- 1Department of Neurology, The Affiliated Hospital of Xuzhou Medical University, Xuzhou, China
- 2Department of Orthopedic Surgery, Heze Municipal Hospital, Heze, China
- 3Department of Endocrinology, The Affiliated Hospital of Xuzhou Medical University, Xuzhou, China
Background: The relationship between testosterone levels and muscle mass and strength remains contentious. This study aimed to explore the relationship among total serum testosterone levels, muscle mass, and strength in young to middle-aged adults.
Methods: The analysis included 4,495 participants (age 39.2 ± 0.2 years, mean ± SE) from the National Health and Nutrition Examination Survey conducted between 2011 and 2014. Weighted regression models were used to assess the association of testosterone levels with muscle mass and strength.
Results: For male participants, log2-transformed testosterone levels were positively associated with appendicular lean mass adjusted for body mass index (β: 0.05, 95% confidence interval [CI]: 0.03–0.07, P < 0.001) and negatively associated with low muscle mass (odds ratio: 0.40, 95% CI: 0.24–0.67, P = 0.006). However, no significant association was found between testosterone levels and grip strength (β: 1.16, 95% CI: 0.26 to 2.58, P = 0.086) or low muscle strength (odds ratio: 0.51, 95% CI: 0.25–1.04, P = 0.059). For female participants, no significant association was observed between testosterone levels and muscle mass (β: 0.01, 95% CI: 0.02 to −0.01, P = 0.294) or muscle strength (β: 0.14, 95% CI: 0.45 to 0.73, P = 0.508). Restricted cubic spline analysis revealed a linear relationship between total testosterone levels and appendicular lean mass adjusted for body mass index in male participants (nonlinear: P = 0.367).
Conclusion: Our study indicates that testosterone levels are positively associated with muscle mass but not with muscle strength in young to middle-aged males.
1 Introduction
As individuals age, their body composition undergoes significant changes, including a decrease in skeletal muscle mass and strength (Dodds et al., 2016). This reduction is closely linked to a decline in muscle function, which increases the risk of adverse outcomes, such as frailty, falls, fractures, physical disability, and loss of independence (Cruz-Jentoft et al., 2019). With the increase in the global elderly population, the decline in muscle mass and strength has emerged as a critical issue with substantial implications for both the economy and public health. Several factors contribute to this decline, including malnutrition, immobility, neurological changes, and chronic illness along with hormonal changes related to aging, such as alterations in insulin-like growth factor-1 (IGF-1), growth hormones, sex hormones, and glucocorticoids (Coletta and Phillips, 2023; Priego et al., 2021).
Testosterone, the primary androgen hormone, is predominantly produced in the testicles, in smaller quantities, in the ovaries. It is essential for the development and maintenance of male reproductive tissues, sexual function, muscle and bone health, and overall wellbeing in both men and women. Additionally, it plays a significant role in maintaining muscle health. Research has demonstrated that testosterone activates satellite cells, promoting myonuclear accretion and replenishing the satellite cell pool (Kadi, 2008). It also influences pluripotent stem cells, favoring their commitment to myogenic lineage while inhibiting adipogenic differentiation (Bhasin et al., 2003). However, the evidence regarding the association between low testosterone levels and muscle mass and strength is inconsistent (Nam et al., 2018). For example, a cross-sectional study reported a significant positive relationship between total testosterone and muscle strength (Schaap et al., 2005), whereas a longitudinal analysis of two independent samples of older men found no association between low testosterone levels and low muscle strength (Schaap et al., 2008). While testosterone’s role in muscle health is well-established in older men, its impact in young to middle-aged adults and women remains understudied. This gap is critical for understanding early interventions and sex-specific hormonal contributions to muscle maintenance.
National Health and Nutrition Examination Survey (NHANES), a major epidemiological survey conducted by the National Center for Health Statistics (NCHS), provides a large, nationally representative sample of the US population (Zipf et al., 2013). It employs standardized and validated methods to measure testosterone levels and muscle mass and strength. In this study, we focused on the young to middle-aged population, which is an understudied demographic in preventive healthcare and chronic disease management. Additionally, the comprehensive set of covariates that we adjusted for, providing a more nuanced understanding of the relationship between testosterone, muscle mass, and strength in this demographic.
2 Methods
2.1 Data sources
The NHANES consists of voluntary US-based surveys administered every 2 years to assess the health of non-institutionalized individuals through interviews, physical examinations, and diagnostic evaluations (Zipf et al., 2013). The NHANES gathers comprehensive information on various health topics, including demographics, socioeconomics, diet, and health-related issues through in-home interviews, followed by blood sampling at mobile examination centers. The study protocol for the NHANES was reviewed and approved by the NCHS Research Ethics Review Board, and all participants provided written informed consent upon enrollment. This study follows the Strengthening the Reporting of Observational Studies in Epidemiology (STROBE) guidelines (von Elm et al., 2007).
2.2 Study design and population
This study analyzed data from two NHANES survey cycles (2011–2014), which were selected because they included data on testosterone (2011–2016) as well as the muscle mass (2011–2018) and muscle strength (2011–2014). Exclusion criteria included participants with unavailable data on testosterone (5,546) or muscle mass and strength (5,858); a medical history of testicular, prostate, ovarian, or breast cancer (Park et al., 2020); treatment with hormones or hormone modifiers (142); missing demographic data (3,534); missing information on diet, physical activity, and weight (115); and other covariates (200). Figure 1 shows a schematic representation of the participant enrollment procedure.
2.3 Measurement of testosterone
Serum blood samples were collected from participants aged ≥6 years during NHANES clinic visits. Total serum testosterone levels were measured using isotope dilution liquid chromatography-tandem mass spectrometry (ID-LC-MS/MS) method. The detection limits were constant for all of the analytes in the NHANES dataset. Specific subgroups (e.g., adolescents, older adults) are generally lower and may fall below the lower limit of detection. Consequently, this can lead to the exclusion of a significant number of minors from analyses involving testosterone levels. In addition, some participants did not undergo blood tests, this could be due to participant refusal, scheduling conflicts, or other logistical issues.
2.4 Assessment of appendicular lean mass (ALM) and handgrip strength
Dual-energy X-ray absorptiometry (DXA) scans using a Hologic QDR-4500A fan-beam densitometer were conducted to assess bone-free lean mass. Certain participants, such as pregnant individuals or those with contraindications to DXA, and self-reported weight over 450 pounds or height over 6′5”(DXA table limitation) were excluded. ALM was defined as the sum of the lean masses of both arms and legs, expressed in kilograms. To ensure accurate and reliable ALM calculations, participants who did not undergo the full set of DXA scans for both arms and legs were excluded. ALM adjusted for body mass index (BMI) was calculated, and low muscle mass was defined according to the Foundation for the National Institutes of Health (FNIH) criteria (ALMBMI: men, <0.789; women, <0.512) (Studenski et al., 2014). Handgrip strength, assessed using a dynamometer, served as a surrogate measure of muscle strength. Participants were excluded if they were unable to hold the dynamometer with both hands, and individuals who had undergone surgery on either hand within the past 3 months were not tested on the affected hand. The highest grip strength value (GSMAX) for each hand was included in the analysis, with low muscle strength defined as < 26 kg for men and <16 kg for women according to the FNIH sarcopenia project guidelines (Studenski et al., 2014).
2.5 Covariates
Based on the literature, demographic factors (age, gender, education, race, and income) directly influence adherence to exercise regimens and health behaviors, thereby affecting muscle health outcomes (Vezina et al., 2014). Nutritional status, through amino acid-mediated protein synthesis and metabolic balance, is critical for muscle homeostasis (White, 2021). Lifestyle factors including smoking and alcohol consumption contribute to muscle damage (Xia et al., 2024; Frontera et al., 1985). Finally, chronic pathologies such as metabolic and neurological disorders, liver and kidney diseases systemically impair muscle function (Cruz-Jentoft and Sayer, 2019). In terms of testosterone, levels exhibit an age-related decline, particularly in men, while women produce significantly lower amounts (Morley et al., 1997). Dietary influences include low-fat/high-fiber diets reducing serum testosterone concentrations through hormonal modulation (Wang et al., 2005). Exercise has been shown to increase testosterone levels in males (Vogel et al., 1985). Substance use demonstrates divergent effects: smoking shows dose-dependent increases in testosterone (Svartberg et al., 2003), whereas alcohol dependence disrupts hepatic testosterone metabolism, paradoxically elevating levels in chronic users (Walter et al., 2007). Systemic pathologies such as diabetes mellitus and CKD impair Leydig cell function through oxidative stress and inflammatory pathways, ultimately causing hypogonadism (Leisegang et al., 2021). Several important covariates, such as genetic factors, inflammatory markers, detailed dietary data, and other hormone levels, were not included in the analysis due to data limitations in NHANES. Thus, the following covariates were included: sex, age, race, educational level, marital status, poverty-income ratio (PIR), nutrients (energy, protein, carbohydrates, fiber, and fat), physical activity, BMI, and comorbidities (hypertension, diabetes, heart failure, and stroke). Information on sociodemographic factors, lifestyle factors, and comorbidities was collected through interviews and questionnaires. Specifically, race was categorized as non-Hispanic white, non-Hispanic black, Mexican American, and other. Educational level was classified as less than high school (<9 years), high school or equivalent (9–12 years), or higher than high school (>12 years). The marital status was classified as married or cohabiting and living alone. Family income was categorized into three groups based on PIR: low income (<1.3), medium income (1.3–3.5), and high income (>3.5). Data on total energy, protein, carbohydrate, fiber, and fat intakes were collected through a 24-hour dietary recall interview. Physical activity was assessed using the Global Physical Activity Questionnaire, which covers three domains: work-, transportation-, and leisure-time-related activities. Activity levels were converted to metabolic equivalent (MET) scores, with 8.0 MET for one minute of vigorous activity and 4.0 MET for one minute of moderate or transported physical activity. Total physical activity was defined as the total number of MET hours/week (Ainsworth et al., 2011). Smokers were defined as individuals who had smoked at least 100 cigarettes in their lifetime. Participants who answered “Yes” to the question, “In any one year, have you had at least 12 drinks of any type of alcoholic beverage?“, were classified as alcohol drinkers. Comorbidities were assessed using a questionnaire that asked participants, “Have you ever been told by a doctor or other health professional that you had … “. The following pre-existing disorders were considered: hypertension, diabetes, congestive heart failure, stroke, chronic bronchitis, liver condition, arthritis, and cancer or malignancy. The estimated glomerular filtration rate (eGFR) was calculated using the Chronic Kidney Disease Epidemiology Collaboration (CKD-EPI) equation, with CKD defined as an eGFR below 60 mL/min/1.73 m2. This comprehensive inclusion of covariates ensured a robust analysis by accounting for multiple factors that could influence the relationship between testosterone levels and muscle mass and strength.
2.6 Statistical analysis
To produce nationally representative estimates, we accounted for a complex sampling design and sampling weights. Specifically, the dietary day 1 sample weight (WTDRD1) was used for the weighted analysis, with the sampling weight calculated as WTDRD1/2. Patient characteristics were stratified according to sex and divided into testosterone quartiles. Continuous variables are represented as mean and standard error of the mean (SEM) when normally distributed, or as median and interquartile range (IQR) when skewed. Categorical variables are presented as unweighted numbers and weighted percentages. Analysis of variance (ANOVA) or the Kruskal–Wallis test was used for continuous variables. Chi-square tests were used for categorical variables to determine variances between participant characteristics across the testosterone quartiles.
Serum testosterone levels were log2-transformed to approximate a normal distribution and divided into quartiles, with the lowest quartile serving as the reference group. Regression analyses were sex-stratified to account for biological differences in sex hormone levels between the sexes. Linear regression models were used to evaluate the associations among testosterone, ALMBMI and GSMAX. Logistic regression models were used to examine the odds ratio (OR) and 95% confidence interval (95% CI) for the association between testosterone levels and low muscle mass and strength. In our study, we employed a multi-step approach to address potential confounding variables in the regression models. All pre-specified covariates were included simultaneously in multivariable models to adjust for known confounders and avoid stepwise selection biases. This approach minimizes residual confounding and aligns with best practices for observational studies. Model 1: Adjusted for sociodemographic variables including age, race, education level, marital status and family income (For GSMAX, additionally adjusted for BMI). Model 2: Further adjusted for dietary variables (energy, protein, carbohydrates, fiber, and fat) and physical activity. Model 3: Additionally adjusted for smoking status, alcohol consumption, and comorbidities (hypertension, diabetes, heart failure, stroke, chronic bronchitis, liver disease, chronic kidney disease, arthritis, and cancer). The calculation of P for trend was conducted by assigning median values to testosterone quartiles and testing linearity in regression models. For female participants, menopausal status was included as an additional variable in Model 3. Restricted cubic splines (RCS) were used to test for linearity and further investigate the dose-response relationship between testosterone and ALMBMI in men, adjusting for confounding factors consistent with Model 3. Interaction and subgroup analyses were conducted to determine the stability of the relationship between testosterone and ALMBMI across different populations stratified by age, marital status, family income, BMI, protein intake, and smoking and alcohol consumption. P for interaction was derived from likelihood ratio tests comparing models with and without interaction terms. Due to the parameter estimates may be biased as a result of the large proportion of missing value in covariables, we used multiple imputations based on five replications for individuals with missing data about covariates.
All data analyses and visualizations were conducted using R (version 4.3.1; The R Foundation) and Free Statistics software (version 1.9.2; Beijing Free Clinical Medical Technology Co., Ltd., Beijing, China). P < 0.05 was considered statistically significant.
3 Results
3.1 Characteristics of the participants
A total of 4,495 participants selected from NHANES 2011–2014 were involved in our study. The essential characteristics of excluded and included participants are presented in Supplementary Table S1. The baseline clinical characteristics of the study participants, which represent approximately 116.9 million adults in the United States are summarized in Table 1. The weighted mean age was 39.2 years (standard error [SE], 0.2), and 52.5% were men. Total testosterone levels were 20.2-fold higher in men than in women [423.8 (3.6) vs 21.0 (15.0) ng/dL; P < 0.001]. Individuals with higher total testosterone levels tended to be younger, living alone, alcohol consumers, with lower BMI, family income, and higher protein intake, total physical activity, and ALMBMI. Additionally, they had a lower prevalence of hypertension, diabetes, liver disease, arthritis, and low muscle mass.
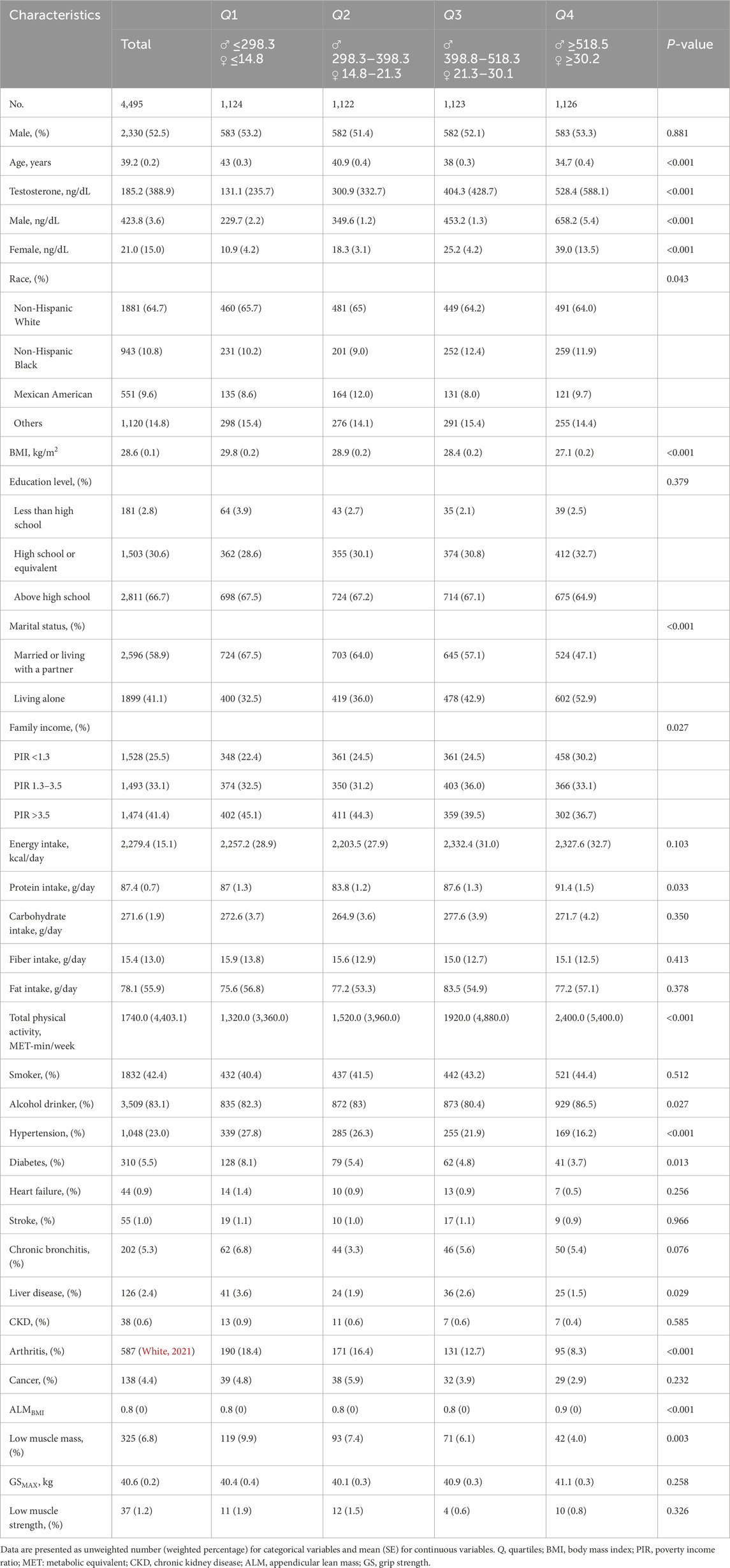
Table 1. Characteristics of participants by sex-stratified quartiles of serum testosterone in the NHANES 2011–2014 cycles.
3.2 Associations between testosterone levels and ALMBMI and GSMAX
Multivariate linear regression analyses of the association between serum testosterone levels and ALMBMI and GSMAX in male participants are shown in Table 2. A significant positive association was discovered between serum testosterone and ALMBMI when analyzed as a continuous variable and subjected to log2-transformation in the crude model (β: 0.06, 95% CI: 0.04–0.07, P < 0.001). This association persisted after adjusting for sociodemographic and dietary variables, total physical activity, smoking status, alcohol consumption status, and comorbidities (Model 3). When testosterone was expressed in quartiles, participants in the highest testosterone quartile had a 0.09 increasement in ALMBMI compared to those in the lowest testosterone quartile (β: 0.09, 95% CI: 0.07–0.11, P < 0.001). After adjusting for potential confounders, this association remained independent (β: 0.08, 95% CI: 0.04–0.11, P = 0.005). Furthermore, a linear relationship was observed between testosterone levels and ALMBMI after adjusting for all potential confounding factors (nonlinearity: P = 0.367) (Figure 2). No clinically meaningful association was observed between testosterone and GSMAX. In women, testosterone levels were not associated with ALMBMI or GSMAX (Supplementary Table S2).
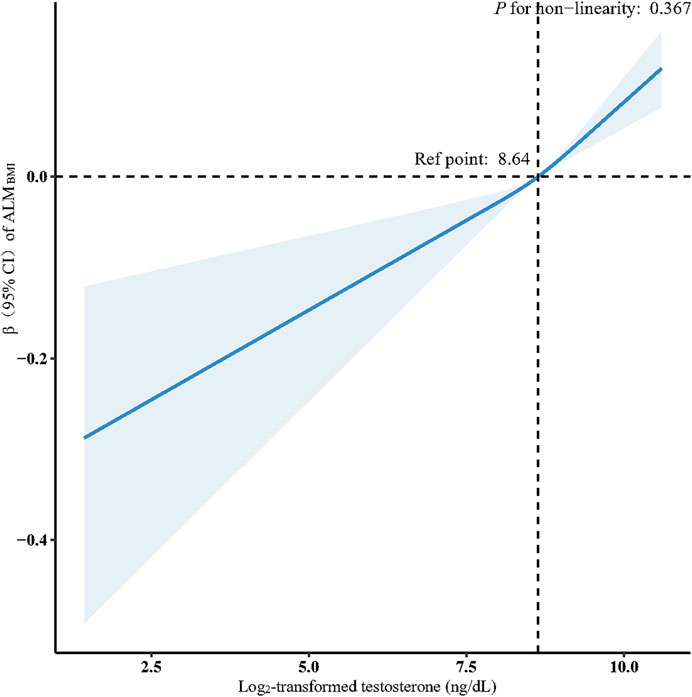
Figure 2. Association between log2-transformed testosterone and ALMBMI in males. Data were fit by a multivariable linear regression model based on restricted cubic splines. Data were adjusted for age, race, educational level, marital status, family income, energy intake, protein intake, carbohydrate intake, dietary fiber, total fat intake, total physical activity, smoker, alcohol drinker, hypertension, diabetes, heart failure, stroke, chronic bronchitis, liver disease, chronic kidney disease, arthritis, and cancer (model 3). Here the median log2-transformed testosterone was defined as the reference standard. Solid and dashed lines indicate the predicted value and 95% CI.
3.3 Associations between testosterone levels and low muscle mass and strength
Logistic regression models showed that higher testosterone levels were associated with a decreased risk of low muscle mass (OR: 0.40, 95% CI: 0.24–0.67, P = 0.006) in men (Table 3). This association was maintained when the testosterone levels were transformed into quartiles. Individuals in the highest testosterone quartile had an 80% lower risk of low muscle mass compared to those in the lowest testosterone quartile (OR: 0.20, 95% CI: 0.06–0.65, P = 0.023). In men, no correlation was observed between testosterone levels and low muscle strength. In women, testosterone levels were not associated with low muscle mass or strength (Supplementary Table S3).
3.4 Subgroup analyses and sensitivity analysis
Stratified and interaction analyses were conducted to determine whether the association between testosterone levels and ALMBMI were consistent across several subgroups (Figure 3). The results showed no significant interactions in any subgroup when stratified by age, marital status, family income, BMI, protein intake, or smoking or alcohol consumption status. Of the 8,344 appropriate participants, 3,849 (46.1%) were further excluded for unavailable data. To address missing data in the analysis, multiple imputations were implemented, which yielded consistent results (Supplementary Table S4).
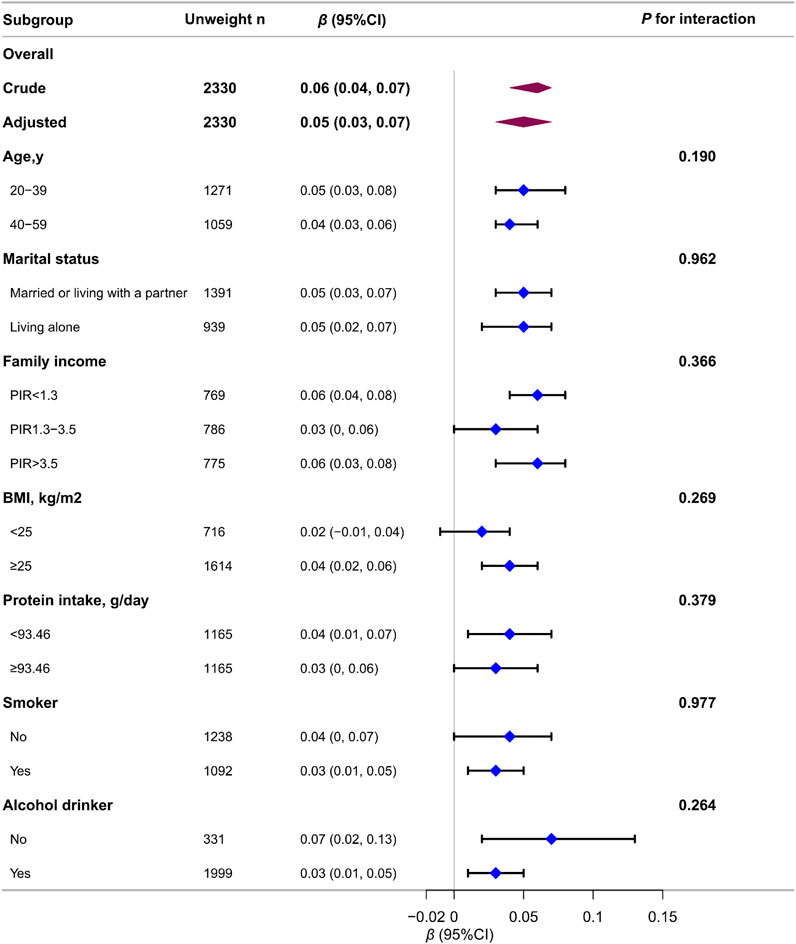
Figure 3. Association between log2-transformed testosterone levels and ALMBMI in males according to the general characteristics. Each stratification was adjusted for all variables (age, race, educational level, marital status, family income, energy intake, protein intake, carbohydrate intake, dietary fiber, total fat intake, total physical activity, smoker, alcohol drinker, hypertension, diabetes, heart failure, stroke, chronic bronchitis, liver disease, chronic kidney disease, arthritis, and cancer) except the stratification factor itself.
4 Discussion
Our analysis of a nationally representative cross-sectional dataset revealed that serum total testosterone was positively associated with ALMBMI and inversely associated with low muscle mass in men, independent of key covariates and potential confounders, such as diet, physical activity, and comorbidities. However, no significant association was observed between testosterone levels and GSMAX or low muscle strength in men. Serum testosterone levels were not associated with muscle mass or muscle strength in women.
Muscle mass and strength decline with age, with strength deterioration occurring threefold faster than mass loss (Goodpaster et al., 2006). Serum testosterone levels also decline by ∼110 ng/dL per decade in older adults (Morley et al., 1997), prompting interest in their interplay with muscle health. Existing studies have documented inconsistent associations between testosterone and muscle outcomes. For example, a recent study in premenopausal women found that free testosterone (FT) levels were associated with muscle mass, but neither FT nor total testosterone was linked to handgrip strength (Alexander et al., 2021). Another study involving 1,879 individuals aged 70–84 years showed that low FT levels predicted decreased handgrip strength in women and reduced physical performance in men, but not muscle mass loss after 2 years of follow-up (Shin et al., 2021). Intervention studies further highlight the impact of testosterone on muscle metabolism. Androgen deprivation therapy (ADT), commonly used in prostate cancer treatment, has been shown to cause significant reductions in lean mass (Chang et al., 2014) and muscle cross-sectional areas (Storer et al., 2017). Conversely, testosterone replacement therapy (TRT) has been found to improve muscle mass and strength in hypogonadal men. A recent double-blind randomized controlled trial (RCT) reported modest but significant improvements in muscle mass and strength after 3 years of testosterone administration in older men with low-to-normal testosterone levels (Hildreth et al., 2013). However, other studies have not observed a direct effect of TRT on muscle mass or strength (Kolind et al., 2022; Barnouin et al., 2021). These inconsistencies may be due to variations in study populations, doses, modes of administration, and other factors such as diet and physical activity levels. Unlike the aforementioned studies, our research specifically focuses on young to middle-aged adults and systematically dissecting sex-based disparities in testosterone’s role in muscle health.
In men, a positive linear relationship was found between testosterone and muscle mass but not muscle strength, suggesting a disconnect between muscle mass preservation and functional strength gains. This disconnect may arise from multifaceted biological and physiological mechanisms. First, muscle strength depends not only on mass but also on neuromuscular efficiency and motor unit recruitment (Sale, 1988). Aging diminishes the number and function of alpha motor neurons, leading to fewer muscle fibers being activated during contraction. Even if muscle mass is preserved, impaired neural drive reduces force generation (Delmonico et al., 2009). Second, type II fibers, critical for strength and power, exhibit more pronounced atrophy and decrease in number with age, while type I fibers are less affected (Lexell, 1995). Third, age-related mitochondrial dysfunction reduces ATP synthesis, limiting muscle contractile capacity. This affects strength disproportionately to mass (Short et al., 2005). Lastly, aging blunts the muscle protein synthesis response to anabolic stimuli (e.g., testosterone, insulin, exercise), impairing strength maintenance (Mitchell et al., 2012). In addition, testosterone alone may not be a key determinant of strength when considering the broader context of health and lifestyle. Diet is a key factor influencing testosterone levels and muscle health. A Western-style diet, characterized by high consumption of bread, pastries, dairy products, and desserts, is associated with lower testosterone levels and an increased risk of hypogonadism (Hu et al., 2018). Conversely, adherence to a Mediterranean diet may improve testosterone levels (La et al., 2018). Physical activity is another critical factor. Regular exercise, especially resistance training, has been shown to potentiate muscle hypertrophy and increase testosterone levels (Vingren et al., 2010). In contrast, a sedentary lifestyle is associated with lower testosterone levels and musculoskeletal diseases (Park et al., 2020). Smoking and drinking behavior not only affects testosterone levels, but also negatively impacts muscle health (Xia et al., 2024; Ko et al., 2020). Health conditions like diabetes, chronic kidney disease, and heart failure can directly impact muscle strength due to their effects on overall health and metabolic processes while neurological conditions affecting the nervous system can also influence muscle strength by altering muscle control and function (Cruz-Jentoft and Sayer, 2019).
In women, no correlation was observed between testosterone levels and ALMBMI, low muscle mass, GSMAX, or low muscle strength. The sex-specific differences in the relationship between testosterone levels and muscle health may be attributed to several biological and hormonal factors. First, women in our cohort had testosterone levels approximately 20-fold lower than men, which may be below the threshold required to exert anabolic effects on muscle tissue (Shin et al., 2021). Alternatively, the effect of testosterone on muscle health in women may be less significant than that of the other factors including age, nutrition, estrogen, physical activity, lifestyle and health status. Second, variations in androgen receptor expression and sensitivity between men and women may influence how testosterone affects muscle tissue. Studies have shown that androgen receptor density and activity can differ between sexes, potentially explaining the disparate effects of testosterone on muscle health (Schuppe et al., 2017). Third, men and women have different muscle fiber compositions, with men generally having a higher proportion of type II (fast-twitch) fibers (Staron et al., 2000), which are more responsive to testosterone and contribute to greater muscle mass and strength. Nationally representative data revealed significant sex-based variations in testosterone levels: in men, the 10th-90th percentile range for total testosterone was 150–698 ng/dL, while in women it spanned 7.1–49.8 ng/dL (Vesper et al., 2015). Furthermore, physiological testosterone levels exhibited a positive correlation with lean mass and an inverse association with fat mass in males (Mouser et al., 2016). Conversely, in pre-menopausal women, total testosterone levels demonstrated no significant relationship with either lean mass or handgrip strength (Alexander et al., 2021).
While our study found no significant association between testosterone levels and grip strength, the distinction between muscle strength and power is critical as grip strength measurements in NHANES may not fully capture dynamic power-related tasks. Muscle power, which reflects the ability to generate force rapidly, declines earlier and more precipitously with aging than muscle strength and is more strongly associated with functional limitations and fall risk in older adults (Bean et al., 2003). Studies have demonstrated that testosterone administration improves stair-climbing power, leg press power, and chest press strength and power (Storer et al., 2017). These effects are dose-dependent, with higher testosterone levels correlating with greater improvements in muscle performance (Storer et al., 2003). While testosterone supplementation improves maximal voluntary strength and leg power, its effects on endurance performance and overall physical function in older adults remain unclear (Bhasin et al., 2001). Future studies incorporating validated measures of muscle power would provide a more comprehensive understanding of testosterone’s role in muscle function and its clinical implications.
The large sample size of the NHANES dataset minimized non-sampling and measurement errors due to the rigorous study design and data-processing criteria. We accounted for complex sampling weights and sample designs to ensure the representativeness and generalizability of our findings. However, this study has several limitations. This study focused on young to middle-aged adults (aged 20–59 years) in the United States, as the NHANES dataset does not include individuals outside this age range in the DXA scans examination. While our findings provide valuable insights into the relationship between testosterone levels and muscle health in this demographic, the generalizability to older adults (≥60 years) or non-U.S. populations remain uncertain. Future studies incorporating broader age ranges and diverse cohorts are needed to validate these findings and explore potential age-related differences. The cross-sectional nature of our study is another limitation, as it precludes the establishment of causality. Our findings are based on a snapshot of data collected at a single point in time, which means that we cannot infer temporal relationships between testosterone levels and muscle health outcomes. Longitudinal studies are needed to determine whether the observed associations are causal and to explore the potential bidirectional relationships between testosterone levels and muscle mass/strength over time. Despite accounting for various lifestyle- and health-related confounders, residual confounding may still exist. For example, certain genetic variants may affect muscle fiber type distribution, muscle protein synthesis, and androgen receptor sensitivity (Ahmetov et al., 2012). Chronic low-grade inflammation is common in older adults and can affect muscle health independently of testosterone levels (Degens, 2010). Detailed dietary intake data, such as specific micronutrient levels (e.g., vitamin D, calcium), were not fully accounted for in our analysis (Annweiler et al., 2009). Other hormones, such as estrogen, growth hormone, and insulin-like growth factor-1 (IGF-1), interact with testosterone and could influence muscle health. Self-reported data on diet, physical activity, and comorbidities are susceptible to recall bias. Moreover, different types of physical activity—such as aerobic and resistance exercise—may have varying impacts on muscle health. However, the NHANES dataset lacks detailed information on the specific types of physical activity, limiting our ability to draw more nuanced conclusions. Additionally, free testosterone is generally considered a more accurate indicator of biologically active hormone levels compared to total testosterone. Unfortunately, our analysis was constrained by the available data, as the NHANES dataset does not provide measurements of free testosterone. Finally, given the differences in baseline data between included and excluded participants, it is imprudent to dismiss the potential impact of non-random omissions on our results.
5 Conclusion
Our findings suggest an epidemiological association between serum testosterone levels and muscle mass, but not muscle strength, in young to middle-aged males. Additional research is necessary to explore the complexities of this relationship further and to address gaps observed in female participants.
Data availability statement
The original contributions presented in the study are included in the article/Supplementary Material, further inquiries can be directed to the corresponding author/s.
Ethics statement
The studies involving humans were approved by the National Center for Health Statistics Research Ethics Review Board. The studies were conducted in accordance with the local legislation and institutional requirements. Written informed consent for participation was not required from the participants or the participants’ legal guardians/next of kin in accordance with the national legislation and institutional requirements.
Author contributions
WZ: Conceptualization, Data curation, Supervision, Validation, Writing–original draft, Writing–review and editing. ZC: Methodology, Supervision, Validation, Writing–original draft. DS: Software, Writing–review and editing. LG: Supervision, Writing–review and editing. QL: Conceptualization, Data curation, Formal Analysis, Resources, Supervision, Writing–review and editing.
Funding
The author(s) declare that no financial support was received for the research and/or publication of this article.
Acknowledgments
We thank Jie Liu (Department of Vascular and Endovascular Surgery, Chinese PLA General Hospital) for his helpful review and comments regarding the manuscript. We acknowledge the participants and investigators of the NHANES. We would like to thank Editage (www.editage.com) for English language editing.
Conflict of interest
The authors declare that the research was conducted in the absence of any commercial or financial relationships that could be construed as a potential conflict of interest.
Generative AI statement
The author(s) declare that no Generative AI was used in the creation of this manuscript.
Publisher’s note
All claims expressed in this article are solely those of the authors and do not necessarily represent those of their affiliated organizations, or those of the publisher, the editors and the reviewers. Any product that may be evaluated in this article, or claim that may be made by its manufacturer, is not guaranteed or endorsed by the publisher.
Supplementary material
The Supplementary Material for this article can be found online at: https://www.frontiersin.org/articles/10.3389/fphys.2025.1512268/full#supplementary-material
References
Ahmetov I. I., Vinogradova O. L., Williams A. G. (2012). Gene polymorphisms and fiber-type composition of human skeletal muscle. Int. J. Sport Nutr. Exerc Metab. 22 (4), 292–303. doi:10.1123/ijsnem.22.4.292
Ainsworth B. E., Haskell W. L., Herrmann S. D., Meckes N., Bassett D. R., Tudor-Locke C., et al. (2011). 2011 Compendium of Physical Activities: a second update of codes and MET values. Med. Sci. Sports Exerc 43 (8), 1575–1581. doi:10.1249/MSS.0b013e31821ece12
Alexander S. E., Abbott G., Aisbett B., Wadley G. D., Hnatiuk J. A., Lamon S. (2021). Total testosterone is not associated with lean mass or handgrip strength in pre-menopausal females. Sci. Rep. 11 (1), 10226. doi:10.1038/s41598-021-89232-1
Annweiler C., Bridenbaugh S., Schott A.-M., Berrut G., Kressig R. W., Beauchet O. (2009). Vitamin D and muscle function: new prospects? Biofactors 35 (1), 3–4. doi:10.1002/biof.4
Barnouin Y., Armamento-Villareal R., Celli A., Jiang B., Paudyal A., Nambi V., et al. (2021). Testosterone replacement therapy added to intensive lifestyle intervention in older men with obesity and hypogonadism. J. Clin. Endocrinol. Metabolism 106 (3), e1096–e1110. doi:10.1210/clinem/dgaa917
Bean J. F., Leveille S. G., Kiely D. K., Bandinelli S., Guralnik J. M., Ferrucci L. (2003). A comparison of leg power and leg strength within the InCHIANTI study: which influences mobility more? J. Gerontol. A Biol. Sci. Med. Sci. 58 (8), 728–733. doi:10.1093/gerona/58.8.m728
Bhasin S., Taylor W. E., Singh R., Artaza J., Sinha-Hikim I., Jasuja R., et al. (2003). The mechanisms of androgen effects on body composition: mesenchymal pluripotent cell as the target of androgen action. J. Gerontol. A Biol. Sci. Med. Sci. 58 (12), M1103–M1110. doi:10.1093/gerona/58.12.m1103
Bhasin S., Woodhouse L., Storer T. W. (2001). Proof of the effect of testosterone on skeletal muscle. J. Endocrinol. 170 (1), 27–38. doi:10.1677/joe.0.1700027
Chang D., Joseph D. J., Ebert M. A., Galvão D. A., Taaffe D. R., Denham J. W., et al. (2014). Effect of androgen deprivation therapy on muscle attenuation in men with prostate cancer. J. Med. Imaging Radiat. Oncol. 58 (2), 223–228. doi:10.1111/1754-9485.12124
Coletta G., Phillips S. M. (2023). An elusive consensus definition of sarcopenia impedes research and clinical treatment: a narrative review. Ageing Res. Rev. 86, 101883. doi:10.1016/j.arr.2023.101883
Cruz-Jentoft A. J., Bahat G., Bauer J., Boirie Y., Bruyère O., Cederholm T., et al. (2019). Sarcopenia: revised European consensus on definition and diagnosis. Age Ageing 48 (4), 601. doi:10.1093/ageing/afz046
Cruz-Jentoft A. J., Sayer A. A. (2019). Sarcopenia. Lancet London, Engl. 393 (10191), 2636–2646. doi:10.1016/S0140-6736(19)31138-9
Degens H. (2010). The role of systemic inflammation in age-related muscle weakness and wasting. Scand. J. Med. Sci. Sports 20 (1), 28–38. doi:10.1111/j.1600-0838.2009.01018.x
Delmonico M. J., Harris T. B., Visser M., Park S. W., Conroy M. B., Velasquez-Mieyer P., et al. (2009). Longitudinal study of muscle strength, quality, and adipose tissue infiltration. Am. J. Clin. Nutr. 90 (6), 1579–1585. doi:10.3945/ajcn.2009.28047
Dodds R. M., Syddall H. E., Cooper R., Kuh D., Cooper C., Sayer A. A. (2016). Global variation in grip strength: a systematic review and meta-analysis of normative data. Age Ageing 45 (2), 209–216. doi:10.1093/ageing/afv192
Frontera W. R., Meredith C. N., O'Reilly K. P., Knuttgen H. G., Evans W. J. (1985). Strength conditioning in older men: skeletal muscle hypertrophy and improved function. J. Appl. Physiol. 64 (3):1038–1044. doi:10.1097/00008483-198806000-00005
Goodpaster B. H., Park S. W., Harris T. B., Kritchevsky S. B., Nevitt M., Schwartz A. V., et al. (2006). The loss of skeletal muscle strength, mass, and quality in older adults: the health, aging and body composition study. J. Gerontol. A Biol. Sci. Med. Sci. 61 (10), 1059–1064. doi:10.1093/gerona/61.10.1059
Hildreth K. L., Barry D. W., Moreau K. L., Vande Griend J., Meacham R. B., Nakamura T., et al. (2013). Effects of testosterone and progressive resistance exercise in healthy, highly functioning older men with low-normal testosterone levels. J. Clin. Endocrinol. Metabolism 98 (5), 1891–1900. doi:10.1210/jc.2013-2227
Hu T.-Y., Chen Y. C., Lin P., Shih C.-K., Bai C.-H., Yuan K.-C., et al. (2018). Testosterone-associated dietary pattern predicts low testosterone levels and hypogonadism. Nutrients 10 (11), 1786. doi:10.3390/nu10111786
Kadi F. (2008). Cellular and molecular mechanisms responsible for the action of testosterone on human skeletal muscle. A basis for illegal performance enhancement. Br. J. Pharmacol. 154 (3), 522–528. doi:10.1038/bjp.2008.118
Ko D. H., Kim S. E., Lee J. Y. (2020). Prevalence of low testosterone according to health behavior in older adults men. Healthc. (Basel) 9 (1), 15. doi:10.3390/healthcare9010015
Kolind M. I., Christensen L. L., Caserotti P., Andersen M. S., Glintborg D. (2022). Muscle function following testosterone replacement in men on opioid therapy for chronic non-cancer pain: a randomized controlled trial. Andrology 10 (3), 551–559. doi:10.1111/andr.13147
La J., Roberts N. H., Yafi F. A. (2018). Diet and men's sexual health. Sex. Med. Rev. 6 (1), 54–68. doi:10.1016/j.sxmr.2017.07.004
Leisegang K., Roychoudhury S., Slama P., Finelli R. (2021). The mechanisms and management of age-related oxidative stress in male hypogonadism associated with non-communicable chronic disease. Antioxidants (Basel) 10 (11), 1834. doi:10.3390/antiox10111834
Lexell J. (1995). Human aging, muscle mass, and fiber type composition. J. Gerontol. A Biol. Sci. Med. Sci. 50 (Spec No), 11–16. doi:10.1093/gerona/50a.special_issue.11
Mitchell W. K., Williams J., Atherton P., Larvin M., Lund J., Narici M. (2012). Sarcopenia, dynapenia, and the impact of advancing age on human skeletal muscle size and strength; a quantitative review. Front. Physiol. 3, 260. doi:10.3389/fphys.2012.00260
Morley J. E., Kaiser F. E., Perry H. M., Patrick P., Morley P. M., Stauber P. M., et al. (1997). Longitudinal changes in testosterone, luteinizing hormone, and follicle-stimulating hormone in healthy older men. Metabolism 46 (4), 410–413. doi:10.1016/s0026-0495(97)90057-3
Mouser J. G., Loprinzi P. D., Loenneke J. P. (2016). The association between physiologic testosterone levels, lean mass, and fat mass in a nationally representative sample of men in the United States. Steroids 115, 62–66. doi:10.1016/j.steroids.2016.08.009
Nam Y. S., Lee G., Yun J. M., Cho B. (2018). Testosterone replacement, muscle strength, and physical function. World J. Mens. Health 36 (2), 110–122. doi:10.5534/wjmh.182001
Park J. H., Moon J. H., Kim H. J., Kong M. H., Oh Y. H. (2020). Sedentary lifestyle: overview of updated evidence of potential health risks. Korean J. Fam. Med. 41 (6), 365–373. doi:10.4082/kjfm.20.0165
Priego T., Martín A. I., González-Hedström D., Granado M., López-Calderón A. (2021). Role of hormones in sarcopenia. Vitam. Horm. 115, 535–570. doi:10.1016/bs.vh.2020.12.021
Sale D. G. (1988). Neural adaptation to resistance training. Med. Sci. Sports Exerc 20 (5 Suppl. l), S135–S145. doi:10.1249/00005768-198810001-00009
Schaap L. A., Pluijm S. M. F., Deeg D. J. H., Penninx B. W., Nicklas B. J., Lips P., et al. (2008). Low testosterone levels and decline in physical performance and muscle strength in older men: findings from two prospective cohort studies. Clin. Endocrinol. (Oxf) 68 (1), 42–50. doi:10.1111/j.1365-2265.2007.02997.x
Schaap L. A., Pluijm S. M. F., Smit J. H., van Schoor N. M., Visser M., Gooren L. J. G., et al. (2005). The association of sex hormone levels with poor mobility, low muscle strength and incidence of falls among older men and women. Clin. Endocrinol. (Oxf) 63 (2), 152–160. doi:10.1111/j.1365-2265.2005.02315.x
Schuppe E. R., Pradhan D. S., Thonkulpitak K., Drilling C., Black M., Grober M. S. (2017). Sex differences in neuromuscular androgen receptor expression and sociosexual behavior in a sex changing fish. PloS One 12 (5), e0177711. doi:10.1371/journal.pone.0177711
Shin H. E., Walston J. D., Kim M., Won C. W. (2021). Sex-specific differences in the effect of free testosterone on sarcopenia components in older adults. Front. Endocrinol. 12, 695614. doi:10.3389/fendo.2021.695614
Short K. R., Bigelow M. L., Kahl J., Singh R., Coenen-Schimke J., Raghavakaimal S., et al. (2005). Decline in skeletal muscle mitochondrial function with aging in humans. Proc. Natl. Acad. Sci. U. S. A. 102 (15), 5618–5623. doi:10.1073/pnas.0501559102
Staron R. S., Hagerman F. C., Hikida R. S., Murray T. F., Hostler D. P., Crill M. T., et al. (2000). Fiber type composition of the vastus lateralis muscle of young men and women. J. Histochem Cytochem 48 (5), 623–629. doi:10.1177/002215540004800506
Storer T. W., Basaria S., Traustadottir T., Harman S. M., Pencina K., Li Z., et al. (2017). Effects of testosterone supplementation for 3 Years on muscle performance and physical function in older men. J. Clin. Endocrinol. Metabolism 102 (2), 583–593. doi:10.1210/jc.2016-2771
Storer T. W., Magliano L., Woodhouse L., Lee M. L., Dzekov C., Dzekov J., et al. (2003). Testosterone dose-dependently increases maximal voluntary strength and leg power, but does not affect fatigability or specific tension. J. Clin. Endocrinol. Metabolism 88 (4), 1478–1485. doi:10.1210/jc.2002-021231
Studenski S. A., Peters K. W., Alley D. E., Cawthon P. M., McLean R. R., Harris T. B., et al. (2014). The FNIH sarcopenia project: rationale, study description, conference recommendations, and final estimates. J. Gerontol. A Biol. Sci. Med. Sci. 69 (5), 547–558. doi:10.1093/gerona/glu010
Svartberg J., Midtby M., Bønaa K. H., Sundsfjord J., Joakimsen R. M., Jorde R. (2003). The associations of age, lifestyle factors and chronic disease with testosterone in men: the Tromsø Study. Eur. J. Endocrinol. 149 (2), 145–152. doi:10.1530/eje.0.1490145
Vesper H. W., Wang Y., Vidal M., Botelho J. C., Caudill S. P. (2015). Serum total testosterone concentrations in the US household population from the NHANES 2011-2012 study population. Clin. Chem. 61 (12), 1495–1504. doi:10.1373/clinchem.2015.245969
Vezina J. W., Der Ananian C. A., Greenberg E., Kurka J. (2014). Sociodemographic correlates of meeting US Department of Health and Human Services muscle strengthening recommendations in middle-aged and older adults. Prev. Chronic Dis. 11, E162. doi:10.5888/pcd11.140007
Vingren J. L., Kraemer W. J., Ratamess N. A., Anderson J. M., Volek J. S., Maresh C. M. (2010). Testosterone physiology in resistance exercise and training: the up-stream regulatory elements. Sports Med. 40 (12), 1037–1053. doi:10.2165/11536910-000000000-00000
Vogel R. B., Books C. A., Ketchum C., Zauner C. W., Murray F. T. (1985). Increase of free and total testosterone during submaximal exercise in normal males. Med. Sci. Sports Exerc 17 (1), 119–123. doi:10.1249/00005768-198502000-00019
von Elm E., Altman D. G., Egger M., Pocock S. J., Gøtzsche P. C., Vandenbroucke J. P., et al. (2007). Strengthening the Reporting of Observational Studies in Epidemiology (STROBE) statement: guidelines for reporting observational studies. BMJ 335 (7624), 806–808. doi:10.1136/bmj.39335.541782.AD
Walter M., Gerhard U., Gerlach M., Weijers H.-G., Boening J., Wiesbeck G. A. (2007). Controlled study on the combined effect of alcohol and tobacco smoking on testosterone in alcohol-dependent men. Alcohol Alcohol 42 (1), 19–23. doi:10.1093/alcalc/agl089
Wang C., Catlin D. H., Starcevic B., Heber D., Ambler C., Berman N., et al. (2005). Low-fat high-fiber diet decreased serum and urine androgens in men. J. Clin. Endocrinol. Metabolism 90 (6), 3550–3559. doi:10.1210/jc.2004-1530
White J. P. (2021). Amino acid trafficking and skeletal muscle protein synthesis: a case of supply and demand. Front. Cell Dev. Biol. 9, 656604. doi:10.3389/fcell.2021.656604
Xia X., Xiang S., Hua L., Sun Q., Wang R. (2024). The relationship between lifestyles and sarcopenia-related traits: a two-sample Mendelian randomization study. Arch. Gerontol. Geriatr. 116, 105169. doi:10.1016/j.archger.2023.105169
Keywords: sex differences, testosterone, muscle mass, muscle strength, NHANES
Citation: Zhang W, Cui Z, Shen D, Gao L and Li Q (2025) Testosterone levels positively linked to muscle mass but not strength in adult males aged 20–59 years: a cross-sectional study. Front. Physiol. 16:1512268. doi: 10.3389/fphys.2025.1512268
Received: 21 October 2024; Accepted: 10 March 2025;
Published: 15 April 2025.
Edited by:
José Antonio de Paz, University of León, SpainReviewed by:
Francesco Sessa, University of Catania, ItalyLeandro Manfredi, Federal University of the Southern Frontier, Brazil
Wael Ramadan, Mansoura University, Egypt
Copyright © 2025 Zhang, Cui, Shen, Gao and Li. This is an open-access article distributed under the terms of the Creative Commons Attribution License (CC BY). The use, distribution or reproduction in other forums is permitted, provided the original author(s) and the copyright owner(s) are credited and that the original publication in this journal is cited, in accordance with accepted academic practice. No use, distribution or reproduction is permitted which does not comply with these terms.
*Correspondence: Qingyun Li, zhsy0720@163.com
†These authors have contributed equally to this work