly control plant pathogens through antibiosis and competition for niches and nutrients has been known for several decades. However, their effect on stimulating plant immunity has been overlooked. In 1991, three independent groups reported microbe IR in cucumber, bean, and carnation (62, 63, 70). The spatial separation of beneficial bacteria (inoculated in the root), the pathogens (on the leaf or separated root), and the confirmation of non-translocation from the root to the leaf of the beneficial bacteria allowed them to confirm that the reduction in disease severity was plant-mediated, providing evidence for the involvement of plant immunity. Many beneficial microbes, including plant growth-promoting rhizobacteria and fungi and mycorrhizal fungi, have been shown to trigger IR under controlled laboratory conditions and in the field (71–76). There is a growing interest in the use of microbial inoculants to stimulate plant resistance to diverse stresses. Many microbial products are already on the market; they usually include spore-forming bacteria (a microbial resting form) such as bacilli (Bacillus spp. and Paenibacillus spp. group) or spore-forming fungi (Trichoderma, Piriformospora, and arbuscular mycorrhizal fungi) (Supplementary Table 1). Accordingly, their successful application in greenhouses and fields has evidenced their potential to protect crops against a broad spectrum of plant pathogens, resulting in marketable yield increases while reducing the need for agrochemicals (synthetic fertilizers and pesticides) and improving food safety and quality (77, 78). Thus, these IR-inducing microbes can be used as probiotics in agriculture to positively modulate plant immunity, which is similar to the reported positive effects of probiotic consumption on human immunity (79, 80).
The mechanisms by which microorganisms trigger IR are diverse; they are mostly common to those triggered by inert elicitors, but beneficial microbes usually act by priming jasmonate-dependent defense responses (66). However, there are specific advantages and limitations associated with the use of microbial inoculants for IR elicitation, as summarized below.
Advantages of microbe IR:
● Microbes can act as biofactories of IR-eliciting molecules (81). If they successfully colonize the target niche, there is no need for reapplication, unlike the repeated application recommended for most abiotic IR elicitors.
● Beneficial microbes can provide benefits to plants beyond IR, such as enhanced abiotic stress tolerance, nutrient acquisition, and increased nutritional/nutraceutical value of the edible parts of the plant (82–86).
Challenges of microbe IR:
● As microbes are living organisms, their shelf-life is limited compared with that of agrochemicals.
● There are more complex requirements for the formulation and large-scale production of microbe-based products than for agrochemical bioactive compounds.
● Niche competence is required as the introduced microbes need to integrate/coexist with the native microbiota.
● High context dependency: microbe survival, replication, and interaction with the plant and its indigenous microbes are highly dependent on abiotic and biotic contexts (87).
● Regulations governing microbial inoculants should be updated to fully recognize the key features of their true biological and living nature, as the current regulations equating them to chemicals hampers their optimal development and implementation (88).
Knowledge gaps and research directions:
● Identify microbial IR determinants. Multiple microbial-derived compounds have been shown to stimulate IR. Microbes can secrete phytohormones such as auxin, cytokinin, ethylene, jasmonic acid, and salicylic acid and antimicrobial compounds such as phenazine, 2,4-diacylphloroglucinal, and lipopeptides that also modulate immune signaling (89). Components of fungal and bacterial cell walls, including chitin, chitosan, glucans, lipopolysaccharides (LPS), and peptidoglycans, can also elicit IR (90, 91). Recently, microbial volatile compounds, such as 2,3-butanediol and acetoin, have been shown to activate IR against bacterial and fungal pathogens (92, 93). However, there is limited knowledge regarding the regulation of their production and elicitation potential under different conditions (93).
● Identify major drivers of microbe IR context dependency. This would be essential for tailoring crop management for improved IR efficiency and stability.
● Improve inoculants’ functionality and context stability by designing microbial consortia and synthetic communities with complementary and/or redundant functions and different requirements.
● Engineer soil microbiota to maximize the stimulation of plant immunity. Understanding of the regulation of microbial community assembly and function is required for such management strategies.
Indirect induced defense by plants was initially described for plant–herbivore interactions, whereby the release of herbivore-induced plant volatiles (HIPVs) recruit natural enemies of the herbivore, such as predatory spider mites or parasitic wasps (94–96). The discovery of this “cry-for-help” led to follow-up studies showing that insect-derived elicitors, such as glucose oxidase and volicitin, boosted wound-induced volatiles (97) and that similar tritrophic interactions could occur below ground, allowing the recruitment of entomopathogenic nematodes (98). Based on enhanced knowledge of the genetic control of HIPVs, subsequent studies also provided proof of concept that the “cry-for-help” mechanism could be exploited in genetic strategies to improve crop protection (99, 100). In subsequent years, it became clear that both volatile and non-volatile metabolites in root exudates could recruit and/or select for disease-suppressive soil microbes (101–103). This precipitated more recent studies that have shown that the “cry-for-help” via root exudates conditions the soil for ISR-eliciting microbial consortia (104, 105). As for the internal epigenetic maintenance of IR (26), this “cry-for-help” in root exudates can protect plants across generations by conditioning the soil microbiome for disease-suppressive activity (17, 36), and it offers a plausible explanation for earlier reports about the development of disease-suppressive soils upon multiple cultivations with high disease incidence (e.g., take-all decline) (106).
Knowledge about microbe-, herbivore-, and damage-associated patterns triggering IR, such as cell wall components (e.g., oligogalacturonides from pectin; laminarin from algae; flagellin from bacteria; and Reynoutria extract, chitin, and chitosan from fungi and insects), as well as endogenous IR signals, such as β-aminobutyric acid (BABA), have allowed the development of commercial products in which microbes themselves or derived molecules constitute the active principle (Supplementary Table 1). Further development of such commercial products is ongoing: for example, there is a product based on the pheromone ascaroside 18 that is secreted by plant-parasitic nematodes (107, 108). Ascaroside 18 induces resistance in a variety of plant species to a broad spectrum of pathogens by activating both the SA-mediated and JA-mediated defense pathways, and its receptor has been identified recently (109). Ascaroside 18 is expected to be placed on the market in 2026 (D. Klessig, Boyce Thompson Institute, personal communication2).
Opportunities for induced resistance
Over six decades of research support IR as a potentially highly promising strategy to protect crops. IR is effective in protecting plants against a broad range of pathogens and pests. Although it may not provide 100% protection under high disease-pressure conditions, it offers an alternative to ETI-based strategies when the R gene repertoire is limited or unavailable, such as against necrotrophic pathogens and chewing herbivore pests (110, 111). Its multigenic (horizontal) character allows it to overcome resistance breakdown, and it can thus be used when resistance breakdown to pesticides or gene-for-gene strategies occurs (112). In the case of emerging diseases and pests, IR can provide faster solutions than traditional breeding. IR also offers an alternative in pathosystems for which no effective pesticides are available or where their use has been (or will be) banned and no gene-for-gene strategies are available. Because of its wide spectrum of effectiveness, IR can provide immediate protection against these challenges (Figure 3). Although the protection provided by IR may not necessarily be complete, it could still offer substantial benefits for farmers and protection against the devastating consequences of newly emerging pests and diseases.
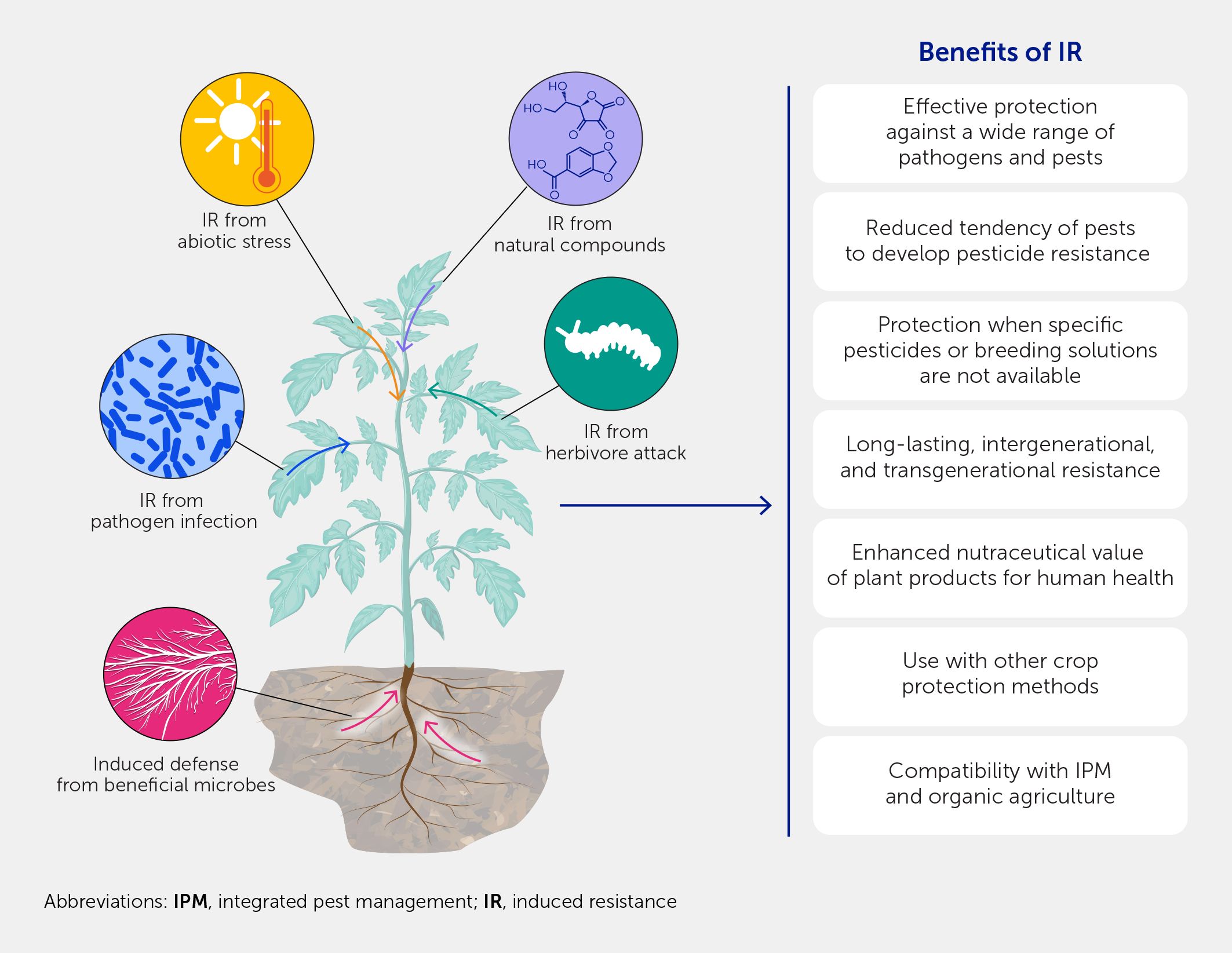
Figure 3. Opportunities arising from induced resistance (IR). IR offers a broad spectrum of protection against pests and pathogens and can act synergistically with other crop protection methods. IR can also offer protection where a pesticide cannot; therefore, it is an ideal strategy to use during outbreaks in which a novel pest is involved. The benefits of IR also extend beyond crop protection to higher levels of bioactive compounds in fruits, vegetables, and herbs as a result of the IR from stimulating plant defenses.
Interestingly, the induction of plant defenses implies that plant secondary metabolism has been activated, which involves the biosynthesis of multiple bioactive compounds with health-promoting activities, such as polyphenols, carotenoids, flavonoids, phytoestrogens, and the activity of several antioxidant enzymes. Thus, the elicitation of IR, while stimulating plant defenses, frequently results in higher levels of these bioactive phytochemical compounds in fruits, vegetables, and herbs. Most of these metabolites have been investigated to assess their potential role in specific organoleptic properties of foods and show protective effects in human cells. Indeed, the application of biotic and abiotic elicitors and defense-related phytohormones during plant growth or the postharvest period has been used to enhance the production of secondary metabolites and produce high-quality and healthy fresh foods (113, 114). Beneficial plant symbionts triggering IR, such as mycorrhizal fungi and PGPR, can also improve the nutritional and nutraceutical value of fruits, as shown by the increased content of lycopene and beta carotene in tomato fruits, stronger anti-estrogenic activity (115–117) and higher concentrations of iridoids (oleuropein and secologanin) and flavonols in olive oil (118), and higher phenolics and anthocyanin content in berries (119).
Finally, therefore, IR in combination with low doses of chemical pesticides reduces the evolutionary pressure for pests and diseases to develop resistance against agrochemicals and may also complement R gene strategies by protecting available R genes against co-evolving pathogens (120–123). Under low- to moderate-pressure stress, IR is sufficient when stronger solutions are lacking or may function in complement or synergy with other plant protection methods. The current and emerging agri-tech solutions offer new opportunities to facilitate the incorporation of IR in common agricultural programs. In the next section, the limitations of IR, their potential solutions, and the application of IR in modern agriculture (Table 1) are discussed.
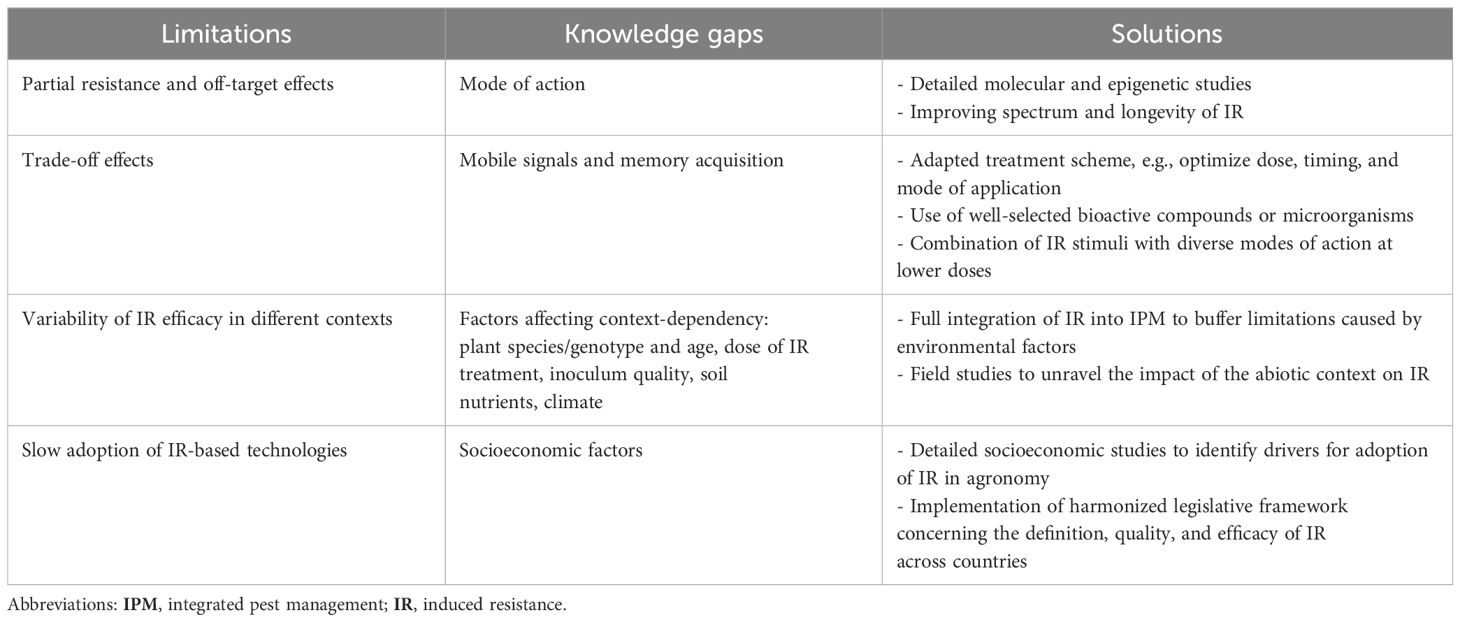
Table 1. Limitations and knowledge gaps relating to induced resistance (IR) and proposed solutions. IR is a promising strategy for crop protection; however, there are identified limitations and research gaps that should be addressed to allow the full potential of IR to be reached. Below, we present the solutions, which will require collaboration from different stakeholders.
Factors limiting the adoption of induced resistance
Growth penalty caused by allocation fitness costs (trade-off)
Limitation
The activation of defense mechanisms requires energy and resources. Hence, the strong induction of IR can lead to a growth penalty, referred to as the allocation fitness cost. For example, negative effects on growth and development are typically more pronounced when plants are exposed to high concentrations of hormones or hormone-mimicking compounds as IR stimuli. These include methyl jasmonate (MeJA), SA, and SA-analogs such as benzothiadiazole (BTH) (124), BABA (125), acibenzolar-S-methyl (ASM) (126, 127), and INA (128, 129). This is unsurprising because an increase in defense hormone levels will result in the direct activation of defense pathways but cause a decrease in the effectiveness of defense priming, which is the more energy-efficient pillar of IR (16, 24). Moreover, defense hormones may perform antagonistic crosstalk with other developmental and environmental pathways and may even render plants more susceptible to other environmental stresses. For example, a recent study showed that treatment of Arabidopsis seedlings with JA results in long-lasting IR against herbivory but is associated with increased susceptibility to both necrotrophic and biotrophic pathogens (130). Therefore, caution is recommended when using hormone-mimicking compounds as IR agents, and the possibility of costs arising from reduced growth or increased susceptibility to other stresses must be considered. Overall, higher yield penalties are expected during IR compared with R gene strategies and pesticides (125). However, high pesticide doses may also lead to phytotoxic effects and have a negative impact on yield (131). Therefore, the adequate choice of IR stimuli, doses, and careful evaluation in the field may be essential before large-scale application. The innate plant immunity system comes with endogenous limitations. Internal feedback mechanisms and the interactions between primary and secondary metabolism engage to balance energy consumption. Consequently, only moderate levels of protection, typically ranging between 20% and 85% (132) and averaging around 40–60% (currently under study), can be achieved with IR. For farmers, the major goal of agriculture is economic benefit from yield. Therefore, yield reduction due to IR treatment can have a critical impact on agriculture, and farmers must consider the balance of cost and benefit when using IR to protect their crops.
Solution
A precise scientific assessment of fitness costs arising from the plants’ attempts to compensate for the growth penalty following IR must be established. Even if a growth penalty occurs, IR treatments can be adapted to avoid the reduction in benefit from economic loss caused by plant pathogens. However, should a growth penalty be expected in certain crops due to treatment with a specific elicitor, it could be countered and minimized by the concomitant application of other microbes or chemicals that promote crop growth.
Indeed, the induction of IR by well-selected bioactive compounds may also yield positive effects by increasing the photosynthesis and nutritional status of plants. For example, IR by natural compounds such as dehydroascorbic acid (DHA) and piperonylic acid (PA) positively affect plant growth and yield when applied in rice fields (133). Carboxylic acids have also been reported to induce resistance against fungal pathogens (134), and they also stimulate plant metabolism and photosynthesis (135) and promote the recruitment of beneficial microbiota (136). Similarly, the induction of IR by beneficial microbes is usually not associated with yield penalties, as microbes confer other benefits to the plant, such as improved nutrient acquisition and enhanced stress tolerance (Box 2). Indeed, numerous commercially available products categorized as biostimulants and biofertilizers, including diverse plant growth-promoting microorganisms, can trigger IR (Supplementary Table 1; Box 2).
Another strategy to improve overall efficacy and reduce the trade-off might be to combine multiple IR stimuli using different modes of action at subtoxic doses (137). Indeed, a combination of half-dose ASM and BABA exerted an additive effect, controlling Plasmopara viticola in grapevine (138). Similarly, a combination of MeJA and Trichoderma harzianum was more effective in controlling spot blotch than either treatment alone in bread wheat (139), and a combination of T. harzianum and ASM provided complete protection against gray mold in fava bean (140). Although these are examples of the use of multiple stimuli with additive or synergistic effects, careful selection of the compounds and doses is required to avoid growth penalties. For example, the drench application of BTH successfully protected pepper plants against Xanthomonas axonopodis pv. vesicatoria and cucumber mosaic virus but led to severe growth retardation and yield decrease. However, IR triggered by a combination of BTH and the PGPR strain Bacillus pumilus increased pepper IR capacity against both pathogens more effectively than individual treatments under field conditions and without any growth penalty (124).
Generating detailed insights into the mode of action of candidate IR stimuli is essential to support agricultural implementation and the design of adequate control strategies. Timing, dose, mode of application, and durability of the IR effect should be evaluated in laboratory experiments to allow adequate experimental design for field trials.
Inconsistency in induced resistance efficacy: external biotic/abiotic factors reduce induced resistance capacity
Limitation
The context-dependency of IR, namely the variability in IR efficacy relating to environmental and agronomic conditions, and phenological plant stages are limitations of IR that require further research.
Arguably, the most confounding factor in IR implementation in agronomy is the fact that environmental conditions can affect the general outcome of IR, leading to limited reproducibility of the resistance level achieved. For example, Walters et al. (141) showed that the efficacy of saccharin IR against Rhynchosporium secalis in barley depends on various parameters, including disease pressure. In addition, differences in effectiveness against different pathogen isolates have been reported (142), although the underlying reasons are not well understood.
IR is also dependent on the plant genotype. Several reports have shown that diverse cultivars of the same plant species can respond differently to IR stimuli. For example, the effects of induction treatment with INA or BTH differed among cucumber and soybean genotypes (143, 144). The efficiency of a combination of ASM, BABA, and cis-jasmonate in eliciting IR also varied among cultivars in barley against powdery mildew (Blumeria graminis) and leaf scald (Rhynchosporium secalis) in both controlled and field trials (145) and in tomato against Phytophthora (142). Similarly, some genotypes are more responsive to elicitation by beneficial microbes than others. Indeed, ISR has been shown to depend on the cultivar under laboratory and field conditions (146). Mycorrhiza-induced resistance (MIR) is also genotype-dependent and graft-transmissible, as reported for common bean and tomato (147), even though the mechanisms may differ depending on the plant genotype. For example, IR against powdery mildew was associated with epicuticular wax deposition and the overexpression of PR genes or with the accumulation of hormones and antimicrobial compounds in different grapevine cultivars (148).
The age of the treated plant also influences the efficacy of IR. As the growth season continues and the crop ages, plant defense mechanisms are expected to be induced by various natural stress factors to which the crop is inevitably exposed. This was contradicted by a study in potato showing that only approximately 30% of the field samples from Swedish wild and cultivated Solanum spp. expressed PR proteins (149). Indeed, the constitutive activation of plant defense is very energy-demanding for a plant and may reduce potential interactions with beneficial microbes. Nevertheless, young plants might benefit more from IR, as they lack age-related basal resistance. There is also evidence that the treatment of seedlings with IR agents can result in long-lasting IR, which is transmitted into newly formed leaves via epigenetic pathways (130, 150). Moreover, recent evidence has shown that the consistency and effect size of intergenerational acquired resistance (IAR) in Arabidopsis is higher upon induction treatment of young seedlings (151). Hence, the treatment of young plants under field conditions can be exploited as a preventive and durable method to enhance disease protection before pathogens can suppress immunity.
Biotic and abiotic contexts also contribute to the context dependency of IR under field conditions. Complex crosstalk occurs between abiotic and biotic stress signaling pathways in plants (152). For example, in Arabidopsis, elevated temperatures promote the expression of MYC2, a master positive regulator of JA signaling but a negative moderator of SA signaling (153). Thus, temperature is expected to modulate IR efficiency. Drought stress induces abscisic acid accumulation in multiple plants and is known to cause susceptibility to some pathogens (154, 155) but increase resistance to other pathogens (156). A thorough evaluation of how combined stresses affect plant immunity signaling and how defense signaling pathways interact and/or prioritize under realistic multi-stress conditions is needed, particularly in the current context of climate change (157).
Multiple lines of evidence indicate the effect of mineral nutrition on IR induction. Nutrient availability has been shown to affect plant susceptibility to pathogens, pests, and IR. For example, the enhanced resistance induced by mycorrhizal fungi in tomato against a fungal pathogen and a herbivorous insect was correlated with primed activation of defense genes, but this primed induction was dependent on the availability of nitrogen (158, 159) and phosphorus (160). Similarly, iron availability is also a key factor for IR (161). De Kesel et al. (32) demonstrated that diproline-induced IR is dependent on the iron supply in rice. Similar metabolites appear to accumulate in both IR and in the iron-deficient roots of dicots and monocots, and IR activation by soil-beneficial microbes requires some of the regulatory elements of iron-starvation responses [see (161) and the references therein, and (32)]. Similarly, BABA application and root colonization by some rhizosphere microbes not only led to broad-spectrum IR but also induced physiological and morphological root responses resembling those induced by iron deficiency [see (161) and references therein, and (162, 163)]. This apparent conserved convergence between nutrient deficiency responses and IR deserves further investigation; moreover, the information generated may provide the basis for fertilization recommendations to optimize MIR.
Solution
We propose combining immunological and ecological defense strategies by consolidating them within integrated pest management (IPM) programs. IPM has emerged in recent decades as an efficient and sustainable alternative to protect crops while minimizing environmental costs (164), and the IPM-framed combination of multiple methods can buffer the limitations imposed by the changing environmental conditions for IR (165).
Despite enormous research efforts into IPM, most approaches rely on the use of natural enemies to control pests; few studies have focused on the use of fungal or bacterial strains that inhibit parasites or compete for resources to control phytopathogenic microbes (166). The exploitation of plant immunity as a complementary strategy to be integrated with IPM remains unexplored. Moreover, IR can complement other biocontrol methods; for example, there are microbes that can directly antagonize pathogens, as in the case of the mycoparasitic fungi Trichoderma spp. and antibiotic-producing bacteria. In addition, these microbes can trigger IR, increasing the versatility and context stability of biocontrol inoculants (71). Recent studies suggest that plant immunity has the potential to strengthen IPM strategies in crops such as tomato (167, 168). The lack of research into the combination of IPM and IR is, in part, due to the complexity of studies analyzing the interplay between three or more interacting biological systems, i.e., the plant, the beneficial microorganism, and the pests (169). Research into dynamic multiway interactions revealed that plant immune responses are not fully conserved when interacting simultaneously with several organisms. Hence, studies in which IR is set in a multiway interaction context require a careful multifactorial design, which complicates the analysis and comprehension of the molecular mechanisms regulating plant immune responses.
Upon perceiving a herbivore attack, plants can release specific odors that attract natural enemies, helping them to get rid of the pest, a response referred to as a “cry-for-help” (170, 171). This is part of the immune response, known as indirect IR. Hence, a combination of evolutionarily acquired indirect resistance and the common release of natural enemies may exert synergistic benefits for the crop. Some recent studies have highlighted the potential of improving pest control using a combination of beneficial microbes and natural enemies. The inoculation of plants with beneficial fungi that trigger IR results in an enhanced attraction for natural enemies (172, 173). These results support the synergistic benefits of IR and IPM. Other studies illustrate even higher complexity, pointing to these interactions as additional valuable tools that contribute to crop protection. Groups of hemipteran insect families, such as Miridae and mites from the Phtyoseiidae family, can attack their prey as well as the host plant [zoophytophagous (173, 174)]. After the plant has been stimulated, it generates new waves of odors that complete additional layers of immunity, and the plant becomes more repellent. For example, plants with wounds induced by zoophytophagous mites are repellent to white fly pests (175), which is known as antixenosis.
Our understanding of how environmental factors negatively impact IR is substantially limited by a lack of research. The precise monitoring of the abiotic context and studies on their impact on IR can provide data to support mathematical modeling of the potential efficacy of IR in a given context.
Side effects and off-target effects of induced resistance
Limitation
Although the activation of IR can lead to broad-spectrum pest and disease resistance (176–178), increased susceptibility to IR has been observed in some studies. Walters et al. (145) reported that combinations of BTH, BABA, and cis-jasmone showed efficacy in the field for the control of barley infection by powdery mildew and leaf scald but enhanced barley susceptibility to Ramularia leaf spot. Similarly, although BTH induces resistance against Pseudomonas syringae in tomato, this was associated with increased susceptibility to Spodoptera exigua (179). In fields with multiple stress factors, this is an issue of concern.
Solution
The exploration of genetic variation may allow the selection of crop varieties expressing higher IR levels with minimal side effects on growth, interactions with beneficial organisms, and/or resistance to other biotic and abiotic stresses. They can be selected for conventional and genetic modification breeding schemes. The identification of key regulatory genes in model species allows their exploitation as breeding targets. For example, the discovery of the BABA receptor (IBI1) (150, 180, 181) and transporter (LHT1) (182) revealed that BABA-induced IR can be genetically uncoupled from the undesirable stress response to BABA, which relies on the protein kinase GCN2. As the IBI1, LHT1, and GCN2 genes are all highly conserved across taxonomically unrelated plant species, these genes can be used as breeding targets in crops to select varieties that require lower concentrations of BABA to reach economically satisfactory levels of disease protection with minimal side effects.
Unlocking the potential of induced resistance using epigenetics
The long-lasting effect of IR and its potential to be transmitted to subsequent generations is attributed to epigenetic reprogramming (Box 1). The role of epigenetic regulation in the plant response to pathogens is an emerging area recently used to elucidate plant immunity mechanisms (26). One of the most remarkable pieces of evidence linking epigenetic mechanisms with plant immunity can be demonstrated through the priming of the SA-dependent defense in Arabidopsis, where transcription-promoting modifications of the histone H3 tail in the promoters of SA-inducible defense genes are found (183, 184). These modifications facilitate the formation of open chromatin to allow for faster and/or stronger induction of defense genes (185). Dynamic and transient changes in genome-wide DNA methylation have also been observed upon the elicitation of IR in plants. DNA hypomethylation appears to be an early hallmark of plant defense activation during the onset of IR.
The descendants of IR-elicited plants exhibit IAR, in which plants remain less susceptible to pathogens (30, 32, 186). However, the mediators, target regions, and physiological consequences of the intergenerational maintenance of IR remain largely unknown. Although the exposure of epigenetically heritable IR phenomena to stress tends to be variable with relatively low effect sizes (31, 151), epigenetic modifications at the level of DNA methylation can provide almost complete disease protection that is metastable over multiple generations. The proof of concept for this approach comes from recent studies of epigenetic inbred lines (epiRILs) of Arabidopsis expressing disease resistance against virulent pathogens (60, 187). These epiRILs are genetically identical but vary in DNA methylation at transposable elements (188, 189). Moreover, the epigenetic quantitative trait loci mediating stably inherited resistance to downy mildew have been shown to act through the priming of pathogen-inducible defense genes without concomitant reductions in plant growth (60). Hence, the introduction of epigenetic variation into genetically identical crop inbred lines would facilitate the selection of meiotically stable IR phenotypes. Intriguingly, the loss of function of decrease in DNA methylation 1 (DDM1), a chromatin remodeler responsible for gene body methylation, was recently shown to lead to enhanced responsiveness to suboptimal doses of BABA (190). To advance the selection of epigenetically primed plant varieties, more precise and adjustable methods are required to introduce epigenetic variation into plant genomes. For example, the recent development of “reverse epigenetics” methods, such as the clustered regularly interspaced short palindromic repeats (CRISPR)-based multimodal targeting of chromatin remodelers, allows the precise modulation of IR-regulating epi-loci (191). Recently, an artificial zinc-finger fusion construct of various proteins involved in epigenetic regulation was demonstrated to direct gene silencing of specific target genes in Arabidopsis (22). Although translation to crop plants is still required, this could be of great value for the crop protection and breeding industry. This could also act as a valuable research tool to explore the complex mechanisms by which epigenetically altered genomes prime defense genes and mediate IR. Therefore, more detailed mechanistic studies are needed, not only to decipher the pathways by which plants acquire and maintain their epigenetic stress memory of IAR but also to understand how they erase these stressful memories. Insight into these epigenetic regulatory mechanisms will help improve the spectrum, effect size, and heritability of IR.
Concluding remarks
A series of hallmark events has accompanied the development of agriculture, from its infancy 12,000 years ago with continuous selection and breeding, through the improvement in yield using fertilizer and irrigation during the green revolution, the generation of crops with new attributes through transgenesis (GMOs), and the emergence of integrated pest management and crop improvements based on CRISPR-associated protein (CRISPR-Cas) technology, to utilizing IR to pathogens and pests, as presented in this review (Figure 4). IR offers a remarkable opportunity to support our agricultural system in an environment exposed to rapidly expanding challenges—not only those relating to climate change that favor the rapid spread of pests and emergence of new diseases but also in response to the rapidly changing socioeconomic environment. As scientists, we can investigate the mechanisms underlying IR, as outlined above, propose research, development, and technological advancements, and strive to obtain feedback from extension workers and farmers who are willing to try and implement IR technology.
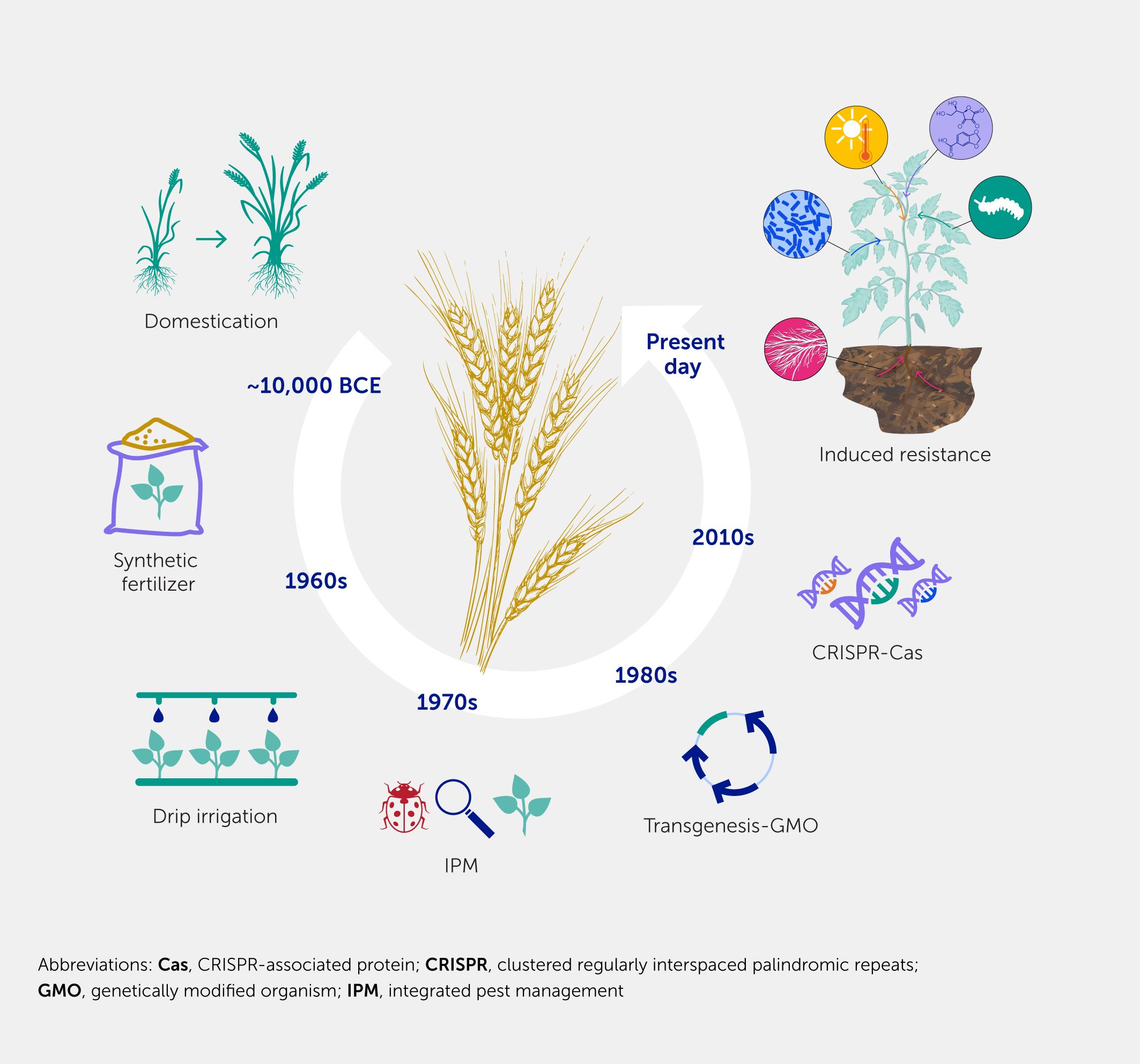
Figure 4. Major events during the history of agriculture. Throughout the history of agriculture, several events have had a huge impact on yield. The first major change was the domestication of wild species, followed by the application of fertilizers and the improvement of water management. Using modern techniques, transgenic crops with improved genetic characteristics were developed. Subsequently, the search for more sustainable techniques, such as integrated pest management, and less invasive techniques based on gene editing has begun. In this review, we propose the management of induced resistance as one of the major events that will impact agriculture.
Efforts should also be invested at the legislative level. Clear guidelines concerning the quality control and efficacy of marketed IR products must be established and harmonized across countries and continents. Implementing quality standards, for example, in terms of stability, inoculum quality in the case of microbes, efficacy, and shelf-life, is of utmost importance.
In the report, The future of crop protection in Europe (192), the European Union proposes IR as one of the options to support the sustainability of European farmers to work sustainably while securing food production, preserving biodiversity and the environment, and supporting farmers’ incomes. According to this report, the potential impacts of IR will be seen in crop yield, food security, and the competitiveness of EU farming. Similar initiatives are taking place in Asia and the United States (193).
Recently, it has also become apparent that there is a substantial added value for the application of IR technologies for improving the nutraceutical value of foods, which can confer medical or health benefits, for example, in obesity, metabolic syndrome, inflammation, diabetes, hypertension, cardiovascular diseases, and cancer, by increasing the levels of bioactive compounds in induced plants (115, 119, 194).
In summary, the elicitation of plant defenses can lead to the production of safe and high-quality food, which is an important societal issue facing high demand by both consumers and producers and, at the same time, reduce the possible negative effects of current agricultural practices on the environment.
Supplementary material
The Supplementary Material for this article can be found online at: https://www.frontiersin.org/articles/10.3389/fsci.2024.1407410/full#supplementary-material
Acknowledgments
We are grateful to our colleagues, commercial stakeholders, farmers, the International Organisation for Biological and Integrated Control Induced Resistance (IOBC IR) working group, and the European Plant Science Organisation (EPSO) for fruitful discussions and feedback.
Statements
Author contributions
VF: Conceptualization, Visualization, Writing – original draft, Writing – review & editing. TK: Conceptualization, Visualization, Writing – original draft, Writing – review & editing. BM-M: Conceptualization, Visualization, Writing – original draft, Writing – review & editing. MP: Conceptualization, Visualization, Writing – original draft, Writing – review & editing. CR: Conceptualization, Visualization, Writing – original draft, Writing – review & editing. JT: Conceptualization, Visualization, Writing – original draft, Writing – review & editing.
Data availability statement
The original contributions presented in the study are included in the article/Supplementary Material. Further inquiries can be directed to the corresponding author.
Funding
The author(s) declare financial support was received for the research, authorship, and/or publication of this article. We acknowledge funding of PDC2022-133600 and PID2021-124813OB from MCIN/AEI/50110001103 and ERDF, “Una manera de hacer Europa” to MP and VF, and the support of the “Cooperative Research Program for Agriculture Science & Technology Development (Project No. RS-2020-RD009221 and Project No. RS-2022-RD010288),” Rural Development Administration and KRIBB Initiative Program (KGM9942421), Republic of Korea to CR. Research activities by JT are supported by a grant from the United Kingdom Biotechnology and Biological Sciences Research Council (BB/W015250/1), and TK acknowledges funding from Bijzonder Onderzoeksfonds (UGent), VLAIO (Flanders Innovation & Entrepreneurship), and FWO (Research Foundation – Flanders). The funders were not involved in the study design, collection, analysis, interpretation of data, the writing of this article, or the decision to submit it for publication.
Conflict of interest
The authors declare that the research was conducted in the absence of any commercial or financial relationships that could be construed as a potential conflict of interest.
The authors declared that they were editorial board members of Frontiers, at the time of submission. This had no impact on the peer review process and the final decision.
Publisher’s note
All claims expressed in this article are solely those of the authors and do not necessarily represent those of their affiliated organizations, or those of the publisher, the editors and the reviewers. Any product that may be evaluated in this article, or claim that may be made by its manufacturer, is not guaranteed or endorsed by the publisher.
Footnotes
- ^ Abbreviations: C, cytosine; G, guanine; H, adenosine, cytosine, or thymine.
- ^ Communication made by D. Klessig to the authors within an anonymized peer review report.
References
1. Aktar MW, SenGupta D, Chowdhury A. Impact of pesticides use in agriculture: their benefits and hazards. Interdiscip Toxicol (2009) 2(1):1–12. doi: 10.2478/v10102-009-0001-7
2. Schmidt-Jeffris RA, Beers EH, Sater C. Meta-analysis and review of pesticide non-target effects on phytoseiids, key biological control agents. Pest Manag Sci (2021) 77(11):4848–62. doi: 10.1002/ps.6531
3. Tang FHM, Lenzen M, McBratney A, Maggi F. Risk of pesticide pollution at the global scale. Nat Geosci (2021) 14(4):206–10. doi: 10.1038/s41561-021-00712-5
4. Janousek WM, Douglas MR, Cannings S, Clément MA, Delphia CM, Everett JG, et al. Recent and future declines of a historically widespread pollinator linked to climate, land cover, and pesticides. Proc Natl Acad Sci USA (2023) 120(5):e2211223120. doi: 10.1073/pnas.2211223120
5. Wyckhuys KAG, Furlong MJ, Zhang W, Gc YD. Carbon benefits of enlisting nature for crop protection. Nat Food (2022) 3(5):299–301. doi: 10.1038/s43016-022-00510-1
6. European Commission. The European Green Deal [online] (2019). Available at: https://commission.europa.eu/strategy-and-policy/priorities-2019-2024/european-green-deal_en
7. Japanese Ministry of Agriculture, Forestry and Fisheries. MAFF [online] (2023). Available at: https://www.maff.go.jp/e/policies/env/env_policy/meadri.html
8. United States Department of Agriculture. USDA Takes Significant Steps to Build More Sustainable, Resilient and Inclusive Food Systems [Press Release No. 020621; online] (2021). Available at: https://www.usda.gov/media/press-releases/2021/09/23/usda-takes-significant-steps-build-more-sustainable-resilient-and
9. United Nations Sustainable Development Goals Summit. Press Release: The UN Secretary General convenes world leaders and key actors for UN Food Systems Summit +2 Stocktaking Moment in push to transform food systems and accelerate action for the SDGs [online] (2023). Available at: https://www.un.org/sustainabledevelopment/blog/2023/07/press-release-the-un-secretary-general-convenes-world-leaders-and-key-actors-for-un-food-systems-summit-2-stocktaking-moment-in-push-to-transform-food-systems-and-accelerate-action-for-the-sdgs/
10. Food and Agriculture Organization of the United Nations Regional Office for Asia and the Pacific. Progress in pesticide risk assessment and phasing-out of highly hazardous pesticides in Asia 2015. Bangkok: RAP Publication (2015). Available at: http://www.fao.org/3/a-i4362e.pdf
11. Jacobi J, Ottiger F, Kiteme BP, Delgado Burgoa JMF, Winkler MS, Lannen A. Making food systems safer: time to curb use of highly hazardous pesticides. CDE Policy Brief, No. 15. Bern: Centre for Development and Environment (2019). Available at: https://www.cde.unibe.ch/research/cde_series/policy_briefs/making_food_systems_safer_time_to_curb_use_of_highly_hazardous_pesticides/index_eng.html
12. Jacquet F, Jeuffroy MH, Jouan J, Le Cadre E, Litrico I, Malausa T, et al. Pesticide-free agriculture as a new paradigm for research. Agron Sustain Dev (2022) 42(1):8. doi: 10.1007/s13593-021-00742-8
13. Rinscheid A, Trencher G, Rosenbloom D. Phase-out as a policy approach to address sustainability challenges: a systematic review. Technol Decline (2022), 225–48. doi: 10.4324/9781003213642-10
14. De Kesel J, Conrath U, Flors V, Luna E, Mageroy MH, Mauch-Mani B, et al. The induced resistance lexicon: do’s and dont’s. Trends Plant Sci (2021) 26(7):685–91. doi: 10.1016/j.tplants.2021.01.001
15. Walters DR, Ratsep J, Havis ND. Controlling crop diseases using induced resistance: challenges for the future. J Exp Bot (2013) 64(5):1263–80. doi: 10.1093/jxb/ert026
16. Mauch–Mani B, Baccelli I, Luna E, Flors V. Defense priming: an adaptive part of induced resistance. Annu Rev Plant Biol (2017) 68:485–512. doi: 10.1146/annurev-arplant-042916-041132
17. Wilkinson SW, Magerøy MH, López Sánchez A, Smith LM, Furci L, Cotton TEA, et al. Surviving in a hostile world: plant strategies to resist pests and diseases. Annu Rev Phytopathol (2019) 57:505–29. doi: 10.1146/annurev-phyto-082718-095959
18. Jones JDG, Dangl JL. The plant immune system. Nature (2006) 444(7117):323–9. doi: 10.1038/nature05286
19. Yuan M, Ngou BPM, Ding P, Xin XF. PTI-ETI crosstalk: an integrative view of plant immunity. Curr Opin Plant Biol (2021) 62:102030. doi: 10.1016/j.pbi.2021.102030
20. Thordal-Christensen H. A holistic view on plant effector-triggered immunity presented as an iceberg model. Cell Mol Life Sci (2020) 77(20):3963–76. doi: 10.1007/s00018-020-03515-w
21. Marchal C, Michalopoulou VA, Zou Z, Cevik V, Sarris PF. Show me your ID: NLR immune receptors with integrated domains in plants. Essays Biochem (2022) 66(5):527–39. doi: 10.1042/EBC20210084
22. Wang J, Song W, Chai J. Structure, biochemical function, and signaling mechanism of plant NLRs. Mol Plant (2023) 16(1):75–95. doi: 10.1016/j.molp.2022.11.011
23. Prime-A-Plant Group, Conrath U, Beckers GJM, Flors V, García-Agustín P, Jakab G, et al. Priming: getting ready for battle. Mol Plant Microbe Interact (2006) 19(10):1062–71. doi: 10.1094/MPMI-19-1062
24. Martinez-Medina A, Flors V, Heil M, Mauch-Mani B, Pieterse CMJ, Pozo MJ, et al. Recognizing plant defense priming. Trends Plant Sci (2016) 21(10):818–22. doi: 10.1016/j.tplants.2016.07.009
25. Conrath U, Beckers GJM, Langenbach CJG, Jaskiewicz MR. Priming for enhanced defense. Annu Rev Phytopathol (2015) 53:97–119. doi: 10.1146/annurev-phyto-080614-120132
26. Hannan Parker A, Wilkinson SW, Ton J. Epigenetics: A catalyst of plant immunity against pathogens. New Phytol (2022) 233(1):66–83. doi: 10.1111/nph.17699
27. Luna E, Bruce TJA, Roberts MR, Flors V, Ton J. Next-generation systemic acquired resistance. Plant Physiol (2012) 158(2):844–53. doi: 10.1104/pp.111.187468
28. Rasmann S, De Vos M, Casteel CL, Tian D, Halitschke R, Sun JY, et al. Herbivory in the previous generation primes plants for enhanced insect resistance. Plant Physiol (2012) 158(2):854–63. doi: 10.1104/pp.111.187831
29. Slaughter A, Daniel X, Flors V, Luna E, Hohn B, Mauch-Mani B. Descendants of primed Arabidopsis plants exhibit resistance to biotic stress. Plant Physiol (2012) 158(2):835–43. doi: 10.1104/pp.111.191593
30. Stassen JHM, López A, Jain R, Pascual-Pardo D, Luna E, Smith LM, et al. The relationship between transgenerational acquired resistance and global DNA methylation in Arabidopsis. Sci Rep (2018) 8(1):14761. doi: 10.1038/s41598-018-32448-5
31. López Sánchez A, Pascual-Pardo D, Furci L, Roberts MR, Ton J. Costs and benefits of transgenerational induced resistance in Arabidopsis. Front Plant Sci (2021) 12:644999. doi: 10.3389/fpls.2021.644999
32. De Kesel J, Bonneure E, Frei M, De Meyer T, Mangelinckx S, Kyndt T. Diproline-induced resistance to parasitic nematodes in the same and subsequent rice generations: roles of iron, nitric oxide and ethylene. Front Plant Sci (2023) 14:1112007. doi: 10.3389/fpls.2023.1112007
33. Heuvelink E, Dicke M, Kierkels T. Pestered plants cry for help by emitting SOS scents: natural enemies of pests respond to plant’s signals. Greenhouses Int Mag Greenhouse Growers (2020) 9(4):32–33R. Available at: https://www.ingreenhouses.com/digimagazine/2020/5/magazine.php?spread=32
34. Zhou S, Jander G. Molecular ecology of plant volatiles in interactions with insect herbivores. J Exp Bot (2022) 73(2):449–62. doi: 10.1093/jxb/erab413
35. Papantoniou D, Chang D, Martínez-Medina A, van Dam NM, Weinhold A. Root symbionts alter herbivore-induced indirect defenses of tomato plants by enhancing predator attraction. Front Physiol (2022) 13:1003746. doi: 10.3389/fphys.2022.1003746
36. Rolfe SA, Griffiths J, Ton J. Crying out for help with root exudates: adaptive mechanisms by which stressed plants assemble health-promoting soil microbiomes. Curr Opin Microbiol (2019) 49:73–82. doi: 10.1016/j.mib.2019.10.003
37. Song GC, Jeon JS, Sim HJ, Lee S, Jung J, Kim SG, et al. Dual functionality of natural mixtures of bacterial volatile compounds on plant growth. J Exp Bot (2022) 73(2):571–83. doi: 10.1093/jxb/erab466
38. Reglinski T, Havis N, Rees HJ, de Jong H. The practical role of induced resistance for crop protection. Phytopathology (2023) 113(4):719–31. doi: 10.1094/PHYTO-10-22-0400-IA
39. Chester KS. The problem of acquired physiological immunity in plants. Q Rev Biol (1933) 8(3):275–324. doi: 10.1086/394440
40. Hammerschmidt R, Yang-Cashman P. Induced resistance in Cucurbits. In: Hammerschmidt R, Kuć J, editors. Induced resistance to disease in plants. Dordrecht: Springer Netherlands (1995) 63–85.
41. White RF. Acetylsalicylic acid (aspirin) induces resistance to tobacco mosaic virus in tobacco. Virology (1979) 99(2):410–2. doi: 10.1016/0042-6822(79)90019-9
42. Métraux JP, Signer H, Ryals J, Ward E, Wyss-Benz M, Gaudin J, et al. Increase in salicylic acid at the onset of systemic acquired resistance in cucumber. Science (1990) 250(4983):1004–6. doi: 10.1126/science.250.4983.1004
43. Malamy J, Carr JP, Klessig DF, Raskin I. Salicylic acid: a likely endogenous signal in the resistance response of tobacco to viral infection. Science (1990) 250(4983):1002–4. doi: 10.1126/science.250.4983.1002
44. Métraux JP, Ahlgoy P, Staub TH, Speich J, Steinemann A, Ryals J, et al. Induced systemic resistance in cucumber in response to 2, 6-dichloro-isonicotinic acid and pathogens. In: Hennecke H, Verma DPS, editors. Advances in molecular genetics of plant-microbe interactions, Vol.1. Dordrecht: Springer Netherlands (1991). 1:432–9. doi: 10.1007/978-94-015-7934-6_66
45. Görlach J, Volrath S, Knauf-Beiter G, Hengy G, Beckhove U, Kogel KH, et al. Benzothiadiazole, a novel class of inducers of systemic acquired resistance, activates gene expression and disease resistance in wheat. Plant Cell (1996) 8(4):629–43. doi: 10.1105/tpc.8.4.629
46. Iwata M. Probenazole—a plant defence activator. Pest Outlook (2001) 12(1):28–31. doi: 10.1039/b100805f
47. Gaffney T, Friedrich L, Vernooij B, Negrotto D, Nye G, Uknes S, et al. Requirement of salicylic acid for the induction of systemic acquired resistance. Science (1993) 261(5122):754–6. doi: 10.1126/science.261.5122.754
48. Cao H, Bowling SA, Gordon AS, Dong X. Characterization of an Arabidopsis mutant that is nonresponsive to inducers of systemic acquired resistance. Plant Cell (1994) 6(11):1583–92. doi: 10.1105/tpc.6.11.1583
49. Delaney TP, Friedrich L, Ryals JA. Arabidopsis signal transduction mutant defective in chemically and biologically induced disease resistance. Proc Natl Acad Sci USA (1995) 92(14):6602–6. doi: 10.1073/pnas.92.14.6602
50. Reymond P, Farmer EE. Jasmonate and salicylate as global signals for defense gene expression. Curr Opin Plant Biol (1998) 1(5):404–11. doi: 10.1016/s1369-5266(98)80264-1
51. Thomma BP, Eggermont K, Penninckx IA, Mauch-Mani B, Vogelsang R, Cammue BP, et al. Separate jasmonate-dependent and salicylate-dependent defense-response pathways in Arabidopsis are essential for resistance to distinct microbial pathogens. Proc Natl Acad Sci USA (1998) 95(25):15107–11. doi: 10.1073/pnas.95.25.15107
52. Shi R, Yu J, Chang X, Qiao L, Liu X, Lu L. Recent advances in research into jasmonate biosynthesis and signaling pathways in agricultural crops and products. Processes (2023) 11(3):736. doi: 10.3390/pr11030736
53. Park SW, Kaimoyo E, Kumar D, Mosher S, Klessig DF. Methyl salicylate is a critical mobile signal for plant systemic acquired resistance. Science (2007) 318(5847):113–6. doi: 10.1126/science.1147113
54. Shah J, Zeier J. Long-distance communication and signal amplification in systemic acquired resistance. Front Plant Sci (2013) 4:30. doi: 10.3389/fpls.2013.00030
55. Chanda B, Xia YE, Mandal MK, Yu K, Sekine KT, Gao QM, et al. Glycerol-3-phosphate is a critical mobile inducer of systemic immunity in plants. Nat Genet (2011) 43(5):421–7. doi: 10.1038/ng.798
56. Chaturvedi R, Venables B, Petros RA, Nalam V, Li M, Wang X, et al. An abietane diterpenoid is a potent activator of systemic acquired resistance. Plant J (2012) 71(1):161–72. doi: 10.1111/j.1365-313X.2012.04981.x
57. Chen YC, Holmes EC, Rajniak J, Kim JG, Tang S, Fischer CR, et al. N-hydroxy-pipecolic acid is a mobile metabolite that induces systemic disease resistance in Arabidopsis. Proc Natl Acad Sci USA (2018) 115(21):E4920–9. doi: 10.1073/pnas.1805291115
58. Mousavi SAR, Chauvin A, Pascaud F, Kellenberger S, Farmer EE. Glutamate receptor-like genes mediate leaf-to-leaf wound signalling. Nature (2013) 500(7463):422–6. doi: 10.1038/nature12478
59. Xu G, Yuan M, Ai C, Liu L, Zhuang E, Karapetyan S, et al. uORF-mediated translation allows engineered plant disease resistance without fitness costs. Nature (2017) 545(7655):491–4. doi: 10.1038/nature22372
60. Furci L, Jain R, Stassen J, Berkowitz O, Whelan J, Roquis D, et al. Identification and characterisation of hypomethylated DNA loci controlling quantitative resistance in Arabidopsis. eLife (2019) 8:e40655. doi: 10.7554/eLife.40655
61. Van Peer R, Punte HLM, de Weger LA, Schippers B. Characterization of root surface and endorhizosphere pseudomonads in relation to their colonization of roots. Appl Environ Microbiol (1990) 56(8):2462–70. doi: 10.1128/aem.56.8.2462-2470.1990
62. Wei G, Kloepper JW, Tuzun S. Induction of systemic resistance of cucumber to Colletotrichum orbiculare by select strains of plant growth-promoting rhizobacteria. Phytopathology (1991) 81(12):1508–12. doi: 10.1094/Phyto-81-1508
63. Alström S. Induction of disease resistance in common bean susceptible to halo blight bacterial pathogen after seed bacterization with rhizosphere pseudomonads. J Gen Appl Microbiol (1991) 37(6):495–501. doi: 10.2323/jgam.37.495
64. Pieterse CM, Van Wees SC, Hoffland E, Van Pelt JA, Van Loon LC. Systemic resistance in Arabidopsis induced by biocontrol bacteria is independent of salicylic acid accumulation and pathogenesis-related gene expression. Plant Cell (1996) 8(8):1225–37. doi: 10.1105/tpc.8.8.1225
65. Pieterse CM, Van Wees SC, Van Pelt JA, Knoester M, Laan R, Gerrits H, et al. A novel signaling pathway controlling induced systemic resistance in Arabidopsis. Plant Cell (1998) 10(9):1571–80. doi: 10.1105/tpc.10.9.1571
66. Pieterse CMJ, Zamioudis C, Berendsen RL, Weller DM, Van Wees SCM, Bakker PAHM. Induced systemic resistance by beneficial microbes. Annu Rev Phytopathol (2014) 52:347–75. doi: 10.1146/annurev-phyto-082712-102340
67. Pozo MJ, Azcón-Aguilar C. Unraveling mycorrhiza-induced resistance. Curr Opin Plant Biol (2007) 10(4):393–8. doi: 10.1016/j.pbi.2007.05.004
68. Liu H, Brettell LE, Qiu Z, Singh BK. Microbiome-mediated stress resistance in plants. Trends Plant Sci (2020) 25(8):733–43. doi: 10.1016/j.tplants.2020.03.014
69. Lee SM, Kong HG, Song GC, Ryu CM. Disruption of Firmicutes and Actinobacteria abundance in tomato rhizosphere causes the incidence of bacterial wilt disease. ISME J (2021) 15(1):330–47. doi: 10.1038/s41396-020-00785-x
70. Van Peer R, Niemann GJ, Schippers B. Induced resistance and phytoalexin accumulation in biological control of Fusarium wilt of carnation by Pseudomonas sp. strain WCS417r. Phytopathology (1991) 81(7):728–34. doi: 10.1094/Phyto-81-728
71. Minchev Z, Kostenko O, Soler R, Pozo MJ. Microbial consortia for effective biocontrol of root and foliar diseases in tomato. Front Plant Sci (2021) 12:756368. doi: 10.3389/fpls.2021.756368
72. Van Wees SCM, Ent S, Pieterse CMJ. Plant immune responses triggered by beneficial microbes. Curr Opin Plant Biol (2008) 11(4):443–8. doi: 10.1016/j.pbi.2008.05.005
73. Martínez-Medina A, Fernandez I, Lok GB, Pozo MJ, Pieterse CMJ, Van Wees SCM. Shifting from priming of salicylic acid- to jasmonic acid-regulated defences by Trichoderma protects tomato against the root knot nematode meloidogyne incognita. New Phytol (2017) 213(3):1363–77. doi: 10.1111/nph.14251
74. Rivero J, Lidoy J, Llopis-Giménez Á, Herrero S, Flors V, Pozo MJ. Mycorrhizal symbiosis primes the accumulation of antiherbivore compounds and enhances herbivore mortality in tomato. J Exp Bot (2021) 72(13):5038–50. doi: 10.1093/jxb/erab171
75. Zhou L, Song C, Li Z, Kuipers OP. Antimicrobial activity screening of rhizosphere soil bacteria from tomato and genome-based analysis of their antimicrobial biosynthetic potential. BMC Genomics (2021) 22(1):29. doi: 10.1186/s12864-020-07346-8
76. Eck JL, Kytöviita MM, Laine AL. Arbuscular mycorrhizal fungi influence host infection during epidemics in a wild plant pathosystem. New Phytol (2022) 236(5):1922–35. doi: 10.1111/nph.18481
77. Chaudhary P, Singh S, Chaudhary A, Sharma A, Kumar G. Overview of biofertilizers in crop production and stress management for sustainable agriculture. Front Plant Sci (2022) 13:930340. doi: 10.3389/fpls.2022.930340
78. Kloepper JW, Ryu CM, Zhang S. Induced systemic resistance and promotion of plant growth by Bacillus spp. Phytopathology (2004) 94(11):1259–66. doi: 10.1094/PHYTO.2004.94.11.1259
79. Maldonado Galdeano C, Cazorla SI, Lemme Dumit JM, Vélez E, Perdigón G. Beneficial effects of probiotic consumption on the immune system. Ann Nutr Metab (2019) 74(2):115–24. doi: 10.1159/000496426
80. Liu Y, Wang J, Wu C. Modulation of gut microbiota and immune system by probiotics, pre-biotics, and post-biotics. Front Nutr (2021) 8:634897. doi: 10.3389/fnut.2021.634897
81. Zhu L, Huang J, Lu X, Zhou C. Development of plant systemic resistance by beneficial rhizobacteria: recognition, initiation, elicitation and regulation. Front Plant Sci (2022) 13:952397. doi: 10.3389/fpls.2022.952397
82. Abhilash PC, Dubey RK, Tripathi V, Gupta VK, Singh HB. Plant growth-promoting microorganisms for environmental sustainability. Trends Biotechnol (2016) 34(11):847–50. doi: 10.1016/j.tibtech.2016.05.005
83. Alori ET, Babalola OO. Microbial inoculants for improving crop quality and human health in Africa. Front Microbiol (2018) 9:2213. doi: 10.3389/fmicb.2018.02213
84. Van Oosten MJ, Pepe O, De Pascale S, Silletti S, Maggio A. The role of biostimulants and bioeffectors as alleviators of abiotic stress in crop plants. Chem Biol Technol Agric (2017) 4:1–2. doi: 10.1186/s40538-017-0089-5
85. Pozo MJ, Zabalgogeazcoa I, Vazquez de Aldana BR, Martinez-Medina A. Untapping the potential of plant mycobiomes for applications in agriculture. Curr Opin Plant Biol (2021) 60:102034. doi: 10.1016/j.pbi.2021.102034
86. Hamid B, Zaman M, Farooq S, Fatima S, Sayyed RZ, Baba ZA, et al. Bacterial plant biostimulants: a sustainable way towards improving growth, productivity, and health of crops. Sustainability (2021) 13(5):2856. doi: 10.3390/su13052856
87. Lee Díaz AS, Macheda D, Saha H, Ploll U, Orine D, Biere A. Tackling the context-dependency of microbial-induced resistance. Agronomy (2021) 11(7):1293. doi: 10.3390/agronomy11071293
88. Sanjuán J, Nápoles MC, Pérez-Mendoza D, Lorite MJ, Rodríguez-Navarro DN. Microbials for agriculture: why do they call them biostimulants when they mean probiotics? Microorganisms (2023) 11(1):153. doi: 10.3390/microorganisms11010153
89. Pršić J, Ongena M. Elicitors of plant immunity triggered by beneficial bacteria. Front Plant Sci (2020) 11:594530. doi: 10.3389/fpls.2020.594530
90. Erbs G, Newman MA. The role of lipopolysaccharide and peptidoglycan, two glycosylated bacterial microbe-associated molecular patterns (MAMPs), in plant innate immunity. Mol Plant Pathol (2012) 13(1):95–104. doi: 10.1111/j.1364-3703.2011.00730.x
91. Guo J, Cheng Y. Advances in fungal elicitor-triggered plant immunity. Int J Mol Sci (2022) 23(19):12003. doi: 10.3390/ijms231912003
92. Sharifi R, Ryu CM. Sniffing bacterial volatile compounds for healthier plants. Curr Opin Plant Biol (2018) 44:88–97. doi: 10.1016/j.pbi.2018.03.004
93. Pescador L, Fernandez I, Pozo MJ, Romero-Puertas MC, Pieterse CMJ, Martinez-Medina A. Nitric oxide signalling in the root is required for MYB72-dependent disease systemic resistance induced by Trichoderma volatiles in Arabidopsis. J Exp Bot (2022) 73(2):584–95. doi: 10.1093/jxb/erab294
94. Dicke M, Sabelis MW. How plants obtain predatory mites as bodyguards. Neth J Zool (1988) 38(2–4):148–65. doi: 10.1163/156854288X00111
95. Turlings TC, Tumlinson JH, Lewis WJ. Exploitation of herbivore-induced plant odors by host-seeking parasitic wasps. Science (1990) 250(4985):1251–3. doi: 10.1126/science.250.4985.1251
96. Mattiacci L, Dicke M, Posthumus MA. Induction of parasitoid attracting synomone in brussels sprouts plants by feeding of Pieris brassicae larvae: role of mechanical damage and herbivore elicitor. J Chem Ecol (1994) 20(9):2229–47. doi: 10.1007/BF02033199
97. Felton GW, Tumlinson JH. Plant-insect dialogs: complex interactions at the plant-insect interface. Curr Opin Plant Biol (2008) 11(4):457–63. doi: 10.1016/j.pbi.2008.07.001
98. Rasmann S, Köllner TG, Degenhardt J, Hiltpold I, Toepfer S, Kuhlmann U, et al. Recruitment of entomopathogenic nematodes by insect-damaged maize roots. Nature (2005) 434(7034):732–7. doi: 10.1038/nature03451
99. Kappers IF, Aharoni A, Van Herpen TWJM, Luckerhoff LLP, Dicke M, Bouwmeester HJ. Genetic engineering of terpenoid metabolism attracts bodyguards to Arabidopsis. Science (2005) 309(5743):2070–2. doi: 10.1126/science.1116232
100. Schnee C, Köllner TG, Held M, Turlings TCJ, Gershenzon J, Degenhardt J. The products of a single maize sesquiterpene synthase form a volatile defense signal that attracts natural enemies of maize herbivores. Proc Natl Acad Sci USA (2006) 103(4):1129–34. doi: 10.1073/pnas.0508027103
101. Rudrappa T, Czymmek KJ, Paré PW, Bais HP. Root-secreted Malic acid recruits beneficial soil bacteria. Plant Physiol (2008) 148(3):1547–56. doi: 10.1104/pp.108.127613
102. Neal AL, Ahmad S, Gordon-Weeks R, Ton J. Benzoxazinoids in root exudates of maize attract Pseudomonas putida to the rhizosphere. PloS One (2012) 7(4):e35498. doi: 10.1371/journal.pone.0035498
103. Neal AL, Ton J. Systemic defense priming by Pseudomonas putida KT2440 in maize depends on benzoxazinoid exudation from the roots. Plant Signal Behav (2013) 8(1):e22655. doi: 10.4161/psb.22655
104. Berendsen RL, Vismans G, Yu K, Song Y, de Jonge R, Burgman WP, et al. Disease-induced assemblage of a plant-beneficial bacterial consortium. ISME J PA: Bakker (2018) 12(6):1496–507. doi: 10.1038/s41396-018-0093-1
105. Yuan J, Zhao J, Wen T, Zhao M, Li R, Goossens P, et al. Root exudates drive the soil-borne legacy of aboveground pathogen infection. Microbiome (2018) 6(1):156. doi: 10.1186/s40168-018-0537-x
106. Cook RJ, Rovira AD. The role of bacteria in the biological control of Gaeumannomyces graminis by suppressive soils. Soil Biol Biochem (1976) 8(4):269–73. doi: 10.1016/0038-0717(76)90056-0
107. Manosalva P, Manohar M, Von Reuss SH, Chen S, Koch A, Kaplan F, et al. Conserved nematode signalling molecules elicit plant defenses and pathogen resistance. Nat Commun (2015) 6:7795. doi: 10.1038/ncomms8795
108. Klessig DF, Manohar M, Baby S, Koch A, Danquah WB, Luna E, et al. Nematode ascaroside enhances resistance in a broad spectrum of plant-pathogen systems. J Phytopathol (2019) 167(5):265–72. doi: 10.1111/jph.12795
109. Huang L, Yuan Y, Lewis C, Kud J, Kuhl JC, Caplan A, et al. NILR1 perceives a nematode ascaroside triggering immune signaling and resistance. Curr Biol (2023) 33(18):3992–97.e3. doi: 10.1016/j.cub.2023.08.017
110. Erb M, Reymond P. Molecular interactions between plants and insect herbivores. Annu Rev Plant Biol (2019) 70:527–57. doi: 10.1146/annurev-arplant-050718-095910
111. Ghozlan MH, EL-Argawy EA, Tokgöz S, Lakshman DK, Mitra A. Plant defense against necrotrophic pathogens. Am J Plant Sci (2020) 11(12):2122–38. doi: 10.4236/ajps.2020.1112149
112. Hawkins NJ, Bass C, Dixon A, Neve P. The evolutionary origins of pesticide resistance. Biol Rev Camb Philos Soc (2019) 94(1):135–55. doi: 10.1111/brv.12440
113. Baenas N, García-Viguera C, Moreno DA. Elicitation: a tool for enriching the bioactive composition of foods. Molecules (2014) 19(9):13541–63. doi: 10.3390/molecules190913541
114. Nuñez-Gómez V, Baenas N, Navarro-González I, García-Alonso J, Moreno DA, González-Barrio R, et al. Seasonal variation of health-promoting bioactives in broccoli and methyl-jasmonate pre-harvest treatments to enhance their contents. Foods (2020) 9:1371. doi: 10.3390/foods9101371
115. Giovannetti M, Avio L, Barale R, Ceccarelli N, Cristofani R, Iezzi A, et al. Nutraceutical value and safety of tomato fruits produced by mycorrhizal plants. Br J Nutr (2012) 107(2):242–51. doi: 10.1017/S000711451100290X
116. Giovannetti M, Avio L, Sbrana C. Improvement of nutraceutical value of food by plant symbionts. In: Ramawat K, Mérillon JM, editors. Natural products. Berlin: Springer-Verlag (2013). 2641–62. doi: 10.1007/978-3-642-22144-6_187
117. Schubert R, Werner S, Cirka H, Rödel P, Tandron Moya Y, Mock HP, et al. Effects of arbuscular mycorrhization on fruit quality in industrialized tomato production. Int J Mol Sci (2020) 21(19):7029. doi: 10.3390/ijms21197029
118. Galicia-Campos E, García-Villaraco A, Montero-Palmero MB, Gutiérrez-Mañero FJ, Ramos-Solano B. Bacillus H47 triggers Olea europaea metabolism activating DOXP and shikimate pathways simultaneously and modifying leaf extracts’ antihypertensive activity. Front Microbiol (2022) 13:1005865. doi: 10.3389/fmicb.2022.1005865
119. Gutierrez-Albanchez E, Kirakosyan A, Bolling SF, García-Villaraco A, Gutierrez-Mañero J, Ramos-Solano B. Biotic elicitation as a tool to improve strawberry and raspberry extract potential on metabolic syndrome-related enzymes in vitro. J Sci Food Agric (2019) 99(6):2939–46. doi: 10.1002/jsfa.9507
120. Leadbeater A, Staub T. Exploitation of induced resistance: a commercial perspective. In: Walters D, Newton A, Lyon G, editors. Induced resistance for plant defence: a sustainable approach to crop protection. (2nd edition). Chichester: John Wiley & Sons (2014). 300–15. doi: 10.1002/9781118371848.ch13
121. Liljeroth E, Bengtsson T, Wiik L, Andreasson E. Induced resistance in potato to Phytphthora infestans—effects of BABA in greenhouse and field tests with different potato varieties. Eur J Plant Pathol (2010) 127(2):171–83. doi: 10.1007/s10658-010-9582-4
122. Liljeroth E, Lankinen Å, Wiik L, Burra DD, Alexandersson E, Andreasson E. Potassium phosphite combined with reduced doses of fungicides provides efficient protection against potato late blight in large-scale field trials. Crop Prot (2016) 86:42–55. doi: 10.1016/j.cropro.2016.04.003
123. Mulugeta T, Abreha K, Tekie H, Mulatu B, Yesuf M, Andreasson E, et al. Phosphite protects against potato and tomato late blight in tropical climates and has varying toxicity depending on the Phytophthora infestans isolate. Crop Prot (2019) 121:139–46. doi: 10.1016/j.cropro.2019.03.019
124. Yi HS, Yang JW, Ryu CM. ISR meets SAR outside: additive action of the endophyte Bacillus pumilus INR7 and the chemical inducer, benzothiadiazole, on induced resistance against bacterial spot in field-grown pepper. Front Plant Sci (2013) 4:122. doi: 10.3389/fpls.2013.00122
125. van Hulten M, Pelser M, Van Loon LC, Pieterse CMJ, Ton J. Costs and benefits of priming for defense in Arabidopsis. Proc Natl Acad Sci USA (2006) 103(14):5602–7. doi: 10.1073/pnas.0510213103
126. Romero AM, Kousik CS, Ritchie DF. Resistance to bacterial spot in bell pepper induced by acibenzolar-s-methyl. Plant Dis (2001) 85(2):189–94. doi: 10.1094/PDIS.2001.85.2.189
127. Prats E, Rubiales D, JorrÍn J. Acibenzolar- S -methyl-induced resistance to sunflower rust (Puccinia helianthi) is associated with an enhancement of coumarins on foliar surface. Physiol Mol Plant Pathol (2002) 60(3):155–62. doi: 10.1006/pmpp.2002.0385
128. Oostendorp M, Kunz W, Dietrich B, Staub T. Induced disease resistance in plants by chemicals. Eur J Plant Pathol (2001) 107(1):19–28. doi: 10.1023/A:1008760518772
129. Wu S, Blackburn K, Amburgey J, Jaworska J, Federle T. A framework for using structural, reactivity, metabolic and physicochemical similarity to evaluate the suitability of analogs for SAR-based toxicological assessments. Regul Toxicol Pharmacol (2010) 56(1):67–81. doi: 10.1016/j.yrtph.2009.09.006
130. Wilkinson SW, Hannan Parker A, Muench A, Wilson RS, Hooshmand K, Henderson MA, et al. Long-lasting memory of jasmonic acid-dependent immunity requires DNA demethylation and ARGONAUTE1. Nat Plants (2023) 9(1):81–95. doi: 10.1038/s41477-022-01313-9
131. Gautam H, Singh S, Prashad H, Kumar A, Choudhary A, Kaur H, et al. Pesticide toxicity and their impact on plant growth, active constituents and productivity. In: Husen A, editor. Plants and their interaction to environmental pollution. Amsterdam: Elsevier (2023). 231–52. doi: 10.1016/B978-0-323-99978-6.00008-X
132. Walters DR, Fountaine JM. Practical application of induced resistance to plant diseases: an appraisal of effectiveness under field conditions. J Agric Sci (2009) 147(5):523–35. doi: 10.1017/S0021859609008806
133. Chavan SN, Tumpa FH, Khokon MAR, Kyndt T. Potential of exogenous treatment with dehydroascorbate to control root-knot nematode infection in rice. Rice (N Y) (2023) 16(1):29. doi: 10.1186/s12284-023-00644-1
134. Balmer A, Pastor V, Glauser G, Mauch-Mani B. Tricarboxylates induce defense priming against bacteria in Arabidopsis thaliana. Front Plant Sci (2018) 9:1221. doi: 10.3389/fpls.2018.01221
135. Zhang Y, Fernie AR. On the role of the tricarboxylic acid cycle in plant productivity. J Integr Plant Biol (2018) 60(12):1199–216. doi: 10.1111/jipb.12690
136. Santoyo G. How plants recruit their microbiome? New insights into beneficial interactions. J Adv Res (2022) 40:45–58. doi: 10.1016/j.jare.2021.11.020
137. Van Wees SC, De Swart EA, Van Pelt JA, Van Loon LC, Pieterse CM. Enhancement of induced disease resistance by simultaneous activation of salicylate- and jasmonate-dependent defense pathways in Arabidopsis thaliana. Proc Natl Acad Sci USA (2000) 97(15):9711–16. doi: 10.1073/pnas.130425197
138. Reuveni M, Zahavi T, Cohen Y. Controlling downy mildew (Plasmopara viticola) in field-grown grapevine with β-aminobutyric acid (BABA). Phytoparasitica (2001) 29(2):125–33. doi: 10.1007/BF02983956
139. Singh UB, Malviya D, Singh S, Kumar M, Sahu PK, Singh HV, et al. Trichoderma harzianum- and methyl jasmonate-induced resistance to Bipolaris sorokiniana through enhanced phenylpropanoid activities in bread wheat (Triticum aestivum L.). Front Microbiol (2019) 10:1697. doi: 10.3389/fmicb.2019.01697
140. Abd El-Rahman SS, Mohamed HI. Application of benzothiadiazole and Trichoderma harzianum to control faba bean chocolate spot disease and their effect on some physiological and biochemical traits. Acta Physiol Plant (2014) 36(2):343–54. doi: 10.1007/s11738-013-1416-5
141. Walters DR, Paterson L, Walsh DJ, Havis ND. Priming for plant defense in barley provides benefits only under high disease pressure. Physiol Mol Plant Pathol (2008) 73(4–5):95–100. doi: 10.1016/j.pmpp.2009.03.002
142. Sharma K, Butz AF, Finckh MR. Effects of host and pathogen genotypes on inducibility of resistance in tomato (Solanum lycopersicum) to Phytophthora infestans. Plant Pathol (2010) 59(6):1062–71. doi: 10.1111/j.1365-3059.2010.02341.x
143. Dann E, Diers B, Byrum J, Hammerschmidt R. Effect of treating soybean with 2, 6-dichloroisonicotinic acid (INA) and benzothiadiazole (BTH) on seed yields and the level of disease caused by Sclerotinia sclerotiorum in field and greenhouse studies. Eur J Plant Pathol (1998) 104(3):271–8. doi: 10.1023/A:1008683316629
144. Hijwegen T, Verhaar MA. Effects of cucumber genotype on the induction of resistance to powdery mildew, Sphaerotheca fuliginea, by 2, 6-dichloroisonicotinic acid. Plant Pathol (1995) 44(4):756–62. doi: 10.1111/j.1365-3059.1995.tb01700.x
145. Walters DR, Havis ND, Sablou C, Walsh DJ. Possible trade-off associated with the use of a combination of resistance elicitors. Physiol Mol Plant Pathol (2011) 75(4):188–92. doi: 10.1016/j.pmpp.2011.02.001
146. Samain E, Aussenac T, Selim S. The effect of plant genotype, growth stage, and Mycosphaerella graminicola strains on the efficiency and durability of wheat-induced resistance by Paenibacillus sp. strain B2. Front Plant Sci (2019) 10:587. doi: 10.3389/fpls.2019.00587
147. Mora-Romero GA, Cervantes-Gámez RG, Galindo-Flores H, González-Ortíz MA, Félix-Gastélum R, Maldonado-Mendoza IE, et al. Mycorrhiza-induced protection against pathogens is both genotype-specific and graft-transmissible. Symbiosis (2015) 66(2):55–64. doi: 10.1007/s13199-015-0334-2
148. Pagliarani C, Moine A, Chitarra W, Meloni GR, Abbà S, Nerva L, et al. The molecular priming of defense responses is differently regulated in grapevine genotypes following elicitor application against powdery mildew. Int J Mol Sci (2020) 21(18):6776. doi: 10.3390/ijms21186776
149. Lankinen Å, Abreha KB, Masini L, Ali A, Resjö S, Andreasson E. Plant immunity in natural populations and agricultural fields: low presence of pathogenesis-related proteins in Solanum leaves. PloS One (2018) 13(11):e0207253. doi: 10.1371/journal.pone.0207253
150. Luna E, López A, Kooiman J, Ton J. Role of NPR1 and KYP in long-lasting induced resistance by β-aminobutyric acid. Front Plant Sci (2014) 5:184. doi: 10.3389/fpls.2014.00184
151. Furci L, Pascual-Pardo D, Tirot L, Zhang P, Hannan Parker A, Ton J. Heritable induced resistance in Arabidopsis thaliana: tips and tools to improve effect size and reproducibility. Plant Direct (2023) 7(8):e523. doi: 10.1002/pld3.523
152. Leisner CP, Potnis N, Sanz-Saez A. Crosstalk and trade-offs: plant responses to climate change-associated abiotic and biotic stresses. Plant Cell Environ (2023) 46(10):2946–63. doi: 10.1111/pce.14532
153. Huot B, Castroverde CDM, Velásquez AC, Hubbard E, Pulman JA, Yao J, et al. Dual impact of elevated temperature on plant defence and bacterial virulence in Arabidopsis. Nat Commun (2017) 8(1):1808. doi: 10.1038/s41467-017-01674-2
154. Bidzinski P, Ballini E, Ducasse A, Michel C, Zuluaga P, Genga A, et al. Transcriptional basis of drought-induced susceptibility to the rice blast fungus Magnaporthe oryzae. Front Plant Sci (2016) 7:1558. doi: 10.3389/fpls.2016.01558
155. Ryu M, Mishra RC, Jeon J, Lee SK, Bae H. Drought-induced susceptibility for Cenangium ferruginosum leads to progression of Cenangium-dieback disease in Pinus koraiensis. Sci Rep (2018) 8(1):16368. doi: 10.1038/s41598-018-34318-6
156. Ton J, Flors V, Mauch-Mani B. The multifaceted role of ABA in disease resistance. Trends Plant Sci (2009) 14(6):310–7. doi: 10.1016/j.tplants.2009.03.006
157. Zarattini M, Farjad M, Launay A, Cannella D, Soulié MC, Bernacchia G, et al. Every cloud has a silver lining: how abiotic stresses affect gene expression in plant-pathogen interactions. J Exp Bot (2021) 72(4):1020–33. doi: 10.1093/jxb/eraa531
158. Sanchez-Bel P, Troncho P, Gamir J, Pozo MJ, Camañes G, Cerezo M, et al. The nitrogen availability interferes with mycorrhiza-induced resistance against Botrytis cinerea in tomato. Front Microbiol (2016) 7:1598. doi: 10.3389/fmicb.2016.01598
159. Sánchez-Bel P, Sanmartín N, Pastor V, Mateu D, Cerezo M, Vidal-Albalat A, et al. Mycorrhizal tomato plants fine tunes the growth-defence balance upon N depleted root environments. Plant Cell Environ (2018) 41(2):406–20. doi: 10.1111/pce.13105
160. Dejana L, Ramírez-Serrano B, Rivero J, Gamir J, López-Ráez JA, Pozo MJ. Phosphorus availability drives mycorrhiza induced resistance in tomato. Front Plant Sci (2022) 13:1060926. doi: 10.3389/fpls.2022.1060926
161. Romera FJ, García MJ, Lucena C, Martínez-Medina A, Aparicio MA, Ramos J, et al. Induced systemic resistance (ISR) and Fe deficiency responses in dicot plants. Front Plant Sci (2019) 10:287. doi: 10.3389/fpls.2019.00287
162. Koen E, Trapet P, Brulé D, Kulik A, Klinguer A, Atauri-Miranda L, et al. β-aminobutyric acid (BABA)-induced resistance in Arabidopsis thaliana: link with iron homeostasis. Mol Plant Microbe Interact (2014) 27(11):1226–40. doi: 10.1094/MPMI-05-14-0142-R
163. Stringlis IA, Yu K, Feussner K, de Jonge R, Van Bentum S, Van Verk MC, et al. MYB72-dependent coumarin exudation shapes root microbiome assembly to promote plant health. Proc Natl Acad Sci USA (2018) 115(22):E5213–22. doi: 10.1073/pnas.1722335115
164. Agut B, Pastor V, Jaques JA, Flors V. Can plant defence mechanisms provide new approaches for the sustainable control of the two-spotted spider mite Tetranychus urticae? Int J Mol Sci (2018) 19(2):614. doi: 10.3390/ijms19020614
165. Heeb L, Jenner E, Cock MJW. Climate-smart pest management: building resilience of farms and landscapes to changing pest threats. J Pest Sci (2019) 92(3):951–69. doi: 10.1007/s10340-019-01083-y
166. Brodeur J, Abram PK, Heimpel GE, Messing RH. Trends in biological control: public interest, international networking and research direction. BioControl (2018) 63(1):11–26. doi: 10.1007/s10526-017-9850-8
167. Iriti M, Vitalini S. Plant immunity and crop yield: a sustainable approach in agri-food systems. Vaccines (2021) 9(2):121. doi: 10.3390/vaccines9020121
168. Tortorici S, Biondi A, Pérez-Hedo M, Larbat R, Zappalà L. Plant defences for enhanced integrated pest management in tomato. Ann Appl Biol (2022) 180(3):328–37. doi: 10.1111/aab.12750
169. Gruden K, Lidoy J, Petek M, Podpečan V, Flors V, Papadopoulou KK, et al. Ménage à Trois: unraveling the mechanisms regulating plant-microbe-arthropod interactions. Trends Plant Sci (2020) 25(12):1215–26. doi: 10.1016/j.tplants.2020.07.008
170. Engelberth J, Alborn HT, Schmelz EA, Tumlinson JH. Airborne signals prime plants against insect herbivore attack. Proc Natl Acad Sci USA (2004) 101(6):1781–5. doi: 10.1073/pnas.0308037100
171. Frost CJ, Mescher MC, Carlson JE, De Moraes CM. Plant defense priming against herbivores: getting ready for a different battle. Plant Physiol (2008) 146(3):818–24. doi: 10.1104/pp.107.113027
172. Kaur J, Chavana J, Soti P, Racelis A, Kariyat R. Arbuscular mycorrhizal fungi (AMF) influences growth and insect community dynamics in Sorghum-Sudan grass (Sorghum x drummondii). Arthropod Plant Interact (2020) 14(3):301–15. doi: 10.1007/s11829-020-09747-8
173. Cruz-Miralles J, Cabedo-López M, Guzzo M, Pérez-Hedo M, Flors V, Jaques JA. Plant defense responses triggered by phytoseiid predatory mites (Mesostigmata: Phytoseiidae) are species-specific, depend on plant genotype and may not be related to direct plant feeding. BioControl (2021) 66(3):381–94. doi: 10.1007/s10526-021-10077-8
174. Pérez-Hedo M, Bouagga S, Zhang NX, Moerkens R, Messelink G, Jaques JA, et al. Induction of plant defenses: the added value of zoophytophagous predators. J Pest Sci (2022) 95(4):1501–17. doi: 10.1007/s10340-022-01506-3
175. Pérez-Hedo M, Urbaneja-Bernat P, Jaques JA, Flors V, Urbaneja A. Defensive plant responses induced by Nesidiocoris tenuis (Hemiptera: Miridae) on tomato plants. J Pest Sci (2015) 88(3):543–54. doi: 10.1007/s10340-014-0640-0
176. Meller Harel Y, Elad Y, Rav-David D, Borenstein M, Shulchani R, Lew B, et al. Biochar mediates systemic response of strawberry to foliar fungal pathogens. Plant Soil (2012) 357(1–2):245–57. doi: 10.1007/s11104-012-1129-3
177. Cohen Y, Vaknin M, Mauch-Mani B. BABA-induced resistance: milestones along a 55-year journey. Phytoparasitica (2016) 44(4):513–38. doi: 10.1007/s12600-016-0546-x
178. Desmedt W, Jonckheere W, Nguyen VH, Ameye M, De Zutter N, De Kock K, et al. The phenylpropanoid pathway inhibitor piperonylic acid induces broad-spectrum pest and disease resistance in plants. Plant Cell Environ (2021) 44(9):3122–39. doi: 10.1111/pce.14119
179. Thaler JS, Fidantsef AL, Duffey SS, Bostock RM. Trade-offs in plant defense against pathogens and herbivores: a field demonstration of chemical elicitors of induced resistance. J Chem Ecol (1999) 25(7):1597–609. doi: 10.1023/A:1020840900595
180. Buswell W, Schwarzenbacher RE, Luna E, Sellwood M, Chen B, Flors V, et al. Chemical priming of immunity without costs to plant growth. New Phytol (2018) 218(3):1205–16. doi: 10.1111/nph.15062
181. Schwarzenbacher RE, Wardell G, Stassen J, Guest E, Zhang P, Luna E, et al. The IBI1 receptor of β-aminobutyric acid interacts with VOZ transcription factors to regulate abscisic acid signaling and callose-associated defense. Mol Plant (2020) 13(10):1455–69. doi: 10.1016/j.molp.2020.07.010
182. Tao CN, Buswell W, Zhang P, Walker H, Johnson I, Field K, et al. A single amino acid transporter controls the uptake of priming-inducing beta-amino acids and the associated tradeoff between induced resistance and plant growth. Plant Cell (2022) 34(12):4840–56. doi: 10.1093/plcell/koac271
183. López A, Ramírez V, García-Andrade J, Flors V, Vera P. The RNA silencing enzyme RNA polymerase V is required for plant immunity. PloS Genet (2011) 7(12):e1002434. doi: 10.1371/journal.pgen.1002434
184. Jaskiewicz M, Conrath U, Peterhänsel C. Chromatin modification acts as a memory for systemic acquired resistance in the plant stress response. EMBO Rep (2011) 12(1):50–5. doi: 10.1038/embor.2010.186
185. Baum S, Reimer-Michalski EM, Bolger A, Mantai AJ, Benes V, Usadel B, et al. Isolation of open chromatin identifies regulators of systemic acquired resistance. Plant Physiol (2019) 181(2):817–33. doi: 10.1104/pp.19.00673
186. Kuźnicki D, Meller B, Arasimowicz-Jelonek M, Braszewska-Zalewska A, Drozda A, Floryszak-Wieczorek J. BABA-induced DNA methylome adjustment to intergenerational defense priming in potato to Phytophthora infestans. Front Plant Sci (2019) 10:650. doi: 10.3389/fpls.2019.00650
187. Liégard B, Baillet V, Etcheverry M, Joseph E, Lariagon C, Lemoine J, et al. Quantitative resistance to clubroot infection mediated by transgenerational epigenetic variation in Arabidopsis. New Phytol (2019) 222(1):468–79. doi: 10.1111/nph.15579
188. Johannes F, Porcher E, Teixeira FK, Saliba-Colombani V, Simon M, Agier N, et al. Assessing the impact of transgenerational epigenetic variation on complex traits. PloS Genet (2009) 5(6):e1000530. doi: 10.1371/journal.pgen.1000530
189. Cortijo S, Wardenaar R, Colomé-Tatché M, Gilly A, Etcheverry M, Labadie K, et al. Mapping the epigenetic basis of complex traits. Science (2014) 343(6175):1145–8. doi: 10.1126/science.1248127
190. Lee SC, Adams DW, Ipsaro JJ, Cahn J, Lynn J, Kim HS, et al. Chromatin remodeling of histone H3 variants by DDM1 underlies epigenetic inheritance of DNA methylation. Cell (2023) 186(19):4100–16.e15. doi: 10.1016/j.cell.2023.08.001
191. Harris CJ, Amtmann A, Ton J. Epigenetic processes in plant stress priming: open questions and new approaches. Curr Opin Plant Biol (2023) 75:102432. doi: 10.1016/j.pbi.2023.102432
192. European Parliament Directorate-General for Parliamentary Research Services, Kempenaar C, Wenneker M, Bai Y, Apeldoorn D, Riemens M, et al. The future of crop protection in Europe. Appendix 1, Overview of current and emerging crop protection practices. Brussels: European Parliament (2021). doi: 10.2861/044114
193. European Plant Science Organisation. Healthy plants for a sustainable production - statement by the EPSO ‘Plant Health’ Working Group [online] (2023). Available at: https://epsoweb.org/epso/healthy-plants-for-a-sustainable-production-statement-by-the-new-epso-plant-health-working-group/2023/07/11/
Keywords: plant immunity, elicitors, integrated pest management, sustainable crop protection, food safety, beneficial microbes
Citation: Flors V, Kyndt T, Mauch-Mani B, Pozo MJ, Ryu C-M and Ton J. Enabling sustainable crop protection with induced resistance in plants. Front Sci (2024) 2:1407410. doi: 10.3389/fsci.2024.1407410
Received: 26 March 2024; Accepted: 25 July 2024;
Published: 15 October 2024.
Edited by:
Ian A. Dubery, University of Johannesburg, South AfricaReviewed by:
Daniel F. Klessig, Boyce Thompson Institute (BTI), United StatesAxel Mithöfer, Max Planck Institute for Chemical Ecology, Germany
Copyright © 2024 Flors, Kyndt, Mauch-Mani, Pozo, Ryu and Ton. This is an open-access article distributed under the terms of the Creative Commons Attribution License (CC BY). The use, distribution or reproduction in other forums is permitted, provided the original author(s) and the copyright owner(s) are credited and that the original publication in this journal is cited, in accordance with accepted academic practice. No use, distribution or reproduction is permitted which does not comply with these terms.
*Correspondence: Brigitte Mauch-Mani, YnJpZ2l0dGUubWF1Y2hAcHJvdG9ubWFpbC5jb20=