- 1Department of Pharmaceutical Sciences, Health Sciences Center, Federal University of Espírito Santo, Vitória, Espírito Santo, Brazil
- 2Biotechnology Program/RENORBIO, Health Sciences Center, Federal University of Espírito Santo, Vitória, Espírito Santo, Brazil
- 3Biochemistry Program, Health Sciences Center, Federal University of Espírito Santo, Vitória, Espírito Santo, Brazil
Ovarian cancer is the second most common malignant neoplasm of gynecological origin and the leading cause of death from cancer in the female reproductive system worldwide. This scenario is largely due to late diagnoses, often in advanced stages, and the development of chemoresistance by cancer cells. These challenges highlight the need for alternative treatments, with immunotherapy being a promising option. Cancer immunotherapy involves triggering an anti-tumor immune response and developing immunological memory to eliminate malignant cells, prevent recurrence, and inhibit metastasis. Some ongoing research investigate potentially immunological advancements in the field of cancer vaccines, immune checkpoint blockade, CAR-T cell, and other strategies.
1 Introduction to ovarian cancer immunotherapy
Ovarian cancer (OC) ranks first among deaths caused by gynecological malignant neoplasms around the world (American Cancer Society, 2024). OC’s dramatic epidemiological scenario is related to diagnoses in advanced stages of the disease, due to the absence of pathognomonic signs and symptoms for early diagnosis (Doubeni et al., 2016), coupled with the first-choice therapeutic regimens chemoresistance acquisition by OC cells (Ghoneum et al., 2021). These conditions require other ways to treat these patients, other than surgeries and non-specific conventional chemotherapy. In consequence, different immunotherapy approaches have arisen as relevant alternatives to overcome this treatment obstacle (Bund et al., 2022).
OC immunotherapy involves the induction of an anti-tumor immune response and the development of immunological memory. This process not only can eradicate malignant cells within the primary tumor site, thereby averting recurrence, but also hampers the metastatic spread to distant anatomical locations (Cha et al., 2020). Presently, the Food and Drugs Administration (FDA) has sanctioned some distinct immunotherapeutic modalities for OC or is actively investigating them in clinical trials (Cha et al., 2020). These approaches can be categorized into active and passive immunotherapies.
Active immunotherapy harnesses the immune system to identify and target specific cancer antigens. It includes vaccines that stimulate the patient’s immune response, or chimeric antigen receptor (CAR) T-cell therapy, which involves the reintroduction of genetically engineered T-cells in the patient (Rui et al., 2023). On the other hand, passive immunotherapy modulates the activity of a patient’s immune system response, as observed with immune checkpoint inhibitors (ICIs) molecules (Rui et al., 2023). In this review, we compile the latest findings concerning OC immunotherapy strategies.
2 Therapeutic OC vaccines
To handle the adverse effects of common therapies for cancer, immunotherapy strategies emerged as a cancer-specific alternative capable of targeting the tumor and causing minimal impact on normal tissues (Aly, 2012; Zhu and Yu, 2022). They are significant considering the usual therapeutic approaches such as surgery, chemotherapy, and radiotherapy which besides the adverse effects show a lack of specificity for tumors (Kaczmarek et al., 2023). Therapeutic cancer vaccination is a strategy of immunotherapy developed to elicit or boost antitumor adaptive immune responses to detect and eliminate them (Luo, et al., 2024; Chambers, 2011). This response is specifically direct against malignant cells leading to the inhibition of tumor growth and/or recurrence (Siminiak et al., 2022). Cancer vaccines use diverse mechanisms to provoke the immune system and develop a specific anti-tumor response (Shafabakhsh et al., 2019; American Cancer Society, 2020) and immunological memory that may prevent recurrences (Janes et al., 2024).
OC, which is a challenging disease to diagnose and treat, usually shows resistance to available chemotherapies and frequently relapses with more aggressiveness (Acharya et al., 2024). The clinical characteristics demonstrate the importance of developing novel therapeutic strategies to treat and overcome chemoresistance in OC. In this scenario, different cancer vaccines have been studied in OC. The main mechanisms of cancer vaccines involve the induction of dendritic cells (DCs) potent antigen-presenting cells (APCs), these cells identify and present the antigen for other cells using major histocompatibility complex (MHC) molecules (Lin M. et al., 2022). Also secrete IL-10, IL-12, IL-23, and TNF-β to stimulate the differentiation of immune system cells (Zhang X. et al., 2021). CD8+ cytotoxic T lymphocytes (CTLs) recognize the antigens presented on MHC class I molecules, leading to their activation and proliferation, consequently, attacking and destroying the tumor (Kaczmarek et al., 2023). CD4+ helper T cells recognize peptides presented on MHC class II molecules and provide support to other immune cells. B cells can also be activated resulting in the production of antibodies specific to the tumor-associated antigens (TAAs) (Janes et al., 2024). These antibodies can directly bind to tumor cells, aiding in their destruction. The vaccine also aims to induce a memory response, which enhances immune protection and provides a more robust response upon future encounters with tumor cells expressing the same TAAs (Fan et al., 2023), see Figure 1.
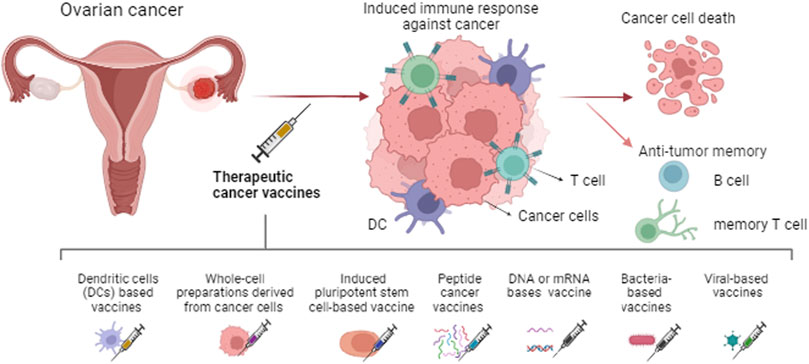
Figure 1. Types of therapeutic cancer vaccines and the main mechanism induced. The immune system response is generated against the tumor leading to cancer cell death and immunological memory.
DCs based vaccines depend on ex vivo modification of DCs from the patient or cells created in the laboratory. Immune-stimulating agents or tumor-specific antigens (TSAs) obtained from tumor cells or genetic material are applied to mature and activate these cells followed by reinfusion into the patient. Once reinfused, these cells interact with T cells, B cells, and natural killer (NK) cells (Lv et al., 2020; Fan et al., 2023). The activation of immune system cells, as mentioned above, enhances the immune response and destroys cancer cells (Laureano et al., 2022). The use of this kind of vaccine has shown relevant results, for example, a study using an autologous dendritic cell-based vaccine with tumor lysate after systemic chemotherapy resulted in a decrease in progression rate, as well as improved overall survival in OC (Zhang X. et al., 2021). A Th17-inducing folate receptor alpha (FRα)-loaded DCs vaccine, resulted in the development of Th1, Th17, and antibody responses to FRα in most patients. These processes are associated with prolonged recurrence-free survival and induce antigen-specific immunity (Block et al., 2020). Another approach combined a whole tumor lysate-pulsed dendritic cell vaccine with bevacizumab, cyclophosphamide, aspirin, and interleukin-2, this vaccine produced T-cell responses and was associated with increased overall survival of patients (Tanyi et al., 2021).
A similar mechanism is induced by the whole-cell preparations or lysates derived from cancer cells reintroduced into the patient (Chiang et al., 2011). Cells are sourced from the patient’s tumor or established cancer cell lines, aiming to prevent their growth and pathogenicity the cells are inactivated or genetically modified (Kaczmarek et al., 2023; Pérez-Baños et al., 2023). Another approach utilizes induced pluripotent stem cell (iPSC)-based cancer vaccines. iPSCs are created from somatic cells and then differentiated into tumor microenvironment (TME)-specific cells, such as tumor-associated fibroblasts, endothelial cells, or immune cells (Chehelgerdi et al., 2023). These iPSC-derived cells express antigens characteristic of the TME, including TSAs or molecules associated with immunosuppression. When administered to the patient, these cells are recognized by immune cells, triggering a robust immune response (Ouyang et al., 2019). Zhang Z. et al. (2012) used human embryonic stem cells as a OC prevention vaccine in rats, this vaccine caused anti-tumor responses and enhanced tumor rejection in the animal models.
Peptide cancer vaccines are also an emerging treatment for OC, using specific epitope peptides derived from TAAs or TSAs (Abd-Aziz and Poh, 2022). This vaccine can stimulate the immune system after being administered and taked up by APCs (Wada et al., 2016; Liu et al., 2024a). Recent studies in phase I or II use mutated p53 peptides (The cancer-testis antigen, named New York esophageal squamous cell carcinoma-1, NY-ESO-1) and also apply diverse technologies to treat OC in association with co-therapies (Odunsi, 2017; Siminiak et al., 2022). Vaccines made from a peptide or antigen may help the body build an effective immune response to kill tumor cells, functioning as a booster for the patient’s anti-tumor immune response and the combination with chemotherapy may induce the death of more tumor cells (Bund et al., 2022; Odunsi, 2017).
In a phase I/IIa trial (Brown et al., 2019) used E39 in patients HLA-A2+, this is an immunogenic peptide derived from the folate-binding protein, frequently found overexpressed in multiple malignancies. When associated with granulocyte macrophage-colony stimulating factor (GM-CSF) was able to improve disease-free survival (DFS) of endometrial cancer and OC patients (90.0% vs. Control Group: 42.9%). Targeting folate receptor (FR) a vaccine was tested in patients with OC or breast cancer. The vaccine stimulated or increased immunity in more than 90% of patients and the FR T cell responses were detectable for at least 12 months. The results demonstrate the benefits of boosting immunity to tumors expressing FR antigen (Kalli et al., 2018). O’Cearbhaill et al. (2019) combined a polyvalent vaccine conjugate responsible for inducing antibody responses (Globo-H, GM2, MUC1-TN, TF) with adjuvant OPT-821 in patients with OC in remission after chemotherapy. Vaccine + OPT-821 compared to OPT-821 alone was modestly more immunogenic.
Cancer vaccines can also involve genetic material (DNA and RNA) encoding TAAs. This DNA or RNA is taken up by cells, such as DCs, and the TAAs are presented on the surface of APCs after being processed. In this process, the activation and proliferation of CD8+ CTLs are induced and CD4+ helper T cells provide support to other immune cells (Pandya et al., 2023). Additionally, B cells can be activated by presented TAAs and induce the production of antibodies. These antibodies can bind directly to tumor cells, aiding in their destruction (Barbier et al., 2022). The vaccine also aims to induce a memory response, which enhances immune protection and ensures a more effective response upon future encounters with tumor cells expressing the same TAAs (Wang B. et al., 2023a). Lu et al. (2023) using immuno-bioinformatics developed a model of a multi-epitope mRNA self-adjuvant vaccine targeting CA-125 neoantigen in breast and ovarian cancers. This in silico analysis provided evidence of using this neoantigen in a mRNA-based vaccine. Posity results were observed using the SynCon FSHR DNA vaccine. In this study synthetic consensus (SynCon) approach was capable of breaking immune tolerance to follicle-stimulating hormone receptor (FSHR). The treatment induced robust CD8+ and CD4+ cellular immune responses and FSHR-redirected antibodies in mice, as well, delayed the progression of aggressive OC model with peritoneal carcinomatosis (Perales-Puchalt et al., 2019).
Neoantigen DNA vaccines were used by Bhojnagarwala et al. (2021) to target ∼40 neoantigens. These plasmid-based vaccines were able to provoke long-term immune responses against lung and ovarian cancer and protected animals from tumor growth for 89 days after the final vaccination. Another DNA vaccine platform targeting tumor neoantigens was applied against lung and ovarian cancers affecting the tumor progression and survival in mouse models. In this pre-clinical study, the vaccine was able to generate potent CD8+ T-cell antitumor–specific responses in vivo. Interestingly, when neoantigen-specific T cells were expanded from immunized mice they were also able to kill tumor cells ex vivo (Duperret et al., 2019).
Bacteria-based cancer vaccines use engineered bacteria to stimulate the immune system (Zhou et al., 2023). These modified bacteria interact with immune cells, initiating an inflammatory response and triggering the production of pro-inflammatory cytokines, chemokines, and other signaling molecules (Zalatan et al., 2024). Viral-based cancer vaccines use engineered viruses to stimulate the immune response directly. These modified viruses interact with immune cells such as DCs, macrophages, and NK cells, triggering an inflammatory response along with the release of pro-inflammatory cytokines and chemokines (Xu et al., 2024). Immune cells then phagocytose the virus particles, and TAAs expressed by the virus or introduced into infected cells are processed and presented to T cells (Muthukutty and Yoo, 2023). Cowpea mosaic virus co-delivered with irradiated OC cells comprises an prophylactic vaccine against a model of OC in mice. After two vaccinations most of the mice (72%) reject the tumor challenges, and survived subsequent rechallenges, indicating immunologic memory (Stump et al., 2021).
These approaches highlight the diverse strategies being employed to develop effective vaccines for OC, with ongoing research focused on optimizing these therapies and evaluating their clinical efficacy. The actual scenario for cancer vaccines is due to years of research and discoveries. Nevertheless, the heterogeneity of the immune system and the capacity of cancer cells to evade immune system attacks, even when naturally endogenous or when induced by vaccine makes this process a challenge. This is why more in-depth studies must be completed to enable the large use of these therapies.
3 CAR-T cell therapy in OC
CAR-T cells are genetically engineered to recognize and attack TSAs (June et al., 2018), bypassing the need of MHC molecules presentation, and behaving as active drugs against tumors (Maus and June, 2016). FDA approved CAR-T therapy in 2017 (reviewed by Yi-Ju et al., 2023), with two treatments, Yescarta (axicabtagene ciloleucel) and Kymriah (tisagenlecleucel), specifically for certain lymphomas and leukemia (Food and Drug Administration, 2024). Despite its clinical success in treating blood cancers, CAR-T therapy can lead to serious complications (reviewed by Brudno and Kochenderfer, 2024). These include cytokine release syndrome (CRS), which can cause extreme symptoms like high fevers, organ failure, and even death (Reagan and Neelapu, 2021). Another risk is “on-target, off-tumor toxicity,” where CAR-T cells attack healthy tissues, causing severe harm (Flugel et al., 2023). Additionally, the required lymphodepleting chemotherapy before CAR-T infusion is genotoxic, raising the risk of secondary cancers and other diseases (Yeh et al., 2020). Since then, extensive global research has been conducted on various hematologic and solid tumors to evaluate the safety and efficacy of CAR-T therapy and it has shown significant success in treating hematologic cancers, with six other FDA approvals, and holds promise as a new treatment option for OC (reviewed by Cappell and Kochenderfer, 2023).
Solid tumors present significant challenges for CAR-T cell therapy due to their heterogeneity and the scarcity of known tumor-specific epitopes (Labanieh and Mackall, 2023). Unlike hematological malignancies, solid tumors often result in toxicity when targeting overexpressed antigens (reviewed by Baker et al., 2023). Additionally, the TME creates physical and immunological barriers that limit CAR-T cell effectiveness (reviewed by Albelda, 2024). To overcome these obstacles, researchers are exploring intratumoral injections (Tchou et al., 2017), peptide and nanoparticle booster vaccines (MA et al., 2019; Reinhard et al., 2020), engineered cytokine-driven expansion (Sockolosky et al., 2018), and modifying the TME with oncolytic viruses and genome editing techniques like CRISPR-Cas9 (reviewed by Baker et al., 2023).
Emerging clinical data show promise for CAR-T cells targeting solid tumors, including prostate cancer (prostate-specific membrane antigen) (Narayan et al., 2022), gastrointestinal cancer (CLDN18.2) (Qi et al., 2022), glioblastoma (IL13RA2 or EGFRv3) (Sampson et al., 2020), and neuroblastoma (GD2) (Del Bufalo et al., 2023). Despite these advances, challenges persist due to the scarcity of unique, tumor-specific targets (Macpherson et al., 2020). In OC, potential targets identified include mesothelin (MSLN) (Schuster et al., 2017), Muc16 (Coelho et al., 2018), TAG72 (Murad et al., 2018), FR (Rodriguez-Garcia et al., 2017), and FSHR (Perales-Puchalt et al., 2017). Furthermore, recent studies have explored the feasibility, safety, and anti-tumor activity of the first-in-human approach of targeting CLDN6 with CAR-T therapy and combining it with a CAR-amplifying vaccine (Mackensen et al., 2023), given the frequent detection of high-level CLDN6 in epithelial OC, endometrial carcinoma, and other solid tumors (Jaeger et al., 2014). Hence, CAR technology using NK cells is being studied for a range of solid tumors, as well as OC (reviewed by Dagher and Posey, 2023). Table 1 highlights some studies that evaluate CAR technology use in OC and other cancer types.
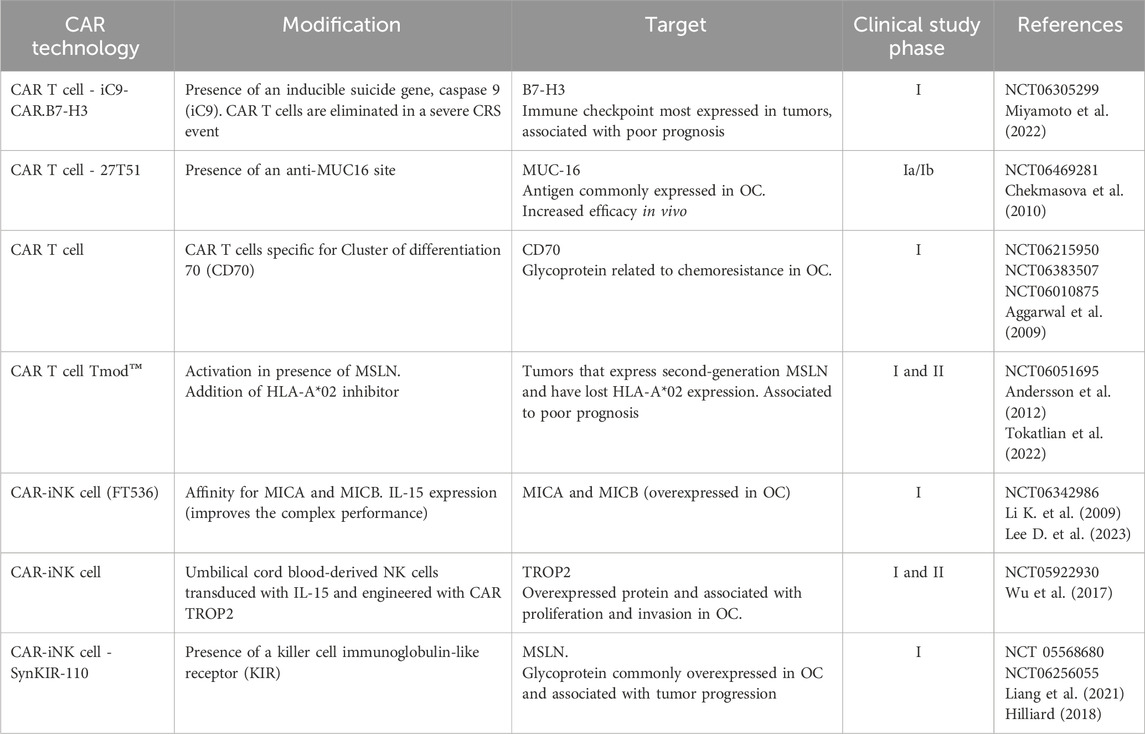
Table 1. Ongoing studies evaluating CAR technology in OC and other tumors treatments. Clinical trials that have recently started using CAR cell technology in OC are currently in “recruiting” status. Some CAR cells have undergone modifications to become more specific or to avoid some side effects, such as CRS.
4 Exosomes in OC treatment
Exosomes represent a promising tool and target for immunotherapy in OC (Zhou W. et al., 2021). Although they are physiological components, their role in cancer remains somewhat ambiguous. In the context of immunotherapy, these lipophilic vesicles are crucial for facilitating communication among immune system cells, which can either elicit positive immune responses or lead to immunosuppression (Kugeratski and Raghu, 2021). Taylor et al. (2003) demonstrated that membrane fragments, which include exosomes and other lipid vesicles, derived from OC cells can induce T cell apoptosis. The influence of exosomes and similar membrane fragments on orchestrating immune system responses has been explored in various cancer types, including breast (Morrissey et al., 2021), lung (Alipoor et al., 2018), pancreatic (Shen et al., 2020), glioma (Li M. et al., 2022), and colorectal cancer (Zhao S. et al., 2020). Consequently, several key aspects regarding the role of exosomes in immunotherapy will be discussed below.
Exosomes are a category of extracellular vesicles with a lipid bilayer, measuring approximately 30–150 nm, found in various body fluids such as blood, urine, saliva, and cerebrospinal fluid (He et al., 2018; Gong et al., 2023). They are believed to have a dual role in the TME (Li X. and Wang, 2017). Exosomes can both promote and inhibit tumors and carry many potential biomarkers for OC (Gong et al., 2023). In normal cells, these small vesicles can interact with membrane receptors or fuse with cells to release components such as proteins, RNA, DNA, mRNA, miRNA, long non-coding RNA (lncRNA), and lipids, aiding in cellular communication, extracellular matrix maintenance, and immune system modulation (Pegtel and Gould, 2019; Kaushik and Cuervo, 2015; Ramirez and Marcilla, 2021; Zhu et al., 2024; Tian et al., 2022). In cancer cells, exosomes perform similar functions but carry components that promote proliferation, migration, invasion, chemoresistance, and other processes that enhance malignancy, complicating treatment, such as modulation of the TME (Bhattacharya et al., 2024; Yim et al., 2020; Li X. et al., 2021b).
In OC, exosomes play a dual role in the acquisition of chemoresistance, a process caused by the lack of cancer cells response to chemotherapy, often resulting in treatment failure (Tian et al., 2022; Liu H. et al., 2024b; Carmi et al., 2024). In this context, their malignant role in OC was elucidated by Pan et al. (2024). Their study found that exosomes derived from OC stem cells were responsible for increasing chemoresistance and proliferation while inhibiting apoptosis in the cisplatin-resistant SKOV3 cell line. Meanwhile, exosomes derived from ascites were observed to carry a lncRNA that sensitized high-grade serous ovarian cancer (HGSOC) cells to cisplatin chemotherapy, a standard drug for this OC subtype. Additionally, it was demonstrated that exosomes carried a lncRNA that reduced cell proliferation, migration, and invasion in both in vitro and in vivo experiments (Liu H. et al., 2024b).
Another factor complicating chemotherapy treatment is the low oxygenation within tumors, resulting from reduced blood perfusion. Wang Q. et al. (2024) analyzed this process and observed that tumor-derived exosomes contributed, in part, to the decreased oxygenation through the previously mentioned mechanism, by altering the tumor vascular network and thereby impeding chemotherapy.
In addition to their ambiguous role, exosomes may serve as a potential tool for OC therapy, as demonstrated in the study by Shimizu et al. (2024). In this study, exosomes were extracted from a cell culture of fibroblasts from OC patients and were loaded with siRNAs targeting a proto-oncogene, the MET receptor. This treatment inhibited OC cells proliferation, migration, and invasion. Another study showed that it is possible to create targeted exosomes for OC treatment (Mousaei Ghasroldasht et al., 2024). Mousaei Ghasroldasht et al. (2024) developed what they termed “enhanced exosomes” using a culture of human umbilical cord-derived mesenchymal stem cells (hUC-MSCs), observing that these exosomes contained proteins and miRNAs capable of regulating and sensitizing OC. Another study, by Kim et al. (2023), used a nanotechnology-modified exosome in glioma to evaluate its effectiveness. The results indicate that there was regulation of the TME and decreased tumor progression both in vitro and in vivo. Furthermore, exosomes can be utilized as biomarkers for an improved and earlier diagnosis, addressing the delays often seen in most cases (Bhavsar et al., 2024; Zhu et al., 2024; Xiao et al., 2022). There is also evidence that these vesicles carry RNAs related to chemoresistance and, therefore, may serve as biomarkers for this process, which precedes clinical interventions (Asare-Werehene et al., 2020; Li T. et al., 2021a). These findings suggest that exosomes have intriguing therapeutic potential warranting further investigation. Therefore, deepening studies in this area is crucial to better understand the contribution of these components in OC immunotherapy and the underlying mechanisms of different kinds of exosomes and how they influence on tumor response to treatment (Figure 2).
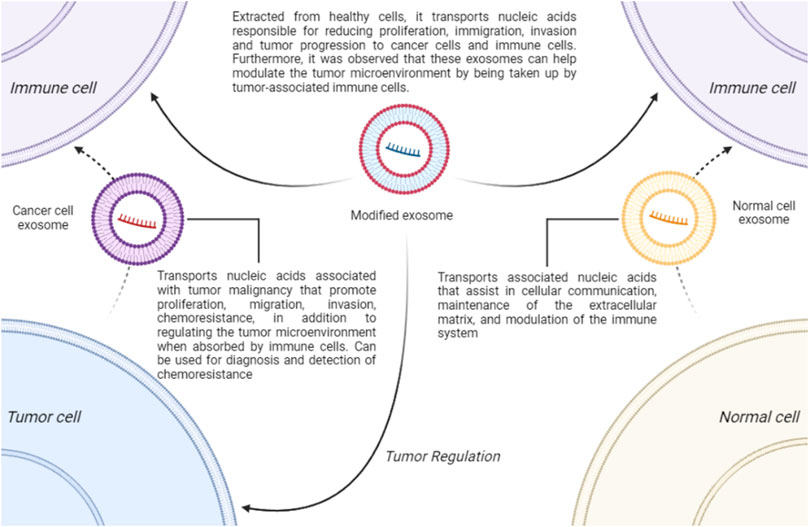
Figure 2. The Role of Exosomes. Exosomes play a physiological role in cellular communication, immune system modulation, and maintenance of the extracellular matrix. In OC, they are associated with tumor progression, proliferation, migration, invasion, and regulation of TME. Tumor cell-derived exosomes can serve as biomarkers for diagnosis and early detection of chemoresistance. Additionally, modified exosomes, such as those derived from hUC-MSCs or engineered using nanotechnology, may aid in treatment by reducing tumor progression and potentially modulating the TME.
5 Antibody-based therapies for OC treatment
Therapeutic monoclonal antibodies have been successfully developed for the treatment of various cancer types (Hafeez et al., 2020).
In this context, with the biotechnology advancement, antibody-drug conjugates (ADCs) have been developed, representing one of the newest classes of cancer medications, with approvals for the treatment of solid tumors as well as hematological malignancies. ADCs exhibit high selectivity for tumors, thereby minimizing their systemic exposure, which potentially leads to an improved therapeutic index, offering greater efficacy and fewer side effects (Dean et al., 2021). To minimize off-target toxicity, the target antigen should be exclusively or preferentially expressed in cancer cells, with minimal expression in healthy tissues (Hafeez et al., 2020). Several monoclonal antibody-based immunotherapies have already been approved by FDA (Zhou et al., 2023). However, numerous clinical trials are still underway with promising prospects for the treatment of OC, including ADCs such as JNJ-78306358, ivonescimab, ipilimumab, durvalumab, oregovomab, catumaxomab, abagovomab, daclizumab and mirvetuximab, which was approved by the FDA in 2022 but remains under study for application in OC treatment (Dilawari et al., 2023).
5.1 Immune checkpoint inhibitors (ICIs)
Cancer cells develop several complex mechanisms to evade the immune system in the TME, among which the inhibition of T cell activity by the PD-1/PD-L1 (Daud et al., 2016) and CTLA-4/B7 pathways can be highlighted (Tang Q. et al., 2022a). PD-1 is an immune receptor expressed on the surface of various immune cells, and the interaction between PD-1 and PD-L1, which is highly expressed on the surface of tumor cells and tumor-infiltrating cells, results in the inhibition of T cell activity, rendering the anti-tumor immune response ineffective and favoring immune evasion (Daud et al., 2016; Naimi et al., 2022; Tang Q. et al., 2022a). Furthermore, the binding of PD-1 to its ligand can inhibit T cell proliferation, B lymphocyte differentiation, and the production of cytokines such as Interferon-gamma (IFN-Y) (Tang S. et al., 2022b).
On the other hand, another immune checkpoint associated with tumor cell evasion is CTLA-4, an inhibitory receptor belonging to the immunoglobulin superfamily (Van Coillie et al., 2020). CTLA-4 is primarily expressed on activated T cells and, like PD-1, has an immunomodulatory function (Tang S. et al., 2022b). The interaction of CTLA-4 with its ligands, B7-1 (CD80) and B7-2 (CD86), expressed on APCs and tumor cells, transmits a signal that negatively regulates or interrupts T cell activity, thereby decreasing the immune response against cancer cells (Naimi et al., 2022; Tang S. et al., 2022b; Van Coillie et al., 2020).
From this perspective, ICIs represent a promising class of drugs in immunotherapy against OC, targeting PD-1/PD-L1 and CTLA-4. They have already demonstrated broad bioactivity and stable response in the treatment of various types of tumors (Naimi et al., 2022; Tang S. et al., 2022b), including OC (Disis et al., 2019).
5.1.1 PD-1/PD-L1 inhibitors
Recent studies conducted by Friedman et al. (2024) involving 35 patients demonstrated that the use of nivolumab, a PD-1 inhibitory monoclonal antibody, in the treatment of uterine cancer and OC with DNA mismatch repair deficiency (dMMR) showed clinical efficacy with an objective response rate (ORR) of 57%. Additionally, 64.7% of patients experienced progression-free survival (PFS) at 24 weeks, and treatment toxicity was moderate. However, while the results are promising, further studies with a larger cohort representing the population of patients with OC-dMMR are necessary, as well as the identification of additional predictive biomarkers for treatment response and resistance.
On the other hand, another notable ICI is ivonescimab, also known as AK112 and SMT112. It is a humanized bispecific antibody whose single-chain variable fragments (ScFv) bind to the C-terminus of each anti-VEGF antibody heavy chain (Wang L. et al., 2023b), forming a complex with high affinity for PD-1 (Zhao et al., 2023). Ivonescimab is currently being evaluated in clinical studies for its anti-PD-1 and anti-VEGF-A activities, with the goal of preventing tumor progression through the inhibition of angiogenesis (Apte et al., 2019). The anticipated outcomes of this inhibition include reduced immunosuppression and decreased tumor angiogenesis (Dhillon, 2024). However, clinical trials have encountered challenges in achieving satisfactory results.
In the phase Ia study by Frentzas et al. (2024), the activity of ivonescimab was evaluated in 19 patients with platinum-resistant OC. Among these patients, 68.4% had received more than three lines of prior therapy. Of the 19 patients, five achieved a partial response, including 3 with high-grade serous pathology and 2 with clear cell pathology, resulting in an ORR of 26.3%. Additionally, the study observed that the disease remained stable for more than 12 months in four patients who had previously been treated with bevacizumab. However, further clinical studies are needed to determine more appropriate dosages and to conduct additional analyses in combination therapies.
5.1.2 CTLA-4 inhibitors
One of the promising antibody-drugs in this class is ipilimumab, a monoclonal antibody targeting CTLA-4 (Saad and Kasi, 2023). Knisely et al. (2024) conducted a phase Ib study evaluating intraperitoneal ipilimumab and nivolumab in patients with recurrent gynecological neoplasms with peritoneal carcinomatosis. The study included 23 patients: 18 with OC, 2 with uterine cancer, and 3 with cervical cancer. In this study, a partial response was observed in two patients (8.7%), one with OC and one with uterine cancer, with a response duration of 14.8 months. Additionally, the treatment safety was assessed, revealing that two patients (8.7%) experienced adverse effects classified as grade 3 or higher. Despite these adverse effects, the study found that treatment with ipilimumab and nivolumab can produce lasting responses in the treatment of OC.
5.1.3 Combined therapies
Hinchcliff et al. (2024) conducted a phase II randomized clinical trial comparing durvalumab (PD-L1 inhibitory monoclonal antibody) and tremelimumab (anti-CTLA-4 antibody) administered either as a combination therapy or sequentially in patients with platinum-resistant OC. Among the patients, 38 received sequential therapy (tremelimumab followed by durvalumab), while 23 received combination therapy (tremelimumab and durvalumab together, followed by durvalumab alone). There was no significant difference in PFS between the combination therapy group (1.84 months) and the sequential therapy group (1.87 months) (p = 0.402). Partial responses were observed in two patients (8.7%) and stable disease in 1 patient (4.4%), with all responses occurring in the combination therapy group.
Landry et al. (2023) reported promising results from a phase Ib study investigating the combination of durvalumab with eribulin, a microtubule inhibitor with established benefits in metastatic breast cancer (MBC). The study included four patients with recurrent OC and five patients with HER2-negative MBC, all of whom received escalating doses of eribulin along with durvalumab. The results indicated an ORR of 55%, with four patients experiencing stable disease, and a PFS of 6.2 months.
On the other hand, Konstantinopoulos et al. (2019) demonstrated that the combination of niraparib, a PARP inhibitor (PARPi), with pembrolizumab (anti-PD-1 antibody) showed promising activity in the treatment of platinum-resistant recurrent OC patients. This combination resulted in reduced tumor size and observed disease stabilization. Furthermore, the study indicated that the combination enhanced treatment efficacy, achieving an ORR of 19%, compared to monotherapy with each agent. No new signs of toxicity were reported in this study. Hence, those studies suggest that the combination between ICIs with other drug classes may offer a viable alternative for improved treatment outcomes.
5.2 Antibody therapies using ADCs
5.2.1 JNJ-78306358
It is well established that human leukocyte antigen G (HLA-G) is minimally expressed in healthy cells but highly expressed in various types of human cancer cells (Lin A. and Yan, 2018), including OC. HLA-G functions as an immune checkpoint and interacts with inhibitory receptors (Geva et al., 2024).
In this context, the phase I study by Geva et al. (2024) found that JNJ-78306358, an ADC that binds simultaneously to the α3 domain of HLA-G isoforms on tumor cells and the CD3 receptor complex on T cells, facilitated the formation of immune synapses and the killing of tumor cells by CTLs in renal cell carcinoma, OC, and colorectal cancer in 39 patients. Conversely, no interaction of this ADC was found with cells that do not express HLA-G, demonstrating its specificity for certain types of tumor cells. In this study, all 39 patients (100%) discontinued treatment. The most frequent reasons for discontinuation were disease progression (82.1%) and death (5.1%), with none attributed to the ADC JNJ-78306358.
5.2.2 Mirvetuximab
Among the highly important and promising ADCs for OC treatment, mirvetuximab was approved by FDA in 2022, based on the results from the SORAYA study (Matulonis et al., 2023). This ADC consists of an IgG1 monoclonal antibody targeting the folate receptor alpha (FRα) conjugated to the cytotoxic maytansinoid DM4, which has demonstrated significant clinical activity in patients with FRα-positive OC (González-Ochoa et al., 2023).
Richardson et al. (2024) presented results from a phase Ib study combining mirvetuximab soravtansine with carboplatin and bevacizumab in patients with platinum-sensitive OC. In this study, 41 patients were enrolled, of whom 34 exhibited an anti-tumor response, resulting in an ORR of 83%. Most adverse effects were graded as two or lower, indicating an acceptable safety profile.
Another study involving mirvetuximab was conducted by Moore et al. (2023), who reported results from a global, phase III, confirmatory, open-label, randomized, and controlled trial for the treatment of platinum-resistant FRα-positive HGSOC. Among the patients, 227 were assigned to the mirvetuximab group and 226 to the chemotherapy group (paclitaxel, pegylated liposomal doxorubicin, or topotecan). The results showed a median PFS of 5.62 months and an ORR of 42.3% in the mirvetuximab group. During treatment, fewer grade 3 or higher adverse events occurred with mirvetuximab (41.7%) compared to chemotherapy (54.1%), as well as fewer serious adverse events of any grade (23.9% vs. 32.9%) and events leading to discontinuation (9.2% vs. 15.9%), demonstrating greater safety with the ADC treatment.
5.2.3 Oregovomab
The ADC oregovomab is a murine monoclonal antibody that binds to cancer antigen-125 (CA-125) in blood and local tissues (Battaglia et al., 2020). It is administered to induce targeted therapeutic immunity against cancer. The oregovomab-CA125 complex has enhanced efficacy in antigen capture and cross-presentation, which activates cellular immune response (Brewer et al., 2020).
In this context, Brewer et al. (2020) conducted a phase II, international, randomized, multicenter study to evaluate the results of chemoimmunotherapy in OC using carboplatin-paclitaxel and indirect immunization with oregovomab. The study involved 94 patients who were randomly assigned to receive either carboplatin-paclitaxel alone or carboplatin-paclitaxel with oregovomab addition. Results showed that all patients achieved cytoreduction to less than 1 cm of residual disease or no macroscopic residual disease. Furthermore, the median PFS was 41.8 months in patients receiving additional oregovomab compared to 12.2 months in the control group, demonstrating a significant difference between the two groups (p = 0.0027).
Additionally, a multicenter phase II study by Park et al. (2024) examined the efficacy of non-platinum-based chemotherapy with the use of oregovomab in patients with recurrent OC. This study demonstrated promising efficacy, achieving a PFS of 11 weeks and a median overall survival of 70.4 weeks.
5.2.4 Catumaxomab (Removab)
Catumaxomab is a trifunctional bispecific ADC and targets epithelial cell adhesion molecule (EpCAM) and CD3 T-cell antigen (Ruf et al., 2021). Its anti-tumor effect results from a complex immune reaction at the tumor site involving T cell-mediated lysis, which includes T cell-mediated destruction of tumor cells, antibody-dependent cellular cytotoxicity, and phagocytosis (Knödler et al., 2018).
Studies with this ADC have demonstrated its success as an immunotherapy (Fossati et al., 2015), leading to its approval by the European Medicines Agency (EMA) in 2009 for the intraperitoneal treatment of malignant ascites. However, the approval of this ADC was withdrawn in 2017 due to commercial reasons (Ruf et al., 2021).
5.2.5 Abagovomab
The murine anti-idiotypic monoclonal antibody abagovomab was developed to functionally mimic the three-dimensional structure of CA-125 and induce a specific immune response directed against the original antigen (Battaglia et al., 2017). In this context, a phase III placebo-controlled study known as MIMOSA was conducted, but it showed that the survival rate of patients with OC was not increased by abagovomab (Battaglia et al., 2017). However, a study by Battaglia et al. (2017) aimed to demonstrate that a healthy immune system conditions the response to this ADC. In their research, 80 patients received abagovomab, and 31 patients received placebo. Patients treated with abagovomab who had a percentage of CD8+ T cells producing IFN-γ above the cutoff point showed better recurrence-free survival (p = 0.042) than those with a percentage of CD8+ T cells producing IFN-γ below the cutoff point. Additionally, this study demonstrated that the recurrence-free survival of patients treated with abagovomab with both a percentage of CD8+ T cells producing IFN-γ and absolute cell counts below the respective cutoff points was identical to that of patients in the placebo group. In this regard, it is concluded that further studies are needed to clarify the effects of abagovomab in OC patients.
5.2.6 Daclizumab (Zenapax)
Daclizumab (Zenapax) is a humanized IgG1 monoclonal antibody specific to IL-2 receptor-α subunit (CD25) (Tse et al., 2014). It irreversibly blocks CD25, thereby preventing signaling through the high-affinity IL-2R while increasing the bioavailability of IL-2 to bind to the low-affinity receptor (Ranganath et al., 2020). As a result, ADC induces various immunological changes, including inhibition of T cell activation, reduction in the frequency and survival of regulatory T cells, and expansion of CD56bright NK cells (Ranganath et al., 2020).
Within this scenario, an interventional phase I clinical trial was conducted with patients with recurrent ovarian, fallopian tube, or primary peritoneal cancer using this ADC. However, the study was terminated in 2018, and the results were not published. Additionally, this drug was suspended by EMA in 2018 due to 12 reported worldwide cases of severe brain inflammation, three of which were fatal (European Medicines Agency, 2018). Table 2 highlights some studies that evaluate ICIs and ADCs technologies in OC treatment.
5.3 T- and NK-cell engaging bispecific antibodies (BsAbs)
Bispecific antibodies (BsAbs) are engineered molecules designed to bind simultaneously to two distinct epitopes or antigens. This dual targeting mechanism allows them to interact with tumor antigens on cancer cells while activating receptors on immune cells, offering a novel approach to immunotherapy (Wang Q. et al., 2019). Recent studies have focused on the roles of T and NK cells in this context, as BsAbs can effectively bring these immune cells into proximity with tumor cells (Wu Z. and Cheung, 2018). By simultaneously binding to tumor antigens on cancer cells and activating receptors such as CD3 on T cells or CD16 on NK cells, BsAbs enhance the capacity of these immune cells to recognize and eliminate malignant cells. This strategy positions engaging BsAbs as a promising approach for cancer immunotherapy (Tapia-Galisteo et al., 2023).
In the context of hematological tumors, numerous clinical trials have demonstrated favorable outcomes with T cell-engaging bispecific antibodies (BsAbs). Notable examples include epcoritamab (Thieblemont et al., 2022), odronextamab (Bannerji et al., 2022), mosunetuzumab (Budde et al., 2022), and glofitamab (Hutchings et al., 2021). These CD3xCD20 T cell-engaging BsAbs bind to T cells via CD3 receptors, effectively directing them to eliminate malignant CD20+ B cells in patients with heavily pretreated B-cell non-Hodgkin lymphoma (van de Donk and Zweegman, 2023). Additionally, Reusing et al. (2021) reported that CD16xCD33 NK cell-engaging BsAbs activated Killer immunoglobulin-like receptor (KIR) signaling, thereby enhancing NK cell-mediated lysis of acute myeloid leukemia (AML) blasts.
Regarding solid tumors, particularly OC, Crawford and colleagues (2019) reported on the BsAb REGN4018, which targets both MUC16, a highly expressed marker in OC cells, and CD3, a receptor on T cells. Overall, their findings indicated that REGN4018 exhibited robust antitumor activity and favorable tolerability, warranting its clinical evaluation in patients with MUC16-expressing advanced OC (Crawford et al., 2019). Oladapo et al. (2021) similarly investigated T cell-engaging BsAbs targeting MUC16. Their findings indicate that these antibodies demonstrate efficacy against OC, both as a monotherapy and in combination with other agents such as PD-1 and VEGF inhibitors (Oladapo et al., 2021). In the other hand, Lee E. and colleagues (2021) examined a BsAb targeting LYPD1, an antigen associated with high-grade serous OC, and their data suggested its compelling efficacy and safety profiles, supporting its potential use as a treatment for high-grade serous OC (Lee E. et al., 2021).
Furthermore, Avanzino and colleagues (2022) studied TNB-928B, a T-cell engaging BsAb that binds to FRα to selectively target FRα overexpressing tumor cells. It was shown that TNB-928B induced preferential effector T-cell activation, proliferation, and selective cytotoxic activity on high FRα expressing OC cells, and also promoted T-cell infiltration and antitumor activity in OC mouse models (Avanzino et al., 2022). Additionally, Vallera et al. (2020) evaluated cam1615B7H3, a tri-specific killer engager that has a camelid CD16 antibody fragment, a wild-type IL-15 moiety, and an anti-B7-H3 single-chain variable fragment, in various types of solid tumors. Their findings suggest that cam1615B7H3 improves NK cell function, expansion, targeted cytotoxicity against various types of B7-H3-positive human cancer cell lines, and delivers an anti-cancer effect in vivo in a solid tumor setting, including in OC (Vallera et al., 2020).
Given the studies conducted, further research is necessary to ensure the safety of these ADCs in OC treatment.
6 Discussion
Overall, immunotherapy for OC faces significant challenges, yet the field holds substantial potential for advancement. Ongoing efforts aim to overcome immune suppression and improve the efficacy of OC immunotherapy. These strategies include combining immunotherapy with other drugs, utilizing targeted and precision-guided particles, developing innovative antigen vaccine delivery systems, and implementing prolonged low-dose immunotherapy regimens. Consequently, recent progress in both active and passive immunotherapy approaches has introduced new perspectives and insights, thereby enhancing the effectiveness of immune-based treatments for OC. Indeed, to handle the adverse effects of common therapies for cancer, immunotherapy strategies emerged as a cancer-specific alternative capable of targeting the tumor and causing minimal impact on normal tissues (Aly, 2012; Zhu and Yu, 2022). They are significant considering the usual therapeutic approaches such as surgery, chemotherapy, and radiotherapy which besides the adverse effects show a lack of specificity for tumors (Kaczmarek et al., 2023). Therapeutic cancer vaccination is a strategy of immunotherapy developed to elicit or boost antitumor adaptive immune responses to detect and eliminate them (Luo et al., 2024; Chambers, 2011). Moreover, CAR-T cells are genetically engineered to recognize and attack tumor-specific antigens (June et al., 2018), bypassing the need of MHC molecules presentation, and behaving as active drugs against tumors (Maus and June, 2016). In turn, exosomes are a category of extracellular vesicles with a lipid bilayer, measuring approximately 30–150 nm, found in various body fluids such as blood, urine, saliva, and cerebrospinal fluid (He et al., 2018; Gong et al., 2023). In addition to their ambiguous role, exosomes may serve as a potential tool for OC therapy (Shimizu et al., 2024). Also of clinical relevance, therapeutic monoclonal antibodies have been successfully developed for the treatment of various cancer types (Hafeez et al., 2020). Numerous clinical trials are still underway with promising prospects for the treatment of OC, including ADCs such as JNJ-78306358, ivonescimab, ipilimumab, durvalumab, oregovomab, catumaxomab, abagovomab, daclizumab and mirvetuximab, which was approved by the FDA in 2022 but remains under study for application in OC treatment (Dilawari et al., 2023). Yet, ICIs represent a promising class of drugs in immunotherapy against OC, targeting PD-1/PD-L1 and CTLA-4. They have already demonstrated broad bioactivity and stable response in the treatment of various types of tumors (Naimi et al., 2022; Tang S. et al., 2022b), including OC (Disis et al., 2019). Therefore, OC immunotherapy involves the induction of an anti-tumor immune response and the development of immunological memory. This process not only can eradicate malignant cells within the primary tumor site, thereby averting recurrence, but also hampers the metastatic spread to distant anatomical locations (Cha et al., 2020).
Author contributions
TM: Writing–original draft, Writing–review and editing. JC: Writing–original draft, Writing–review and editing. BS: Writing–original draft, Writing–review and editing. SS: Writing–original draft, Writing–review and editing. JS: Writing–original draft, Writing–review and editing. MG: Writing–original draft, Writing–review and editing. GS: Writing–original draft, Writing–review and editing. LR: Project administration, Supervision, Writing–review and editing.
Funding
The author(s) declare that no financial support was received for the research, authorship, and/or publication of this article.
Acknowledgments
We would like to thank the funding agencies FAPES and CAPES for the PhD and master’s scholarships, and the UFES and CNPQ for the scientific initiation scholarships.
Conflict of interest
The authors declare that the research was conducted in the absence of any commercial or financial relationships that could be construed as a potential conflict of interest.
Publisher’s note
All claims expressed in this article are solely those of the authors and do not necessarily represent those of their affiliated organizations, or those of the publisher, the editors and the reviewers. Any product that may be evaluated in this article, or claim that may be made by its manufacturer, is not guaranteed or endorsed by the publisher.
References
Abd-Aziz, N., and Poh, C. L. (2022). Development of peptide-based vaccines for cancer. J. Oncol. 2022, 9749363. doi:10.1155/2022/9749363
Acharya, G., Mani, C., Sah, N., Saamarthy, K., Young, R., Reedy, M. B., et al. (2024). CHK1 inhibitor induced PARylation by targeting PARG causes excessive replication and metabolic stress and overcomes chemoresistance in ovarian cancer. Cell Death Discov. 10 (1), 278. doi:10.1038/s41420-024-02040-0
Aggarwal, S., He, T., Fitzhugh, W., Rosenthal, K., Feild, B., Heidbrink, J., et al. (2009). Immune modulator CD70 as a potential cisplatin resistance predictive marker in ovarian cancer. Gynecol. Oncol. 115 (3), 430–437. doi:10.1016/j.ygyno.2009.08.031
Albelda, S. M. (2024). CAR T cell therapy for patients with solid tumours: key lessons to learn and unlearn. Nat. Rev. Clin. Oncol. 21 (1), 47–66. doi:10.1038/s41571-023-00832-4
Alipoor, S. D., Mortaz, E., Varahram, M., Movassaghi, M., Kraneveld, A. D., Garssen, J., et al. (2018). The potential biomarkers and immunological effects of tumor-derived exosomes in lung cancer. Front. Immunol. 9, 819. doi:10.3389/fimmu.2018.00819
Aly, H. A. (2012). Cancer therapy and vaccination. J. Immunol. methods 382 (1-2), 1–23. doi:10.1016/j.jim.2012.05.014
American Cancer Society (2020). Cancer vaccines and their side effects. Available at: https://www.cancer.org/cancer/managing-cancer/treatment-types/immunotherapy/cancer-vaccines.html (Accessed July 29, 2024).
American Cancer Society (2024). Key statistics for ovarian cancer. Atlanta: ACS Publications. Available at: https://www.cancer.org/cancer/types/ovarian-cancer/about/key-statistics.html (Accessed July 30, 2024).
Andersson, E., Villabona, L., Bergfeldt, K., Carlson, J. W., Ferrone, S., Kiessling, R., et al. (2012). Correlation of HLA-A02* genotype and HLA class I antigen down-regulation with the prognosis of epithelial ovarian cancer. Cancer Immunol. Immunother. CII. 61 (8), 1243–1253. doi:10.1007/s00262-012-1201-0
Apte, R. S., Chen, D. S., and Ferrara, N. (2019). VEGF in signaling and disease: beyond discovery and development. Cell 176 (6), 1248–1264. doi:10.1016/j.cell.2019.01.021
Asare-Werehene, M., Nakka, K., Reunov, A., Chiu, C. T., Lee, W. T., Abedini, M. R., et al. (2020). The exosome-mediated autocrine and paracrine actions of plasma gelsolin in ovarian cancer chemoresistance. Oncogene 39 (7), 1600–1616. doi:10.1038/s41388-019-1087-9
Avanzino, B. C., Prabhakar, K., Dalvi, P., Hartstein, S., Kehm, H., Balasubramani, A., et al. (2022). A T-cell engaging bispecific antibody with a tumor-selective bivalent folate receptor alpha binding arm for the treatment of ovarian cancer. Oncoimmunology 11 (1), 2113697. doi:10.1080/2162402X.2022.2113697
Battaglia, A., Buzzonetti, A., Fossati, M., Scambia, G., Fattorossi, A., Madiyalakan, M. R., et al. (2020). ranslational immune correlates of indirect antibody immunization in a randomized phase II study using scheduled combination therapy with carboplatin/paclitaxel plus oregovomab in ovarian cancer patients. Cancer immunology, immunotherapy: CII. 69 (3), 383–397. doi:10.1007/s00262-019-02456-z
Battaglia, A., Fossati, M., Buzzonetti, A., Scambia, G., and Fattorossi, A. (2017). A robust immune system conditions the response to abagovomab (anti-idiotypic monoclonal antibody mimicking the CA125 protein) vaccination in ovarian cancer patients. Immunology letters. 191, 35–39. doi:10.1016/j.imlet.2017.09.006
Baker, D. J., Arany, Z., Baur, J. A., Epstein, J. A., and June, C. H. (2023). CAR T therapy beyond cancer: the evolution of a living drug. Nature 619 (7971), 707–715. doi:10.1038/s41586-023-06243-w
Bannerji, R., Arnason, J. E., Advani, R. H., Brown, J. R., Allan, J. N., Ansell, S. M., et al. (2022). Odronextamab, a human CD20×CD3 bispecific antibody in patients with CD20-positive B-cell malignancies (ELM-1): results from the relapsed or refractory non-Hodgkin lymphoma cohort in a single-arm, multicentre, phase 1 trial. Lancet Haematol. 9 (5), 327–339. doi:10.1016/s2352-3026(22)00072-2
Barbier, A. J., Jiang, A. Y., Zhang, P., Wooster, R., and Anderson, D. G. (2022). The clinical progress of mRNA vaccines and immunotherapies. Nat. Biotechnol. 40 (6), 840–854. doi:10.1038/s41587-022-01294-2
Bhattacharya, B., Nag, S., Mukherjee, S., Kulkarni, M., Chandane, P., Mandal, D., et al. (2024). Role of exosomes in epithelial-mesenchymal transition. ACS Appl. bio Mater. 7 (1), 44–58. doi:10.1021/acsabm.3c00941
Bhavsar, D., Raguraman, R., Kim, D., Ren, X., Munshi, A., Moore, K., et al. (2024). Exosomes in diagnostic and therapeutic applications of ovarian cancer. J. ovarian Res. 17 (1), 113. doi:10.1186/s13048-024-01417-0
Bhojnagarwala, P. S., Perales-Puchalt, A., Cooch, N., Sardesai, N. Y., and Weiner, D. B. (2021). A synDNA vaccine delivering neoAg collections controls heterogenous, multifocal murine lung and ovarian tumors via robust T cell generation. Mol. Ther. oncolytics 21, 278–287. doi:10.1016/j.omto.2021.04.005
Block, M. S., Dietz, A. B., Gustafson, M. P., Kalli, K. R., Erskine, C. L., Youssef, B., et al. (2020). Th17-inducing autologous dendritic cell vaccination promotes antigen-specific cellular and humoral immunity in ovarian cancer patients. Nat. Commun. 11 (1), 5173. doi:10.1038/s41467-020-18962-z
Brewer, M., Angioli, R., Scambia, G., Lorusso, D., Terranova, C., Panici, P. B., et al. (2020). Front-line chemo-immunotherapy with carboplatin-paclitaxel using oregovomab indirect immunization in advanced ovarian cancer: a randomized phase II study. Gynecol. Oncol. 156 (3), 523–529. doi:10.1016/j.ygyno.2019.12.024
Brown, T. A., Byrd, K., Vreeland, T. J., Clifton, G. T., Jackson, D. O., Hale, D. F., et al. (2019). Final analysis of a phase I/IIa trial of the folate-binding protein-derived E39 peptide vaccine to prevent recurrence in ovarian and endometrial cancer patients. Cancer Med. 8 (10), 4678–4687. doi:10.1002/cam4.2378
Brudno, J. N., and Kochenderfer, J. N. (2024). Current understanding and management of CAR T cell-associated toxicities. Nat. Rev. Clin. Oncol. 21, 501–521. doi:10.1038/s41571-024-00903-0
Budde, L. E., Assouline, S., Sehn, L. H., Schuster, S. J., Yoon, S.-S., Yoon, D. H., et al. (2022). Single-agent mosunetuzumab shows durable complete responses in patients with relapsed or refractory B-cell lymphomas: phase I dose-escalation study. J. Clin. Oncol. 40 (5), 481–491. doi:10.1200/JCO.21.00931
Bund, V., Azais, H., Bibi-Triki, S., Lecointre, L., Betrian, S. B., Angeles, M. A., et al. (2022). Basics of immunotherapy for epithelial ovarian cancer. J. Gynecol. Obstetrics Hum. Reproduction 51 (2), 102283. doi:10.1016/j.jogoh.2021.102283
Cappell, K. M., and Kochenderfer, J. N. (2023). Long-term outcomes following CAR T cell therapy: what we know so far. Nat. Rev. Clin. Oncol. 20 (6), 359–371. doi:10.1038/s41571-023-00754-1
Carmi, Y. K., Agbarya, A., Khamaisi, H., Farah, R., Shechtman, Y., Korobochka, R., et al. (2024). Ovarian cancer ascites confers platinum chemoresistance to ovarian cancer cells. Transl. Oncol. 44 (101939), 101939. doi:10.1016/j.tranon.2024.101939
Cha, J. H., Chan, L. C., Song, M. S., and Hung, M. C. (2020). New approaches on cancer immunotherapy. Cold Spring Harb. Perspect. Med. 10 (8), a036863. doi:10.1101/cshperspect.a036863
Chambers, C. V. (2011). Cancer vaccines. Prim. Care Clin. Office Pract. 38 (4), 703–715. doi:10.1016/j.pop.2011.07.008
Chehelgerdi, M., Behdarvand Dehkordi, F., Chehelgerdi, M., Kabiri, H., Salehian-Dehkordi, H., Abdolvand, M., et al. (2023). Exploring the promising potential of induced pluripotent stem cells in cancer research and therapy. Mol. Cancer 22 (1), 189. doi:10.1186/s12943-023-01873-0
Chekmasova, A. A., Rao, T. D., Nikhamin, Y., Park, K. J., Levine, D. A., Spriggs, D. R., et al. (2010). Successful eradication of established peritoneal ovarian tumors in SCID-Beige mice following adoptive transfer of T cells genetically targeted to the MUC16 antigen. Clin. cancer Res. official J. Am. Assoc. Cancer Res. 16 (14), 3594–3606. doi:10.1158/1078-0432.ccr-10-0192
Chiang, C. L. L., Kandalaft, L. E., and Coukos, G. (2011). Adjuvants for enhancing the immunogenicity of whole tumor cell vaccines. Int. Rev. Immunol. 30 (2-3), 150–182. doi:10.3109/08830185.2011.572210
Coelho, R., Marcos-Silva, L., Ricardo, S., Ponte, F., Costa, A., Lopes, J. M., et al. (2018). Peritoneal dissemination of ovarian cancer: role of MUC16-mesothelin interaction and implications for treatment. Expert Rev. anticancer Ther. 18 (2), 177–186. doi:10.1080/14737140.2018.1418326
Crawford, A., Haber, L., Kelly, M. P., Vazzana, K., Canova, L., Ram, P., et al. (2019). A Mucin 16 bispecific T cell-engaging antibody for the treatment of ovarian cancer. Sci. Transl. Med. 11 (497), eaau7534. doi:10.1126/scitranslmed.aau7534
Dagher, O. K., and Posey Jr, A. D. (2023). Forks in the road for CAR T and CAR NK cell cancer therapies. Nat. Immunol. 24 (12), 1994–2007. doi:10.1038/s41590-023-01659-y
Daud, A. I., Wolchok, J. D., Robert, C., Hwu, W., Weber, J. S., Ribas, A., et al. (2016). Programmed death-ligand 1 expression and response to the anti-programmed death 1 antibody pembrolizumab in melanoma. J. Clin. Oncol. 34 (34), 4102–4109. doi:10.1200/JCO.2016.67.2477
Dean, A. Q., Luo, S., Twomey, J. D., and Zhang, B. (2021). Targeting cancer with antibody-drug conjugates: Promises and challenges. mAbs 13 (1), 1951427. doi:10.1080/19420862.2021.1951427
Del Bufalo, F., De Angelis, B., Caruana, I., Del Baldo, G., De Ioris, M. A., Serra, A., et al. (2023). GD2-CART01 for relapsed or refractory high-risk neuroblastoma. N. Engl. J. Med. 388 (14), 1284–1295. doi:10.1056/NEJMoa2210859
Dhillon, S. (2024). Ivonescimab: first approval. Drugs 84, 1135–1142. doi:10.1007/s40265-024-02073-w
Dilawari, A., Shah, M., Ison, G., Gittleman, H., Fiero, M. H., Shah, A., et al. (2023). FDA approval summary: mirvetuximab soravtansine-gynx for FRα-positive, platinum-resistant ovarian cancer. Clin. Cancer Res. 29 (19), 3835–3840. doi:10.1158/1078-0432.CCR-23-0991
Disis, M. L., Taylor, M. H., Kelly, K., Beck, J. T., Gordon, M., Moore, K. M., et al. (2019). Efficacy and safety of avelumab for patients with recurrent or refractory ovarian cancer: phase 1b results from the JAVELIN Solid Tumor trial. JAMA Oncol. 5 (3), 393–401. doi:10.1001/jamaoncol.2018.6258
Doubeni, C. A., Doubeni, A. R., and Myers, A. E. (2016). Diagnosis and management of ovarian cancer. Am. Fam. physician. 93 (11), 937–944.
Duperret, E. K., Perales-Puchalt, A., Stoltz, R., G H, H., Mandloi, N., Barlow, J., et al. (2019). A synthetic DNA, multi-neoantigen vaccine drives predominately MHC class I CD8+ T-cell responses, impacting tumor challenge. Cancer Immunol. Res. 7 (2), 174–182. doi:10.1158/2326-6066.CIR-18-0283
European Medicines Agency (2018). Multiple sclerosis medicine Zinbryta suspended in the EU. Available at: https://www.ema.europa.eu/system/files/documents/referral/wc500245166_en.pdf (Accessed July 27, 2024).
Fan, T., Zhang, M., Yang, J., Zhu, Z., Cao, W., and Dong, C. (2023). Therapeutic cancer vaccines: advancements, challenges, and prospects. Signal Transduct. Target. Ther. 8 (1), 450. doi:10.1038/s41392-023-01674-3
Flugel, C. L., Majzner, R. G., Krenciute, G., Dotti, G., Riddell, S. R., Wagner, D. L., et al. (2023). Overcoming on-target, off-tumour toxicity of CAR T cell therapy for solid tumours. Nat. Rev. Clin. Oncol. 20 (1), 49–62. doi:10.1038/s41571-022-00704-3
Food and Drug Administration (2024). Package insert and medication guide - Yescarta. Available at: https://www.fda.gov/media/108377/download (Accessed August 1, 2024).
Fossati, M., Buzzonetti, A., Monego, G., Catzola, V., Scambia, G., Fattorossi, A., et al. (2015). Immunological changes in the ascites of cancer patients after intraperitoneal administration of the bispecific antibody catumaxomab (anti-EpCAM×anti-CD3). Gynecol. Oncol. 138 (2), 343–351. doi:10.1016/j.ygyno.2015.06.003
Frentzas, S., Mislang, A. R. A., Lemech, C., Nagrial, A., Underhill, C., Wang, W., et al. (2024). Phase 1a dose escalation study of ivonescimab (AK112/SMT112), an anti-PD-1/VEGF-A bispecific antibody, in patients with advanced solid tumors. J. Immunother. Cancer 12 (4), e008037. doi:10.1136/jitc-2023-008037
Friedman, C. F., Manning-Geist, B. L., Zhou, Q., Soumerai, T., Holland, A., Paula, A. D. C., et al. (2024). Nivolumab for mismatch-repair-deficient or hypermutated gynecologic cancers: a phase 2 trial with biomarker analyses. Nat. Med. 30 (5), 1330–1338. doi:10.1038/s41591-024-02942-7
Geva, R., Vieito, M., Ramon, J., Perets, R., Pedregal, M., Corral, E., et al. (2024). Safety and clinical activity of JNJ-78306358, a human leukocyte antigen-G (HLA-G) x CD3 bispecific antibody, for the treatment of advanced stage solid tumors. Cancer Immunol. Immunother. 73 (10), 205. doi:10.1007/s00262-024-03790-7
Ghoneum, A., Almousa, S., Warren, B., Abdulfattah, A. Y., Shu, J., Abouelfadl, H., et al. (2021). Exploring the clinical value of tumor microenvironment in platinum-resistant ovarian cancer. Seminars cancer Biol. 77, 83–98. doi:10.1016/j.semcancer.2020.12.024
Gong, X., Chi, H., Strohmer, D. F., Teichmann, A. T., Xia, Z., and Wang, Q. (2023). Exosomes: a potential tool for immunotherapy of ovarian cancer. Front. Immunol. 13, 1089410. doi:10.3389/fimmu.2022.1089410
Gonzalez-Ochoa, E., Veneziani, A. C., and Oza, A. M. (2023). Mirvetuximab soravtansine in platinum-resistant ovarian cancer. Clin. Med. Insights Oncol. 17, 11795549231187264. doi:10.1177/11795549231187264
Hafeez, U., Parakh, S., Gan, H. K., and Scott, A. M. (2020). Antibody-drug conjugates for cancer therapy. Molecules 25 (20), 4764. doi:10.3390/molecules25204764
He, C., Zheng, S., Luo, Y., and Wang, B. (2018). Exosome theranostics: biology and translational medicine. Theranostics 8 (1), 237–255. doi:10.7150/thno.21945
Hilliard, T. S. (2018). The impact of mesothelin in the ovarian cancer tumor microenvironment. Cancers 10 (9), 277. doi:10.3390/cancers10090277
Hinchcliff, E. M., Knisely, A., Adjei, N., Fellman, B., Yuan, Y., Patel, A., et al. (2024). Randomized phase 2 trial of tremelimumab and durvalumab in combination versus sequentially in recurrent platinum-resistant ovarian cancer. Cancer. 130 (7), 1061–1071. doi:10.1002/cncr.35126
Hutchings, M., Morschhauser, F., Iacoboni, G., Carlo-Stella, C., Offner, F. C., Sureda, A., et al. (2021). Glofitamab, a novel, bivalent CD20-targeting T-cell–engaging bispecific antibody, induces durable complete remissions in relapsed or refractory B-cell lymphoma: a phase I trial. J. Clin. Oncol. 39 (18), 1959–1970. doi:10.1200/jco.20.03175
Jaeger, D., Sahin, U., and Tureci, O. (2014). A first-in-human dose escalation and dose-finding phase I/II trial of IMAB027 in patients with recurrent advanced ovarian cancer (GM-IMAB-002-01). J. Clin. Oncol. 32 (15), TPS5623. doi:10.1200/jco.2014.32.15_suppl.tps5623
Janes, M. E., Gottlieb, A. P., Park, K. S., Zhao, Z., and Mitragotri, S. (2024). Cancer vaccines in the clinic. Bioeng. & Transl. Med. 9 (1), e10588. doi:10.1002/btm2.10588
June, C. H., O’Connor, R. S., Kawalekar, O. U., Ghassemi, S., and Milone, M. C. (2018). CAR T cell immunotherapy for human cancer. Science 359 (6382), 1361–1365. doi:10.1126/science.aar6711
Kaczmarek, M., Poznańska, J., Fechner, F., Michalska, N., Paszkowska, S., Napierała, A., et al. (2023). Cancer vaccine therapeutics: limitations and effectiveness—a literature review. Cells 12 (17), 2159. doi:10.3390/cells12172159
Kalli, K. R., Block, M. S., Kasi, P. M., Erskine, C. L., Hobday, T. J., Dietz, A., et al. (2018). Folate receptor alpha peptide vaccine generates immunity in breast and ovarian cancer patients. Clin. Cancer Res. 24 (13), 3014–3025. doi:10.1158/1078-0432.CCR-17-2499
Kaushik, S., and Cuervo, A. M. (2015). Proteostasis and aging. Nat. Med. 21 (12), 1406–1415. doi:10.1038/nm.4001
Kim, J., Zhu, Y., Chen, S., Wang, D., Zhang, S., Xia, J., et al. (2023). Anti-glioma effect of ginseng-derived exosomes-like nanoparticles by active blood-brain-barrier penetration and tumor microenvironment modulation. J. nanobiotechnology 21 (1), 253. doi:10.1186/s12951-023-02006-x
Knisely, A., Hinchcliff, E., Fellman, B., Mosley, A., Lito, K., Hull, S., et al. (2024). Phase 1b study of intraperitoneal ipilimumab and nivolumab in patients with recurrent gynecologic malignancies with peritoneal carcinomatosis. Med 5 (4), 311–320.e3. doi:10.1016/j.medj.2024.02.003
Knödler, M., Körfer, J., Kunzmann, V., Trojan, J., Daum, S., Schenk, M., et al. (2018). Randomised phase II trial to investigate catumaxomab (anti-EpCAM × anti-CD3) for treatment of peritoneal carcinomatosis in patients with gastric cancer. Br. J. Cancer 119 (3), 296–302. doi:10.1038/s41416-018-0150-6
Konstantinopoulos, P. A., Waggoner, S., Vidal, G. A., Mita, M., Moroney, J. W., Holloway, R., et al. (2019). Single-Arm phases 1 and 2 trial of niraparib in combination with pembrolizumab in patients with recurrent platinum-resistant ovarian carcinoma. JAMA Oncol. 5 (8), 1141–1149. doi:10.1001/jamaoncol.2019.1048
Kugeratski, F. G., and Raghu, K. (2021). Exosomes as mediators of immune regulation and immunotherapy in cancer. FEBS J. 288 (1), 10–35. doi:10.1111/febs.15558
Labanieh, L., and Mackall, C. L. (2023). CAR immune cells: design principles, resistance and the next generation. Nature 614 (7949), 635–648. doi:10.1038/s41586-023-05707-3
Landry, C. A., Blanter, J., Ru, M., Fasano, J., Klein, P., Shao, T., et al. (2023). Results of a phase Ib study investigating durvalumab in combination with eribulin in patients with HER2-negative metastatic breast cancer and recurrent ovarian cancer. 102, 9, 16. doi:10.1159/000533420
Laureano, R. S., Sprooten, J., Vanmeerbeerk, I., Borras, D. M., Govaerts, J., Naulaerts, S., et al. (2022). Trial watch: dendritic cell (DC)-based immunotherapy for cancer. Oncoimmunology 11 (1), 2096363. doi:10.1080/2162402X.2022.2096363
Lee, D., Dunn, Z. S., Guo, W., Rosenthal, C. J., Penn, N. E., Yu, Y., et al. (2023). Unlocking the potential of allogeneic Vδ2 T cells for ovarian cancer therapy through CD16 biomarker selection and CAR/IL-15 engineering. Nat. Commun. 14 (1), 6942. doi:10.1038/s41467-023-42619-2
Lee, E., Szvetecz, S., Polli, R., Grauel, A., Chen, J., Judge, J., et al. (2021). PAX8 lineage-driven T cell engaging antibody for the treatment of high-grade serous ovarian cancer. Sci. Rep. 11 (1), 14841. doi:10.1038/s41598-021-93992-1
Li, K., Mandai, M., Hamanishi, J., Matsumura, N., Suzuki, A., Yagi, H., et al. (2009). Clinical significance of the NKG2D ligands, MICA/B and ULBP2 in ovarian cancer: high expression of ULBP2 is an indicator of poor prognosis. Cancer Immunol. Immunother. 58 (5), 641–652. doi:10.1007/s00262-008-0585-3
Li, M., Xu, H., Qi, Y., Pan, Z., Li, B., Gao, Z., et al. (2022). Tumor-derived exosomes deliver the tumor suppressor miR-3591-3p to induce M2 macrophage polarization and promote glioma progression. Oncogene 41 (41), 4618–4632. doi:10.1038/s41388-022-02457-w
Li, T., Lin, L., Liu, Q., Gao, W., Chen, L., Sha, C., et al. (2021a). Exosomal transfer of miR-429 confers chemoresistance in epithelial ovarian cancer. Am. J. cancer Res. 11 (5), 2124–2141.
Li, X., Liu, Y., Zheng, S., Zhang, T., Wu, J., Sun, Y., et al. (2021b). Role of exosomes in the immune microenvironment of ovarian cancer. Oncol. Lett. 21 (5), 377. doi:10.3892/ol.2021.12638
Li, X., and Wang, X. (2017). The emerging roles and therapeutic potential of exosomes in epithelial ovarian cancer. Mol. cancer 16 (1), 92. doi:10.1186/s12943-017-0659-y
Liang, Z., Dong, J., Yang, N., Li, S. D., Yang, Z. Y., Huang, R., et al. (2021). Tandem CAR-T cells targeting FOLR1 and MSLN enhance the antitumor effects in ovarian cancer. Int. J. Biol. Sci. 17 (15), 4365–4376. doi:10.7150/ijbs.63181
Lin, A., and Yan, W. H. (2018). Heterogeneity of HLA-G expression in cancers: facing the challenges. Front. Immunol. 9, 2164. doi:10.3389/fimmu.2018.02164
Lin, M. J., Svensson-Arvelund, J., Lubitz, G. S., Marabelle, A., Melero, I., Brown, B. D., et al. (2022). Cancer vaccines: the next immunotherapy frontier. Nat. cancer 3 (8), 911–926. doi:10.1038/s43018-022-00418-6
Liu, D., Liu, L., Li, X., Wang, S., Wu, G., and Che, X. (2024a). Advancements and challenges in peptide-based cancer vaccination: a multidisciplinary perspective. Vaccines 12, 950. doi:10.3390/vaccines12080950
Liu, H., Deng, S., Yao, X., Liu, Y., Qian, L., Wang, Y., et al. (2024b). Ascites exosomal lncRNA PLADE enhances platinum sensitivity by inducing R-loops in ovarian cancer. Oncogene 43 (10), 714–728. doi:10.1038/s41388-024-02940-6
Lu, L., Ma, W., Johnson, C. H., Khan, S. A., Irwin, M. L., and Pusztai, L. (2023). In silico designed mRNA vaccines targeting CA-125 neoantigen in breast and ovarian cancer. Vaccine 41 (12), 2073–2083. doi:10.1016/j.vaccine.2023.02.048
Luo, J., Mo, F., Zhang, Z., Hong, W., Lan, T., Cheng, Y., et al. (2024). Engineered mitochondria exert potent antitumor immunity as a cancer vaccine platform. Cell. & Mol. Immunol., 1–15. doi:10.1038/s41423-024-01203-4
Lv, L., Huang, J., Xi, H., and Zhou, X. (2020). Efficacy and safety of dendritic cell vaccines for patients with glioblastoma: a meta-analysis of randomized controlled trials. Int. Immunopharmacol. 83, 106336. doi:10.1016/j.intimp.2020.106336
Ma, L., Dichwalkar, T., Chang, J. Y., Cossette, B., Garafola, D., Zhang, A. Q., et al. (2019). Enhanced CAR–T cell activity against solid tumors by vaccine boosting through the chimeric receptor. Science 365 (6449), 162–168. doi:10.1126/science.aav8692
Mackensen, A., Haanen, J. B., Koenecke, C., Alsdorf, W., Wagner-Drouet, E., Borchmann, P., et al. (2023). CLDN6-specific CAR-T cells plus amplifying RNA vaccine in relapsed or refractory solid tumors: the phase 1 BNT211-01 trial. Nat. Med. 29 (11), 2844–2853. doi:10.1038/s41591-023-02612-0
Macpherson, A. M., Barry, S. C., Ricciardelli, C., and Oehler, M. K. (2020). Epithelial ovarian cancer and the immune system: biology, interactions, challenges and potential advances for immunotherapy. J. Clin. Med. 9 (9), 2967. doi:10.3390/jcm9092967
Matulonis, U. A., Lorusso, D., Oaknin, A., Pignata, S., Dean, A., Denys, H., et al. (2023). Efficacy and safety of mirvetuximab soravtansine in patients with platinum-resistant ovarian cancer with high folate receptor alpha expression: results from the SORAYA study. J. Clin. Oncol. 41 (13), 2436–2445. doi:10.1200/JCO.22.01900
Maus, M. V., and June, C. H. (2016). Making better chimeric antigen receptors for adoptive T-cell therapy. Clin. cancer Res. 22 (8), 1875–1884. doi:10.1158/1078-0432.CCR-15-1433
Miyamoto, T., Murakami, R., Hamanishi, J., Tanigaki, K., Hosoe, Y., Mise, N., et al. (2022). B7-H3 suppresses antitumor immunity via the CCL2-CCR2-M2 macrophage Axis and contributes to ovarian cancer progression. Cancer Immunol. Res. 10 (1), 56–69. doi:10.1158/2326-6066.cir-21-0407
Moore, K. N., Angelergues, A., Konecny, G. E., García, Y., Banerjee, S., Lorusso, D., et al. (2023). Mirvetuximab soravtansine in FRα-positive, platinum-resistant ovarian cancer. N. Engl. J. Med. 389 (23), 2162–2174. doi:10.1056/NEJMoa2309169
Morrissey, S. M., Zhang, F., Ding, C., Montoya-Durango, D. E., Hu, X., Yang, C., et al. (2021). Tumor-derived exosomes drive immunosuppressive macrophages in a pre-metastatic niche through glycolytic dominant metabolic reprogramming. Cell metab. 33 (10), 2040–2058.e10. doi:10.1016/j.cmet.2021.09.002
Mousaei Ghasroldasht, M., Liakath Ali, F., Park, H. S., Hadizadeh, M., Weng, S. H. S., Huff, A., et al. (2024). A comparative analysis of naïve exosomes and enhanced exosomes with a focus on the treatment potential in ovarian disorders. J. personalized Med. 14 (5), 482. doi:10.3390/jpm14050482
Murad, J. P., Kozlowska, A. K., Lee, H. J., Ramamurthy, M., Chang, W. C., Yazaki, P., et al. (2018). Effective targeting of TAG72+ peritoneal ovarian tumors via regional delivery of CAR-engineered T cells. Front. Immunol. 9, 2268. doi:10.3389/fimmu.2018.02268
Muthukutty, P., and Yoo, S. Y. (2023). Oncolytic virus engineering and utilizations: cancer immunotherapy perspective. Viruses 15 (8), 1645. doi:10.3390/v15081645
Naimi, A., Mohammed, R. N., Raji, A., Chupradit, S., Yumashev, A. V., Suksatan, W., et al. (2022). Tumor immunotherapies by immune checkpoint inhibitors (ICIs); the pros and cons. Cell Commun. Signal. CCS 20 (1), 44. doi:10.1186/s12964-022-00854-y
Narayan, V., Barber-Rotenberg, J. S., Jung, I. Y., Lacey, S. F., Rech, A. J., Davis, M. M., et al. (2022). PSMA-targeting TGFβ-insensitive armored CAR T cells in metastatic castration-resistant prostate cancer: a phase 1 trial. Nat. Med. 28 (4), 724–734. doi:10.1038/s41591-022-01726-1
O’Cearbhaill, R. E., Deng, W., Chen, L. M., Lucci III, J. A., Behbakht, K., Spirtos, N. M., et al. (2019). A phase II randomized, double-blind trial of a polyvalent Vaccine-KLH conjugate (NSC 748933 IND# 14384)+ OPT-821 versus OPT-821 in patients with epithelial ovarian, fallopian tube, or peritoneal cancer who are in second or third complete remission: an NRG Oncology/GOG study. Gynecol. Oncol. 155 (3), 393–399. doi:10.1016/j.ygyno.2019.09.015
Odunsi, K. (2017). Immunotherapy in ovarian cancer. Ann. Oncol. 28, viii1–viii7. doi:10.1093/annonc/mdx444
Oladapo, O. Y., Rao, T. D., Laster, I., Kononenko, A. P., Purdon, T. J., Wang, P., et al. (2021). Bispecific T-cell engaging antibodies against MUC16 demonstrate efficacy against ovarian cancer in monotherapy and in combination with PD-1 and VEGF inhibition. Front. Immunol. 12, 663379. doi:10.3389/fimmu.2021.663379
Ouyang, X., Telli, M. L., and Wu, J. C. (2019). Induced pluripotent stem cell-based cancer vaccines. Front. Immunol. 10, 1510. doi:10.3389/fimmu.2019.01510
Park, J., Cho, H. W., Lim, M. C., Choi, C. H., and Lee, J. Y. (2024). OPERA: a phase II trial of oregovomab plus non-platinum chemotherapy in PARP inhibitor/platinum-resistant ovarian cancer. Future oncology. 20 (26), 1893–1899. doi:10.1080/14796694.2024.2357533
Pan, X., Shi, X., Zhang, H., Chen, Y., Zhou, J., Shen, F., et al. (2024). Exosomal miR-4516 derived from ovarian cancer stem cells enhanced cisplatin tolerance in ovarian cancer by inhibiting GAS7. Gene 927, 148738. doi:10.1016/j.gene.2024.148738
Pandya, A., Shah, Y., Kothari, N., Postwala, H., Shah, A., Parekh, P., et al. (2023). The future of cancer immunotherapy: DNA vaccines leading the way. Med. Oncol. 40 (7), 200. doi:10.1007/s12032-023-02060-3
Pegtel, D. M., and Gould, S. J. (2019). Exosomes. Annu. Rev. Biochem. 88, 487–514. doi:10.1146/annurev-biochem-013118-111902
Perales-Puchalt, A., Svoronos, N., Rutkowski, M. R., Allegrezza, M. J., Tesone, A. J., Payne, K. K., et al. (2017). Follicle-stimulating hormone receptor is expressed by most ovarian cancer subtypes and is a safe and effective immunotherapeutic target. Clin. Cancer Res. 23 (2), 441–453. doi:10.1158/1078-0432.CCR-16-0492
Perales-Puchalt, A., Wojtak, K., Duperret, E. K., Yang, X., Slager, A. M., Yan, J., et al. (2019). Engineered DNA vaccination against follicle-stimulating hormone receptor delays ovarian cancer progression in animal models. Mol. Ther. 27 (2), 314–325. doi:10.1016/j.ymthe.2018.11.014
Pérez-Baños, A., Gleisner, M. A., Flores, I., Pereda, C., Navarrete, M., Araya, J. P., et al. (2023). Whole tumour cell-based vaccines: tuning the instruments to orchestrate an optimal antitumour immune response. Br. J. Cancer 129 (4), 572–585. doi:10.1038/s41416-023-02327-6
Qi, C., Gong, J., Li, J., Liu, D., Qin, Y., Ge, S., et al. (2022). Claudin18.2-specific CAR T cells in gastrointestinal cancers: phase 1 trial interim results. Nat. Med. 28 (6), 1189–1198. doi:10.1038/s41591-022-01800-8
Ramirez, M. I., and Marcilla, A. (2021). Pathogens and extracellular vesicles: new paths and challenges to understanding and treating diseases. Editorial opinion. Mol. Immunol. 139, 155–156. doi:10.1016/j.molimm.2021.09.006
Ranganath, T., Simpson, L. J., Ferreira, A. M., Seiler, C., Vendrame, E., Zhao, N., et al. (2020). Characterization of the impact of daclizumab beta on circulating natural killer cells by mass cytometry. Front. Immunol. 11, 714. doi:10.3389/fimmu.2020.00714
Reagan, P. M., and Neelapu, S. S. (2021). How I manage: pathophysiology and management of toxicity of chimeric antigen receptor T-cell therapies. J. Clin. Oncol. 39 (5), 456–466. doi:10.1200/JCO.20.01616
Reinhard, K., Rengstl, B., Oehm, P., Michel, K., Billmeier, A., Hayduk, N., et al. (2020). An RNA vaccine drives expansion and efficacy of claudin-CAR-T cells against solid tumors. Science 367 (6476), 446–453. doi:10.1126/science.aay5967
Reusing, S. B., Vallera, D. A., Manser, A. R., Vatrin, T., Bhatia, S., Felices, M., et al. (2021). CD16xCD33 Bispecific Killer Cell Engager (BiKE) as potential immunotherapeutic in pediatric patients with AML and biphenotypic ALL. Cancer Immunol. Immunother. 70 (12), 3701–3708. doi:10.1007/s00262-021-03008-0
Richardson, D. L., Moore, K. N., Vergote, I., Gilbert, L., Martin, L. P., Mantia-Smaldone, G. M., et al. (2024). Phase 1b study of mirvetuximab soravtansine, a folate receptor alpha (FRα)–targeting antibody-drug conjugate, in combination with carboplatin and bevacizumab in patients with platinum-sensitive ovarian cancer. Gynecol. Oncol. 185, 186–193. doi:10.1016/j.ygyno.2024.01.045
Rodriguez-Garcia, A., Minutolo, N. G., Robinson, J. M., and Powell, D. J. (2017). T-cell target antigens across major gynecologic cancers. Gynecol. Oncol. 145 (3), 426–435. doi:10.1016/j.ygyno.2017.03.510
Ruf, P., Bauer, H. W., Schoberth, A., Kellermann, C., and Lindhofer, H. (2021). First time intravesically administered trifunctional antibody catumaxomab in patients with recurrent non-muscle invasive bladder cancer indicates high tolerability and local immunological activity. Cancer Immunol. Immunother. 70 (9), 2727–2735. doi:10.1007/s00262-021-02930-7
Rui, R., Zhou, L., and He, S. (2023). Cancer immunotherapies: advances and bottlenecks. Front. Immunol. 14, 1212476. doi:10.3389/fimmu.2023.1212476
Saad, P., and Kasi, A. (2023). Ipilimumab. Treasure Island (FL): StatPearls Publishing. Available from: https://www.ncbi.nlm.nih.gov/books/NBK557795/ (Accessed April 10, 2023).
Sampson, J. H., Gunn, M. D., Fecci, P. E., and Ashley, D. M. (2020). Brain immunology and immunotherapy in brain tumours. Nat. Rev. Cancer 20 (1), 12–25. doi:10.1038/s41568-019-0224-7
Schuster, H., Peper, J. K., Bösmüller, H. C., Röhle, K., Backert, L., Bilich, T., et al. (2017). The immunopeptidomic landscape of ovarian carcinomas. Proc. Natl. Acad. Sci. 114 (46), E9942-E9951–E9951. doi:10.1073/pnas.1707658114
Shafabakhsh, R., Pourhanifeh, M. H., Mirzaei, H. R., Sahebkar, A., Asemi, Z., and Mirzaei, H. (2019). Targeting regulatory T cells by curcumin: a potential for cancer immunotherapy. Pharmacol. Res. 147, 104353. doi:10.1016/j.phrs.2019.104353
Shen, T., Huang, Z., Shi, C., Pu, X., Xu, X., Wu, Z., et al. (2020). Pancreatic cancer-derived exosomes induce apoptosis of T lymphocytes through the p38 MAPK-mediated endoplasmic reticulum stress. FASEB J. 34 (6), 8442–8458. doi:10.1096/fj.201902186r
Shimizu, A., Sawada, K., Kobayashi, M., Oi, Y., Oride, T., Kinose, Y., et al. (2024). Patient-derived exosomes as siRNA carriers in ovarian cancer treatment. Cancers 16 (8), 1482. doi:10.3390/cancers16081482
Siminiak, N., Czepczyński, R., Zaborowski, M. P., and Iżycki, D. (2022). Immunotherapy in ovarian cancer. Archivum Immunol. Ther. Exp. 70 (1), 19. doi:10.1007/s00005-022-00655-8
Sockolosky, J. T., Trotta, E., Parisi, G., Picton, L., Su, L. L., Le, A. C., et al. (2018). Selective targeting of engineered T cells using orthogonal IL-2 cytokine-receptor complexes. Science 359 (6379), 1037–1042. doi:10.1126/science.aar3246
Stump, C. T., Ho, G., Mao, C., Veliz, F. A., Beiss, V., Fields, J., et al. (2021). Remission-stage ovarian cancer cell vaccine with cowpea mosaic virus adjuvant prevents tumor growth. Cancers 13 (4), 627. doi:10.3390/cancers13040627
Tang, Q., Chen, Y., Li, X., Long, S., Shi, Y., Yu, Y., et al. (2022a). The role of PD-1/PD-L1 and application of immune-checkpoint inhibitors in human cancers. Front. Immunol. 13, 964442. doi:10.3389/fimmu.2022.964442
Tang, S., Qin, C., Hu, H., Liu, T., He, Y., Guo, H., et al. (2022b). Immune checkpoint inhibitors in non-small cell lung cancer: progress, challenges, and prospects. Cells 11 (3), 320. doi:10.3390/cells11030320
Tanyi, J. L., Chiang, C. L. L., Chiffelle, J., Thierry, A. C., Baumgartener, P., Huber, F., et al. (2021). Personalized cancer vaccine strategy elicits polyfunctional T cells and demonstrates clinical benefits in ovarian cancer. npj Vaccines 6 (1), 36. doi:10.1038/s41541-021-00332-5
Tapia-Galisteo, A., Álvarez-Vallina, L., and Sanz, L. (2023). Bi- and trispecific immune cell engagers for immunotherapy of hematological malignancies. J. Hematol. & Oncol. 16 (1), 83. doi:10.1186/s13045-023-01482-w
Taylor, D. D., Gerçel-Taylor, C., Lyons, K. S., Stanson, J., and Whiteside, T. L. (2003). T-cell apoptosis and suppression of T-cell receptor/CD3-zeta by Fas ligand-containing membrane vesicles shed from ovarian tumors. Clin. Cancer Res. 9 (14), 5113–5119.
Tchou, J., Zhao, Y., Levine, B. L., Zhang, P. J., Davis, M. M., Melenhorst, J. J., et al. (2017). Safety and efficacy of intratumoral injections of chimeric antigen receptor (CAR) T cells in metastatic breast cancer. Cancer Immunol. Res. 5 (12), 1152–1161. doi:10.1158/2326-6066.CIR-17-0189
Thieblemont, C., Phillips, T., Ghesquieres, H., Cheah, C. Y., Clausen, M. R., Cunningham, D., et al. (2022). Epcoritamab, a novel, subcutaneous CD3xCD20 bispecific T-cell–engaging antibody, in relapsed or refractory large B-cell lymphoma: dose expansion in a phase I/II trial. J. Clin. Oncol. 41 (12), 2238–2247. doi:10.1200/jco.22.01725
Tian, W., Lei, N., Zhou, J., Chen, M., Guo, R., Qin, B., et al. (2022). Extracellular vesicles in ovarian cancer chemoresistance, metastasis, and immune evasion. Cell death & Dis. 13 (1), 64. doi:10.1038/s41419-022-04510-8
Tokatlian, T., Asuelime, G. E., Mock, J. Y., DiAndreth, B., Sharma, S., Toledo Warshaviak, D., et al. (2022). Mesothelin-specific CAR-T cell therapy that incorporates an HLA-gated safety mechanism selectively kills tumor cells. J. Immunother. cancer 10 (1), e003826. doi:10.1136/jitc-2021-003826
Tse, B. W., Collins, A., Oehler, M. K., Zippelius, A., and Heinzelmann-Schwarz, V. A. (2014). Antibody-based immunotherapy for ovarian cancer: where are we at? Ann. Oncol. 25 (2), 322–331. doi:10.1093/annonc/mdt405
Vallera, D. A., Ferrone, S., Kodal, B., Hinderlie, P., Bendzick, L., Ettestad, B., et al. (2020). NK-Cell-Mediated targeting of various solid tumors using a B7-H3 tri-specific killer engager in vitro and in vivo. Cancers 12 (9), 2659. doi:10.3390/cancers12092659
Van Coillie, S., Wiernicki, B., and Xu, J. (2020). Molecular and cellular functions of CTLA-4. Adv. Exp. Med. Biol. 1248, 7–32. doi:10.1007/978-981-15-3266-5_2
van de Donk, N. W. C. J., and Zweegman, S. (2023). T-cell-engaging bispecific antibodies in cancer. Lancet 402 (10396), 142–158. doi:10.1016/S0140-6736(23)00521-4
Wada, S., Yada, E., Ohtake, J., Fujimoto, Y., Uchiyama, H., Yoshida, S., et al. (2016). Current status and future prospects of peptide-based cancer vaccines. Immunotherapy 8 (11), 1321–1333. doi:10.2217/imt-2016-0063
Wang, B., Pei, J., Xu, S., Liu, J., and Yu, J. (2023a). Recent advances in mRNA cancer vaccines: meeting challenges and embracing opportunities. Front. Immunol. 14, 1246682. doi:10.3389/fimmu.2023.1246682
Wang, L., Luo, Y., Ren, S., Zhang, Z., Xiong, A., Su, C., et al. (2023b). A phase 1b study of ivonescimab, a PD-1/VEGF bispecific antibody, as first-or second-line therapy for advanced or metastatic immunotherapy naïve non-small-cell lung cancer. J. Thorac. Oncol. Official Publ. Int. Assoc. Study Lung Cancer, S1556–S0864. doi:10.1016/j.jtho.2023.10.014
Wang, Q., Chen, Y., Park, J., Liu, X., Hu, Y., Wang, T., et al. (2019). Design and production of bispecific antibodies. Antibodies 8 (3), 43. doi:10.3390/antib8030043
Wang, Q., Zhang, X., Li, B., Liu, X., Li, A., Li, H., et al. (2024). Tumor-derived exosomes promote tumor growth through modulating microvascular hemodynamics in a human ovarian cancer xenograft model. Microcirculation 31, e12876. doi:10.1111/micc.12876
Wu, B., Yu, C., Zhou, B., Huang, T., Gao, L., Liu, T., et al. (2017). Overexpression of TROP2 promotes proliferation and invasion of ovarian cancer cells. Exp. Ther. Med. 14 (3), 1947–1952. doi:10.3892/etm.2017.4788
Wu, Z., and Cheung, N. V. (2018). T cell engaging bispecific antibody (T-BsAb): from technology to therapeutics. Pharmacol. & Ther. 182, 161–175. doi:10.1016/j.pharmthera.2017.08.005
Xiao, Y., Bi, M., Guo, H., and Li, M. (2022). Multi-omics approaches for biomarker discovery in early ovarian cancer diagnosis. EBioMedicine 79, 104001. doi:10.1016/j.ebiom.2022.104001
Xu, L., Sun, H., Lemoine, N. R., Xuan, Y., and Wang, P. (2024). Oncolytic vaccinia virus and cancer immunotherapy. Front. Immunol. 14, 1324744. doi:10.3389/fimmu.2023.1324744
Yeh, J. M., Ward, Z. J., Chaudhry, A., Liu, Q., Yasui, Y., Armstrong, G. T., et al. (2020). Life expectancy of adult survivors of childhood cancer over 3 decades. JAMA Oncol. 6 (3), 350–357. doi:10.1001/jamaoncol.2019.5582
Yi-Ju, C., Abila, B., and Kamel, Y. M. (2023). CAR-T: what is next? Cancers 15 (3), 663. doi:10.3390/cancers15030663
Yim, K. H. W., Al Hrout, A., Borgoni, S., and Chahwan, R. (2020). Extracellular vesicles orchestrate immune and tumor interaction networks. Cancers 12 (12), 3696. doi:10.3390/cancers12123696
Zalatan, J. G., Petrini, L., and Geiger, R. (2024). Engineering bacteria for cancer immunotherapy. Curr. Opin. Biotechnol. 85, 103061. doi:10.1016/j.copbio.2023.103061
Zhang, X., He, T., Li, Y., Chen, L., Liu, H., Wu, Y., et al. (2021). Dendritic cell vaccines in ovarian cancer. Front. Immunol. 11, 613773. doi:10.3389/fimmu.2020.613773
Zhang, Z. J., Chen, X. H., Chang, X. H., Ye, X., Li, Y., and Cui, H. (2012). Human embryonic stem cells-a potential vaccine for ovarian cancer. Asian Pac. J. cancer Prev. APJCP 13 (9), 4295–4300. doi:10.7314/apjcp.2012.13.9.4295
Zhao, S., Mi, Y., Guan, B., Zheng, B., Wei, P., Gu, Y., et al. (2020). Tumor-derived exosomal miR-934 induces macrophage M2 polarization to promote liver metastasis of colorectal cancer. J. Hematol. & Oncol. 13 (1), 156. doi:10.1186/s13045-020-00991-2
Zhao, Y., Chen, G., Chen, J., Zhuang, L., Du, Y., Yu, Q., et al. (2023). AK112, a novel PD-1/VEGF bispecific antibody, in combination with chemotherapy in patients with advanced non-small cell lung cancer (NSCLC): an open-label, multicenter, phase II trial. EClinicalMedicine 62, 102106. doi:10.1016/j.eclinm.2023.102106
Zhou, M., Tang, Y., Xu, W., Hao, X., Li, Y., Huang, S., et al. (2023). Bacteria-based immunotherapy for cancer: a systematic review of preclinical studies. Front. Immunol. 14, 1140463. doi:10.3389/fimmu.2023.1140463
Zhou, W., Zhou, Y., Chen, X., Ning, T., Chen, H., Guo, Q., et al. (2021). Pancreatic cancer-targeting exosomes for enhancing immunotherapy and reprogramming tumor microenvironment. Biomaterials 268, 120546. doi:10.1016/j.biomaterials.2020.120546
Zhu, K., Ma, J., Tian, Y., Liu, Q., and Zhang, J. (2024). An immune-related exosome signature predicts the prognosis and immunotherapy response in ovarian cancer. BMC women's health 24 (1), 49. doi:10.1186/s12905-024-02881-y
Keywords: ovarian cancer, immunotherapy, cancer vaccines, CAR-T cell therapy, antibody therapy
Citation: Massariol Pimenta T, Carlos de Souza J, da Silva Martins B, Silva Butzene SM, Simões Padilha JM, Ganho Marçal M, dos Santos Elias G and Rangel LBA (2024) Emerging strategies to overcome ovarian cancer: advances in immunotherapy. Front. Pharmacol. 15:1490896. doi: 10.3389/fphar.2024.1490896
Received: 03 September 2024; Accepted: 21 October 2024;
Published: 05 November 2024.
Edited by:
Zhaoqian Liu, Central South University, ChinaReviewed by:
Pratik Bhojnagarwala, Wistar Institute, United StatesFabian Benencia, Ohio University, United States
Ming Yi, Zhejiang University, China
Copyright © 2024 Massariol Pimenta, Carlos de Souza, da Silva Martins, Silva Butzene, Simões Padilha, Ganho Marçal, dos Santos Elias and Rangel. This is an open-access article distributed under the terms of the Creative Commons Attribution License (CC BY). The use, distribution or reproduction in other forums is permitted, provided the original author(s) and the copyright owner(s) are credited and that the original publication in this journal is cited, in accordance with accepted academic practice. No use, distribution or reproduction is permitted which does not comply with these terms.
*Correspondence: Leticia Batista Azevedo Rangel, bGJhcmFuZ2VsQHlhaG9vLmNvbQ==
†These authors have contributed equally to this work