Explore article hub
- 1Centre for Environmental Policy, Imperial College London, South Kensington, London, United Kingdom
- 2Grantham Institute, Imperial College London, London, United Kingdom
- 3Tremough House, University of Exeter, Penryn, United Kingdom
- 4Department of Physics, Imperial College London, London, United Kingdom
- 5Department of Earth, Environmental, and Planetary Sciences, Brown University, Providence, RI, United States
- 6Climate and Environmental Physics Division, University of Bern, Bern, Switzerland
- 7Oeschger Center for Climate Change Research, University of Bern, Bern, Switzerland
- 8Department of Geography, University of Exeter, Exeter, United Kingdom
- 9School of Geosciences, University of Edinburgh, Edinburgh, United Kingdom
- 10Met Office Hadley Centre, Met Office, Exeter, United Kingdom
- 11Department of Environmental Systems Science, ETH Zürich, Zürich, Switzerland
- 12Climate and Ecosystem Science Division, Lawrence Berkeley National Laboratory (DOE), Berkeley, CA, United States
- 13Climate and Environment Earth and Environmental Sciences, St. Francis Xavier University, Antigonish, NS, Canada
- 14School of Geography, Earth and Atmospheric Sciences, University of Melbourne, Parkville, VIC, Australia
- 15LOCEAN, Sorbonne Université, CNRS/IRD/MNHN, Paris, France
- 16Centre for International Climate and Environmental Research (CICERO), Oslo, Norway
- 17Centre National de Recherches Météorologiques (CNRM), Université de Toulouse/Météo-France/CNRS, Toulouse, France
- 18Institute of Arctic and Alpine Research, University of Colorado Boulder, Boulder, CO, United States
- 19Department of Earth, Ocean and Ecological Sciences, University of Liverpool, Liverpool, United Kingdom
- 20Biogeochemical Signals Department, Max Planck Institute for Biogeochemistry, Jena, Germany
- 21International Institute for Applied Systems Analysis, Laxenburg, Austria
Abstract
How do we halt global warming? Reaching net zero carbon dioxide (CO2) emissions is understood to be a key milestone on the path to a safer planet. But how confident are we that when we stop carbon emissions, we also stop global warming? The Zero Emissions Commitment (ZEC) quantifies how much warming or cooling we can expect following a complete cessation of anthropogenic CO2 emissions. To date, the best estimate by the Intergovernmental Panel on Climate Change (IPCC) Sixth Assessment Report is zero change, though with substantial uncertainty. In this article, we present an overview of the changes expected in major Earth system processes after net zero and their potential impact on global surface temperature, providing an outlook toward building a more confident assessment of ZEC in the decades to come. We propose a structure to guide research into ZEC and associated changes in the climate, separating the impacts expected over decades, centuries, and millennia. As we look ahead at the century billed to mark the end of net anthropogenic CO2 emissions, we ask: what is the prospect of a stable climate in a post-net zero world?
Key points
- Substantial uncertainty remains in both the sign and magnitude of the Zero Emissions Commitment (ZEC): the expected additional change in global surface temperature once we achieve net zero CO2 emissions.
- Uncertainty in ZEC has implications for the remaining carbon budget to stay below the temperature limits of the Paris Agreement: a positive ZEC reduces the remaining budget; a negative ZEC opens the door for more ambitious targets or more time to reach net zero.
- The prospect of additional warming after net zero is both plausible and significant, with a chance that ZEC could exceed 15% of total global warming.
- While a ZEC of 0 means no further change to global surface temperatures, other aspects of the Earth system, such as sea levels, will continue to change in a net zero world due to warming realized previously. These changes should be factored into the assessment of safe warming limits and adaptation plans.
- Current climate models do not adequately represent the full scope of complex and interdependent Earth system processes that determine ZEC. We present a structure for quantifying uncertainty in ZEC and propose a roadmap for future research into quantifying ZEC and reducing its uncertainties.
Introduction and definitions
As we advance into the 21st century, a drumbeat of climate-related disasters is sounding an ever-louder alarm. Be they floods, heatwaves, or hurricanes, their impacts are projected to increase in regularity and intensity as global warming continues (1). Tackling the climate crisis is one of the defining societal challenges of this century. In recent decades, the geophysical requirements for halting global warming have been subject to intense research (2–6). Our current models and understanding of relevant processes support the argument that if global carbon dioxide (CO2) emissions from human activities are brought back to ‘net zero’ (i.e., we emit into the atmosphere no more than our activities absorb from it), the increase in global surface temperature may be halted. In other words, by no longer adding CO2 to the atmosphere, we may cautiously expect that global warming can be stopped. The sixth assessment (AR6) of the Intergovernmental Panel on Climate Change (IPCC) (7) supports this key geophysical insight, and this understanding underpins the many ‘net zero’ targets that countries and companies have announced over recent years.
The Zero Emissions Commitment (ZEC) describes the net change to global surface warming after a complete cessation of anthropogenic emissions, in many cases with a focus on CO2. In combination with the transient climate response to cumulative emissions (TCRE), it defines the total amount of global surface warming for a given amount of CO2. In turn, this total expected warming determines the carbon budget: the amount of carbon dioxide that can be released before reaching a certain temperature threshold. A positive ZEC indicates additional surface warming after net zero, a negative ZEC a drop in surface temperature, and a zero ZEC no further averaged surface temperature change (Figure 1). Emerging literature on this topic (4, 8) led the IPCC to conclude that global surface temperatures 50 years after a complete cessation of CO2 emissions (referred to as ZEC50) would stabilize with no substantial further warming, but this assessment came with an important uncertainty range (9). Total surface warming could still increase or decrease once we stop adding CO2 to the atmosphere, with a non-negligible chance that the change would exceed 15% of total warming. IPCC AR6 estimated that the absolute magnitude of ZEC is less than 0.3°C (9) for an initial warming of approximately 2°C.
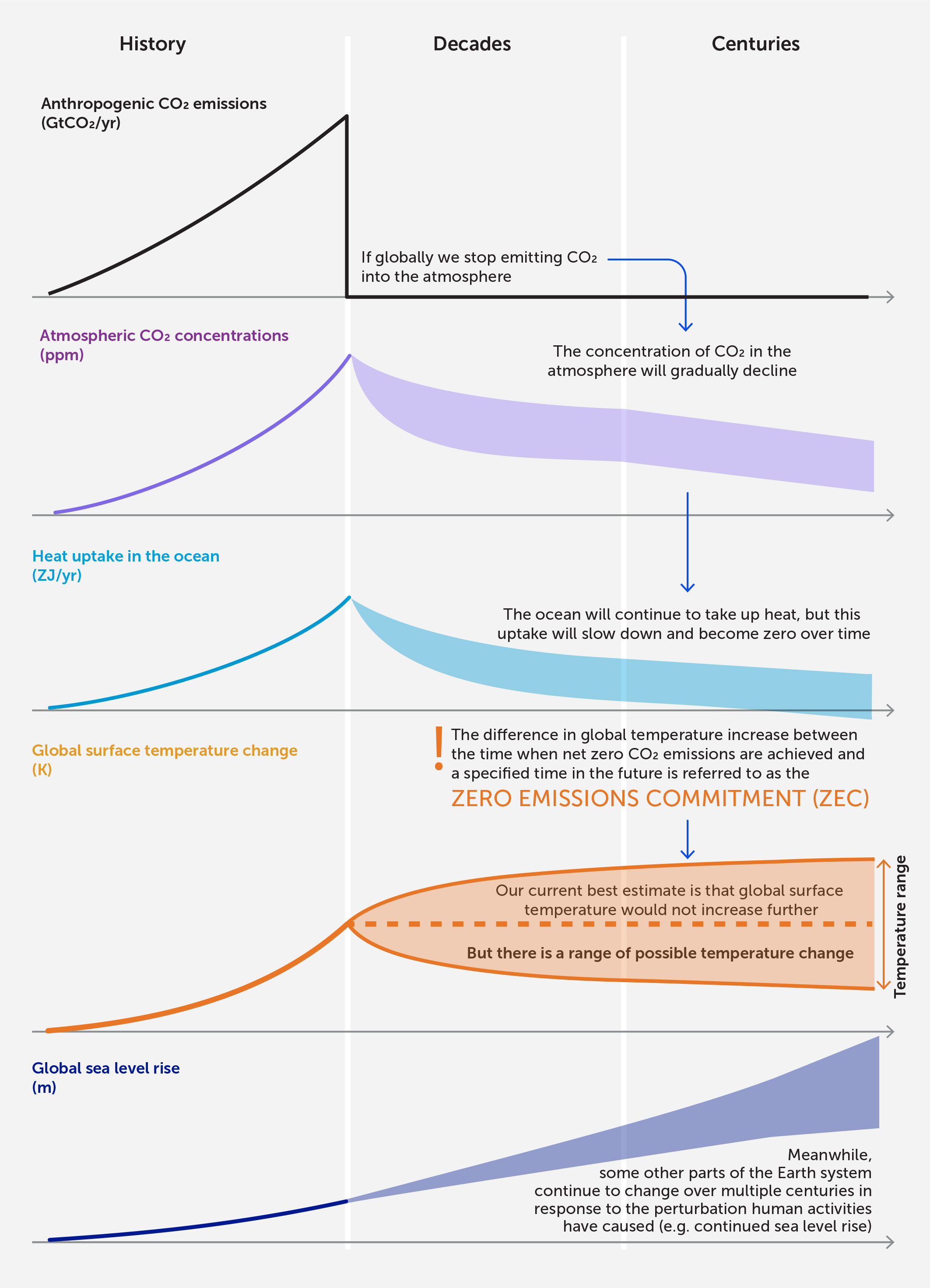
Figure 1 Stylised schematic of how atmospheric carbon dioxide (CO2) concentrations, ocean heat uptake, and global surface temperature can evolve under net zero CO2 emissions. Process timescales are illustrative.
For global surface temperatures to become and remain stable, the behavior of large-scale planetary sub-systems must cancel each other out and equilibrate at similar timescales (10). However, halting global warming does not mean that all Earth system components will stabilize at the same time. Many components of the system will continue to adjust to the perturbation that human activities have caused, including deep ocean warming, sea level rise, and changes in glacier-fed river systems (11).
In this article, we first present key definitions and the current high-level scientific understanding of the ZEC concept. We delve into the processes that determine the ZEC outcome and their uncertainties to better understand what to expect in a world that manages to bring its carbon emissions to net zero. We describe our current best understanding as well as key unknowns and reflect on the implications of these insights for climate policy today.
Definitions
Net zero CO2 emissions are defined by the IPCC as the “condition in which anthropogenic CO2 emissions are balanced by anthropogenic CO2 removals over a specified period” (12). In this article, net zero anthropogenic emissions are considered equivalent to zero anthropogenic emissions, while recognizing that net zero achieved via substantial CO2 removal may be inequivalent to true zero emissions due to impacts on Earth system processes, such as changes to the land carbon sink. Ongoing research exploring the potential difference in warming response between positive and negative emissions is also acknowledged (13).
The Zero CO2 Emissions Commitment is the estimated amount of additional change in global warming we can expect following zero anthropogenic emissions of CO2 and is referred to in this article as ZEC. ZEC is defined by two dimensions: the time since the onset of net zero CO2 emissions, and the global surface temperature change that occurs over this period. Though prone to false equivalence in wider climate communications, ZEC is distinct from the ‘constant composition commitment’, which describes the temperature change expected when atmospheric composition and hence radiative forcing (14, 15) is kept fixed at its present-day value for multiple centuries. Several commonly used phrases such as ‘warming in the pipeline’ (4), ‘committed warming’ (16), and ‘unrealized warming’ (17) risk further conflating the two concepts when insufficiently defined. Unless otherwise specified, in this article, “net zero” will refer to anthropogenic CO2 emissions and removals, and “warming” to the near-surface global mean temperature change.
The broader Zero Emissions Commitment concept can also be applied to non-CO2 gases and wider CO2 inclusive groups, with studies considering ZEC for all anthropogenic forcing agents combined (12, 18–21), individual forcing agents (5, 6, 22–25), or for select combinations of these agents (3, 26). The latest assessments of the current global warming contributions of non-CO2 greenhouse gases and aerosols show that they are of approximately the same magnitude (7). Owing to the relatively short atmospheric lifetime of most anthropogenic greenhouse gases and aerosols compared to the atmospheric lifetime of CO2 on centennial timescales, ZEC for all anthropogenic forcing agents largely depends on the response to CO2 (3, 27). Recent model experiments designed to quantify ZEC have thus focused on CO2 emissions only (28). In addition, while strategies to reduce global CO2 emissions to net zero have been identified in the broad climate change mitigation scenario literature, no existing pathways show a complete elimination of methane (CH4), nitrous oxide (N2O), or aerosol emissions (29, 30). Reflecting this context, we consider ZEC for CO2 only.
ZEC overview
Outline of the ZEC mechanism
ZEC is a function of the geophysical properties of the interconnected Earth system (Figure 2) and how these change as new thermal and biogeochemical steady states are established. ZEC can be thought of as a measure of the lag of the global warming response to a total cessation of net emissions, which results in either additional warming (positive ZEC), cooling (negative ZEC), or a stable temperature (zero ZEC). After emissions of CO2 are reduced to net zero, both atmospheric CO2 concentration (31) and ocean heat uptake are expected to decline (4, 32, 33). In isolation, the drop in atmospheric CO2 concentration has a cooling effect on the climate, while the reduction in ocean heat uptake has a warming effect (4, 33, 34). In Earth system model (ESM) simulations, the drop in atmospheric CO2 concentration is determined by carbon cycle processes that redistribute carbon between the atmosphere, ocean, land, and geological reservoirs. In its simplest form, ZEC can thus be thought of as a surface energy balance relationship in a zero-dimensional model: if, after emissions cease, Earth’s surface and atmosphere absorb more energy than they emit back to space or is stored in the deep ocean, ZEC will be positive; if, on the other hand, Earth’s surface and atmosphere emit more energy than the planet absorbs, ZEC will be negative. Under this simple relationship, temperature stabilization is reached when energy out equals energy in (Figure 3). Changes to feedback mechanisms affecting clouds and surface albedo (or reflectivity), patterns of warming, and exchanges of heat with the deep ocean may modify how Earth absorbs and emits energy in the future compared to the recent past, adding uncertainty to the ZEC outcome.
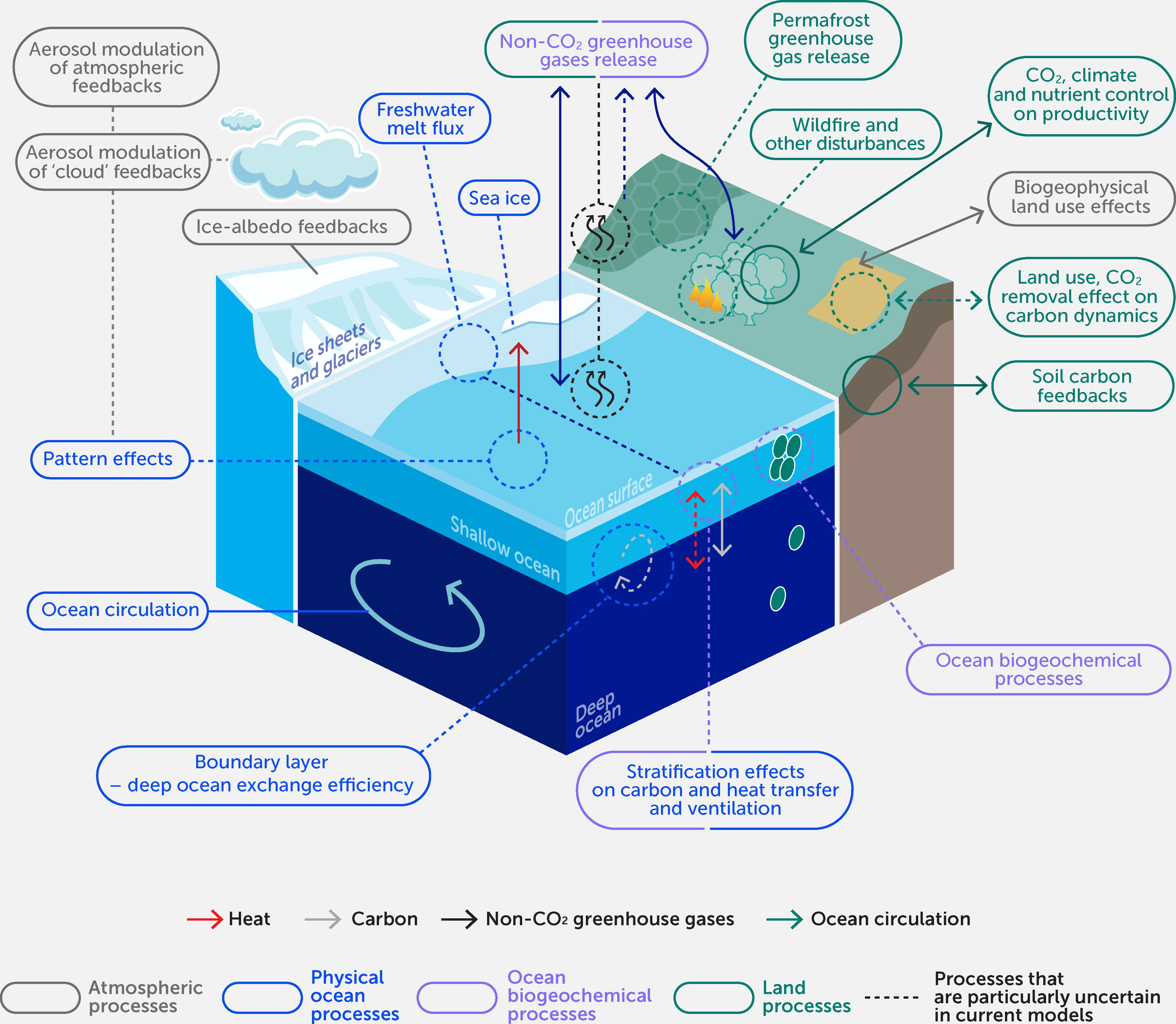
Figure 2 An overview of some of the processes that determine the Zero Emissions Commitment (ZEC), including ocean heat uptake, the ocean biogeochemical cycle, the land carbon cycle, and physical climate feedbacks.
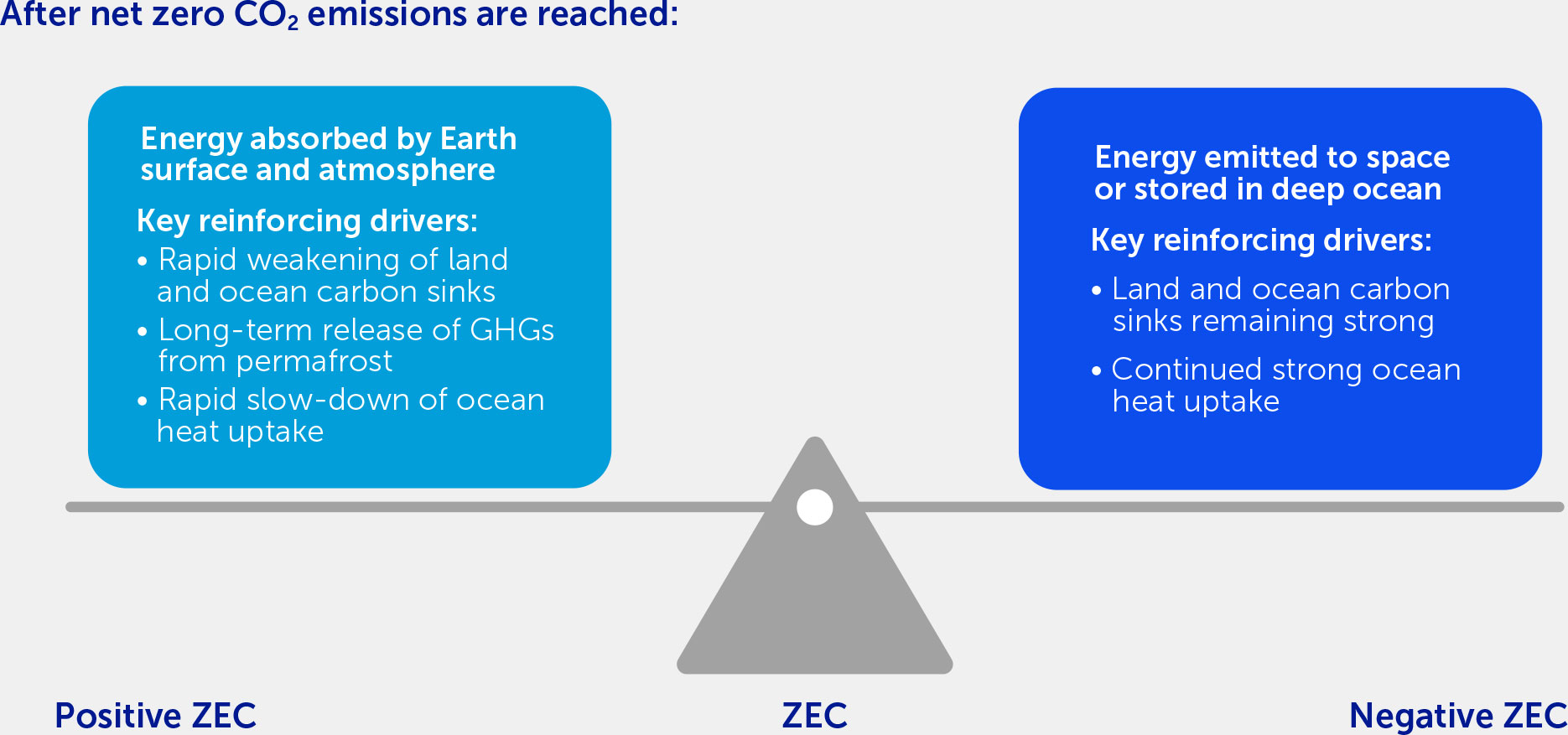
Figure 3 The Zero Emissions Commitment (ZEC) can be thought of as a surface energy balance relation in a simplified “zero-dimensional” model. If, after carbon dioxide (CO2) emissions cease and Earth’s surface and lower atmosphere absorb more energy than they emit back to space or to the deep ocean, ZEC will be positive. On the other hand, if Earth’s surface and lower atmosphere emit more energy than they absorb, ZEC will be negative. Under this simplified relationship, temperature stabilization is reached when energy out equals energy in. Abbreviation: GHG, greenhouse gas.
Even a zero ZEC scenario will not stop regional temperature change (35), long-timescale environmental consequences of climate heating, and Earth’s natural long-term climate variability. For example, global sea level rise will continue owing to mass input from ice sheet dynamics and volume expansion from deep ocean warming driven by past anthropogenic emissions, with centennial to millennial time-lags until ocean heat uptake and ice sheet mass adjustment reach new steady states (11).
ZEC modeling
Studies of how much warming could be expected under particular conditions originated in the 1980s with early analyses of response timescales by Hansen et al. and Wigley and Schlesinger (36, 37) strengthened by constant composition runs in the third phase of the Coupled Model Intercomparison Project (CMIP3) (38). The IPCC Fourth Assessment Report in 2007 included a section on long-term changes (38), presenting results from multiple Earth system models of intermediate complexity (EMICs) specifically running a zero emissions scenario. The Fifth Assessment Report in 2013 featured a “Frequently Asked Question” titled “12.3: What Would Happen to Future Climate if We Stopped Emissions Today?” (10), alongside results from ZEC simulations with EMICs (19). Nevertheless, uncertainty in ZEC remains and contemporary modeling exercises have been designed to structure and expand these investigations to determine the sign and magnitude of ZEC with increased confidence.
The ZEC Model Intercomparison Project (ZECMIP) (28) is the largest ZEC modeling exercise to date, performing coordinated idealized experiments in 18 ESMs and EMICs. ZECMIP considered two types of emissions pathways. The first pathway involved “1pctCO2” simulations in which atmospheric CO2 concentrations increase by 1% per year. For ZECMIP, these “1pctCO2” simulations were run until their diagnosed cumulative CO2 emissions reached a certain value and were then suddenly switched to an emission-driven zero emissions case. The second type of ZECMIP experiments were “bell-shaped” pathway experiments, in which emissions evolved gradually from zero to a peak, and then decreased gradually again to zero. While both experiments converged to roughly equal global mean temperature anomalies, in the latter much of ZEC is already realized by the time zero emissions is reached (4), owing to the slower forcing trajectory in the bell curve experiment.
ZECMIP (4) found a surface temperature change ranging from –0.36 to +0.29°C across participating models 50 years after emissions cease (ZEC50) for cumulative emissions of 1000 PgC, increasing to –0.40 to +0.52°C for 2000 PgC. These models suggest that substantial delayed warming or cooling could occur after emissions are curtailed and that ZEC is state-dependent, meaning that the (relative) magnitude of ZEC can differ depending on the level of anthropogenic cumulative emissions (4). The ESM and EMIC modeling results assessed in IPCC AR6 showed a slightly negative ZEC50 smaller than –0.1°C (–0.34°C to +0.28°C) (4, 9, 28). This informed the latest IPCC assessment, which concluded that the absolute magnitude of ZEC50 for emissions of 1000 PgC is <0.3°C with >66% probability (9). As 1000 PgC emissions leads to approximately 2°C of global warming (39), this implies a ZEC50 of approximately ±15% of realized warming at zero emissions. IPCC AR6 provided no assessment of ZEC on longer timescales and expressed low confidence in the sign of ZEC.
Simple models have also been used to explore what values of ZEC are consistent with observations. The results suggest that industrial-era temperatures alone do not strongly constrain ZEC, but paleoclimate records and independent evidence about equilibrium climate sensitivity could lead to potential further constraints (40). These findings depend on the structural assumptions within the models used and the inclusion of independent degrees of freedom for carbon and thermal climate processes.
Two significant caveats apply to the current modeling evidence relating to ZEC. First, ZECMIP features only a subset of available ESMs, and the 100-year zero emission extensions for ESMs cannot inform us about the potential for carbon-climate, deep ocean, and ice sheet dynamics, which occur on longer timescales. Multi-century simulations with individual ESMs have revealed markedly different behaviors, with an increase in warming after a century-long decrease despite zero CO2 emissions (27, 32, 33, 41), whereas earlier experiments with EMICs did not show such multi-century behavior (19). Second, while there is consensus on a near-zero ZEC as a model mean in the participating ZECMIP models, further research to elucidate the carbon and climate process decomposition is needed to determine if this result arises from common or oversimplified structural underpinnings, from model calibration, or simply by chance—especially given that intermediate complexity models exhibit more diverse behavior (4).
Earth subsystems post net zero
To assess the potential for significant warming or cooling post net zero emissions, we consider the evolution of key Earth subsystem processes that both drive and respond to changing carbon concentrations and heat gradients under net zero CO2 emissions. The shifting dominance of processes from decadal to millennial timescales is discussed, building a picture of interconnected Earth system elements and the changes to global mean temperature these can bring. Some processes require or result in temperature changes, while others are set to evolve even in a world with stable global temperature.
Evolution of Earth subsystems post net zero
Ocean heat uptake
The ocean is the largest reservoir of accumulated energy as a result of anthropogenic climate forcing, having stored roughly 91% of Earth’s additional retained heat since 1971 compared with approximately 1% stored in the atmosphere (4, 5, 33, 42). After net zero CO2 emissions are reached, ocean heat uptake is expected to decline as the ocean approaches a thermal steady state relationship with the atmosphere (4, 5, 33). However, the rate and amount of this decline may be sensitive to processes not well understood, and models are sensitive to the ways in which these processes are represented.
Two parameters are particularly important to understand the role of the ocean in global surface temperature: the efficiency of ocean heat uptake, which is a measure of the ability of the ocean to transport upper ocean waters to depths away from the atmosphere; and the efficacy of ocean heat uptake, which is a measure of the global surface temperature response per unit of heat taken up by the ocean, and which depends on climate feedbacks in response to ocean heat uptake (43).
Efficiency
While most of the energy in recent decades has been absorbed by the upper ocean, the link between the upper and deep ocean is important to understand the ocean temperature of an ocean thermal steady state, and the time needed to reach it. One simple model that accounts for a surface energy reservoir (effectively the upper ocean) and a deeper reservoir (taking surface energy down to greater depths) is the “two-layer energy balance model” (44–47), where heat transfer is assumed to be proportional to the temperature difference (48). Other simple models, which comprise more ocean layers, include an additional structural element that provides a slightly modified long-term response, i.e., the inclusion of polar sinking water and upwelling. This leads to longer response timescales compared with diffusive-only models and replicates general circulation model (GCM) ocean heat uptake relatively well (49–53).
On diurnal to seasonal timescales, the evolving upper 200 m stratification and turbulent mixed layer govern the uptake of energy, where deeper mixed layers have a higher heat capacity and thus the sea surface temperature warms less per unit of energy taken up (54). On decadal timescales, the tropical oceans continue to operate as a mixed layer, while the subtropical oceans and their gyres overturn in subtropical cells, subducting surface waters and their energy anomalies into the pycnocline. On still longer timescales, the polar oceans form subsurface water masses by deep convection that overturns the surface waters into North Atlantic Deep Water (NADW) via the Atlantic Meridional Overturning Circulation (AMOC) and Southern Ocean waters near the Ross and Weddell Seas to form Antarctic Bottom Water (AABW). These water masses will remain away from the surface for centuries and millennia, respectively (55).
Efficacy
The specific patterns of surface heat uptake and surface warming affect the marine boundary layer and thus the atmosphere, leading to complex cloud feedbacks that affect radiative and atmospheric warming and cooling of the oceans (56). For instance, ocean heat uptake at high latitudes is thought to be more effective at cooling the atmosphere than ocean heat uptake at low latitudes (43, 57, 58), which is suspected to originate from shortwave radiation cloud feedback (56).
Biases where the tropical ocean mixed layer depth is excessive tend to increase the short timescale heat capacity of the ocean, while excessive mixed layer depths in the North Atlantic deep convection region tend to warm the NADW, and excessive mixed layer depths in the Southern Ocean deep convection tend to cool the NADW and warm the upper tropical oceans. The CMIP6 ensemble spread in observable indicators of these water formation processes is much larger than that which is supported by observational uncertainty. Thus, the efficacy and climate sensitivity of the CMIP6 ensemble, and two-layer models trained to match it, can likely be improved substantially through better ocean process representation (Hall and Fox-Kemper, 2022)1. While all ESMs have deep oceans that warm during emissions and continue to do so long after emissions cease and surface exchanges stabilize, the rate at which the surface ocean loses heat to the deep ocean (and the strength of feedbacks on surface ocean temperature anomalies) varies among models, affecting the precision of ZEC estimates on the multi-decadal scale and longer.
Longer term change
The timescales of the deep and bottom oceanic overturning are on the order of centuries to millennia. Neither the NADW nor AABW have yet been fully equilibrated to the surface climate changes of the last century, let alone modern changes. That means that the deep ocean will continue to warm, acidify, and deoxygenize over the coming centuries and millennia even if net zero CO2 emissions are reached (11). We expect continued warming and volume adjustment in the deep-water masses, which implies a continuing global mean thermosteric sea level rise of roughly 0.25 m/°C of surface warming after 2000 years, along with continued mean ocean temperature and heat content change. As the cryosphere is also slow to adjust, the total global mean sea level rise is expected to be closer to 0.6 m/°C of surface warming after 100 years, or 4 m/°C after 2000 years and 7 m/°C after 10,000 years. During the last interglacial period, for example, temperatures were 0.5°C to 1.5°C warmer, and the sea level was 5–10 m higher (11, 59).
Ocean carbon uptake and marine emissions of other biogeochemical cycles
The processes governing the ocean carbon response to the cessation of CO2 emissions result from the same processes and mechanisms that drive the sign and magnitude of the ocean carbon sink during rising emissions (60). Anthropogenic CO2 accumulates in the surface ocean via enhanced air-sea gas exchange, which is then transported to depth by ocean currents and mixing (61, 62). This newly accumulated CO2 alters the chemistry in the ocean, acidifying it through carbonic acid (63). Climate change-induced perturbations to ocean temperature, circulation, and biological processes then act to further alter the ocean carbon cycle (64, 65). The relative contribution of each process in setting the regional and global response to emission cessation remains largely uncertain, however, and may depend on the state (i.e., the level of warming and atmospheric CO2) at which emissions are brought to net zero.
Current knowledge, mostly based on multi-model assessment, suggests that the role of the ocean carbon cycle varies substantially across timescales. On annual to multi-decadal timelines after net zero CO2, the magnitude of ocean carbon uptake is projected to be similar to that absorbed by the terrestrial biosphere (4, 31). On centennial to millennial timescales, the role of the ocean becomes increasingly prominent. The results of ESM simulations (4, 31, 41, 66) indicate that while the cumulative land carbon sink stops growing within about a century after reaching zero emissions, and even begins to decrease thereafter, the ocean continues to absorb carbon. Joos et al. (31) reported that, after 1000 years of simulation, on average 59% of the 100 GtC of emitted carbon (under present-day conditions) will have been transferred to the ocean and 16% to the land, with 25% remaining in the atmosphere.
Beyond the ocean carbon cycle, many other ocean processes may influence the overall ocean carbon contribution to ZEC, including mesoscale eddies, melting from ice sheets, sea ice cover, and CH4 release—all of which add to the uncertainty in defining the outcome of ZEC.
Land carbon
The land carbon contribution to ZEC is dominated by three types of processes: those that govern the carbon response to CO2 concentrations in terrestrial ecosystems; those that govern the response to climate change; and anthropogenic land use and land use change. Each of these processes may also interact with one another.
Land carbon response to CO2
In a world with excess CO2 emissions, the magnitude of the land carbon sink increases via CO2 fertilization of leaf photosynthesis, which is one of the most important drivers of current land carbon uptake (67). Increased ecosystem productivity, resulting from increased CO2 fertilization, leads to the accumulation of carbon in biomass and soil organic matter as ecosystem respiration is exceeded, at least temporarily. This land carbon accumulation in response to CO2 tends to be limited, however, by the availability of nutrients, especially nitrogen and phosphorus (68, 69). Over time, decomposition also increases and balances the increased production. Thus, declines in CO2 fertilization, due either to increased nutrient limitation or declines in the growth rate of atmospheric CO2, lead to a reduction of the land carbon sink (70). When CO2 emissions reach net zero, the speed with which terrestrial ecosystems respond to the change from rising to falling atmospheric CO2 concentrations (by slowing or potentially stopping their net carbon uptake) will strongly influence the land contribution to ZEC.
Land carbon response to climate change
The land carbon sink capacity is further modulated by climate feedbacks (60, 71, 72). Cumulative carbon emissions result in persistent global warming after reaching net zero emissions, and the committed long-term land response to this warming represents a key set of processes that in turn will govern the magnitude of ZEC. Processes such as the loss of carbon from peatland and permafrost soils, which are currently not well represented in most ESMs, are likely to increase the magnitude of a positive ZEC as time progresses (73). Changes to biome distributions and ecosystem structures may also continue long after temperatures have stabilized, thus representing a long-term committed carbon stock change (74). Since many of the CMIP6 ESMs do not account for vegetation dynamics, these changes are also likely underrepresented throughout the CMIP6 ensemble and in the ensemble mean. Pugh et al. (74) suggest that the long-term committed sink from vegetation expansion offsets the loss from permafrost, but both are highly uncertain. Other processes, such as the committed ecosystem response to changed disturbance processes, such as fire and drought, lead to large carbon losses in forests and wetlands, and may also increase ZEC (75). Interactions between processes should not be neglected. For example, thawing permafrost not only liberates carbon but also nutrients that may stimulate plant growth and thereby locally and partially counterbalance the carbon loss from permafrost thaw (76).
Land use
To date, ZEC has been quantified in idealized scenarios that do not include historical or potential future land use dynamics. ESM results predicting continued carbon uptake by terrestrial ecosystems post zero emissions should therefore be qualified; this carbon sink may rely in part on substantial contributions from regions where historical land use, or future changes, may preclude such uptake owing to deforestation (which reduces sink capacity) and continued carbon emissions from agricultural lands. However, comparing idealized ZECMIP runs with simulations of the SSP5-3.4-overshoot scenario that include land use (77) suggests that the idealized scenario does not substantially change the magnitude of the committed land carbon response (41). Further, the coupled carbon-climate responses to a net zero state that is characterized by substantial gross fossil-fuel emissions mitigated by land-based CO2 removal may differ from those of idealized zero gross fossil-fuel emissions because of the biophysical responses to land-based mitigation. A wider diversity of ESM experiments using emissions-driven net zero scenarios that vary in their land-based mitigation methods and contributions are required to understand these dynamics.
Physical climate feedbacks
Physical climate feedbacks involve the responses of clouds, surface albedo, water vapor, and temperature via their effects on the global energy balance. A challenge in predicting the long-term evolution of global warming is that these feedbacks vary over time. Indeed, under sustained CO2 forcing, most coupled atmosphere-ocean climate models predict more amplifying feedbacks as time progresses (56). This feedback time dependence may result in ZEC being positive. For example, one ESM experiment revealing a positive ZEC featured a change in the magnitude of the physical climate feedback acting to enhance surface warming (33). This long-term evolution of physical climate feedbacks is poorly understood, particularly in a scenario of carbon emissions declining to net zero.
On all timescales relevant to ZEC, much of the feedback time-dependence comes from low clouds via their impact on solar reflection and thus the global energy balance (78). The amount and distribution of low clouds strongly depend on the spatial pattern of sea surface temperatures (SST), a phenomenon known as the “SST pattern effect” (79). Of particular importance is the SST differential between regions of deep atmospheric convection (e.g., the Indo-Pacific warm pool) and other regions; this controls the stability of the atmosphere and thereby the amount of marine low clouds (80). Under sustained CO2 forcing, coupled climate models typically simulate a decrease in this SST differential, resulting in more amplifying low cloud feedback over time (albeit with large uncertainty), acting to enhance surface warming. How the SST pattern and clouds evolve under a scenario of net emissions declining toward zero is unknown, meaning that the impact of cloud feedback on ZEC is poorly understood.
In addition to the SST pattern effect, additional drivers of time-dependence in low cloud feedback include state-dependent cloud responses, for example, in mixed-phase clouds where the feedback depends on the initial ratio of liquid versus ice phase (81) and in regime transitions resulting from coupled ocean-atmosphere feedbacks (82). Furthermore, deep convective clouds may also exhibit regime transitions between different levels of spatial aggregation (83), affecting the global energy balance by modulating the greenhouse effect of high clouds.
On millennial timescales, the melting of ice sheets will fundamentally alter the global radiative budget, both via local changes in surface albedo and via remote climate impacts mediated by changes in ocean overturning and global sea level. Ocean circulation changes would likely interact with the SST pattern effect, allowing clouds to modulate the global climate response to ice sheet melting. The coupling of ice sheets with global climate allows for a potentially large impact on ZEC, but we currently have no confidence in the sign or magnitude of this effect.
Earth subsystem processes assessed by uncertainty
In this section, we outline the processes in each Earth subsystem that drive uncertainty in ZEC. This uncertainty can be organized into five categories:
● processes known or suspected to impact ZEC, but currently missing in models
● processes with high uncertainty, whose uncertainty and interactions are not sufficiently represented in models
● common structural biases in current modeling frameworks that tend to bias ZEC estimates to the upper or lower ranges
● the possibility of abrupt change or irreversible tipping of system dynamics, and
● the potential of state and pathway dependence for all these subsystem processes.
These uncertainties warrant caution in two aspects. First, temperature stabilization could take longer than anticipated and be both pathway- and state-dependent. Second, a world that reaches net zero emissions will still be locked into long-term changes for which we must prepare.
Many of the uncertainties in ZEC are related to uncertainties in the transient climate response to cumulative carbon emissions (TCRE) as they are driven by similar processes whose response is correlated over time. For example, MacDougall et al. (4) showed that the natural carbon uptake at net zero is correlated with the ongoing carbon uptake after net zero [(4) see figure 9 therein]. For ZEC on millennial timescales, processes that contribute more prominently to uncertainty in the equilibrium response also start to emerge.
Ocean heat and carbon uptake: drivers of uncertainty
Heat and carbon are absorbed by the ocean via the mixed layer. They are then transported and distributed vertically at a rate determined by layer depths, turbulence, convection, overturning, and detrainment. These ocean physical processes therefore control both excess heat and anthropogenic carbon and their relative uptake and transport to the deep ocean, which is a major determinant of ZEC. However, uncertainty remains in the quantification and modeling of these processes (84–86). Currently, we can only assess if an increase or decrease in each process would increase or decrease ZEC, not whether a more realistic model tends toward increasing or decreasing each process. The impact of these activities on ZEC is the approach shown in Table 1.
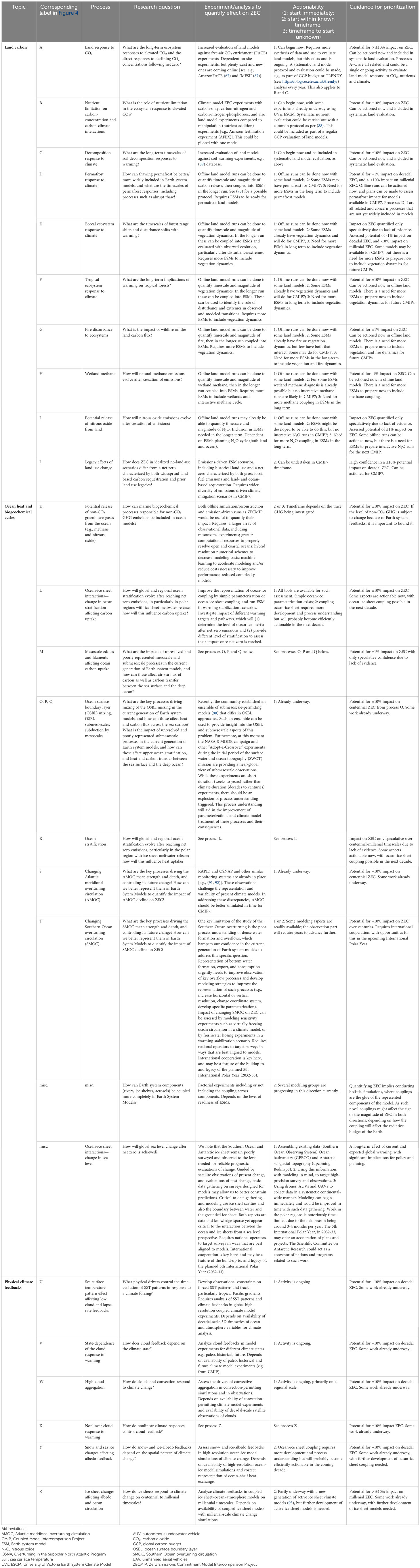
Table 1 Future research to improve the sign and magnitude of the Zero Emissions Commitment (ZEC) must strengthen couplings between the relevant Earth system components (both physical and biogeochemical) to determine their responses to rising carbon dioxide and climate change. This table poses research questions and suggests modeling experiments to address the uncertainty of the processes listed in Figure 4.
Uncertainty caused by processes affecting ocean heat and carbon, as well as physical climate feedback
Air-sea fluxes
In general, atmospheric anomalies in temperature and carbon concentration are well mixed and thus largely uniform across the air-sea surface, meaning the ocean uptake for heat and carbon behave similarly. However, in some regions and processes, they differ; for example, polar amplification results in increased regional warming affecting only heat uptake (and not carbon), while seawater carbonate chemistry and biological processes affect only carbon. These may lead to subtle long-term differences in ZEC. Separately, upper ocean processes involved in air-sea fluxes, such as mixing and submesoscale, change the rates and sensitivity of large-scale processes such as overturning.
Large-scale overturning
The North Atlantic and Southern Ocean overturning cells are critical for the transport of carbon and heat at depth, and hence small changes in the overturning strength affect ocean storage of heat and carbon. Overturning also impacts the rate of change in ocean surface temperature, and hence physical climate feedback via the pattern effect and associated cloud response (58, 94). AMOC is projected to weaken in the coming century owing to anthropogenic climate heating (11). After the cessation of emissions, it is expected to continue weakening and then stabilize on a multi-decadal timescale, recovering on a multi-century timescale (95, 96), though many uncertainties remain. Deep convection in the Southern Ocean is also expected to decline in the coming century (11, 97), though changes after net zero emissions remain poorly understood (98). Over the same timescale, changes in the lateral redistribution of heat and carbon are expected because of alterations in the properties of the water masses (58, 86, 99, 100), though the overall response to emission cessation is poorly understood. The combination of uncertainties may be greater than the uncertainty in each isolated process owing to the interplay between physical and chemical processes (101, 102).
Ice sheets
Changes in glacial meltwater from polar ice sheets have important consequences for upper ocean stratification, and hence the strength of the global ocean overturning (103–106). Important uncertainties remain in the future of ice sheet mass loss at different timescales (from decades to millennia) and in the consequences of meltwater-induced feedbacks that may affect the overall Earth energy balance. Additional uncertainty exists due to state dependency on global temperatures when net zero is reached. Long-term committed ice mass loss will be larger for higher CO2 levels than for lower levels (11). In addition, there may be non-linear behaviors via which too much glacial meltwater could entirely curtail bottom water convection, at least temporarily, with consequences for ocean transport of heat and carbon and the SST pattern effect (94, 106). Instabilities in the ice sheet system have a particularly important impact in Greenland (where surface albedo change can lead to enhanced melting and further albedo impacts) and Antarctica (where the ice sheet rests on a bed well below sea level and retreats into deeper terrain). In both cases, ice-loss processes triggered by emissions may now act in isolation, potentially supplying freshwater to the ocean over the coming centuries after net zero is reached. Current ESMs do not represent ice sheets interactively (4, 107) and hence do not capture the impact of their melt and feedback on the ocean. These knowledge gaps prevent us from properly quantifying the combined uncertainty.
Meso and submesoscale processes
Most climate models do not resolve the small-scale (10–100 m) turbulence that mixes the boundary layer of the ocean and the submesoscale (1–10 km) and mesoscale (10–100 km) turbulent eddies that influence the boundary layer, deep convection, and transport of water out of the boundary layers and convection zones into the global overturning (108). This is because such simulations would require unattainably high horizontal and vertical ocean model resolutions, especially at high latitudes. Beyond differences in ocean forcing, the ensemble spread can largely be related to differences in the treatment of these turbulent processes (84). The role of mesoscale eddies for anthropogenic carbon and heat uptake remains unclear. Consideration of small-scale processes suggests a reinforcement of anthropogenic carbon uptake (109). However, the influence of mesoscale eddies through eddy-driven circulation may counteract large-scale circulation features, impacting the overall capacity of the Southern Ocean to take up CO2 over the long term (110, 111). In the ocean interior, deep-reaching submesoscale processes might be central to a correct representation of vertical heat distribution and, therefore, stratification (112, 113), with consequences for carbon uptake and ocean surface temperature.
Uncertainty caused by processes affecting ocean carbon only
Carbonate chemistry
While the circulation determines the volume of anthropogenic CO2 transported into the deep ocean, the carbonate chemistry, which is well understood, determines the concentration of anthropogenic CO2 in these water masses (114). CO2 is a weak acid, and seawater dissociates most of it into bicarbonate, while the seawater carbonate ion concentration is reduced and the proton concentration rises (115). However, current climate models have difficulties in correctly representing the surface ocean carbonate chemistry (e.g., accounting for the Revelle factor), especially in regions with the largest anthropogenic carbon uptake, such as in the Southern Ocean and the North Atlantic (116).
Biological processes
The role of biological processes in long-term carbon storage, especially the transport and transformation of living and dead particulate matter in the mesopelagic zone (117), is highly uncertain (118). It remains unclear how the hard-tissue pump responds to ocean acidification (119). Biological processes missing in current ESMs may impact ZEC in various ways. Although small, these contributions are tightly linked to highly climate-responsive components of the Earth system (e.g., coastal sediments, sea ice, and permafrost), which makes their contribution uncertain. For instance, sediment and weathering interactions are often ignored on very long timescales (66). Sea ice biogeochemistry may lead to important dimethylsulfide (DMS) emissions with respect to the proportion controlled by the open-ocean biogeochemistry (120). We understand little about how these processes may contribute to ZEC, but, overall, the contribution of biological processes to the oceanic uptake of anthropogenic carbon is small, at least on the decadal to centennial timescales (121–123).
Potential release of non-CO2 greenhouse gases from the ocean (e.g., CH4 and N2O)
It is well known that potential CH4 emission sources in the coastal and open ocean (e.g., clathrates in marine sediments) are sensitive to climate change (124). Though the exact magnitude of future CH4 emissions remains uncertain, its dependence on the magnitude of future warming is better supported. Despite polar amplification, substantial releases from the permafrost-embedded subsea clathrates are very unlikely during the 21st century (39) but cannot be ruled out under very high warming levels. Archer et al. (66), for example, showed that coastal CH4 emissions may increase with ocean warming >3°C. They estimate that this would further burden total atmospheric carbon, with published estimates ranging between 35 and 940 PgC, leading to an additional 0.4–0.5°C warming. Further, large physical uncertainties remain in quantifying the magnitude of CH4 release, particularly with respect to how gas migrates through the sediment column. Individual studies suggest the ocean N2O cycle might be one of the only biogeochemical cycles where climate change induces negative feedback, i.e., a decrease in ocean N2O emissions (125). This is because of increased ocean stratification (which tends to counteract the vertical transfer of N2O from the deep ocean to the surface), decreases in ocean productivity, and the impact of increasing atmospheric N2O abundance on the air-sea flux. As such, potential cooling induced by emission cessation may reinforce N2O emissions from the ocean to the atmosphere. However, current climate models often do not explicitly simulate N2O and CH4 cycles. Consequently, the resulting uncertainty associated with these processes cannot be assessed.
Land carbon: drivers of uncertainty
The response of the land biogeochemical cycle to net zero is tightly linked to the drivers and constraints of the current natural land carbon sink. Here, we provide an initial expert estimate of both the magnitude and direction of uncertainty in ZEC due to uncertainty in the underlying processes, and the timescales to which they apply. Some of these processes have already been included in ESMs but are uncertain, while others are still missing.
Land response to CO2
The largest driver of the land carbon sink—the productivity and allocation response to CO2—is also the largest contributor to its uncertainty. It is known that the uncertainty in the land response to CO2 exceeds that for the ocean and that the response to CO2 is more uncertain than the response to climate (39, 71). This remains true of the drivers of sink change contributing to ZEC. In ZECMIP, the land carbon sink decreases by approximately half after 20 years, nearly 90% after 50 years, and approaches close to zero after 100 years. These ESM assessments do not take into account committed responses of ecosystem recovery from historical disturbances due to land use and land management. The processes at play may lead to greater land uptake after net zero is achieved than is projected in the idealized ZECMIP framework, particularly if the recovery timescales are longer than those used to define the land-use emissions that partially define net zero (74). Land-use drivers that continue to act on longer timescales, such as committed peat loss due to hydrologic alteration of peatlands (126, 127), are not well integrated into ESMs and so may be missing from current assessments of committed land use dynamics and their role in governing ZEC.
As the majority of ESMs used to quantify ZEC are biased by not including nutrient constraints and processes that affect these over long timescales, there may be consequential biases in the calculated magnitude of ZEC (69, 128). In the small sample of ESMs contributing to ZECMIP, there is no systematic difference in ZEC between models with and without representation of the terrestrial nitrogen cycle although there is evidence that the nitrogen cycle reduces both the land carbon response to CO2 and climate change (60). However, the nitrogen effect gradually diminishes with a decreasing atmospheric CO2 growth rate (70), as organisms that engage in nitrogen fixation and other nitrogen cycle processes (such as losses due to leaching) gradually relieve ecosystem nitrogen limitations (70, 129, 130).
Phosphorus limitation on land is likely to further reduce the land carbon response to CO2 and climate change (60). However, there is no clear evidence of how nutrient cycles affect the further response of the sink after net zero.
There is no evidence that the existing estimates would be biased, so this uncertainty (a) is large, (b) symmetrically impacts ZEC, and (c) operates on short to mid-term timescales. Confidence in this assessment is medium due to the relatively large body of literature and some experimental evidence but with a substantial spread in modeling results.
Decomposition response to climate (excluding permafrost soils)
As there is only a small change in global temperature following zero emissions, the expected response of land carbon to the climate post net zero will largely be the long-term continuation of its response to the climate change that had occurred during the period of rising CO2. Warmer temperatures lead to accelerated decomposition of organic matter, and this remains stubbornly uncertain across CMIP6 models even with nutrient cycling considered, as it was in CMIP5. Therefore, this uncertainty (a) is moderate, (b) symmetrically impacts ZEC, and (c) operates on short timescales. Confidence in this assessment is medium due to the relatively large body of literature and some experimental evidence but with a substantial spread in modeling results.
Permafrost feedbacks to climate
Only represented in a small number of the models contributing to ZECMIP, permafrost thaw is a process that contributes to uncertainty in ZEC. It is virtually certain that large areas of permafrost will thaw under a warmer climate. However, the amount of carbon released with decomposition of previously frozen organic matter, the timescale of release and its form (CO2 versus CH4), and the potential compensation by plants growing in areas impacted by permafrost thaw are all uncertain. IPCC assessments (39, 131) suggest that carbon release during this century will likely be small, but if warming persists then the thaw of permafrost will lead to irreversible loss of frozen organic carbon. Abrupt permafrost thaw, which occurs in a narrow region of ice-rich permafrost, has the potential to increase the magnitude of permafrost feedback to the climate, accelerate the timing of those losses, and lead to higher fluxes of CH4 (132). However, the role of abrupt thaw is not addressed by large-scale models, and there is little understanding of emissions after net zero. Overall for permafrost emissions, we assess that the uncertainty will (a) be small initially but grow to be large, (b) lead to a higher ZEC as more CO2 is released, and (c) operate on long (>100-year) timescales. Confidence in this assessment is low due to limited modeling results and the substantial spread of results between studies.
Boreal ecosystems response to climate
Converse to permafrost loss of carbon from high latitude regions, woody encroachment and boreal forest expansion represent potentially long-term committed carbon sinks, while at the same time altering the biogeophysical properties of the surface and therefore the local climate (133). Pugh et al. (74) suggest that these could be of comparable magnitude to permafrost loss, although both are highly uncertain. While some ESMs include dynamic vegetation, it is still relatively rare. We therefore assess this uncertainty will (a) be small initially but grow to be large, (b) lead to a lower ZEC as more CO2 is taken up, and (c) operate on long (>100-year) timescales. Confidence in this assessment is speculative due to a very limited literature base.
Tropical ecosystem response to climate
Tropical ecosystems respond to elevated temperatures and associated changes in rainfall by potentially reducing productivity and wood growth. Research is heavily focused on the Amazon forest, which is seen as especially vulnerable to future climate change (134) and additional pressures from human activity (135). Recently observed increases in mortality (136), decreased sink (137), and changes in variability suggesting decreased resilience (138) all point toward the credible risk of an Amazon forest tipping point occurring if global warming reaches 2°C (77, 139).
Conversely, assessing tropics-wide carbon sinks (140) provides quantitative constraints on tropical temperature sensitivity (across productivity and decomposition), which indicates that smaller rather than larger carbon cycle sensitivities are consistent with observed variability. Therefore, for ZEC, the contribution of tropical ecosystems to uncertainty is (a) moderate to large, (b) possibly biased toward a smaller contribution, but with potential for abrupt changes releasing carbon, and (c) operates on short timescales. Confidence in this assessment is low due to competing observational and modeling constraints on different spaces and timescales and at different levels of global warming.
Fire disturbance to ecosystems
Fire may increase CO2 (and other) emissions from land. It may also reduce equilibrium carbon storage for a given ecosystem. If global climate change is small following zero emissions, then further changes in fire disturbance may also be small, but this process is missing in most ESM models and no studies have assessed the response of fire disturbance after zero emissions. Therefore, this uncertainty (a) is moderate, (b) symmetrically impacts ZEC, and (c) operates on short to mid-term timescales. Confidence in this assessment is low due to limited literature and poor model agreement.
Wetland CH4
Wetland CH4 emissions are driven by both climate (temperature and moisture) and vegetation productivity and therefore are directly responsive to CO2. Warming generally tends to increase emissions (39). However, as CO2 declines post net zero, wetland productivity and thus CH4 emissions may decrease. The balance of climate versus CO2 as a driver of CH4 emission is not well agreed, and modeling techniques differ widely. If this process is significant, it will operate on short timescales as CO2 affects tundra ecosystem productivity. Therefore, we assess this uncertainty to (a) be small to moderate, (b) lead to a lower ZEC as reduced CO2 reduces CH4, and (c) operate on short timescales. Confidence in this assessment is low due to a limited literature base.
Potential release of N2O from land
N2O emissions from natural and semi-natural ecosystems are driven by climate and nitrogen cycle processes, which are directly or indirectly affected by the productivity response of plants to atmospheric CO2 changes. The sign and magnitude of the response to changes in either driving factor are location-dependent, and there is no general understanding of the large-scale responses of terrestrial N2O fluxes to either CO2 or climate change. Models suggest that rising CO2 decreases and climate change increases N2O emissions (39, 141). We assess the uncertainty to (a) be moderate, (b) symmetrically impact ZEC, and (c) operate on a mid- to long-term timescale. The confidence in this assessment is low due to a limited observational and literature base.
Land-use change: legacy effects and continued change
Past land-use changes and land management have left the land out of equilibrium with regard to carbon storage and have reduced the additional sink capacity the land may have provided. The legacy effects of this disturbance could lead to either increased carbon accumulation (e.g., forest regrowth after abandonment) or carbon loss (e.g., following peatland drainage or drying) post zero emissions and hence could affect ZEC. As the land continues to adjust to past disturbances, the impact of these will decrease over time.
Continued land-use changes and changes in management are not directly related to ZEC but represent one of the dimensions in which a gross zero emissions world can differ from a net zero world. Given the large potential variations in net zero configurations (29, 142), they represent an additional source of uncertainty. This uncertainty is potentially large but either positive or negative in terms of carbon emissions depending on the balance of continued deforestation and any use of land for CO2 removal. Both are very scenario-dependent.
Physical climate feedbacks: drivers of uncertainty
Inter-model differences in climate feedbacks are the dominant driver of the inter-model uncertainty in surface warming projections in CMIP6 projections (following a scenario of CO2 concentrations increasing by 1% per year), as quantified by the proportionality between surface warming and cumulative carbon emissions (143). This inter-model uncertainty is primarily due to shortwave and longwave cloud effects, as well as changes in surface albedo. With regard to clouds, key uncertainties include the coupling of low clouds with surface temperature warming patterns, state dependence in the cloud response involving changes in the cloud water phase, and regime transitions in the coverage of cloud types. All of these changes in cloud distribution and cloud type alter the radiative energy balance and so will affect ZEC. Surface albedo changes involving snow and sea ice coverage also alter the physical climate feedback, and their distribution is coupled to the patterns of surface warming. On millennial timescales, there are potentially important changes in ice sheets, affecting surface albedo and the ocean overturning, with knock-on effects on the global energy balance. These sources of uncertainty from physical feedbacks affecting projections of future warming are likely to carry over to uncertainties in the warming projections when net zero is approached and may be sufficiently large to alter the sign of ZEC. In summary, key knowledge gaps remain in how physical climate feedbacks are likely to change over time with declining atmospheric CO2, and the extent to which physical climate feedbacks affect the sign and magnitude of ZEC.
Expert assessment and future research
Expert assessment
Here we present an expert assessment by the article’s co-authors, supported by available published evidence from the literature where possible, to quantify the plausible impact of these Earth system processes on the magnitude and sign of ZEC over decades, centuries, and millennia (Figure 4, Table 1). We propose this structure as a guide for future research into quantifying ZEC and reducing its uncertainties.
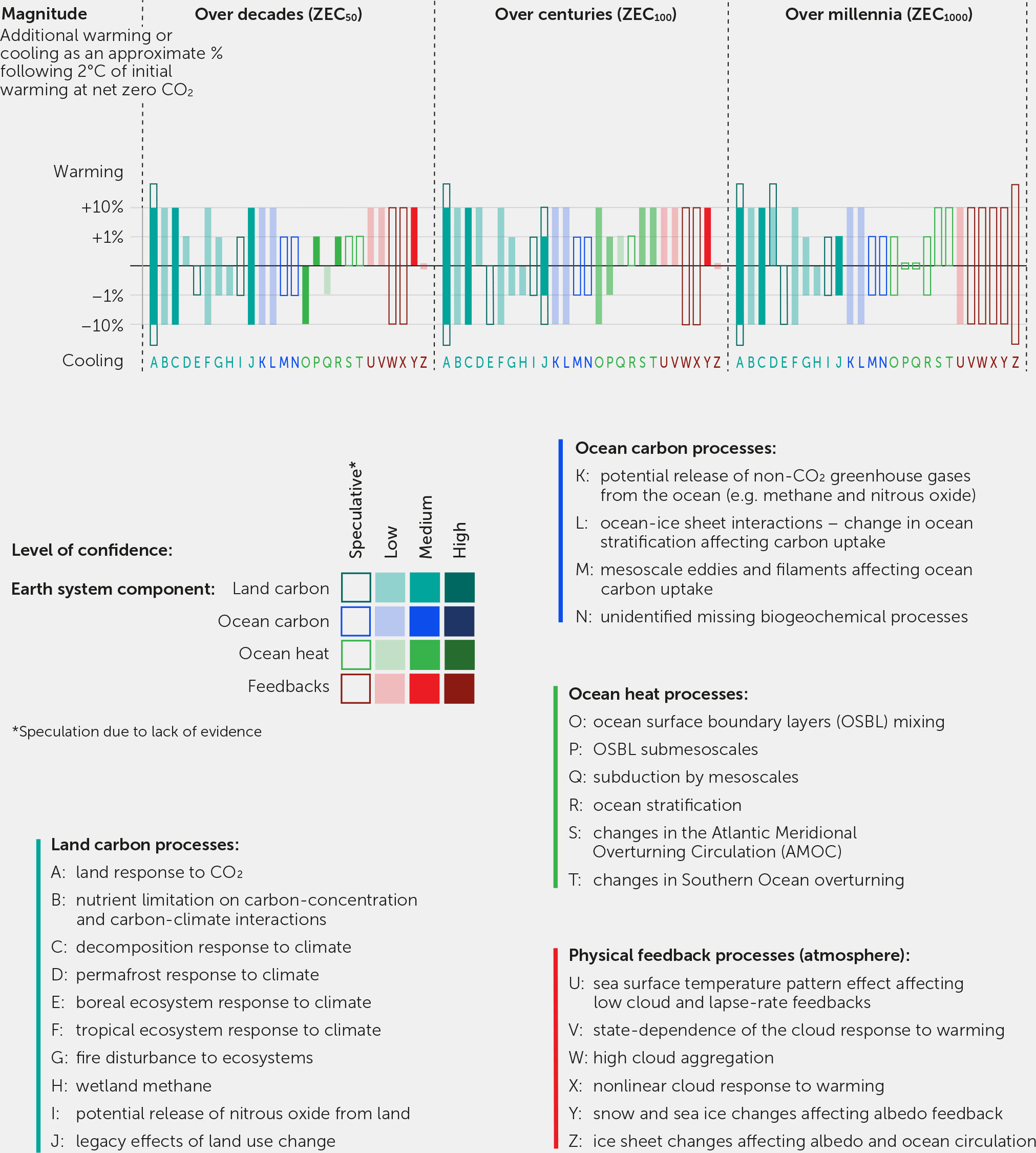
Figure 4 Expert assessment of the hypothesized impact of Earth system uncertainties on the magnitude of the Zero Emissions Commitment (ZEC) for futures initially aiming to limit global warming to 2°C.
A key take-home message is the large proportion of low—and often only speculative—confidence assigned to the impact of many processes on ZEC. It is clear that uncertainty remains high in both ZEC’s sign and magnitude over short- and long-term timeframes, owing to uncertainties in the impact of its constituent parts. Though it is known that some long-term changes to the Earth system, such as sea level rise, will continue beyond net zero, it is now clear that we must also consider the possibility of significant continued temperature rise even after anthropogenic emissions cease. This uncertainty should be factored into the risk assessment of global temperature limits, carbon budgets, and emissions reduction plans for countries and businesses.
Future research
Ocean heat and biogeochemical cycles
One of the key properties of ZEC is that it emerges from the coupling of the climate and the carbon cycle. As such, improving ZEC in terms of sign and magnitude requires improving the couplings between Earth system components, including both physical and biogeochemical ocean components. Current models have evolved toward a more comprehensive treatment of biological boundary conditions (e.g., atmospheric deposition, riverine inputs, sediments, ice sheets, and geothermal sources) but the latter is currently largely represented using climatological data rather than dynamic connections (144). Progress toward more complete couplings between Earth system components such as rivers, ice sheet/iceberg calving, and ice shelves or atmospheric aerosols can help to better simulate interactions between marine biogeochemistry, biogeochemical cycles, and climate, and hence result in a more comprehensive assessment of ZEC. The representation of the coupling between ocean and ice sheets has been shown to be critical in simulating the decline in global ocean overturning circulation (145), with key implications for the ocean surface temperature affecting low cloud development (146), as well as for ocean heat and carbon uptake (147), though the quantification of the decline and its impact on ZEC remains to be clarified.
The emergence of high-resolution ocean models that include marine biogeochemical processes may be supported in a number of ways: (1) the availability of even greater computational resources; (2) the use of hybrid-resolution numerical schemes to decrease the cost of biogeochemical models (148); (3) reduced complexity models of marine biogeochemical processes [e.g., miniBLING (149)]; and (4) the use of machine learning to either accelerate marine biogeochemical models or to reduce the numerical cost necessary to improve their performance (i.e., via tuning). These (and potentially other) step-changes will help us to understand the extent to which mesoscale or submesoscale ocean physics might change the response of marine biogeochemistry to rising CO2 and climate change—a missing factor in such models already highlighted about a decade ago (64).
Land carbon
Land carbon fluxes dominate the total uncertainty in assessments of changes in the natural carbon cycle on decadal timescales, with ocean processes dominating on longer timescales. Priorities for reducing uncertainty in ZEC are tightly related to priorities for modeling the land carbon cycle in general and its role in determining carbon cycle feedbacks, the transient response to cumulative CO2 emissions (TCRE), and carbon budgets. Notably, the response of ecosystems to elevated CO2 and the role of nutrient limitation is considered the highest priority. Model data comparisons against site-level manipulation experiments [e.g., free-air CO2 enrichment (FACE) experiments] or nutrient supply experiments [e.g., the Amazon Fertilisation Experiment (AFEX)] offer valuable ways forward. Forthcoming FACE experiments in a tropical forest in Brazil will prove extremely valuable. However, experiments must also capture the long-term response of ecosystems; it is well established that elevated CO2 causes an initial increase in productivity, but less is known about the long-term impacts on ecosystem carbon storage. Similarly, ecosystem responses to environmental changes can be improved in models with valuable observational experiments, such as the Caxiuana throughfall exclusion site (150, 151) which intercepts rainfall to mimic drought conditions, or global syntheses of soil warming experiments (90, 152).
Representing missing processes is also increasingly important, including physical and carbon permafrost processes and vegetation dynamics. Some, but not all, models have improved in their representation of permafrost physics from CMIP5 to CMIP6 (153), but very few models represent loss of carbon from thawing permafrost.
Physical climate feedbacks
The role of time-varying physical feedbacks in determining the future climate response is under active investigation for increasing carbon emissions. There are key uncertainties in the representation of clouds over different parts of the atmosphere and in surface albedo changes linked to the presence of snow and sea ice. We are beginning to understand the physical mechanisms controlling the forced pattern of sea surface warming and its time evolution (154, 155), which is critical for the long-term evolution of climate feedbacks. Developments in high-resolution modeling are also elucidating possible state-dependence and regime transitions in cloud responses to warming (82). However, work so far has focused on cases of increasing or constant climate forcing; little attention has been given to the role of physical climate feedbacks in the climate response to the specific scenario of carbon emissions declining to net zero and the resulting effect on ZEC.
Simple climate models
Simple climate models (SCMs) provide tools for exploring how process interaction may lead to results that are not observed in the current ESM ensemble, which constitutes an “ensemble of opportunity” since the ESMs that have examined ZEC are a serendipitous selection of models from researchers interested in participating in the ZECMIP exercise. SCMs contain modules that represent many of the processes controlling ZEC, albeit in simplified ways. By tuning these modules to capture behaviors at different ends of the spectrum of scientific uncertainty, the link between ZEC and relevant processes can be examined in a simplified manner. This approach allows the exploration of results that would be expected if the behavior of multiple processes were at the extreme end of their potential range, recognizing that this simplified approach may miss couplings such as that between ocean carbon and heat uptake. Given the multiple feedbacks and non-linear processes involved, the high-end result could be much larger than a simple linear superposition would imply. The key to performing such investigations is a strong understanding of how simple climate model modules and behavior relate to the processes controlling ZEC. While the broad mappings are understood, research is needed to understand them in detail. With this detailed understanding, individual (partial) and combined (feedback) effects on ZEC can be isolated from variations in physical processes.
Implications of ZEC for climate policy
The identified uncertainties in ZEC come with implications for climate policy, both in the near and the long term. Over the coming decades, the potential variation in ZEC on timescales of decades to centuries is most relevant. To understand the implications for climate policy, ZEC insights need to be translated from model studies to the real world. ZEC is studied with idealized model experiments, often assuming a sudden stop in global emissions (4, 28). The real-world evolution of global emissions will be different with a presumably more gradual decline to net zero over multiple decades. In such a scenario, ZEC over decadal timescales will already be partially realized at the time of reaching global net zero CO2 emissions (4, 156). This suggests that for practical applications, ZEC can be usefully defined as the committed warming relative to the expected linear TCRE relationship, instead of as the warming still to occur after reaching net zero (156). The potential additional increase or decrease in warming implied by ZEC should be folded into assessments of the remaining carbon budget (17, 64, 141). If the decadal ZEC is positive, the remaining carbon budget until net zero CO2 emissions are reached will be smaller. If it is negative, there could be a bit more leeway, or alternatively, more stringent climate targets could be achieved. Our current assessment of ZEC uncertainties, however, shows more uncertainties pointing toward a potentially higher ZEC (Figure 4) and thus a further tightening of the already very small remaining carbon budget.
Because ZEC already partially materializes before total global CO2 emissions reach zero (4, 156), the sign and magnitude of ZEC to some degree also inform the timing of peak warming relative to reaching net zero CO2 emissions. The evolution of non-CO2 emissions further modulates this relative timing. Understanding and communicating clearly on these issues can be important, as the general public might expect temperatures to peak once net zero CO2 emissions are reached.
Over the long term, the uncertainty in ZEC comes with further implications for policy. In case of a negative ZEC over centuries to millennia, future societies will have fewer challenges to reverse some of the global warming. This would be good news. However, the assessed uncertainties on these timescales indicate a positive rather than a negative ZEC (Figure 4). Over millennia, some Earth system feedbacks could result in significant additional amounts of warming. Such additional warming would frustrate achieving a peak and decline in global warming, as is assumed in many global mitigation scenarios (29, 30) and potentially even the Paris Agreement (157, 158).
To counteract potential continued gradual warming over centuries to millennia, our global society will most likely have to prepare for continued active management of the global carbon cycle by drawing CO2 out of the atmosphere, while taking issues related to land use and biodiversity into account. The long-term consequences of the CO2 already emitted, and that which will be emitted until global net zero is reached, imply that net zero CO2 remains an important milestone but that it might not be enough. The uncertainties in ZEC point toward further long-term warming risks and the imperative to develop sustainable, safe, and reliable options for long-term CO2 removal to keep Earth’s climate stable. Importantly, the assessment of ZEC uncertainties also emphasizes that to limit risks it is essential to keep the initial disturbance of the Earth system as low as possible. This can be achieved first and foremost by reducing global CO2 emissions to net zero swiftly in this decade and over the coming decades, and by continuing to pursue limiting global warming to as close to 1.5°C as possible.
Supplementary material
The Supplementary Material for this article can be found online at: https://www.frontiersin.org/articles/10.3389/fsci.2023.1170744/full#supplementary-material
Supplementary Data Sheet 1 | Expert assessment supporting Figure 4. Hypothesised impact of individual processes on the uncertainty in ZEC over decades, centuries and millennia.
Statements
Author contributions
SPC: Investigation, Project administration, Visualization, Writing – original draft, Writing – review & editing. MS: Conceptualization, Investigation, Writing – original draft, Writing – review & editing. PC: Investigation, Writing – original draft, Writing – review & editing. BF-K: Investigation, Writing – original draft, Writing – review & editing. TF: Investigation, Writing – original draft, Writing – review & editing. AG-S: Investigation, Writing – original draft, Writing – review & editing. JH: Investigation, Writing – original draft, Writing – review & editing. GH: Investigation, Writing – original draft, Writing – review & editing. CJ: Investigation, Writing – original draft, Writing – review & editing. RK: Investigation, Writing – original draft, Writing – review & editing. CK: Investigation, Writing – original draft, Writing – review & editing. AM: Investigation, Writing – original draft, Writing – review & editing. MM: Investigation, Writing – original draft, Writing – review & editing. ZN: Investigation, Writing – original draft, Writing – review & editing. JS: Investigation, Writing – original draft, Writing – review & editing. BS: Investigation, Writing – original draft, Writing – review & editing. RS: Investigation, Writing – original draft, Writing – review & editing. MT: Investigation, Writing – original draft, Writing – review & editing. RW: Investigation, Writing – original draft, Writing – review & editing. SZ: Investigation, Writing – original draft, Writing – review & editing. JR: Conceptualization, Investigation, Visualization, Writing – original draft, Writing – review & editing.
Data availability statement
The original contributions presented in the study are included in the article/Supplementary Material. Further inquiries can be directed to the corresponding authors.
Funding
The author(s) declare financial support was received for the research, authorship, and/or publication of this article. The study presented in this manuscript benefitted from the direct funding of ESM2025 (grant number: 101003536), through the author SPC. The authors JR, CJ, RS, BS and SZ acknowledge support from ESM2025. The funder was not involved in the study design, collection, analysis, interpretation of data, the writing of this article, or the decision to submit it for publication. The authors also declare the following indirect funders which were not involved in the study design, collection, analysis, interpretation of data, the writing of this article, or the decision to submit it for publication: SPC was funded by the NERC 'Science and Solutions for a Changing Planet' doctoral training partnership, and the Grantham Institute for Climate Change and the Environment. JR acknowledges support from the European Union’s Horizon 2020 Research and Innovation Programme’s CONSTRAIN (grant number: 820829), ESM2025 (grant number: 101003536), and PROVIDE (grant number: 101003687) projects. AG-S received funding from the European Research Council (ERC) under the European Union’s Horizon 2020 research and innovation programme (grant agreement No 865403). The article reflects the authors’ view and the European Commission is not responsible for any use that may be made of the information it contains. CJ was supported by the Joint UK BEIS/Defra Met Office Hadley Centre Climate Programme (GA01101) and the European Union's Horizon 2020 research and innovation programme under Grant Agreement No 101003536 (ESM2025 - Earth System Models for the Future). CK acknowledges support by the Director, Office of Science, Office of Biological and Environmental Research of the US Department of Energy under contract DE-AC02-05CH11231 through the Regional and Global Model Analysis Program (RUBISCO SFA). TF was funded by the European Union’s Horizon 2020 research and innovation program under grant agreement no. 821003 (project 4C, Climate-Carbon Interactions in the Current Century) and no. 820989 (project COMFORT, Our common future ocean in the Earth system-quantifying coupled cycles of carbon, oxygen and nutrients for determining and achieving safe operating spaces with respect to tipping points) as well as by the Swiss National Science Foundation under grant PP00P2_198897. JS received funding from the European Union’s Horizon 2020 research and innovation program under grant agreement N°821001. BS acknowledges 4C (Grant agreement ID: 821003), PROVIDE (grant agreement No. 101003687) and ESM2025 (Grant agreement ID: 101003536). AM was funded by the Natural Science and Engineering Research Council of Canada Discovery Grant program. RW acknowledges the U.K. Natural Environment Research Council (NE/T007788/1, NE/T010657/1).
Conflict of interest
The authors declare that the research was conducted in the absence of financial relationships that could be construed as a potential conflict of interest.
The reviewer HDM declared a past co-authorship with the authors JR, CK, SZ, RK, CJ, AM, MM to the handling editor.
The authors MS, TF, JR, CJ declared that they were an editorial board member of Frontiers, at the time of submission. This had no impact on the peer review process and the final decision.
Publisher’s note
All claims expressed in this article are solely those of the authors and do not necessarily represent those of their affiliated organizations, or those of the publisher, the editors and the reviewers. Any product that may be evaluated in this article, or claim that may be made by its manufacturer, is not guaranteed or endorsed by the publisher.
Footnotes
- ^ Hall G and Fox-Kemper B. Regional mixed layer depth as a climate diagnostic and emergent constraint. (submitted to) Geophys Res Lett (2022).
References
1. Seneviratne SI, Zhang X, Adnan M, Badi W, Dereczynski C, Luca AD, et al. Chapter 11: Weather and climate extreme events in a changing climate. In: Climate Change 2021: The Physical Science Basis. Contribution of Working Group I to the Sixth Assessment Report of the Intergovernmental Panel on Climate Change. Cambridge, United Kingdom and New York, NY, USA: Cambridge University Press (2021). 1513–766. Available at: https://www.ipcc.ch/report/ar6/wg1/downloads/report/IPCC_AR6_WGI_Chapter11.pdf.
2. Allen MR, Friedlingstein P, Girardin CAJ, Jenkins S, Malhi Y, Mitchell-Larson E, et al. Net zero: science, origins, and implications. Annu Rev Environ Resour (2022) 47:849–87. doi: 10.1146/annurev-environ-112320-105050
3. Matthews HD, Zickfeld K. Climate response to zeroed emissions of greenhouse gases and aerosols. Nat Clim Change (2012) 2:338–41. doi: 10.1038/NCLIMATE1424
4. MacDougall AH, Frölicher TL, Jones CD, Rogelj J, Matthews HD, Zickfeld K, et al. Is there warming in the pipeline? A multi-model analysis of the Zero Emissions Commitment from CO2. Biogeosciences (2020) 17:2987–3016. doi: 10.5194/BG-17-2987-2020
5. Matthews HD, Caldeira K. Stabilizing climate requires near-zero emissions. Geophys Res Lett (2008) 35:4705. doi: 10.1029/2007GL032388
6. Hare B, Meinshausen M. How much warming are we committed to and how much can be avoided? Clim Change (2006) 75:111–49. doi: 10.1007/s10584-005-9027-9
7. Masson-Delmotte V, Zhai P, Chen Y, Goldfarb L, Gomis MI, Matthews JBR, et al. Climate Change 2021: The Physical Science Basis. Contribution of Working Group I to the Sixth Assessment Report of the Intergovernmental Panel on Climate Change. IPCC. Cambridge, UK: Cambridge University Press (2021). Available at: www.ipcc.ch.
8. Jenkins S, Sanderson B, Peters G, Frölicher TL, Friedlingstein P, Allen M. The multi-decadal response to net zero CO2 emissions and implications for emissions policy. Geophys Res Lett (2022) 49:e2022GL101047.
9. Lee J-Y, Marotzke J, Bala G, Cao L, Corti S, Dunne JP, et al. Chapter 4. Future global climate: scenario-based projections and near term information. In: In: Climate Change 2021: The Physical Science Basis. Contribution of Working Group I to the Sixth Assessment Report of the Intergovernmental Panel on Climate Change. Cambridge, United Kingdom and New York, NY, USA: Cambridge University Press (2021). 553–672. doi: 10.1017/9781009157896.006
10. Collins M, Knutti R, Arblaster J, Dufresne J-L, Fichefet T, Friedlingstein P, et al. Chapter 12: Long-term climate change: projections, commitments and irreversibility. In: Climate Change: The Physical Science Basis. Contribution of Working Group I to the Fifth Assessment Report of the Intergovernmental Panel on Climate Change. Cambridge, United Kingdom: Cambridge University Press (2013). Available at: https://pure.iiasa.ac.at/id/eprint/10551/.
11. Fox-Kemper B, Hewitt HT, Xiao C, Aðalgeirsdóttir G, Drijfhout SS, Edwards TL, et al. Chapter 9. Ocean, cryosphere and sea level change. Ocean, cryosphere and sea level change. In: Masson-Delmotte V, Zhai P, Pirani A, Connors SL, Péan C, Berger S, Caud N, Chen Y, Goldfarb L, Gomis MI, Huang M, Leitzell K, Lonnoy E, Matthews JBR, Maycock TK, Waterfield T, Yelekçi O, Yu R, Zhou B, editors. Climate Change 2021: The Physical Science Basis. Contribution of Working Group I to the Sixth Assessment Report of the Intergovernmental Panel on Climate Change. Cambridge, United Kingdom and New York, NY, USA: Cambridge University Press (2021). 1211–362. doi: 10.1017/9781009157896.011
12. Matthews JBR, Möller V, van Diemen R, Fuglestvedt JS, Masson-Delmotte V, Méndez C, et al. IPCC, 2021: Annex VII: Glossary. In: Climate Change 2021: The Physical Science Basis. Contribution of Working Group I to the Sixth Assessment Report of the Intergovernmental Panel on Climate Change. Cambridge, United Kingdom and New York, NY, USA: Cambridge University Press (2021). 2215–56. doi: 10.1017/9781009157896.022
13. Zickfeld K, Azevedo D, Mathesius S, Matthews HD. Asymmetry in the climate–carbon cycle response to positive and negative CO2 emissions. Nat Clim Chang (2021) 11:613–7. doi: 10.1038/s41558-021-01061-2
14. Matthews JBR, Babiker M, de Coninck H, Connors S, van Diemen R, Djalante R, et al. IPCC, 2018: Annex I: Glossary [Matthews JBR (editor)]. In: Global warming of 1.5°C. An IPCC Special Report on the impacts of global warming of 1.5°C above pre-industrial levels and related global greenhouse gas emission pathways, in the context of strengthening the global response to the threat of climate change, sustainable development, and efforts to eradicate poverty. Masson-Delmotte V, Zhai P, Pörtner H-O, Roberts D, Skea J, Shukla PR, Pirani A, Moufouma-Okia W, Péan C, Pidcock R, Connors S, Matthews JBR, Chen Y, Zhou X, Gomis MI, Lonnoy E, Maycock T, Tignor M, Waterfield T, editors. Cambridge, UK and New York, NY, USA: Cambridge University Press (2018) 541–62. Available at: https://www.ipcc.ch/sr15/chapter/glossary/.
15. Matthews HD, Weaver AJ. Committed climate warming. Nat Geosci (2010) 3:142–3. doi: 10.1038/ngeo813
16. Sherwood SC, Sen Gupta AS, Schwartz SE. Probability of committed warming exceeding 1.5°C and 2.0°C Paris targets. Environ Res Lett (2022) 17. doi: 10.1088/1748-9326/ac6ff6
17. Damon Matthews HD, Tokarska KB, Rogelj J, Smith CJ, MacDougall AH, Haustein K, et al. An integrated approach to quantifying uncertainties in the remaining carbon budget. Commun Earth Environ (2021) 2:1–11. doi: 10.1038/s43247-020-00064-9
18. Frölicher TL, Joos F, Plattner G-K, Steinacher M, Doney SC. Natural variability and anthropogenic trends in oceanic oxygen in a coupled carbon cycle–climate model ensemble. Global Biogeochem Cycles (2009) 23. doi: 10.1029/2008GB003316
19. Zickfeld K, Eby M, Weaver AJ, Alexander K, Crespin E, Edwards NR, et al. Long-term climate change commitment and reversibility: an EMIC intercomparison. J Clim (2013) 26:5782–809. doi: 10.1175/JCLI-D-12-00584.1
20. Mauritsen T, Pincus R. Committed warming inferred from observations. Nat Clim Change (2017) 7:652–5. doi: 10.1038/nclimate3357
21. Smith CJ, Forster PM, Allen M, Fuglestvedt J, Millar RJ, Rogelj J, et al. Current fossil fuel infrastructure does not yet commit us to 1.5°C warming. Nat Commun (2019) 10:101. doi: 10.1038/s41467-018-07999-w
22. Eby M, Zickfeld K, Montenegro A, Archer D, Meissner KJ, Weaver AJ. Lifetime of anthropogenic climate change: millennial time scales of potential CO2 and surface temperature perturbations. J Clim (2009) 22:2501–11. doi: 10.1175/2008JCLI2554.1
23. Gillett NP, Arora VK, Zickfeld K, Marshall SJ, Merryfield WJ. Ongoing climate change following a complete cessation of carbon dioxide emissions. Nat Geosci (2011) 4:83–7. doi: 10.1038/NGEO1047
24. Plattner G-K, Knutti R, Joos F, Stocker TF, von Bloh W, Brovkin V, et al. Long-term climate commitments projected with climate–carbon cycle models. J Clim (2008) 21:2721–51. doi: 10.1175/2007JCLI1905.1
25. Solomon S, Plattner GK, Knutti R, Friedlingstein P. Irreversible climate change due to carbon dioxide emissions (2009). Available at: www.pnas.org/cgi/content/full/.
26. Dvorak MT, Armour KC, Frierson DMW, Proistosescu C, Baker MB, Smith CJ. Estimating the timing of geophysical commitment to 1.5 and 2.0°C of global warming. Nat Clim Chang (2022) 12:547–52. doi: 10.1038/s41558-022-01372-y
27. Frölicher TL, Paynter DJ. Extending the relationship between global warming and cumulative carbon emissions to multi-millennial timescales. Environ Res Lett (2015) 10:75002. doi: 10.1088/1748-9326/10/7/075002
28. Jones CD, Frölicher TL, Koven C, MacDougall AH, Matthews HD, Zickfeld K, et al. The Zero Emissions Commitment Model Intercomparison Project (ZECMIP) contribution to C4MIP: quantifying committed climate changes following zero carbon emissions. Geosci Model Dev (2019) 12:4375–85. doi: 10.5194/GMD-12-4375-2019
29. Riahi K, Schaeffer R, Arango J, Calvin K, Guivarch C, Hasegawa T, et al. Mitigation pathways compatible with long-term goals. In: IPCC, 2022. Climate Change 2022: Mitigation of Climate Change. Contribution of Working Group III to the Sixth Assessment Report of the Intergovernmental Panel on Climate Change. Cambridge: Cambridge University Press (2022).
30. Rogelj J, Shindell D, Jiang K, Fifita S, Forster P, Ginzburg V, et al. Global warming of 1.5°C: An IPCC Special Report on the Impacts of Global Warming of 1.5°C above Pre-Industrial Levels and Related Global Greenhouse Gas Emission Pathways, in the Context of Strengthening the Global Response to the Threat of Climate Change, Sustainable Development, and Efforts to Eradicate Poverty. Available at: http://www.ipcc.ch/report/sr15/.
31. Joos F, Roth R, Fuglestvedt JS, Peters GP, Enting IG, von Bloh W, et al. Carbon dioxide and climate impulse response functions for the computation of greenhouse gas metrics: A multi-model analysis. Atmos Chem Phys (2013) 13:2793–825. doi: 10.5194/ACP-13-2793-2013
32. Frölicher TL, Winton M, Sarmiento JL. Continued global warming after CO 2 emissions stoppage. Nat Clim Chang (2014) 4:40–4. doi: 10.1038/NCLIMATE2060
33. Williams RG, Roussenov V, Frölicher TL, Goodwin P. Drivers of continued surface warming after cessation of carbon emissions. Geophys Res Lett (2017) 44:633–10642. doi: 10.1002/2017GL075080
34. Ehlert D, Zickfeld K. What determines the warming commitment after cessation of CO2 emissions? Environ Res Lett (2017) 12. doi: 10.1088/1748-9326/aa564a
35. Rugenstein M, Bloch-Johnson J, Gregory J, Andrews T, Mauritsen T, Li C, et al. Equilibrium climate sensitivity estimated by equilibrating climate models. Geophys Res Lett (2020) 47. doi: 10.1029/2019GL083898
36. Hansen J, Russell G, Lacis A, Fung I, Rind D, Stone P. Climate response times: dependence on climate sensitivity and ocean mixing. Science (1985) 229:857–9. doi: 10.1126/science.229.4716.857
37. Wigley TML, Schlesinger ME. Analytical solution for the effect of increasing CO2 on global mean temperature. Nature (1985) 315:649–52. doi: 10.1038/315649a0
38. Meehl GA, Stocker TF, Collins WD, Friedlingstein P, Gaye AT, Gregory JM, et al. Chapter 10. Global climate projections. In: Solomon S, Qin D, Manning M, Chen Z, Marquis M, Averyt KB, Tignor M, Miller HL, editors. Climate Change: The Physical Science Basis. Contribution of Working Group I to the Fourth Assessment Report of the Intergovernmental Panel on Climate Change. Cambridge, United Kingdom and New York, NY, USA: Cambridge University Press (2007). Available at: https://www.ipcc.ch/site/assets/uploads/2018/02/ar4-wg1-chapter10-1.pdf.
39. Canadell JG, Monteiro PMS, Costa MH, da Cunha LC, Cox PM, Eliseev AV, et al. Chapter 5. Global carbon and other biogeochemical cycles and feedbacks. In: Masson-Delmotte PZ, Pirani A, Connors SL, Péan C, Berger S, et al. editors. Climate Change: The Physical Science Basis. Contribution of Working Group I to the Sixth Assessment Report of the Intergovernmental Panel on Climate Change. Cambridge, United Kingdom and New York, NY, USA: Cambridge University Press (2021) 673–816. doi: 10.1017/9781009157896.007
40. Sanderson B. Relating climate sensitivity indices to projection uncertainty. Earth Syst Dynam (2020) 11:721–35. doi: 10.5194/ESD-11-721-2020
41. Koven CD, Arora VK, Cadule P, Fisher RA, Jones CD, Lawrence DM, et al. Multi-century dynamics of the climate and carbon cycle under both high and net negative emissions scenarios. Earth Syst Dynam (2022) 13:885–909. doi: 10.5194/esd-13-885-2022
42. Forster P, Storelvmo T, Armour K, Collins W, Dufresne J-L, Frame D, et al. Chapter 7: The Earth’s energy budget, climate feedbacks and climate sensitivity. In: Masson-Delmotte V, Zhai P, Pirani A, Connors SL, Péan C, Berger S, Caud N, Chen Y, Goldfarb L, Gomis MI, Huang M, Leitzell K, Lonnoy E, Matthews JBR, Maycock TK, Waterfield T, Yelekçi O, Yu R, Zhou B, editors. Climate Change: The Physical Science Basis. Contribution of Working Group I to the Sixth Assessment Report of the Intergovernmental Panel on Climate Change, vol. 132. Cambridge, United Kingdom and New York, NY, USA: Cambridge University Press (2021). doi: 10.1017/9781009157896.009
43. Winton M, Takahashi K, Held IM. Importance of ocean heat uptake efficacy to transient climate change. J Clim (2010) 23:2333–44. doi: 10.1175/2009JCLI3139.1
44. Geoffroy O, Saint-martin D, Olivié DJL, Voldoire A, Bellon G, Tytéca S. Transient climate response in a two-layer energy-balance model. Part I: analytical solution and parameter calibration using CMIP5 AOGCM experiments. J Clim (2013) 26:1841–57. doi: 10.1175/JCLI-D-12-00195.1
45. Held IM, Winton M, Takahashi K, Delworth T, Zeng F, Vallis GK. Probing the fast and slow components of global warming by returning abruptly to preindustrial forcing. J Clim (2010) 23:2418–27. doi: 10.1175/2009JCLI3466.1
46. Soldatenko S, Colman R. Climate variability from annual to multi-decadal timescales in a two-layer stochastic energy balance model: analytic solutions and implications for general circulation models. Tellus A (2019) 71:1554421. doi: 10.1080/16000870.2018.1554421
47. Winton M, Adcroft A, Dunne JP, Held IM, Shevliakova E, Zhao M, et al. Climate sensitivity of GFDL’s CM4.0. J Adv Model Earth Syst (2020) 12:MS001838–e2019MS001838:e2019. doi: 10.1029/2019MS001838
48. Gregory JM. Vertical heat transports in the ocean and their effect on time-dependent climate change. Clim Dyn (2000) 16:501–15. doi: 10.1007/s003820000059
49. Houghton JT, Gylvan L, Filho M, Griggs DJ, Maskell K. An Introduction to simple climate models used in the IPCC second assessment report. (1997). Available at: https://www.ipcc.ch/publication/an-introduction-to-simple-climate-models-used-in-the-ipcc-second-assessment-report/.
50. Meinshausen M, Raper SCB, Wigley TML. Emulating coupled atmosphere-ocean and carbon cycle models with a simpler model, MAGICC6 - Part 1: model description and calibration. Atmos Chem Phys (2011) 11:1417–56. doi: 10.5194/acp-11-1417-2011
51. Raper SCB, Gregory JM, Osborn TJ. Use of an upwelling-diffusion energy balance climate model to simulate and diagnose A/OGCM results. Clim Dyn (2001) 17:601–13. doi: 10.1007/PL00007931
52. Schlesinger ME, Jiang X. Climatic responses to increasing greenhouse gases. Eos Trans Am Geophys Union (1991) 72:593. doi: 10.1029/90EO00417
53. Wigley TML, Raper SCB. Thermal expansion of sea water associated with global warming. Nature (1987) 330:127–31. doi: 10.1038/330127a0
54. Hasselmann K. Determination of ocean wave spectra from Doppler radio return from the sea surface. Nat Phys Sci (1971) 229:16–7. doi: 10.1038/physci229016a0
55. Gebbie G, Huybers P. The mean age of ocean waters inferred from radiocarbon observations: sensitivity to surface sources and accounting for mixing histories. J Phys Oceanogr (2012) 42:291–305. doi: 10.1175/JPO-D-11-043.1
56. Andrews T, Gregory JM, Webb MJ. The dependence of radiative forcing and feedback on evolving patterns of surface temperature change in climate models. J Clim (2015) 28:1630–48. doi: 10.1175/JCLI-D-14-00545.1
57. Rose BEJ, Armour KC, Battisti DS, Feldl N, Koll DDB. The dependence of transient climate sensitivity and radiative feedbacks on the spatial pattern of ocean heat uptake. Geophys Res Lett (2014) 41:1071–8. doi: 10.1002/2013GL058955
58. Winton M, Griffies SM, Samuels BL, Sarmiento JL, Frölicher TLF. Connecting changing ocean circulation with changing climate. J Clim (2013) 26:2268–78. doi: 10.1175/JCLI-D-12-00296.1
59. Siegert M, Pearson P. Reducing uncertainty in 21st century sea-level predictions and beyond. Front Environ Sci (2021) 9:751978. doi: 10.3389/fenvs.2021.751978
60. Arora VK, Katavouta A, Williams RG, Jones CD, Brovkin V, Friedlingstein P, et al. Carbon-concentration and carbon-climate feedbacks in CMIP6 models and their comparison to CMIP5 models. Biogeosciences (2020) 17:4173–222. doi: 10.5194/BG-17-4173-2020
61. Doney SC, Lindsay K, Caldeira K, Campin J-M, Drange H, Dutay J-C, et al. Evaluating global ocean carbon models: the importance of realistic physics. Global Biogeochem Cycles (2004) 18:3017. doi: 10.1029/2003GB002150
62. Sarmiento JL, Sundquist ET. Revised budget for the oceanic uptake of anthropogenic carbon dioxide. Nature (1992) 356:589–93. doi: 10.1038/356589a0
63. Zeebe RE. History of seawater carbonate chemistry, atmospheric CO2, and ocean acidification. Annu Rev Earth Planet Sci (2012) 40:141–65. doi: 10.1146/annurev-earth-042711-105521
64. Ciais P, Sabine C, Bala G, Bopp L, Brovkin V, Canadell J, et al. Carbon and other biogeochemical cycles. In: Climate Change: The Physical Science Basis. Contribution of Working Group I to the Fifth Assessment Report of the Intergovernmental Panel on Climate Change. Cambridge, United Kingdom and New York, NY, USA: Cambridge University Press (2013). Available at: https://www.ipcc.ch/report/ar5/wg1/carbon-and-other-biogeochemical-cycles/.
65. Joos F, Plattner GK, Stocker TF, Marchal O, Schmittner A. Global warming and marine carbon cycle feedbacks on future atmospheric CO2. Science (1999) 284:464–7. doi: 10.1126/SCIENCE.284.5413.464/ASSET/C16D7227-356D-4904-B833-45BE5D681124/ASSETS/GRAPHIC/SE1497417004.JPEG
66. Archer D, Eby M, Brovkin V, Ridgwell A, Cao L, Mikolajewicz U, et al. Atmospheric lifetime of fossil fuel carbon dioxide. Annu Rev Earth Planet Sci (2009) 37:117–34. doi: 10.1146/ANNUREV.EARTH.031208.100206
67. Walker AP, De Kauwe MG, Bastos A, Belmecheri S, Georgiou K, et al. Integrating the evidence for a terrestrial carbon sink caused by increasing atmospheric CO2. New Phytol (2021) 229(5):2413–45. doi: 10.1111/NPH.16866
68. Wieder WR, Cleveland CC, Smith WK, Todd-Brown K. Future productivity and carbon storage limited by terrestrial nutrient availability. Nat Geosci (2015) 8:441–4. doi: 10.1038/NGEO2413
69. Zaehle S, Jones CD, Houlton B, Lamarque JF, Robertson E. Nitrogen availability reduces CMIP5 projections of twenty-first-century land carbon uptake. J Clim (2015) 28:2494–511. doi: 10.1175/JCLI-D-13-00776.1
70. Meyerholt J, Sickel K, Zaehle S. Ensemble projections elucidate effects of uncertainty in terrestrial nitrogen limitation on future carbon uptake. Glob Chang Biol (2020) 26:3978–96. doi: 10.1111/gcb.15114
71. Jones CD, Friedlingstein P. Quantifying process-level uncertainty contributions to TCRE and carbon budgets for meeting Paris Agreement climate targets. Environ Res Lett (2020) 15:074019. doi: 10.1088/1748-9326/AB858A
72. Raupach MR, Davis SJ, Peters GP, Andrew RM, Canadell JG, Ciais P, et al. Sharing a quota on cumulative carbon emissions. Nat Clim Chang (2014) 4:873–9. doi: 10.1038/nclimate2384
73. Macdougall AH. Estimated effect of the permafrost carbon feedback on the Zero Emissions Commitment to climate change. Biogeosciences (2021) 18:4937–52. doi: 10.5194/BG-18-4937-2021
74. Pugh TAM, Jones CD, Huntingford C, Burton C, Arneth A, Brovkin V, et al. A large committed long-term sink of carbon due to vegetation dynamics. Earths Future (2018) 6:1413–32. doi: 10.1029/2018EF000935
75. Waring B, Neumann M, Prentice IC, Adams M, Smith P, Siegert M. Forests and decarbonization – roles of natural and planted forests. Front Glob Change (2020) 3:58. doi: 10.3389/ffgc.2020.00058
76. Lacroix F, Zaehle S, Caldararu S, Schaller J, Stimmler P, Holl D, et al. Mismatch of N release from the permafrost and vegetative uptake opens pathways of increasing nitrous oxide emissions in the high Arctic. Glob Chang Biol (2022) 28:5973–90. doi: 10.1111/GCB.16345
77. O’Neill BC, Tebaldi C, van Vuuren DP, Eyring V, Friedlingstein P, Hurtt G, et al. The scenario model intercomparison project (ScenarioMIP) for CMIP6. Geosci Model Dev (2016) 9:3461–82. doi: 10.5194/gmd-9-3461-2016
78. Ceppi P, Gregory JM. Relationship of tropospheric stability to climate sensitivity and Earth’s observed radiation budget. Proc Natl Acad Sci USA (2017) 114:13126–31. doi: 10.1073/pnas.1714308114
79. Stevens B, Sherwood SC, Bony S, Webb MJ. Prospects for narrowing bounds on Earth’s equilibrium climate sensitivity. Earths Future (2016) 4:512–22. doi: 10.1002/2016EF000376
80. Andrews T, Webb MJ. The dependence of global cloud and lapse rate feedbacks on the spatial structure of tropical Pacific warming. J Climate (2018) 31:641–54. doi: 10.1175/JCLI-D-17-0087.1
81. Bjordal J, Storelvmo T, Alterskjær K, Carlsen T. Equilibrium climate sensitivity above 5°C plausible due to state-dependent cloud feedback. Nat Geosci (2020) 13:718–21. doi: 10.1038/s41561-020-00649-1
82. Schneider T, Kaul CM, Pressel KG. Possible climate transitions from breakup of stratocumulus decks under greenhouse warming. Nat Geosci (2019) 12:163–7. doi: 10.1038/s41561-019-0310-1
83. Wing AA, Reed KA, Satoh M, Stevens B, Bony S, Ohno T. Radiative-convective equilibrium model intercomparison project. Geosci Model Dev (2018) 11:793–813. doi: 10.5194/GMD-11-793-2018
84. Li Q, Reichl BG, Fox-Kemper B, Adcroft AJ, Belcher SE, Danabasoglu G, et al. Comparing ocean surface boundary vertical mixing schemes including langmuir turbulence. J Adv Model Earth Syst (2019) 11:3545–92. doi: 10.1029/2019MS001810
85. Li Q, Fox-Kemper B. Assessing the effects of langmuir turbulence on the entrainment buoyancy flux in the ocean surface boundary layer. J Phys Oceanogr (2017) 47:2863–86. doi: 10.1175/JPO-D-17-0085.1
86. Sallée J-B, Shuckburgh E, Bruneau N, Meijers AJS, Bracegirdle TJ, Wang Z. Assessment of Southern Ocean mixed-layer depths in CMIP5 models: historical bias and forcing response. J Geophys Res Oceans (2013) 118:1845–62. doi: 10.1002/JGRC.20157
87. Van Sundert K, Leuzinger S, Bader MK-F, Chang SX, De Kauwe MG, et al. When things get MESI: The Manipulation Experiments Synthesis Initiative—A coordinated effort to synthesize terrestrial global change experiments. Global Change Biol (2023) 29(7):1922–38. doi: 10.1111/gcb.16585
88. Davies-Barnard T, Meyerholt J, Zaehle S, Friedlingstein P, Brovkin V, Fan Y, et al. Nitrogen cycling in CMIP6 land surface models: progress and limitations. Biogeosciences (2020) 17(20):5129–48. doi: 10.5194/bg-17-5129-2020
89. van Gestel N, Shi Z, van Groenigen KJ, Osenberg CW, Andresen LC, Dukes JS, et al. Predicting soil carbon loss with warming. Nature (2018) 554(7693):E4–5. doi: 10.1038/nature25745
90. Uchida T, Le Sommer J, Stern C, Abernathey RP, Holdgraf C, et al. Cloud-based framework for inter-comparing submesoscale-permitting realistic ocean models. Geosci Model Dev (2022) 15(14):5829–56. doi: 10.5194/gmd-15-5829-2022
91. Germe A, Hirschi JJ-M, Blaker AT, Sinha B. Chaotic variability of the atlantic meridional overturning circulation at subannual time scales. J Phys Oceanogr (2022) 52(5):929–49. doi: 10.1175/JPO-D-21-0100.1
92. Menary MB, Jackson LC, Lozier MS. Reconciling the relationship between the AMOC and labrador sea in OSNAP observations and climate models. Geophys Res Lett (2020) 47(18):e2020GL089793. doi: 10.1029/2020GL089793
93. Muntjewerf L, Sacks WJ, Lofverstrom M, Fyke J, Lipscomb WH, Ernani da Silva C, et al. Description and Demonstration of the Coupled Community Earth System Model v2 – Community Ice Sheet Model v2 (CESM2-CISM2). J Adv Modeling Earth Syst (2021) 13(6):e2020MS002356. doi: 10.1029/2020MS002356
94. Gjermundsen A, Nummelin A, Olivié D, Bentsen M, Seland Ø, Schulz M. Shutdown of Southern Ocean convection controls long-term greenhouse gas-induced warming. Nat Geosci (2021) 14:724–31. doi: 10.1038/s41561-021-00825-x
95. Haskins RK, Oliver KIC, Jackson LC, Drijfhout SS, Wood RA. Explaining asymmetry between weakening and recovery of the AMOC in a coupled climate model. Clim Dyn (2019) 53:67–79. doi: 10.1007/s00382-018-4570-z
96. Schwinger J, Asaadi A, Goris N, Lee H. Possibility for strong northern hemisphere high-latitude cooling under negative emissions. Nat Commun (2022) 13:1095. doi: 10.1038/s41467-022-28573-5
97. de Lavergne C, Palter JB, Galbraith ED, Bernardello R, Marinov I. Cessation of deep convection in the open Southern Ocean under anthropogenic climate change. Nat Clim Chang (2014) 4:278–82. doi: 10.1038/nclimate2132
98. Frölicher TL, Aschwanden MT, Gruber N, Jaccard SL, Dunne JP, Paynter D. Contrasting upper and Deep Ocean oxygen response to protracted global warming. Global Biogeochem Cycles (2020) 34:e2020. doi: 10.1029/2020GB006601
99. Downes SM, Bindoff NL, Rintoul SR. Impacts of climate change on the subduction of mode and intermediate water masses in the Southern Ocean. J Clim (2009) 22:3289–302. doi: 10.1175/2008JCLI2653.1
100. Séférian R, Iudicone D, Bopp L, Roy T, Madec G. Water mass analysis of effect of climate change on air–sea CO2 fluxes: the Southern Ocean. J Climate (2012) 25:3894–908. doi: 10.1175/JCLI-D-11-00291.1
101. Schmittner A, Galbraith ED, Hostetler SW, Pedersen TF, Zhang R. Large fluctuations of dissolved oxygen in the Indian and Pacific Oceans during Dansgaard-Oeschger oscillations caused by variations of North Atlantic Deep Water subduction. Paleoceanography (2007) 22. doi: 10.1029/2006PA001384
102. Toggweiler JR, Gnanadesikan A, Carson S, Murnane R, Sarmiento JL. Representation of the carbon cycle in box models and GCMs: 1. Solubility pump. Global Biogeochem Cycles (2003) 17. doi: 10.1029/2001GB001401
103. Bronselaer B, Winton M, Griffies SM, Hurlin WJ, Rodgers KB, Sergienko OV, et al. Change in future climate due to Antarctic meltwater. Nature (2018) 564:53–8. doi: 10.1038/s41586-018-0712-z
104. Golledge NR, Keller ED, Gomez N, Naughten KA, Bernales J, Trusel LD, et al. Global environmental consequences of twenty-first-century ice sheet melt. Nature (2019) 566:65–72. doi: 10.1038/s41586-019-0889-9
105. Lago V, England MH. Projected slowdown of Antarctic bottom water formation in response to amplified meltwater contributions. J Clim (2019) 32:6319–35. doi: 10.1175/JCLI-D-18-0622.1
106. Moorman R, Morrison AK, Hogg AMC. Thermal responses to Antarctic ice shelf melt in an eddy-rich global ocean–sea ice model. J Clim (2020) 33:6599–620. doi: 10.1175/JCLI-D-19-0846.1
107. Seroussi H, Nowicki S, Simon E, Abe-Ouchi A, Albrecht T, Brondex J, et al. InitMIP-Antarctica: an ice sheet model initialization experiment of ISMIP6. Cryosphere (2019) 13:1441–71. doi: 10.5194/TC-13-1441-2019
108. Fox-Kemper B, Adcroft A, Böning CW, Chassignet EP, Curchitser E, Danabasoglu G, et al. Challenges and prospects in ocean circulation models. Front Mar Sci (2019) 6:65. doi: 10.3389/fmars.2019.00065
109. Munday DR, Johnson HL, Marshall DP. Impacts and effects of mesoscale ocean eddies on ocean carbon storage and atmospheric pCO2. Global Biogeochem Cycles (2014) 28:877–96. doi: 10.1002/2014GB004836
110. Ito T, Woloszyn M, Mazloff M. Anthropogenic carbon dioxide transport in the Southern Ocean driven by Ekman flow. Nature (2010) 463:80–3. doi: 10.1038/nature08687
111. Lachkar Z, Orr JC, Dutay J-C, Delecluse P. Effects of mesoscale eddies on global ocean distributions of CFC-11, CO2, and & Delta;14C. Ocean Sci (2007) 3:461–82. doi: 10.5194/os-3-461-2007
112. Siegelman L, Klein P, Rivière P, Thompson AF, Torres HS, Flexas M, et al. Enhanced upward heat transport at deep submesoscale ocean fronts. Nat Geosci (2020) 13:50–5. doi: 10.1038/s41561-019-0489-1
113. Su Z, Torres H, Klein P, Thompson AF, Siegelman L, Wang J, et al. High-frequency submesoscale motions enhance the upward vertical heat transport in the global ocean. J Geophys Res Oceans (2020) 125:e2020. doi: 10.1029/2020JC016544
114. Sarmiento JL, Gruber N. Ocean biogeochemical dynamics (2006). Available at: https://press.princeton.edu/books/hardcover/9780691017075/ocean-biogeochemical-dynamics.
115. Zeebe RE, Wolf-Gladrow D. Google. Books-ID: g3j3Zn4kEscC. In: CO2 in seawater: equilibrium, kinetics, isotopes. Gulf Professional Publishing (2001).
116. Terhaar J, Frölicher TL, Joos F. Observation-constrained estimates of the global ocean carbon sink from Earth system models. Biogeosciences (2022) 19:4431–57. doi: 10.5194/bg-19-4431-2022
117. Laufkötter C, Vogt M, Gruber N, Aita-Noguchi M, Aumont O, Bopp L, et al. Drivers and uncertainties of future global marine primary production in marine ecosystem models. Biogeosciences (2015) 12:6955–84. doi: 10.5194/bg-12-6955-2015
118. Hauck J, Völker C, Wolf-Gladrow DA, Laufkötter C, Vogt M, Aumont O, et al. On the Southern Ocean CO2 uptake and the role of the biological carbon pump in the 21st century. Global Biogeochem Cycles (2015) 29:1451–70. doi: 10.1002/2015GB005140
119. Heinze C, Meyer S, Goris N, Anderson L, Steinfeldt R, Chang N, et al. The ocean carbon sink – impacts, vulnerabilities and challenges. Earth Syst Dynam (2015) 6:327–58. doi: 10.5194/esd-6-327-2015
120. Bock J, Michou M, Nabat P, Abe M, Mulcahy JP, Olivié DJL, et al. Evaluation of ocean dimethylsulfide concentration and emission in CMIP6 models. Biogeosciences (2021) 18:3823–60. doi: 10.5194/BG-18-3823-2021
121. Frölicher TL, Joos F. Reversible and irreversible impacts of greenhouse gas emissions in multi-century projections with the NCAR global coupled carbon cycle-climate model. Clim Dyn (2010) 35:1439–59. doi: 10.1007/s00382-009-0727-0
122. Plattner G-K, Joos F, Stocker TF, Marchal O. Feedback mechanisms and sensitivities of ocean carbon uptake under global warming. Tellus B Chem Phys Meteorol (2001) 53:564–92. doi: 10.3402/tellusb.v53i5.16637
123. Sarmiento JL, Orr JC, Siegenthaler U. A perturbation simulation of CO2 uptake in an ocean general circulation model. J Geophys Res Oceans (1992) 97:3621–45. doi: 10.1029/91JC02849
124. Hamdan LJ, Wickland KP. Methane emissions from oceans, coasts, and freshwater habitats: new perspectives and feedbacks on climate. Limnol Oceanogr (2016) 61:S3–SS12. doi: 10.1002/lno.10449
125. Martinez-Rey J, Bopp L, Gehlen M, Tagliabue A, Gruber N. Projections of oceanic N2O emissions in the 21st century using the IPSL Earth system model. Biogeosciences (2015) 12:4133–48. doi: 10.5194/BG-12-4133-2015
126. Leifeld J, Wüst-Galley C, Page S. Intact and managed peatland soils as a source and sink of GHGs from 1850 to 2100. Nat Clim Chang (2019) 9:945–7. doi: 10.1038/s41558-019-0615-5
127. Müller J, Joos F. Committed and projected future changes in global peatlands-continued transient model simulations since the Last Glacial Maximum. Biogeosciences (2021) 18:3657–87. doi: 10.5194/BG-18-3657-2021
128. Fleischer K, Rammig A, De Kauwe MG, Walker AP, Domingues TF, Fuchslueger L, et al. Amazon forest response to CO2 fertilization dependent on plant phosphorus acquisition. Nat Geosci (2019) 12:736–41. doi: 10.1038/s41561-019-0404-9
129. Vitousek PM, Porder S, Houlton BZ, Chadwick OA. Terrestrial phosphorus limitation: mechanisms, implications, and nitrogen–phosphorus interactions. Ecol Appl (2010) 20:5–15. doi: 10.1890/08-0127.1
130. Walker AP, Zaehle S, Medlyn BE, De Kauwe MG, Asao S, Hickler T, et al. Predicting long-term carbon sequestration in response to CO2 enrichment: how and why do current ecosystem models differ? Global Biogeochem Cycles (2015) 29:476–95. doi: 10.1002/2014GB004995
131. Pörtner H-O, Roberts DC, Masson-Delmotte V, Zhai P, Tignor M, Poloczanska E, et al. The ocean and cryosphere in a changing climate: special report of the Intergovernmental Panel on Climate Change. 1st ed. Cambridge: Cambridge University Press (2022). doi: 10.1017/9781009157964
132. Turetsky MR, Abbott BW, Jones MC, Anthony KW, Olefeldt D, Schuur EAG, et al. Carbon release through abrupt permafrost thaw. Nat Geosci (2020) 13:138–43. doi: 10.1038/s41561-019-0526-0
133. Falloon PD, Dankers R, Betts RA, Jones CD, Booth BBB, Lambert FH. Role of vegetation change in future climate under the A1B scenario and a climate stabilisation scenario, using the HadCM3C Earth system model. Biogeosciences (2012) 9:4739–56. doi: 10.5194/bg-9-4739-2012
134. Lenton TM, Held H, Kriegler E, Hall JW, Lucht W, Rahmstorf S, et al. Tipping elements in the Earth’s climate system. Proc Natl Acad Sci USA (2008) 105:1786–93. doi: 10.1073/pnas.0705414105
135. Nobre CA, Sampaio G, Borma LS, Castilla-Rubio JC, Silva JS, Cardoso M. Land-use and climate change risks in the Amazon and the need of a novel sustainable development paradigm. Proc Natl Acad Sci USA (2016) 113:10759–68. doi: 10.1073/pnas.1605516113
136. Brienen RJW, Phillips OL, Feldpausch TR, Gloor E, Baker TR, Lloyd J, et al. Long-term decline of the Amazon carbon sink. Nature (2015) 519:344–8. doi: 10.1038/nature14283
137. Gatti LV, Basso LS, Miller JB, Gloor M, Gatti Domingues L, Cassol HLG, et al. Amazonia as a carbon source linked to deforestation and climate change. Nature (2021) 595:388–93. doi: 10.1038/s41586-021-03629-6
138. Boulton CA, Lenton TM, Boers N. Pronounced loss of Amazon rainforest resilience since the early 2000s. Nat Clim Chang (2022) 12:271–8. doi: 10.1038/s41558-022-01287-8
139. Armstrong McKay DIA, Staal A, Abrams JF, Winkelmann R, Sakschewski B, Loriani S, et al. Exceeding 1.5°C global warming could trigger multiple climate tipping points. Science (2022) 377:eabn7950. doi: 10.1126/science.abn7950
140. Cox PM, Pearson D, Booth BB, Friedlingstein P, Huntingford C, Jones CD, et al. Sensitivity of tropical carbon to climate change constrained by carbon dioxide variability. Nature (2013) 494:341–4. doi: 10.1038/nature11882
141. Tian H, Xu R, Canadell JG, Thompson RL, Winiwarter W, Suntharalingam P, et al. A comprehensive quantification of global nitrous oxide sources and sinks. Nature (2020) 586:248–56. doi: 10.1038/s41586-020-2780-0
142. Rogelj J, Huppmann D, Krey V, Riahi K, Clarke L, Gidden M, et al. A new scenario logic for the Paris Agreement long-term temperature goal. Nature (2019) 573:357–63. doi: 10.1038/s41586-019-1541-4
143. Williams RG, Ceppi P, Katavouta A. Controls of the transient climate response to emissions by physical feedbacks, heat uptake and carbon cycling. Environ Res Lett (2020) 15:0940c1. doi: 10.1088/1748-9326/ab97c9
144. Séférian R, Berthet S, Yool A, Palmiéri J, Bopp L, Tagliabue A, et al. Tracking improvement in simulated marine biogeochemistry between CMIP5 and CMIP6. Curr Clim Change Rep (2020) 6:95–119. doi: 10.1007/S40641-020-00160-0
145. Li Q, England MH, Hogg AM, Rintoul SR, Morrison AK. Abyssal ocean overturning slowdown and warming driven by Antarctic meltwater. Nature (2023) 615:841–7. doi: 10.1038/s41586-023-05762-w
146. Dong Y, Pauling AG, Sadai S, Armour KC. Antarctic ice sheet meltwater reduces transient warming and climate sensitivity through the sea-surface temperature pattern effect. Geophys Res Lett (2022) 49:e2022. doi: 10.1029/2022GL101249
147. Liu M, Soden BJ, Vecchi GA, Wang C. The spread of ocean heat uptake efficiency traced to ocean salinity. Geophys Res Lett (2023) 50:e2022. doi: 10.1029/2022GL100171
148. Berthet S, Séférian R, Bricaud C, Chevallier M, Voldoire A, Ethé C. Evaluation of an online grid-coarsening algorithm in a global eddy-admitting ocean biogeochemical model. J Adv Model (2019) 11(6):1759–83. doi: 10.1029/2019MS001644
149. Galbraith ED, Dunne JP, Gnanadesikan A, Slater RD, Sarmiento JL, Dufour CO, et al. Complex functionality with minimal computation: promise and pitfalls of reduced-tracer ocean biogeochemistry models. J Adv Model Earth Syst (2015) 7:2012–28. doi: 10.1002/2015MS000463
150. da Costa ACL, Galbraith D, Almeida S, Portela BTT, da Costa M, Silva Junior J ão de A, et al. Effect of 7 yr of experimental drought on vegetation dynamics and biomass storage of an eastern Amazonian rainforest - da Costa - 2010 - New Phytologist - Wiley Online Library (2010) (Accessed June 6, 2023).
151. Rowland L, da Costa AC L, Oliveira AA R, Oliveira RS, Bittencourt PL, Costa PB, et al. Drought stress and tree size determine stem CO2 efflux in a tropical forest. New Phytol (2018) 218:1393–405. doi: 10.1111/nph.15024
152. Crowther TW, Todd-brown KE O, Rowe W, Wieder WR, Carey J, et al. Quantifying global soil carbon losses in response to warming. (2016). doi: 10.1038/nature20150
153. Burke EJ, Zhang Y, Krinner G. Evaluating permafrost physics in the Coupled Model Intercomparison Project 6 (CMIP6) models and their sensitivity to climate change. Cryosphere (2020) 14:3155–74. doi: 10.5194/TC-14-3155-2020
154. Heede UK, Fedorov AV, Burls NJ. Time scales and mechanisms for the tropical Pacific response to global warming: a tug of war between the ocean thermostat and weaker Walker. J Clim (2020) 33:6101–18. doi: 10.1175/JCLI-D-19-0690.1
155. Seager R, Cane M, Henderson N, Lee D-E, Abernathey R, Zhang H. Strengthening tropical Pacific zonal sea surface temperature gradient consistent with rising greenhouse gases. Nat Clim Chang (2019) 9:517–22. doi: 10.1038/s41558-019-0505-x
156. Koven CD, Sanderson BM, Swann ALS. Much of Zero Emissions Commitment occurs before reaching net zero emissions. Environ Res Lett (2023) 18:014017. doi: 10.1088/1748-9326/acab1a
157. Mace MJ. Mitigation commitments under the Paris agreement and the way forward. Clim Law (2016) 6:21–39. doi: 10.1163/18786561-00601002
Keywords: Zero Emissions Commitment, ZEC, net zero, warming in the pipeline, locked in warming, climate neutrality, climate stabilization, uncertainty
Citation: Palazzo Corner S, Siegert M, Ceppi P, Fox-Kemper B, Frölicher TL, Gallego-Sala A, Haigh J, Hegerl GC, Jones CD, Knutti R, Koven CD, MacDougall AH, Meinshausen M, Nicholls Z, Sallée JB, Sanderson BM, Séférian R, Turetsky M, Williams RG, Zaehle S and Rogelj J. The Zero Emissions Commitment and climate stabilization. Front Sci (2023) 1:1170744. doi: 10.3389/fsci.2023.1170744
Received: 21 February 2023; Accepted: 23 October 2023;
Published: 14 November 2023.
Edited by:
Hayley Jane Fowler, Newcastle University, United KingdomReviewed by:
Jean-Francois Lamarque, McKinsey & Company, United StatesH Damon Matthews, Concordia University, Canada
Copyright © 2023 Palazzo Corner, Siegert, Ceppi, Fox-Kemper, Frölicher, Gallego-Sala, Haigh, Hegerl, Jones, Knutti, Koven, MacDougall, Meinshausen, Nicholls, Sallée, Sanderson, Séférian, Turetsky, Williams, Zaehle and Rogelj. This is an open-access article distributed under the terms of the Creative Commons Attribution License (CC BY). The use, distribution or reproduction in other forums is permitted, provided the original author(s) and the copyright owner(s) are credited and that the original publication in this journal is cited, in accordance with accepted academic practice. No use, distribution or reproduction is permitted which does not comply with these terms.
*Correspondence: Sofia Palazzo Corner, cy5wYWxhenpvLWNvcm5lcjE5QGltcGVyaWFsLmFjLnVr; Martin Siegert, bS5zaWVnZXJ0QGltcGVyaWFsLmFjLnVr; Joeri Rogelj, ai5yb2dlbGpAaW1wZXJpYWwuYWMudWs=