- 1College of Biomass Science and Engineering, Sichuan University, Chengdu, China
- 2College of Life Science and Engineering, Northwest Minzu University, Lanzhou, China
- 3School of Liquor-Brewing Engineering, Sichuan University of Jinjiang College, Meishan, China
This study investigated the metabolic characteristics of mulberry wine produced by co-fermentation with Saccharomyces cerevisiae (SC) and two different Pichia kluyveri (PK). Although S. cerevisiae inhibited the growth of P. kluyveri during co-fermentation, P. kluyveri showed robust growth adaptability. Classical oenological parameters were not significantly altered by co-fermentation compared to pure-fermentation. The P. kluyveri significantly modulated amino acid metabolism pathways during co-fermentation, enhancing the biosynthesis of higher alcohol acetate compounds. Furthermore, co-fermentation strategy promoted the production of volatile flavor compounds, particularly esters and alcohols, which enriched the wine with distinct floral and fruity flavors. This study provides novel insights into the metabolic mechanisms of co-fermentation with SC and PK strains and highlights the potential of P. kluyveri as a co-fermentation agent for improving the aromatic complexity of fruit wines.
1 Introduction
Although grapes still dominate the fruit wine market, changing consumer habits mean that other types of fruit wine are gradually coming to the fore (1). Actually, due to their unique flavor and reasonable sugar level, many temperate and tropical fruits have great potential in the new fruit wine industry (2). Mulberry (Morus alba L.) is widespread throughout the world, from tropical to temperate areas (3, 4). After alcoholic fermentation, mulberry wine has a complex aroma, elegant taste and a distinctive purple-black color (5). Mulberries consist of clusters of small, juicy drupelets, making them highly susceptible to mechanical damage and resulting in a limited postharvest shelf-life. Consequently, the production of fruit wines represents a viable strategy for enhancing the economic value of mulberries.
In the fermentation process of fruit wine, yeast plays an important role due to its high capacity to produce alcohol and aromatic compounds (6). The yeast mentioned here is, of course, mainly Saccharomyces cerevisiae. S. cerevisiae is mainly used for its high alcohol production capacity and good tolerance in the traditional fruit wine industry. However, the widespread use of commercial yeasts can lead to homogenization and a lack of flavor diversity in fruit wines made from different raw materials (7). On the other hand, it has been confirmed that some non-Saccharomyces are more efficient at producing flavor substances such as esters and other aroma compounds, but less efficient at producing alcohol (7). This is an important factor that limits the industrial use of non-Saccharomyces yeasts. As a promising way to increase the flavor diversity of fruit wines, co-fermentation by S. cerevisiae and non-Saccharomyces has been proposed (8, 9). With this in mind, there is great interest in exploring new co-fermentation strategies using S. cerevisiae and different non-Saccharomyces yeasts, as these yeasts add complexity to flavors and increase the yield of desirable compounds (10, 11).
Previous report has confirmed that co-fermentation by Pichia and S. cerevisiae could increases the flavor composition (12). In our previous research, we found the “increased flavor composition” due to the excellent performance of Pichia kluyveri in production of esters and higher alcohols (13). This characteristic of P. kluyveri has the potential to improve the flavor of fruit wines fermented with materials that lack varietal aroma. Therefore, to achieve effective control and standardization of co-fermentation processes, it is essential to investigate the interaction mechanisms between S. cerevisiae and P. kluyveri that contribute to the enhancement of wine flavor characteristics.
In this sense, the main objective of this study was to investigate the fermentation quality, yeast cell viability, and metabolic profiles (volatile and non-volatile) in mulberry juice fermentations using pure or co-fermentation with P. kluyveri and S. cerevisiae. Finally, elucidation of the major impacted metabolic regulation pathways and key metabolites involved in co-fermentation, for a comprehensive evaluation of the effect of the co-fermentation strategy on mulberry wine characteristics.
2 Materials and methods
2.1 Materials and reagents
Fresh mulberries of “Yun No. 2” (with 12 ± 2 °Bx) were provided by Sixi Agricultural Development Co., Ltd. (Panzhihua, China). All strains used in this study were screened from the “Yanbian” mulberry orchard (Panzhihua, China). The Saccharomyces cerevisiae and two different Pichia kluyveri were abbreviated as SC, PK1, and PK2, respectively.
Yeast peptone dextrose (YPD) broth medium and Wallerstein nutrient agar (WL) were purchased from Qingdao Hope Bio-Technology Co., Ltd (Qingdao, China). The 4-Methyl-2-pentanol (internal standard for GC-MS) and n-alkanes C6 to C30 (calculate the retention index, RI) were purchased from Sigma-Aldrich Co. (Saint Louis, USA).
2.2 Fermentation conditions
Mulberry wine-making followed our previously described method (13). Mulberry juice was prepared by crushing mulberry fruit. Mulberry juice with pectinase (> 500 U/mg) for enzymatic hydrolysis at 20°C for 24 h. Addition of sucrose to pasteurized mulberry juice for standardization (Brix, 25 °Bx). The effective concentration of sulfur dioxide in the mulberry juice was adjusted to 50 mg/L using potassium metabisulphite. The different strains were pre-cultured (28°C, 170 rpm) separately in YPD medium for 40 h. After incubation, resuspend the yeast cells in a sterile physiological saline solution. Each strain was inoculated separately at 106 CFU/mL.
The fermentation groups by SC (S. cerevisia), PK1 (P. kluyveri), and PK2 (P. kluyveri) strains pure-fermented were set up as F(S), F(P1), and F(P2), respectively. The group co-fermented by SC and PK1 strains as group F(S-P1). Similarly, F(S-P2) represented the co-fermentation group of SC and PK2 strains. The fermentation process for each experimental group was terminated when the PK strain was no longer detectable in the co-fermentation.
2.3 Microbial counting
During fermentation, yeast counts were carried out every 3 days using WL agar plate (14). One milliliter of fermenting mulberry juice was diluted to 10−4 and 10−5. Plated on WL agar, incubated at 28°C for 48 h to facilitate separate yeast population counts. The colonies of S. cerevisiae (dark green, smooth colony) and P. kluyveri (light green, rough colony) could be distinguished by different color and morphology.
2.4 Physicochemical analysis
According to previous method (15), soluble solids content (SSC) and pH were monitored every 3 days. The content of SSC was measured by a digital refractometer (BM-04S, Tianjin Nohawk Optoelectronic Technology Co., Ltd, Tianjin, China). The pH was determined using a pH meter (PHSJ-5T, Shanghai INESA Scientific Instrument Co., Ltd, Shanghai, China). The titratable acidity content (TA), the ethanol content and the residual sugars content of the wine were determined at the end of the fermentation process.
2.5 Non-volatile component analysis
The Samples (the fermentation broth containing the strains) were thawed at 4°C and vortexed for 1 min to ensure homogeneous mixing. The sample was transferred to a 2 mL centrifuge tube, 500 μL of methanol solution (−20°C) was added. The tube was vortexed for 1 min. Following centrifugation at 12,000 rpm for 10 min at 4 °C, the entire supernatant was transferred to a new 2 mL centrifuge tube for concentration and drying. Subsequently, 300 μL of a solution of 2-amino-3-(2-chlorophenyl) propionic acid (4 ppm) prepared in 80% methanol/water (v/v) was added to the sample. The supernatant was finally filtered through a 0.22 μm membrane, and the filtrate was collected into sample vials for subsequent LC-MS analysis.
Non-volatile component analyzed by Vanquish UHPLC System (Thermo Fisher Scientific, USA) coupled to an Q Exactive Focus (Thermo Fisher Scientific, USA). The connected chromatographic column of choice was of ACQUITY UPLC HSS T3 (150 × 2.1 mm, 1.8 μm, Waters Corp., USA). The temperature of column was kept at 40°C. Flow rate was set at 0.25 mL/min and the volume of the injection at 2 μL. Preparation of four sets of mobile phases, 0.1% formic acid in water (labeled as A1), 0.1% formic acid in acetonitrile (labeled as B1), 0.005 mol/L ammonium formate (labeled as A2) and acetonitrile (labeled as B2). Mobile phases A1 and B1 were used in the positive ion detection mode. Separation gradient programme followed 2% B1 at 0–1 min, 2–50% B1 at 1–9 min, 50–95% B1 at 9–12 min, 95% B1 at 12–14 min, and 95–2% B1 at 14–15 min, 2% B1 at 15–20 min. For negative ion detection mode, the mobile phases A2 and B2 were used. Separation gradient programme followed 2% B2 at 0–1 min, 2–50% B2 at 1–10 min, 50–95% B2 at 10–12 min, 95% B2 at 12–14 min, 95–2% B2 at 14–15 min and at 2% B2 at 15–20 min. The MS/MS parameters were based on previous research (16). It should be noted that the spray voltage set at 2.50 kV and −2.50 kV in positive and negative ion detection mode respectively.
2.6 Volatile composition analysis
The volatile compounds of young mulberry wine were analyzed by Headspace solid-phase microextraction-gas chromatography-mass spectrometry (HS-SPME/GC-MS) with some changes based on previous study (17). Briefly, 4 mL of mulberry wine was mixed with 0.4 g NaCl and 10 μL of internal standard (4-Methyl-2-pentyl alcohol, 81.8 μg/mL in water) and placed in headspace vials, then stored at 4°C before analysis. Each sample should be heated 10 min at 60°C before extraction. Volatiles were then extracted from headspace vials using SPME fibers (50/30 μm DVB/CAR/PDMS, Supelco, Bellefonte, PA). The temperature of extraction process was kept at 60°C for 40 min. All samples were analyzed for the presence of volatiles using Trace 1,310 GC (Thermo Fisher Scientific, USA) coupled to a TSQ 9,000 MS (Thermo Fisher Scientific, USA). Selection of a capillary column of the VF-WAXms (30 m × 0.25 mm × 0.25 μm, Agilent Technologies, USA). The adsorbed compounds in the SPME fiber were desorbed in the Trace 1,310 in the splitless mode at 240°C for 10 min. A flow rate of 1.6 mL/min was set for helium as the carrier gas. The oven temperature was set as 40°C for 2 min. The oven temperature was then increased to 50°C at a rate of 4°C/min, followed by an increase to the end point of 200°C at a rate of 5°C/min and held for 5 min to the end of the programme. The analytes were scanned from 30 to 400 m/z using electron impact (EI) ionization mode at 70 eV. The temperature of MS transfer line was set at 200°C and the ionization source temperature was at 220°C. All compounds detected were identified by comparing their retention indices (RI) with those of alkane (C6–C30) and by mass matching against the National Institute of Standards and Technology (NIST) database.
2.7 Data analysis
SPSS 24.0 (SPSS-IBM Inc., USA) was used for analysis of variance (ANOVA). The principal component analysis (PCA) was performed using the online tool MetaboAnalyst (https://www.metaboanalyst.ca/). PCA was normalized using Pareto scaling. The heatmap was performed using the online tool Chiplot (www.chiplot.online).
3 Results and discussion
3.1 Growth of yeasts cells during fermentation
The growth kinetics of S. cerevisia (SC), P. kluyveri (PK1), and P. kluyveri (PK2) in pure and co-fermentation were shown in Figure 1. All three pure culture strains showed similar growth trends during the first 3 days. After 3 days, a different trend of slow growth was observed. It was also similar to previous reports from Yu et al. (17). As shown in Figure 1A, the SC cells showed consistent growth after 3 days, eventually reaching the maximum number at 8.25 Log CFU/mL. The PK1 cell population remained stable from day 3 to day 15, followed by a brief increase, reaching a maximum concentration of 8.39 Log CFU/mL at day 18 (Figure 1B). In contrast, increasing numbers of PK2 cells were observed prior to day 9, followed by a relatively short period of stability from day 9 to day 15 (Figure 1C). The number of cells tended to decrease gradually after reaching a maximum of 8.52 Log CFU/mL at 15 d (Figure 1C). For the co-fermentation of SC and PK (PK1 or PK2), the growth of two yeasts were affected by the presence of each other (Figures 1D, E). In the co-fermentation group of F(S-P1) and F(S-P2), the cells of the SC and PK (PK1 or PK2) grew rapidly in the first 3 days and then became essentially stable. After 3 days, the SC strains in group F(S-P1) exhibited a slight improvement in stability, reaching its maximum of 8.23 Log CFU/mL at 18 d. In group F(S-P1), the PK1 strains displayed a declining trend in stability. The PK1 cell population underwent a sharp decline, particularly at day 15, and was no longer detectable by day 18 (Figure 1D).
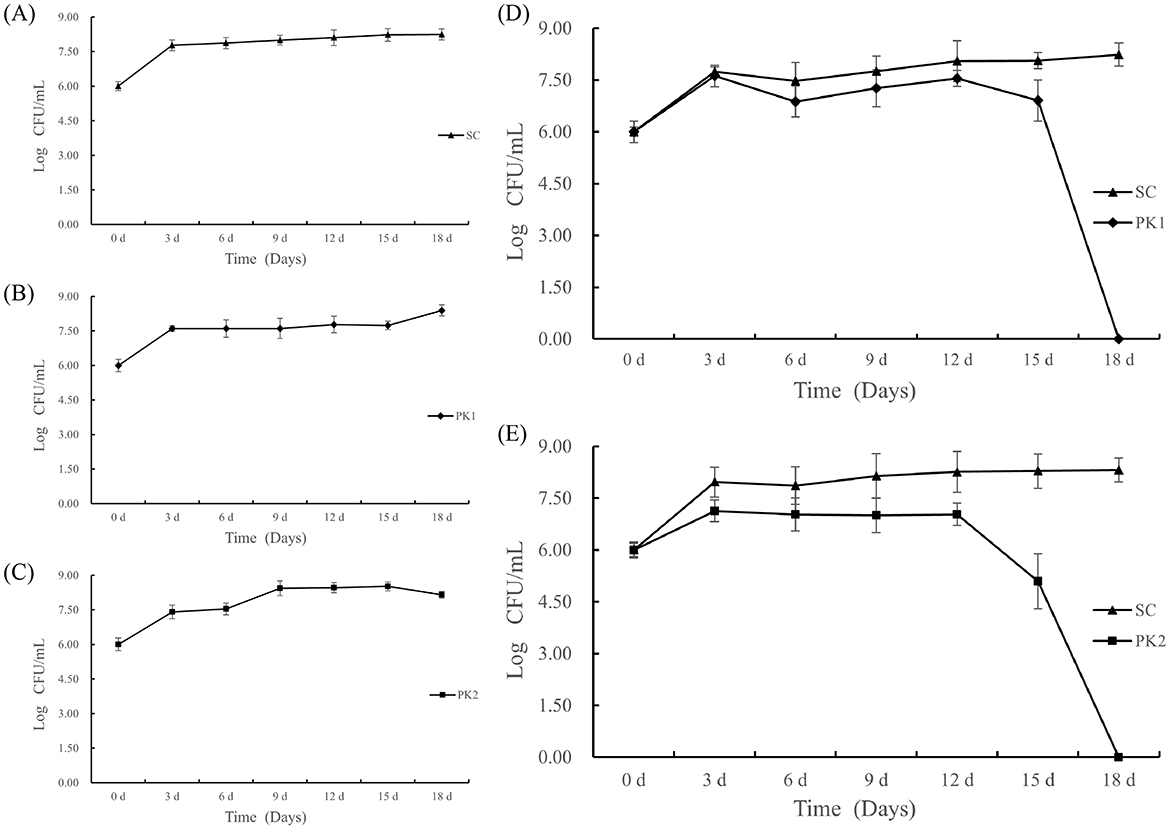
Figure 1. Changes in yeast cell counts (expressed as log CFU/mL) during mulberry wine fermentation. (A) Represent pure-fermentation with S. cerevisiae (SC), (B) Represent pure-fermentation with P. kluyveri (PK1), (C) Represent pure-fermentation with P. kluyveri (PK2), (D) Represent co-fermentation with S. cerevisiae (SC) and P. kluyveri (PK1), (E) Represent co-fermentation with S. cerevisiae (SC) and P. kluyveri (PK2).
Correspondingly, the growth kinetics of SC and PK2 strains in group F(S-P2) exhibited patterns similar to those observed in group F(S-P1). However, the PK2 strains exhibited an earlier onset of extinction during co-fermentation, commencing at day 12 and reaching complete depletion by day 18 (Figure 1E). In the F(S-P2) group, the SC strains reached its maximum cell count of 8.31 Log CFU/mL at 18 d, whereas the PK2 strains did not reach its maximum cell count of 7.13 Log CFU/mL until 3 d (Figure 1E). This suggested that the presence of SC influenced the growth of PK (PK1 or PK2) (18). This result might be due to the toxic effect of alcohol and nutritional competition (17). Compared to the PK1, the PK2 was more drastically affected by SC strains.
3.2 Classical oenological parameters
The physicochemical characteristics of mulberry juice and wines were shown in Table 1. The final pH values were almost the same in the pure-fermentation broth F(S), F(P1), and F(P2). The co-fermentation groups F(S-P1) and F(S-P2) were also almost identical. Compared to MJ, all fermentation groups improved slightly. The levels of titratable acidity in all fermentation solution samples were between 2.6 and 3.1 g/L. MJ had a relatively low original acidity of only 1.7 g/L in terms of titratable acidity. All samples had a variable increase in acidity after fermentation. An appropriate increase in the acidity level could help to improve the flavor of the fruit wine and make it more mellow (19). In this study the titratable acid of F(S) was found to be only 2.6 g/L, which was close to that of F(P1). It was found that SC and PK1 strains had limited ability to produce acid during fermentation. This resulted in the acid content of co-fermentation F(S-P1) was only 2.7 g/L. However, the PK2 strain was more capable of acid production. The titratable acid of sample F(S-P2) was 3.1 g/L. These results indicated that the acid production capacity of SC and PK (PK1 and PK2) strains in co-fermentation did not have an effect on each other. In comparison with F(S-P1), F(S-P2) had a more acidic taste in the same pH conditions.
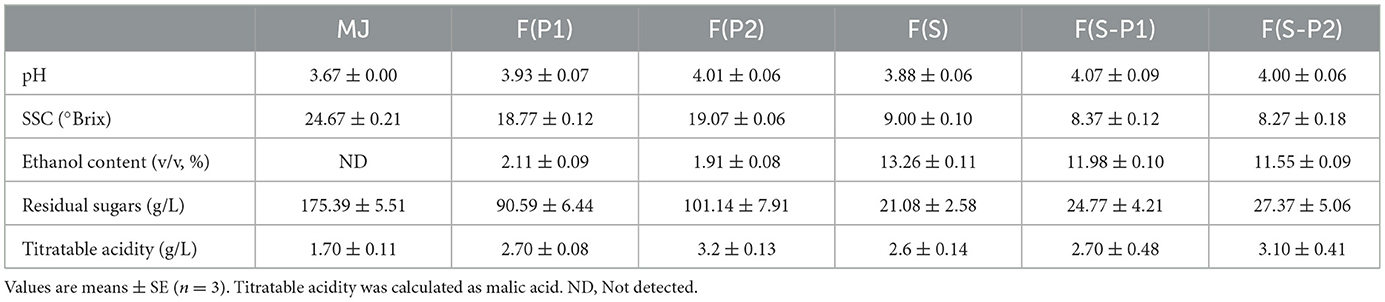
Table 1. Physicochemical parameters of mulberry juice and mulberry wine (day 18) fermented with S. cerevisiae and P. kluyveri.
There was a significant loss of sugar from the mulberry juice in all mulberry wines with SC strains involved in fermentation. The SSC for F(S), F(S-P1), and F(S-P2) were 9, 8.37 and 8.27%, respectively. Correspondingly, the residual sugar content of the three groups of mulberry wines was 21.08, 24.77, and 27.37 g/L, respectively. The large reduction in the sugar content of the three groups of mulberry wines was also reflected in the increased alcohol content. F(S) had the highest alcohol content at 13.26%. The alcohol content of F(S-P1) and F(S-P2) was 11.98 and 11.55%, respectively. This result indicated that the addition of PK strains did not negatively affect the dominance of SC strains in the fermentation. The alcohol-producing capacity of the S. cerevisiae still functioned properly in the co-fermentation. It should be noted that co-fermentation produced less alcohol than the pure-fermentation of S. cerevisiae. This may be related to the nutrient competition effect caused by non-Saccharomyces in the co-fermentation, which prolongs the latency of the S. cerevisiae growth process and delays the start of alcoholic fermentation (20).
3.3 Analysis of non-volatile compounds
All samples corresponding to different fermentation strains were first visually distinguished using Principal Component Analysis (PCA). As shown in Figure 2A, the quality control (QC) samples had a good tendency to cluster. This was an indication that the analysis was reliable and reproducible. The two principal components expressed about 44.2% of the total variance, with PC1 and PC2 accounting for 30.4 and 13.8%, respectively (Figure 2A).
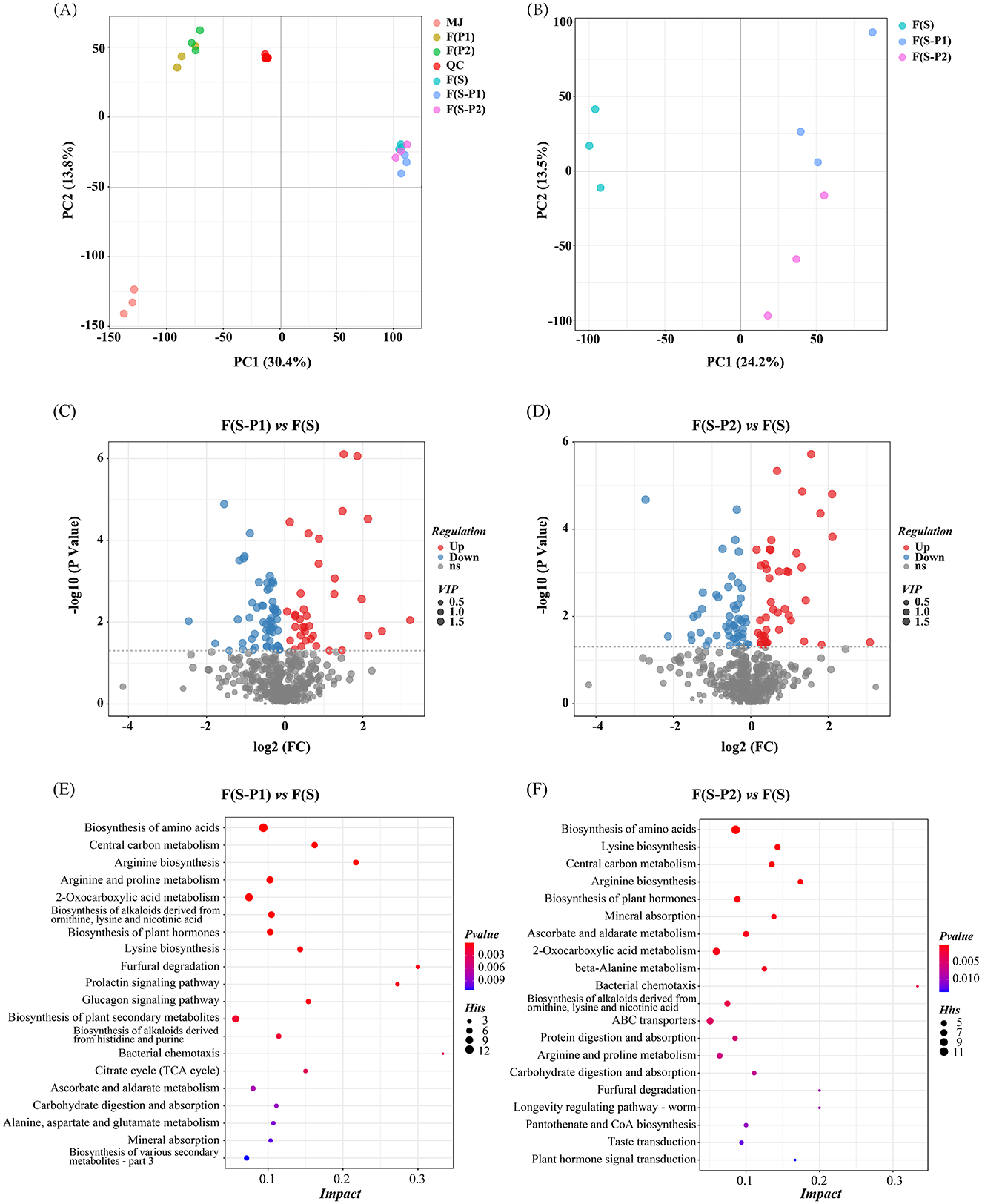
Figure 2. (A, B) Principal component analysis of non-volatile components (PCA) score plot of non-volatile components for reliability verification, positive ionization mode; (C, D) Volcano plots showing differential metabolites between different mulberry wines (P < 0.05, VIP values > 1); (E, F) The most prominent pathway (Top 20) enriched KEGG terms among the differential accumulated metabolites (DAMs).
The results also showed significant differences in the grouping of fermentation broth from different strains. Furthermore, F(P1) and F(P2) metabolite spectra were relatively similar and clustered together. Instead, F(S-P1) and F(S-P2) had been clustered together with F(S). Two specimen sets showed good separation at PC1. This result indicated that the fermenting broth of SC strains dominated the grouping. With a further focus on three types of mulberry wine F(S), F(S-P1), and F(S-P2), as shown in Figure 2B, all wine samples showed clear separation. The first component (PC1, 24.2%) separated F(S) from other co-fermentation samples. The F(S-P1) and F(S-P2) samples were clearly separated by the second component (PC2, 13.5 %). F(S) was clearly separated from the other samples and held a dominant position, as shown in Figure 2B.
The differing patterns of metabolite accumulation could be clearly visualized using clustering heatmaps (Supplementary Figure S1). The results showed significant differences in metabolites between different samples and clear clusters were formed. The clustering heatmap also showed good clustering between different biological replicates. Meanwhile, the clustering trend of the samples was consistent with the PCA (Figure 2B) conclusion. The three types of mulberry wines [F(S), F(S-P1), and F(S-P2)] appeared to be clustered, with F(S) occupying a dominant position (Figure 2A). All metabolites detected in Supplementary Figure S1 were listed in Supplementary Table S1.
Finally, the focus was on the differences between pure fermentation (SC) and co-fermentation (with PK1 or PK2) of mulberry wine. The P < 0.05 and VIP values >1 were considered to be statistically significant metabolites (Figures 2C, D). By comparing F(S-P1) and F(S), a total of 97 differential metabolites were identified (Figure 2C, Supplementary Table S2, and Supplementary Figure S2). On the other hand, In the comparison of F(S-P2) and F(S), 94 different metabolites were identified (Figure 2D, Supplementary Table S3, and Supplementary Figure S3).
To further identify metabolic pathways in the different mulberry wines, the differential accumulated metabolites (DAMs) were mapped in the KEGG database. KEGG functional enrichment analysis was also performed to assess the presence and distribution of DAMs (Figures 2E, F). Amino acid biosynthesis or metabolism were the most prominent pathway enriched KEGG term among the DAMs detected for all compared samples. Given the unique role of amino acids in the flavor of fruit wine (17), it was reasonable to speculate that DAMs in the amino acids biosynthesis or metabolism pathway might be the key reason leading to flavor changes in the co-fermentation of mulberry wine. These results underlined the importance of these compounds in the flavor differences of co-fermented mulberry wine.
The key pathways involved in the significant differential metabolites (SDMs) provided a visual demonstration of the differences between the different co-fermentation strategies (Figure 3). The initial metabolism of the SC strains was under the influence of the co-fermentation. The PK strains significantly disturbed the TCA cycle and the corresponding amino acid metabolism as shown in Figure 3. In comparison to the PK2 strains, the PK1 strains had a greater effect on the TCA cycle, which in turn had a more extensive global effect on amino acid metabolism, fatty acid metabolism and other processes (Figure 3A). The PK2 strains, however, mainly improved the metabolism of amino acids. Several pathways of amino acid metabolism were involved in SDMs (Figure 3B). Furthermore, the comparison showed that SDMs in the different co-fermentation strategies could be traced to substances, L-aspartic acid and 2-oxoglutarate. Coincidentally, both substances showed a downward trend in different co-fermentation strategies. The amino acid pathways involved in 2-Oxoglutarate became active and the corresponding TCA cycle was disturbed. This also explained the accumulation of citrate and isocitrate in the TCA pathway of F(S-P1). But this phenomenon did not appear at F(S-P2). In addition, L-aspartic acid is a key substance for the formation of other amino acids by micro-organisms (21). This also confirmed the decreased concentration of L-asparagine in co-fermentation, possibly due to the overall high level of amino acid metabolism. The amino acids are precursors for many flavor compounds (22). It is possible that the PK strain improved amino acid metabolism during the co-fermentation process, which contributed to a significant increase in the levels of aroma compounds and an improvement in the flavor type of mulberry wine.
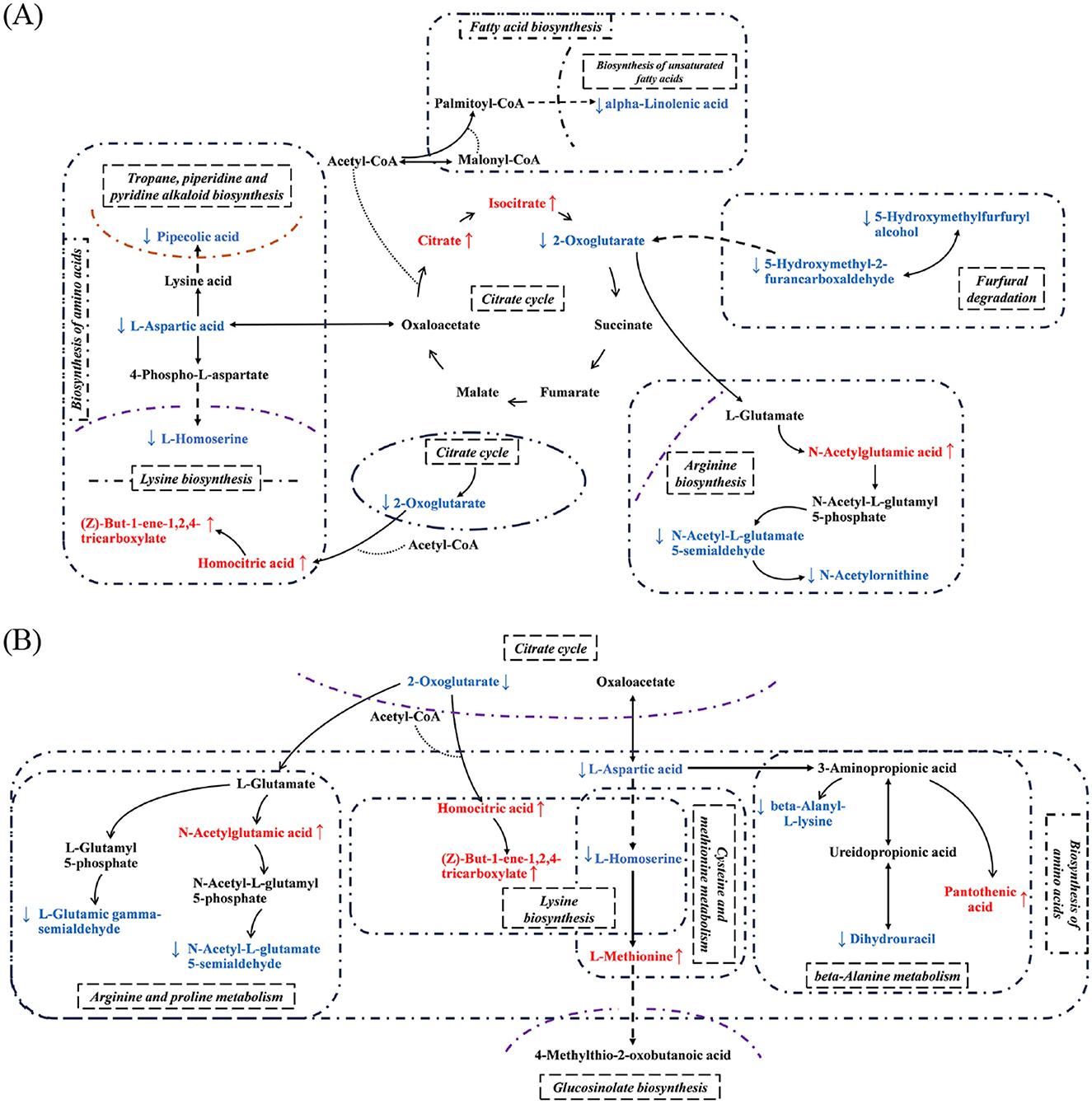
Figure 3. Integration map of prominent pathway. (A) F(S-P1), (B) F(S-P2). The arrow pointing upwards indicates increased in concentration levels; The arrow pointing downwards indicates decreased in concentration levels.
3.4 Volatile flavor compounds of mulberry wine
After fermentation with pure or co-culture, as shown in Figure 4A, the types and levels of volatile flavor compounds varied considerably. The contents of identified volatiles were presented in Table 2. A total of 63 volatiles were detected in different fermentation broths, as shown in Figure 4. The volatile compounds included 29 esters, 19 alcohols, and 3 ketones compounds. There were four each of acids, aldehydes and others.
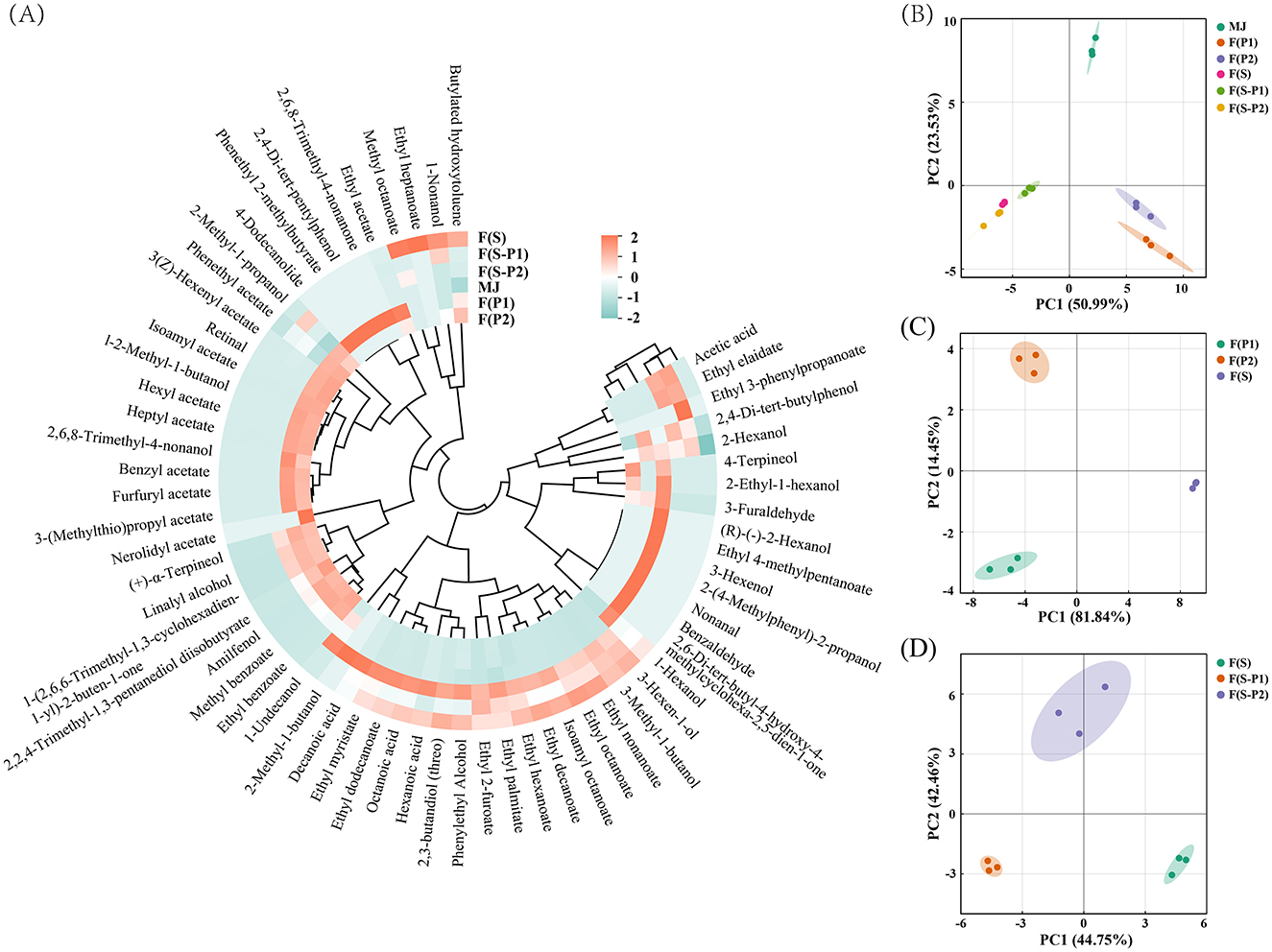
Figure 4. (A) Heatmap of characteristic volatile compounds in different mulberry fermentation broth (18 d), (B–D) Principal component analysis (PCA) score plot of volatile components.
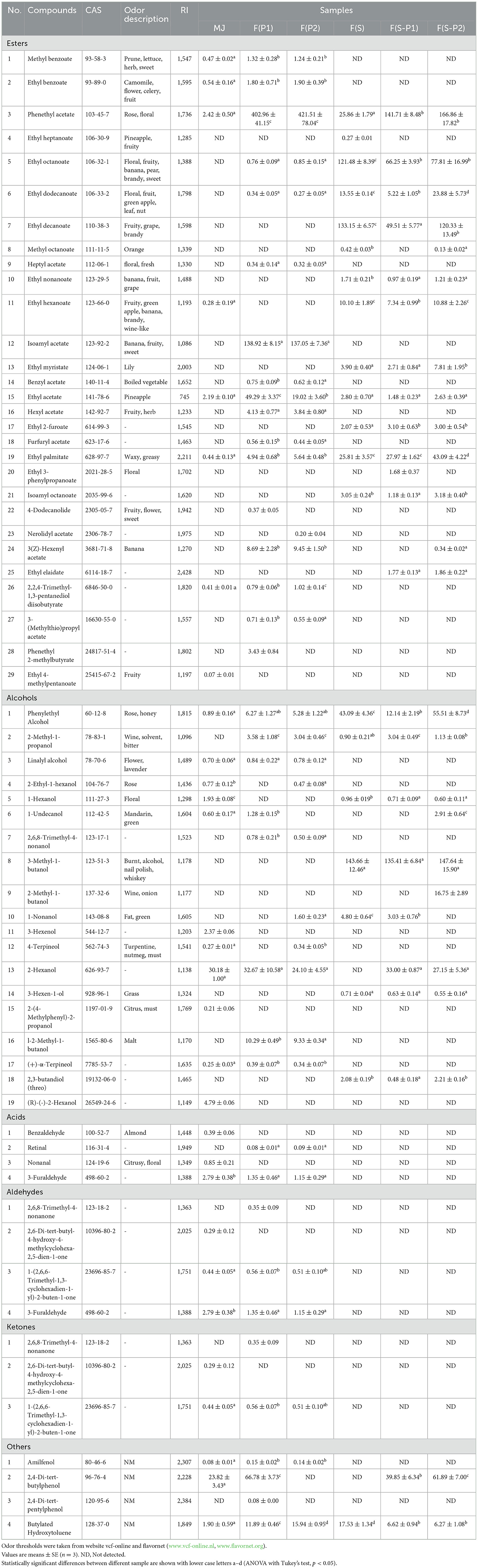
Table 2. Volatile compounds (μg/mL) from mulberry juice and mulberry wine (day 18) fermented with S. cerevisiae and P. kluyveri.
In order to further identify the differences in the volatiles of the different samples, a principal components analysis (PCA) was carried out. As shown in Figure 4B, all samples were clearly separated from each other. In addition, the PCA results also exactly indicated that the fermentation with pure and co-culture of yeast would be distinguished (Figure 4D). In Figure 4D, the F(S) group was divided by the positive PC1 scores, and the F(S-P1) group was divided by the negative PC1 scores. On the other hand, the F(S-P2) could be separated by the scores of PC2. The classification trend of the different fermentation broths was also shown by the PCA results (Figures 4C, D). The PC1 results (81.84%, 44.75%) in Figures 4C, D initially showed the dominance of SC strains in fermentation. In Figure 4C, PK1 and PK2 could be separated by second component (PC2, 14.45 %). When the PK strains were used in a co-fermentation, the F(S-P1) and F(S-P2) could also be well separated by the second component (PC2, 42.46%) and showed significant differences (Figure 4D). This was an indication that the differences between PK1 strain and PK2 strain could be amplified during co-fermentation with SC strain. In other words, the fermentation process was dominated by the SC strain, and PK strains formed the flavor of the different types of mulberry wine.
The samples of mulberry juice (MJ) had a simple volatile composition and low levels of the individual components. This was similar to the insignificant flavor of the mulberry fruit. After fermentation by different strains, seven substances in MJ were not found. These were mainly aldehydes (nonanal and benzaldehyde) and some heterocyclic compounds. This result was in agreement with a previous study (23).
The esters are one of the main aroma components in fermentation, creating the main flavor type of fruit wine (24). The total amount of esters produced in F(P1) and F(P2) was high, and their relative content among all the components was also relatively high (Figure 4A, Table 2). This indicated the excellent ester production performance of the PK1 and PK2 strains, similar to previous reports (25). Phenethyl acetate was the most abundant of all the esters detected, with 402.96 μg/mL and 421.51 μg/mL detected in F(P1) and F(P2), respectively. Similarly, phenethyl acetate was also the ester with the highest content in F(S-P1) and F(S-P2), reaching 141.71 and 166.86 μg/mL, respectively. The content of phenethyl acetate in the F(S) was only 25.86 μg/mL. This suggested that phenethyl acetate was responsible for a “rose” and “floral” odor (24) in co-fermented mulberry wines. In other words, these results indicated that co-fermentation completely changed the flavor type of fruit wine. On the other hand, the content of ethyl decanoate and ethyl octanoate in F(S) reached 133.15 and 121.48 μg/mL, respectively, which was the highest of all groups. This also constructed the “brandy” odor as the main flavor type in F(S). Comparing F(S-P1) and F(S-P2), it was found that there was a significant difference in the content of ethyl palmitate, ethyl myristate, ethyl dodecanoate, isoamyl octanoate, and ethyl decanoate (Figure 4A). This also explained the flavor differences between the co-fermentation groups.
Although alcohols were found in all groups, the types and amounts of alcohols in MJ, F(P1) and F(P2) were on low levels. The alcohol content increased significantly and its type changed after fermentation (SC involved), becoming one of the most important components in the resulting mulberry wines (Figure 4A). It should be noted that 3-Methyl-1-butanol was significantly increased in the F(S) and co-fermentation groups, whereas it was not found in the MJ and non-Saccharomyces fermentation groups F(P1) and F(P2). This indicated that the production of 3-Methyl-1-butanol came mainly from the metabolism of the SC strain. The addition of the PK1 and PK2 strains had no significant effect on it. The presence of 3-Methyl-1-butanol also enhanced the “floral” and “nail polish” flavors (26) of mulberry wine. High concentration of phenylethyl alcohol was found in F(S-P2) from the co-fermentation, followed by that from F(S). This also contributed to the “floral” and “honey” flavors of mulberry wines.
The acids were mostly produced during fermentation (27). The content of octanoic acid (65.98 μg/mL) and decanoic acid (32.16 μg/mL) in F(S-P2) is significantly higher than in F(S) and F(S-P1). However, no acid of any kind was found in the F(P1) and F(P2). This means that the SC strain could produce these two acids, while the PK2 strain helped to increase their production during co-fermentation process. In F(S-P1), on the other hand, octanoic acid had only 16.41 μg/mL and hexanoic acid could not be detected. This suggests that PK1 strain inhibited both acids during co-fermentation. At least the influence of metabolic mechanisms between SC and PK (PK1 and PK2) strains was clearly demonstrated by the conclusion of titratable acidity (TA). In particular, acetic acid was not found in F(S). This indicated that the SC strain was effective in inhibiting acetic acid production.
The aldehydes were not found in either pure cultured F(S) or co-fermented [F(S-P1) and F(S-P2)] mulberry wines containing SC strain. The amount and content of aldehydes in F(P1) and F(P2) were lower than in MJ. This result was in line with the findings of the study by YU et al. (17). Similar results were obtained for ketones. In particular, no ketones were detected in F(S) or in the co-fermentations of F(S-P1) and F(S-P2). Previous studies have shown that it had little effect on the flavor of fruit wine (14).
3.5 Comprehensive metabolic characteristic analyses
Amino acid metabolism in the co-fermentation system showed very active with the addition of the PK strain. The formation of various amino acids was also based on this. The positive effect of amino acid metabolism on fermentation was confirmed (28). The amino acids were essential nutrients for the growth and metabolism of yeast (29). In addition, some of the amino acids could be metabolized by the yeast (both SC and PK strains) to form higher alcohols (30). The PK strain in this study showed excellent growth characteristics during co-fermentation compared to previous studies (18, 25). The PK1 and PK2 strains gradually disappeared after 15 days. This was relatively rare in co-fermentation with SC strains. In general, the growth of yeast has a certain preference in terms of amino acid requirements (29). To maintain strain cell growth, P. kluyveri strains rely on amino acids such as Asp and Phe (31). In this study, we observed a significant decreased concentration of the Asp and Phe (Tab S2, S3). This was an indication that the PK1 and PK2 strains may have effectively utilized these two types of nutrients for growth. From this we concluded that the co-fermentation of P. kluyveri and S. cerevisiae objectively improves biosynthesis of amino acids (Figure 3), which improved the growth status of the P. kluyveri. This could be related to the characteristics of the strains and the fermentation temperature (16°C) we chose, but further genetic evidence was needed.
On the other hand, there was also a high demand for amino acid nutrients for the growth of SC strains. In this study, the SC strain was able to maintain an excellent growing state and dominate the co-fermentation process. Obviously, it also benefits from adequate amino acid nutrition (Figures 2E, F, Supplementary Tables S2, S3). In particular, the SC strains were able to maintain higher vitality in the later stages (12–18 d) of co-fermentation. In addition to the positive effects brought about by an active pathway of biosynthesis of amino acids (Figures 2E, F), the dead PK strains were also able to passively provide more amino acids to the SC by autolysis due to the accumulation of large numbers of PK cells.
Generally, most higher alcohols are derived from the anabolic pathway of sugars (32). The metabolomics results in this study showed that the co-fermentation group had a significantly up-regulated central carbon metabolism compared to the F(S) group (Figures 2E, F). Amino acid metabolism was also found to play an important role in flavoring accumulation (21). As an example, some amino acids can be catabolized through the Ehrlich pathway to the given higher alcohols and subsequently to higher alcohol acetates (32). In line with this, we found that substances such as 2-Hexanol and 2-Methyl-1-butanol were only present in the co-fermentation group. Therefore, there was every reason to believe that PK strains involved in co-fermentation would lead to active central carbon metabolism, inevitably accumulating more acetic acid, acetyl-CoA and higher alcohols, which could form higher alcohol esters.
We found that there was a certain difference in the accumulation of higher alcohols between the co-fermenting group and the pure culture group, but it was not very significant (Figure 4). The corresponding ester substances, however, increased significantly (Figure 4). For example, the content of phenylethyl alcohol in F(S) and F(S-P1) was 43.09 and 12.14 μg/mL, respectively. While phenethyl acetate levels reached 25.86 and 141.71μg/mL, respectively (Table 2). In particular, similar results were also observed in the F(S-P2). There are two main pathways by which yeast can produce phenethyl acetate, according to a previous study (33). The first is the synthesis from acetic acid and phenylethyl alcohol under the promotion of esterase. Secondly, it is synthesized from acetyl-CoA and phenylethyl alcohol under the promotion of alcohol acyltransferase (AAT). We further illustrated that PK strain could strongly promote given amino acid (L-phenylalanine, etc.) induced ethanol dehydrogenase (ADH) for the conversion of ethanol to acetic acid in co-fermentation. At the same time, promoted the formation of phenethyl acetate from phenylethyl alcohol and acetyl-CoA via the AAT pathway. In other words, PK strains could promote the formation of more higher alcohol acetates through the AAT pathway in the co-fermentation process. This is all due to the PK strain promoting amino acid metabolism in the co-fermentation system. Correspondingly, we also found that a certain amount of acetic acid was accumulated in the F(S-P1) and F(S-P2), whereas it was not detected in the F(S) group (Table 2). The accumulation of acetic acid also supports our view to some extent. However, this hypothesis is in need of confirmation by further research on enzyme activity.
4 Conclusion
In summary, this study provides insights into the co-fermentation of S. cerevisiae with different P. kluyveri strains and offers a novel fermentation strategy for expanding the flavor profiles of fruit wines. The PK strains, by modulating amino acid metabolism during co-fermentation, impart unique “floral” and “fruity” aromas to mulberry wine, particularly the “rose-like” flavor contributed by ester compounds. The different PK strains improved the levels of aroma categories and types in the co-fermented mulberry wine to varying degrees. Investigating the effect of PK strains on the metabolic progression during co-fermentation will further enable the relatively precise prediction and control of aromatic profiles in fruit wines for practical production applications.
Data availability statement
The original contributions presented in the study are included in the article/Supplementary material, further inquiries can be directed to the corresponding authors.
Author contributions
BD: Data curation, Formal analysis, Investigation, Methodology, Validation, Visualization, Writing – original draft, Writing – review & editing. LX: Writing – review & editing, Data curation, Investigation. SZ: Investigation, Writing – review & editing. YL: Writing – review & editing. PG: Writing – review & editing, Formal analysis. WZ: Conceptualization, Funding acquisition, Investigation, Project administration, Writing – review & editing.
Funding
The author(s) declare financial support was received for the research, authorship, and/or publication of this article. This research was funded by the Sichuan Provincial Science and Technology Plan Project (2021YFN0012), Science and Technology Department of Sichuan Province.
Conflict of interest
The authors declare that the research was conducted in the absence of any commercial or financial relationships that could be construed as a potential conflict of interest.
Generative AI statement
The author(s) declare that no Gen AI was used in the creation of this manuscript.
Publisher's note
All claims expressed in this article are solely those of the authors and do not necessarily represent those of their affiliated organizations, or those of the publisher, the editors and the reviewers. Any product that may be evaluated in this article, or claim that may be made by its manufacturer, is not guaranteed or endorsed by the publisher.
Supplementary material
The Supplementary Material for this article can be found online at: https://www.frontiersin.org/articles/10.3389/fnut.2025.1559599/full#supplementary-material
References
1. Lu YY, Chan LJ, Li X, Liu SQ. Effects of different inoculation strategies of Saccharomyces cerevisiae and Williopsis saturnus on chemical components of mango wine. LWT—Food Sci Technol. (2018) 87:85–92. doi: 10.1016/j.lwt.2017.08.074
2. Dellacassa E, Trenchs O, Fariña L, Debernardis F, Perez G, Boido E, et al. Pineapple (Ananas comosus L. Merr) wine production in Angola: characterisation of volatile aroma compounds and yeast native flora. Int J Food Microbiol. (2017) 241:161–7. doi: 10.1016/j.ijfoodmicro.2016.10.014
3. Ercisli S, Orhan E. Chemical composition of white (Morus alba), red (Morus rubra) and black (Morus nigra) mulberry fruits. Food Chem. (2007) 103:1380–4. doi: 10.1016/j.foodchem.2006.10.054
4. Yuan QX, Zhao LY. The Mulberry (Morus alba L) fruit-A review of characteristic components and health benefits. J Agric Food Chem. (2017) 65:10383–94. doi: 10.1021/acs.jafc.7b03614
5. Tchabo W, Ma YK, Kwaw E, Zhang HN, Xiao LL, Tahir HE. Aroma profile and sensory characteristics of a sulfur dioxide-free mulberry (Morus nigra) wine subjected to non-thermal accelerating aging techniques. Food Chem. (2017) 232:89–97. doi: 10.1016/j.foodchem.2017.03.160
6. Rêgo ESB, Rosa CA, Freire AL, Machado AMD, Gomes FDO, da Costa ASP, et al. Cashew wine and volatile compounds produced during fermentation by non-Saccharomyces and Saccharomyces yeast. LWT—Food Sci Technol. (2020) 126:109291. doi: 10.1016/j.lwt.2020.109291
7. Liu SX, Laaksonen O, Kortesniemi M, Kalpio M, Yang BR. Chemical composition of bilberry wine fermented with non-Saccharomyces yeasts (Torulaspora delbrueckii and Schizosaccharomyces pombe) and Saccharomyces cerevisiae in pure, sequential and mixed fermentations. Food Chem. (2018) 266:262–74. doi: 10.1016/j.foodchem.2018.06.003
8. Shi WK, Wang J, Chen FS, Zhang XY. Effect of Issatchenkia terricola and Pichia kudriavzevii on wine flavor and quality through simultaneous and sequential co-fermentation with Saccharomyces cerevisiae. LWT—Food Sci Technol. (2019) 116:108477. doi: 10.1016/j.lwt.2019.108477
9. Wei JP, Wang SY, Zhang YX, Yuan YH, Yue TL. Characterization and screening of non-Saccharomyces yeasts used to produce fragrant cider. LWT—Food Sci Technol. (2019) 107:191–8. doi: 10.1016/j.lwt.2019.03.028
10. Lorenzini M, Simonato B, Slaghenaufi D, Ugliano M, Zapparoli G. Assessment of yeasts for apple juice fermentation and production of cider volatile compounds. LWT—Food Sci Technol. (2019) 99:224–30. doi: 10.1016/j.lwt.2018.09.075
11. Lemos WJF, Binati RL, Felis GE, Slaghenaufi D, Ugliano M, Torriani S. Volatile organic compounds from Starmerella bacillaris to control gray mold on apples and modulate cider aroma profile. Food Microbiol. (2020) 89:103446. doi: 10.1016/j.fm.2020.103446
12. Clemente-Jimenez JM, Mingorance-Cazorla L, Martínez-Rodriguez S, Heras-Vázquez FJL, Rodríguez-Vico F. Influence of sequential yeast mixtures on wine fermentation. Int J Food Microbiol. (2005) 98:301–8. doi: 10.1016/j.ijfoodmicro.2004.06.007
13. Ding B, Zhao ST, Zhang WX, Lin Y, Xiong L. The effect of co-culture with different Pichia kluyveri and Saccharomyces cerevisiae on volatile compound and characteristic fingerprints of mulberry wine. Foods. (2024) 13:422. doi: 10.3390/foods13030422
14. Wei JP, Zhang YX, Wang YW, Ju HM, Niu C, Song ZH, et al. Assessment of chemical composition and sensorial properties of ciders fermented with different non-Saccharomyces yeasts in pure and mixed fermentations. Int J Food Microbiol. (2020) 318:108471. doi: 10.1016/j.ijfoodmicro.2019.108471
15. Ye MQ, Yue TL, Yuan YH. Effects of sequential mixed cultures of Wickerhamomyces anomalus and Saccharomyces cerevisiae on apple cider fermentation. FEMS Yeast Res. (2014) 14:873–82. doi: 10.1111/1567-1364.12175
16. Want EJ, Masson P, Michopoulos F, Wilson ID, Theodoridis G, Plumb RS, et al. Global metabolic profiling of animal and human tissues via UPLC-MS. Nat Protoc. (2013) 8:17–32. doi: 10.1038/nprot.2012.135
17. Yu WY, Zhu YY, Zhu RX, Bai JR, Qiu JH, Wu YP, et al. Insight into the characteristics of cider fermented by single and co-culture with Saccharomyces cerevisiae and Schizosaccharomyces pombe based on metabolomic and transcriptomic approaches. LWT—Food Sci Technol. (2022) 163:113538. doi: 10.1016/j.lwt.2022.113538
18. Anfang N, Brajkovich M, Goddard MR. Co-fermentation with Pichia kluyveri increases varietal thiol concentrations in sauvignon blanc. Aust J Grape Wine Res. (2009) 15:1–8. doi: 10.1111/j.1755-0238.2008.00031.x
19. Cappello MS, Zapparoli G, Logrieco A, Bartowsky EJ. Linking wine lactic acid bacteria diversity with wine aroma and flavour. Int J Food Microbiol. (2017) 243:16–27. doi: 10.1016/j.ijfoodmicro.2016.11.025
20. Bordet F, Romanet R, Bahut F, Ferreira V, Peña C, Julien-Ortiz A, et al. Impact of Saccharomyces cerevisiae yeast inoculation mode on wine composition. Food Chem. (2024) 441:138391. doi: 10.1016/j.foodchem.2024.138391
21. Fairbairn S, McKinnon A, Musarurwa HT, Ferreira AC, Bauer FF. The impact of single amino acids on growth and volatile aroma production by Saccharomyces cerevisiae strains. Front Microbiol. (2018) 8:2554. doi: 10.3389/fmicb.2017.02554
22. Liu XF Li NY, Zhao XY, Zhang Y, Muhammad H, Zhong H, et al. Sensory and chemical characterizations of aroma during the loquat wine fermentation. Food Biosci. (2024) 58:103731. doi: 10.1016/j.fbio.2024.103731
23. Liu SX, Laaksonen O, Yang BR. Volatile composition of bilberry wines fermented with non-Saccharomyces and Saccharomyces yeasts in pure, sequential and simultaneous inoculations. Food Microbiol. (2019) 80:25–39. doi: 10.1016/j.fm.2018.12.015
24. Domizio P, Romani C, Lencioni L, Comitini F, Gobbi M, Mannazzu I, et al. Outlining a future for non-Saccharomyces yeasts: Selection of putative spoilage wine strains to be used in association with Saccharomyces cerevisiae for grape juice fermentation. Int J Food Microbiol. (2011) 147:170–80. doi: 10.1016/j.ijfoodmicro.2011.03.020
25. Kurita O. Increase of acetate ester-hydrolysing esterase activity in mixed cultures of Saccharomyces cerevisiae and Pichia anomala. J Appl Microbiol. (2008) 104:1051–8. doi: 10.1111/j.1365-2672.2007.03625.x
26. Wei JP, Zhang YX, Qiu Y, Guo H, Ju HM, Wang YW, et al. Chemical composition, sensorial properties, and aroma-active compounds of ciders fermented with Hanseniaspora osmophila and Torulaspora quercuum in co- and sequential fermentations. Food Chem. (2020) 306:125623. doi: 10.1016/j.foodchem.2019.125623
27. Qin ZH, Petersen MA, Bredie WLP. Flavor profiling of apple ciders from the UK and Scandinavian region. Food Res Int. (2018) 105:713–23. doi: 10.1016/j.foodres.2017.12.003
28. Kevvai K, Kütt ML, Nisamedtinov I, Paalme T. Simultaneous utilization of ammonia, free amino acids and peptides during fermentative growth of Saccharomyces cerevisiae. J Inst Brew. (2016) 122:110–5. doi: 10.1002/jib.298
29. Su Y, Seguinot P, Sanchez I, Ortiz-Julien A, Heras JM, Querol A, et al. Nitrogen sources preferences of non-Saccharomyces yeasts to sustain growth and fermentation under winemaking conditions. Food Microbiol. (2020) 85:103287. doi: 10.1016/j.fm.2019.103287
30. Rollero S, Bloem A, Ortiz-Julien A, Camarasa C, Divol B. Fermentation performances and aroma production of non-conventional wine yeasts are influenced by nitrogen preferences. FEMS Yeast Res. (2018) 18:foy055. doi: 10.1093/femsyr/foy055
31. Li YQ, Xu LB, Sam FE, Li AH, Hu K, Tao YS, et al. Improving aromatic higher alcohol acetates in wines by co-fermentation of Pichia kluyveri and Saccharomyces cerevisiae: growth interaction and amino acid competition. J Sci Food Agric. (2024) 104:6875–83. doi: 10.1002/jsfa.13519
32. Dzialo MC, Park R, Steensels J, Lievens B, Verstrepen KJ. Physiology, ecology and industrial applications of aroma formation in yeast. FEMS Microbiol Rev. (2017) 41:S95–S128. doi: 10.1093/femsre/fux031
Keywords: mulberry wine, Saccharomyces cerevisiae, Pichia kluyveri, metabolic characteristics, co-fermentation
Citation: Ding B, Xiong L, Zhao S, Lin Y, Guo P and Zhang W (2025) Impact of co-fermentation of Saccharomyces cerevisiae and Pichia kluyveri on the metabolic characteristics of the flavor compounds in mulberry wine. Front. Nutr. 12:1559599. doi: 10.3389/fnut.2025.1559599
Received: 13 January 2025; Accepted: 11 February 2025;
Published: 25 February 2025.
Edited by:
Jiajia Song, Southwest University, ChinaReviewed by:
Jing Yang, Chongqing Technology and Business University, ChinaRan Wang, China Agricultural University, China
Junhua Jin, Beijing University of Agriculture, China
Sławomir Jabłoński, University of Wrocław, Poland
Copyright © 2025 Ding, Xiong, Zhao, Lin, Guo and Zhang. This is an open-access article distributed under the terms of the Creative Commons Attribution License (CC BY). The use, distribution or reproduction in other forums is permitted, provided the original author(s) and the copyright owner(s) are credited and that the original publication in this journal is cited, in accordance with accepted academic practice. No use, distribution or reproduction is permitted which does not comply with these terms.
*Correspondence: Penghui Guo, eGJtdXNreUAxNjMuY29t; Wenxue Zhang, emhhbmd3ZW54dWVAc2N1LmVkdS5jbg==