- 1Research Service, Department of Veterans Affairs, Nebraska-Western Iowa Health Care System, Omaha, NE, United States
- 2Department of Internal Medicine, University of Nebraska Medical Center, Omaha, NE, United States
- 3Cancer Biology Lab, Centre for Molecular and Nanomedical Sciences, Sathyabama Institute of Science and Technology, Chennai, Tamil Nadu, India
- 4Department of Pharmacology and Experimental Neuroscience, University of Nebraska Medical Center, Omaha, NE, United States
- 5Department of Biochemistry & Molecular Biology, University of Nebraska Medical Center, Omaha, NE, United States
Chronic liver disease is defined by persistent harm to the liver that might result in decreased liver function. The two prevalent chronic liver diseases are alcohol-associated liver disease (ALD) and metabolic dysfunction-associated steatotic liver disease (MASLD). There is ample evidence that the pathogenesis of these two chronic liver diseases is closely linked to gastrointestinal dysfunctions that alters the gut-liver crosstalk. These alterations are mediated through the imbalances in the gut microbiota composition/function that combined with disruption in the gut barrier integrity allows for harmful gut microbes and their toxins to enter the portal circulation and reach the liver to elicit an inflammatory response. This leads to further recruitment of systemic inflammatory cells, such as neutrophils, T-cells, and monocytes into the liver, which perpetuate additional inflammation and the development of progressive liver damage. Many therapeutic modalities, currently used to prevent, attenuate, or treat chronic liver diseases are aimed at modulating gut dysbiosis and improving intestinal barrier function. Betaine is a choline-derived metabolite and a methyl group donor with antioxidant, anti-inflammatory and osmoprotectant properties. Studies have shown that low betaine levels are associated with higher levels of organ damage. There have been several publications demonstrating the role of betaine supplementation in preventing the development of ALD and MASLD. This review explores the protective effects of betaine through its role as a methyl donor and its capacity to regulate the protective gut microbiota and maintain intestinal barrier integrity to prevent the development of these chronic liver diseases. Further studies are needed to enhance our understanding of its therapeutic potential that could pave the way for targeted interventions in the management of not only chronic liver diseases, but other inflammatory bowel diseases or systemic inflammatory conditions.
Introduction
A variety of illnesses are grouped together under the umbrella term “chronic liver disease,” which is defined by persistent harm to the liver that might result in decreased liver function (1–3). Common causes for liver diseases are hepatitis B and C infections, autoimmune illnesses, hereditary factors and Western lifestyle, which includes dietary changes, inactivity, and alcohol intake (4–8). Hippocrates, the father of modern medicine claimed ~2,500 years ago that “all diseases begin in the gut.” There is now ample evidence that indeed gut dysfunction is involved in the pathogenesis of many diseases especially those of the liver (9–13). This is because the gut and liver are anatomically linked via the portal system (14). Normally, the gut epithelial cells maintain barrier integrity through microvilli, tight junctions, and production of antimicrobial peptides (15, 16). These gastrointestinal epithelial cell boundary frameworks prevent the translocation of most of gut luminal content and in healthy conditions only small number of microbial products can reach the liver (17). In this mutual relationship, the liver acts as a second firewall toward the low number of potentially harmful substances translocated from the gut that are eliminated by the liver resident macrophages, Kupffer cells (14). However, alterations in gut homeostasis characterized by changes in the composition of the gut microbiota, microbial products, antimicrobial peptide levels and mucosal barrier disruption play a major role in the onset and progression of liver diseases including two prevalent diseases, alcohol-associated liver disease (ALD) and metabolic dysfunction-associated steatotic liver disease (MASLD) (9–11, 13, 18, 19).
Betaine, a naturally occurring derivative of choline, plays an important role in various cellular processes due to its function as a methyl donor in the methionine-homocysteine cycle (20). Studies show that the role of betaine in remethylating homocysteine to methionine through the betaine-homocysteine methyltransferase (BHMT)-catalyzed reaction helps reduce homocysteine and S-adenosylhomocysteine (SAH) levels (21–23), factors linked to adipose, gut and liver dysfunction (24–28). In the context of liver diseases, betaine has been shown to mitigate lipid accumulation, oxidative stress, proteasomal/lysosomal dysfunction, hepatocyte death, and inflammation, making it a promising therapeutic agent for ALD (24, 29, 30) and MASLD (31, 32). Additionally, betaine is significant for gut health as it stabilizes intestinal barrier integrity, regulates osmolality, and modulates the gut microbiota (33, 34). In addition, betaine increases the villus height and crypt depth and by maintaining the tight junctional protein complexes prevents luminal antigens/microbial translocation from the gut to the liver (35). These effects on the gut-liver axis highlight its therapeutic potential in preventing liver inflammation and progressive injury. Hence, in this review, we highlight the protective role of betaine on the gut-liver axis and its relevance in managing liver diseases.
Alcohol-associated liver disease (ALD)
Alcohol-associated liver disease is liver damage that arises from excessive consumption of beverages that contain ethyl alcohol (ethanol) (36–38). As the principal site of alcohol absorption, the gastrointestinal tract plays an important role in regulating the systemic availability of ethanol (39, 40). While ethanol metabolism in the gastrointestinal tract locally generates low levels of acetaldehyde and other toxic metabolites, the liver is the primary organ that carries out ethanol metabolism and consequently sustains the greatest organ damage from heavy drinking (41, 42). The hepatic metabolism of ethanol occurs through both oxidative and non-oxidative pathways. Oxidative ethanol metabolism is catalyzed by alcohol dehydrogenase (ADH) which converts ethanol to acetaldehyde, which is subsequently oxidized to acetate (43, 44). In addition, another major ethanol-metabolizing enzyme is cytochrome P450 2E1 (CYP2E1), a microsomal enzyme that is significantly induced by ethanol consumption (45).
Alcohol-associated liver disease encompasses a broad spectrum of pathologies, including steatosis (fatty liver), which can progress to steatohepatitis [steatosis with inflammation and necrosis and fibrosis (Figure 1)]. If harmful drinking continues, liver cirrhosis can develop, during which hepatocytes dedifferentiate, lose their phenotype, and can become neoplastic, resulting in hepatocellular carcinoma (46–48). ALD progression from steatosis to more severe liver damage is associated with the amount, type, pattern, and duration of alcohol consumption (45). Other factors involved in the pathogenesis and progression of ALD include detrimental changes in gut microbiota and intestinal barrier disruption (45, 49–51). This translocation of gut luminal pathobionts and products triggers inflammatory responses in the liver, involving resident hepatic macrophages, Kupffer cells, and other infiltrating immune cells that activates hepatic stellate cells (HSC), causing aberrant production of extracellular matrix (ECM) proteins and fibrosis development. Furthermore, due to aberrant ECM production by activated HSCs, liver sinusoidal endothelial cells undergo capillarization, including the loss of fenestrations and a shift toward a vascular phenotype. These changes collectively contribute to the development of advanced alcohol-induced liver injury (52, 53).
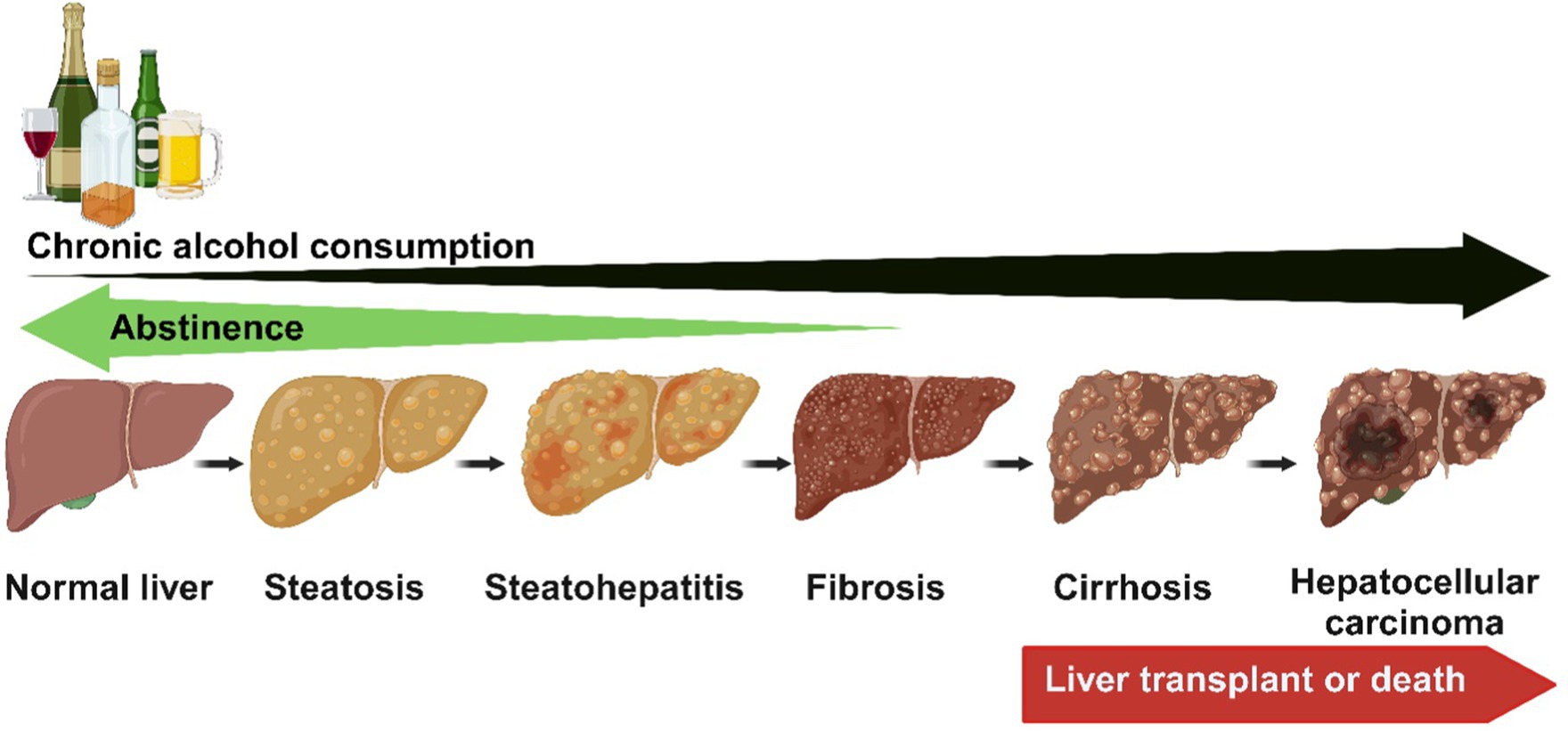
Figure 1. The spectrum of ALD encompasses a broad spectrum of pathologies, including steatosis (fatty liver), which can progress to steatohepatitis (steatosis with inflammation and necrosis) and fibrosis. If harmful drinking continues, liver cirrhosis can develop, during which hepatocytes dedifferentiate, lose their phenotype, and can become neoplastic, resulting in hepatocellular carcinoma. The early stages of ALD reverse with abstinence. Created with biorender.com.
Metabolic dysfunction-associated steatotic liver disease (MASLD)
Metabolic dysfunction-associated steatotic liver disease is an important hepatic manifestation of metabolic syndrome (54) characterized by fat accumulation in more than 5% of hepatocytes (55). MASLD is the most widespread chronic liver disease, impacting around one-quarter of the global population due to the growing rates of metabolic syndrome, obesity, and diabetes (56, 57). Patients with MASLD typically follow an unhealthy dietary pattern marked by elevated intake of saturated fats, cholesterol, and fructose and low physical activity (54). Conversely, there is a lower consumption of antioxidant vitamins and polyunsaturated fats (58). MASLD displays a similar spectrum of progression as ALD ranging from steatosis through steatohepatitis, hepatitis, cirrhosis, and to hepatocellular carcinoma (54, 59–61). The early stages of MASLD, as ALD, are marked by steatosis that progresses to metabolic dysfunction-associated steatohepatitis (MASH) distinguished by hepatocellular damage that includes hepatocyte ballooning degeneration, diffuse lobular inflammation, and fibrosis (62). MASH is increasingly being recognized as the leading indication for liver transplant listing for women and is expected to overtake ALD as the leading liver transplant indication for all patients within the next few years (60, 63).
The underlying mechanism for the initiation and progression of MASLD is complex and multifactorial. Different theories have been postulated leading initially to the ‘two-hit’ hypothesis (64). According to this, the ‘first hit’ is induced by the accumulation of lipids in hepatocytes from high-caloric intake, obesity, insulin resistance, which makes the liver vulnerable to other damaging factors that are referred to as the ‘second hits’, such as oxidative stress, genetic polymorphisms (65). These second hits increase the vulnerability to the disease, activation of inflammatory pathways triggered by the release of pro-inflammatory cytokines by Kupffer cells or from adipocytes, dysregulated hepatocyte apoptosis, and activation of HSC, among others (66). When acting individually, these factors drive the progression from macrovesicular steatosis to MASH, which involves a gradual increase in liver injury parameters in association with hepatocyte apoptosis, inflammation, and fibrogenesis. Fibrosis advances from periportal to bridging fibrosis, eventually leading to cirrhotic remodeling, liver failure, and can ultimately result in hepatocellular carcinoma (67). The simplistic and outdated ‘two-hit’ hypothesis has been replaced by a “multi-hit’ model that integrates various interconnected processes, as shown in Figure 2, where multiple factors act synergistically, encompassing lipotoxicity, gut dysfunction, altered gut-liver axis and innate immune activation, within the context of genetic and environmental factors (68).
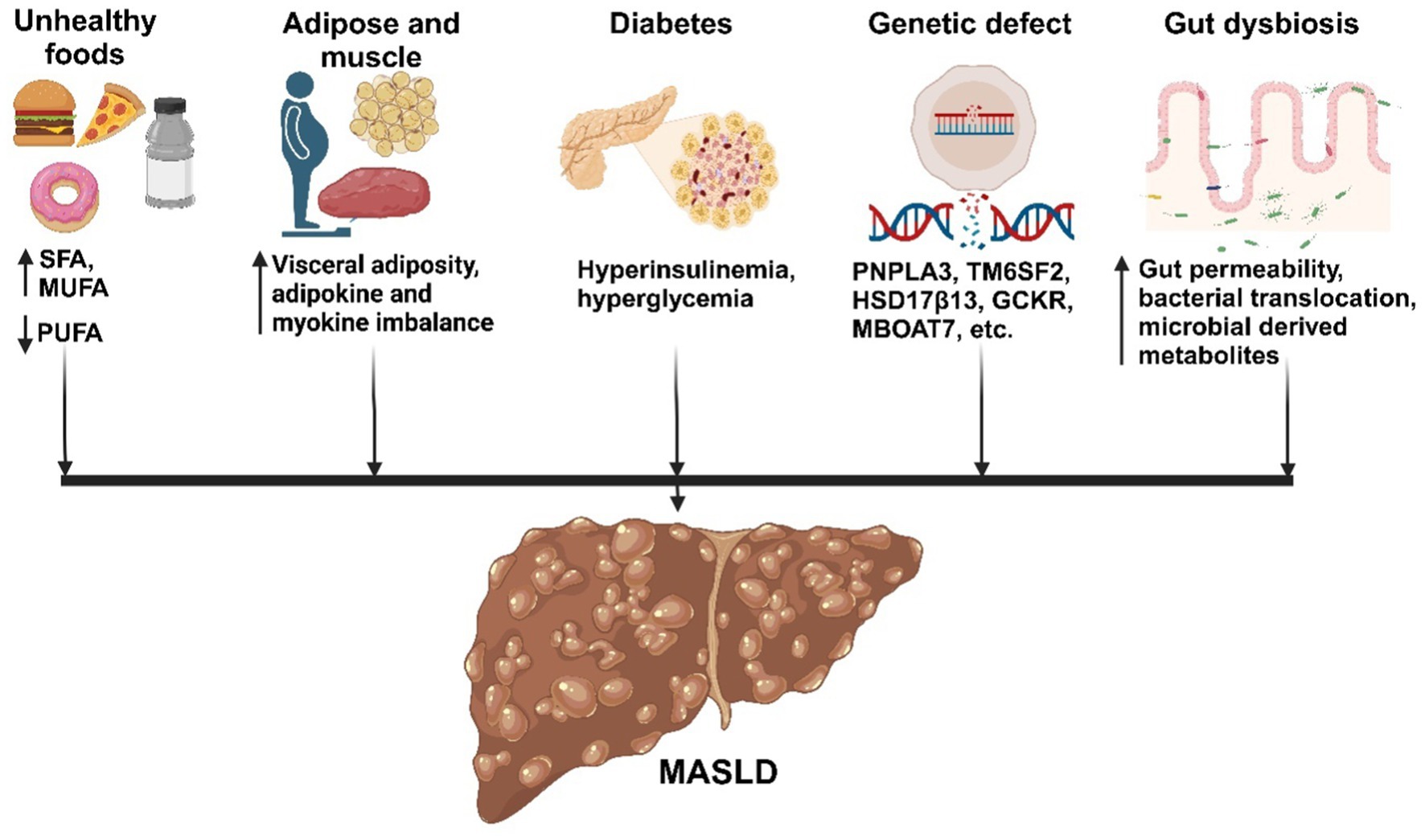
Figure 2. Factors influencing the development of MASLD. Metabolic factors promote the accumulation of fat in the liver, leading to inflammation, cell death, and fibrosis. Adipose tissue and muscle produce adipokines and myokines, respectively, which promote inflammation and oxidative stress in the liver. Additionally, the gut microbiota influences fat accumulation and inflammation in the liver, contributing to MASLD development. Created with biorender.com.
Liver-gut axis
A continuous “dialogue” exists between the gut and liver, heavily influenced by the trillions of microbes residing within, the food we consume, and the world around us. This multifaceted communication has been named the gut-liver axis (69). In both the gut and liver, a “symphony of nutrients, microbial antigens, metabolites, and bile acids” conducts the “orchestra” of metabolism and immune responses. This intricate “dance,” in turn, shapes the composition and function of the gut microbiome. Gut microbes aren’t just passive residents; they are active players influencing our health throughout the body. Generally, gut and liver are in constant “conversation” for the digestion, nutrient processing, and even filtering out microbial leftovers. However, in experimental models of liver disease, this harmonious interplay is disrupted. A weakened intestinal barrier or a “leaky gut” allows for the melody to go off-key, that allows harmful gut microbes and their toxins to enter the portal circulation and reach the liver to elicit an inflammatory response by the Kupffer cells (70, 71). This local hepatic inflammation leads to further recruitment of systemic inflammatory cells, such as neutrophils, T-cells and monocytes, which perpetuate additional inflammation, hepatic fibrosis, hepatocyte cell death (68, 72, 73) that ultimately leads to rapid progression to multiple organ failure (74).
Indeed, alterations in the gut-liver axis characterized by increased portal and circulating levels of gut microbes/microbial components from compromised gut barrier and microbiota changes has emerged as central mediators in promoting ALD and MASLD progression (50, 51, 75–78).
The gut barrier
Three main defense mechanisms make up the intestinal barrier function: (1) The immune barrier, which is made up of gut associated lymphoid tissue (GALT), effector and regulatory T cells, IgA-producing B cells, group 3 innate lymphoid cells, resident macrophages, and dendritic cells in the lamina propria; (2) the biological barrier, which is made up of normal intestinal flora, is responsible for colonization resistance; and (3) the mechanical barrier, which consists of intestinal epithelial cells that line the lumen (79, 80). This physical barrier is finely regulated by large molecular complexes which link intestinal epithelial cells to each other and seal the intercellular spaces on the luminal surface (81). These intercellular complexes regulate passage of molecules through the paracellular spaces (82) that simultaneously performs two opposing tasks. It selectively permits the passage of necessary nutrients from the intestinal lumen into the bloodstream and the internal milieu at large; on the other hand, it prevents the entry of hazardous substances such as microbes, the luminal antigens and proinflammatory factors (83). The most apical part of the junctional complexes are tight junctions (TJs), which are highly specialized and dynamic supramolecular entities composed of several transmembrane proteins (such as the claudin family, junctional adhesion molecule (JAM)-A, occludin, tricellulin) and the cytoplasmic plaques comprised of scaffolding proteins [zonulae occludens (ZO-1, ZO-2, ZO-3, cingulin)] and effector proteins (84–87) that provide a physical link to the cytoskeleton. There are also biochemical components, including enzymes and antimicrobial proteins that regulate the survival and proliferation of gut microbes (88, 89).
Gut microbiota
The gastrointestinal tract harbors a complex and dynamic population of microorganisms, known as the gut microbiota. The gut microbiome encompasses a complete array of microorganisms including bacteria, viruses, fungi, and protozoa (90). It is an intricate ecosystem consisting of 10–100 trillion microorganisms, with gut bacteria being the most well-studied component (88). The major gut bacterial species belong to the phyla Firmicutes and Bacteroidetes (Figure 3), while smaller proportions belong to Actinobacteria, Fusobacteria, Proteobacteria, and Verrucomicrobia (91).
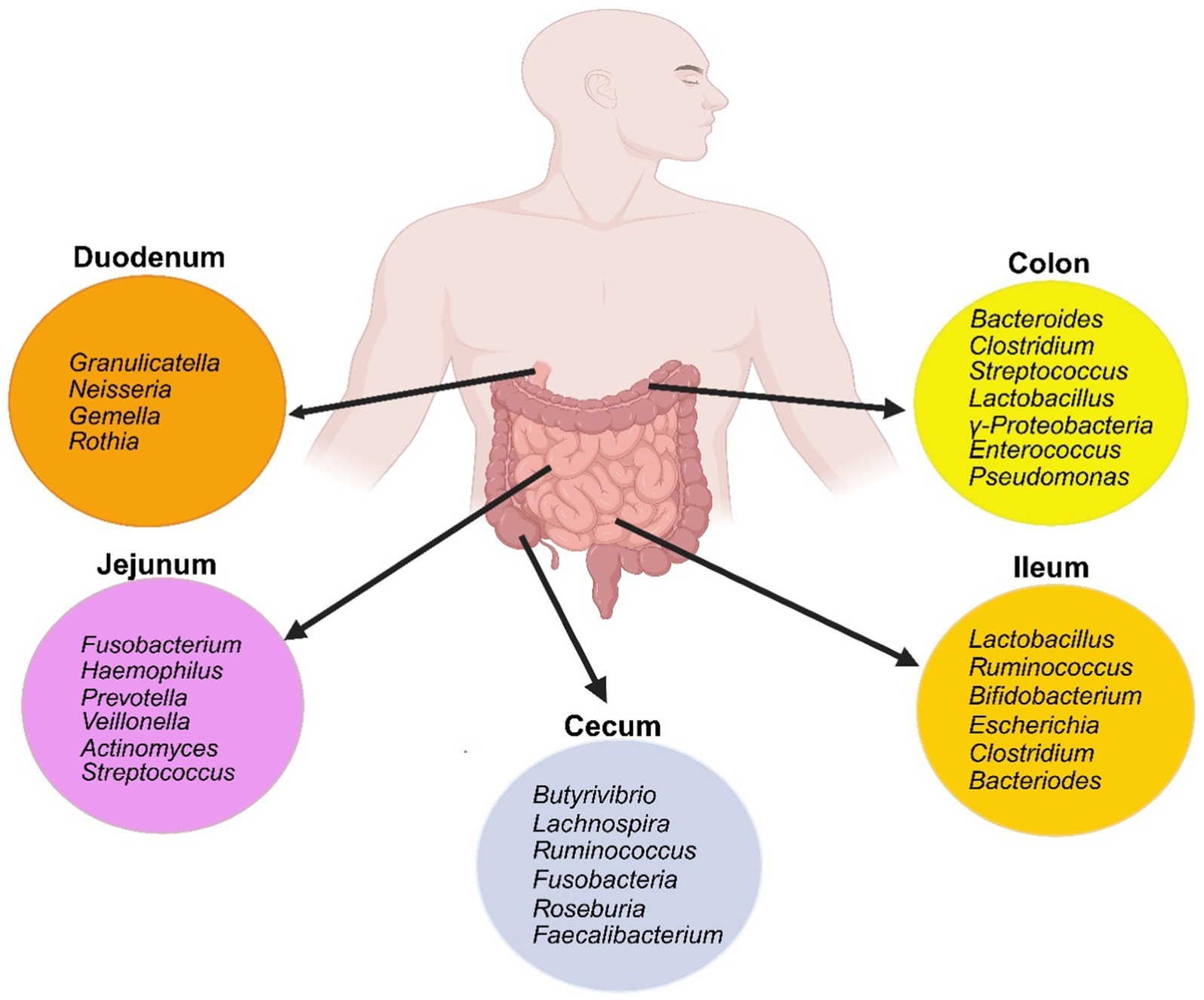
Figure 3. Schematic of the gut bacterial composition along the different segments of the small and large intestine. Created with biorender.com.
These microorganisms contribute to various physiological functions, such as strengthening gut integrity, shaping the intestinal epithelium, harvesting energy, protecting against pathogens, and regulating host immunity (92). The microbiome has significantly enriched metabolism of glycans, amino acids, xenobiotics, methanogenesis and 2-methyl-d-erythritol 4-phosphate pathway-mediated biosynthesis of vitamins and isoprenoids (88, 93). The concentration and makeup of bacterial species vary throughout the entire gastrointestinal tract. These differences can vary between individuals due to age, ethnicity, lifestyle, medications, and dietary patterns indicating that the composition of the microbiota is shaped by both host and environmental selection pressures. The complexity of this symbiotic relationship and its implications for overall health are highlighted by the fact that the survival and proliferation of gut microbes are ultimately determined by their phenotypic features (94, 95). The intricate intestinal barrier comprised of physical, biochemical, and immunological elements acts as a shield, preventing the host immune system from being exposed to the microbiota while still permitting vital interactions to take place (96).
In summary, the intricate interplay between the gut microbiota and the host’s gastrointestinal tract is essential for maintaining overall health and homeostasis as shown in Figure 4.
Alterations in gut homeostasis and liver disease pathogenesis
Several patient and animal studies have established that TJ disassembly occurs from altered expression of claudins, occludin and ZO1 (Figure 5), which promotes the pathogenesis of several diseases including ALD and MASLD (96–109).
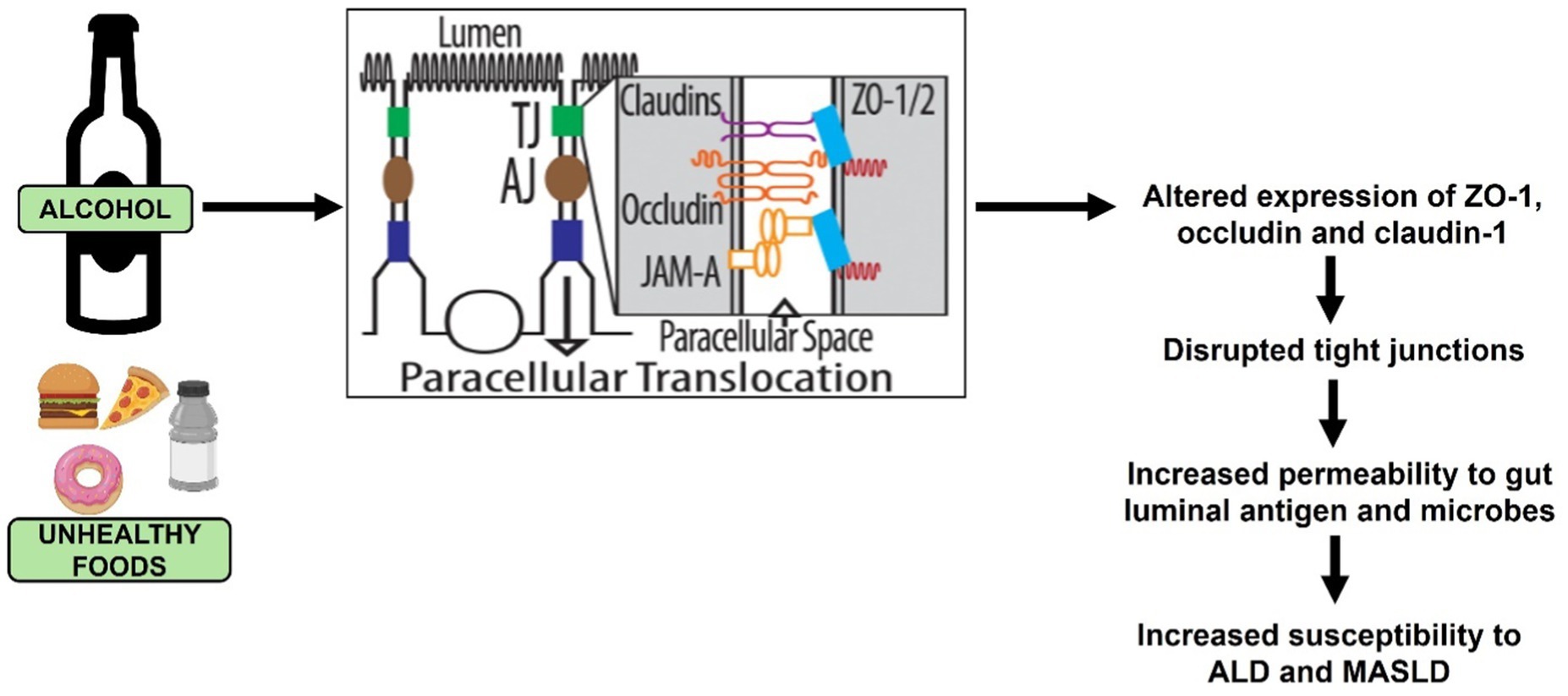
Figure 5. Ethanol and unhealthy food intake-induced tight junction (TJ) disruption that promotes the development of ALD and MASLD.
In the context of ALD, ethanol and its primary metabolite, acetaldehyde, induce redistribution of TJ proteins to disrupt apical junctional complexes, facilitating increased intestinal permeability (34, 110–115). Studies have addressed the mechanisms by which ethanol and acetaldehyde disrupt these junctional complexes, but no unifying theory has yet been presented. It is clear, however, that alcohol/acetaldehyde alters post-translational modifications on the protein components of TJ that disrupt the formation and function of these apical junctional complexes (116–118). Another compelling evidence indicates that alcohol-induced intestinal TJ disruption is caused through elevations in intracellular levels of SAH, a detrimental metabolite of the methionine metabolic pathway (34). The importance of maintaining the barrier integrity is reinforced by reports that successful therapeutic interventions are associated with restoration of normal TJ protein expression (119). A recent study compared gut barrier dysfunction in patients with MASLD and ALD and reported more abnormalities in early stage of MASLD than in ALD (120).
Alcohol consumption stands as the primary cause of liver disease and influences the quantity and makeup of gut microbiota (121, 122). These imbalances or alterations in the gut microbiota composition and function are generally referred to as ‘dysbiosis’ (123). Alcohol intake, a Western diet high in animal fats and sugars, as well as factors such as bowel movement frequency, genetic predisposition, and disturbances in circadian rhythm, can increase the likelihood of dysbiosis (124). Ethanol acts as a modulator of the gastric microenvironment (Figure 6) through complex mechanisms, including altering gastric juice output, impairing gastric motility, damaging gut mucosa, weakening gastric barrier and disrupting mucosal defense (44, 125–127). Ethanol exposure also promotes the release of inflammatory and vasoactive substances, which can cause ischemia resulting in further mucosal damage (126). Alcohol by significantly changing the gastric microenvironment affects the composition of not only the gastric microbiota but also the microbes in the lower segments of the gastrointestinal tract. Interestingly, moderate alcohol use, particularly wine or beer, is linked to a lower risk of Helicobacter pylori infection (128). Conversely, alcohol consumption is associated with an increased risk of gastric cancer, especially in heavy drinkers (129). Alcohol misuse leads to bacterial overgrowth primarily in the upper small bowel, as reported in both preclinical models and human studies (130). This overgrowth results in qualitative changes in the intestinal microflora, dysbiosis in the cecum, and suppression of bactericidal proteins in the small intestine. The changes in alcohol-induced gut composition shows a decrease in beneficial bacteria and an increase in harmful bacteria. As summarized recently, alcohol increases the relative abundance of Proteobacteria, Fusobacteria, Clostridium, and Lactococcus and Enterobacteriaceae and decreases in that of Firmicutes and Bacteroidetes (131). Importantly, administrations of probiotics and their culture supernatants suppresses alcohol-induced increased intestinal permeability and endotoxemia in mice with ALD (132–134). In humans, the severity of ALD is linked to a specific microbiota signature, since fecal microbiota transplantation from patients with severe alcohol-associated hepatitis promotes liver inflammation in germ-free mice (135). Conversely, transplanting intestinal flora of a healthy donor to steroid-ineligible patients with hepatitis improved gut dysbiosis and clinical outcomes (136).
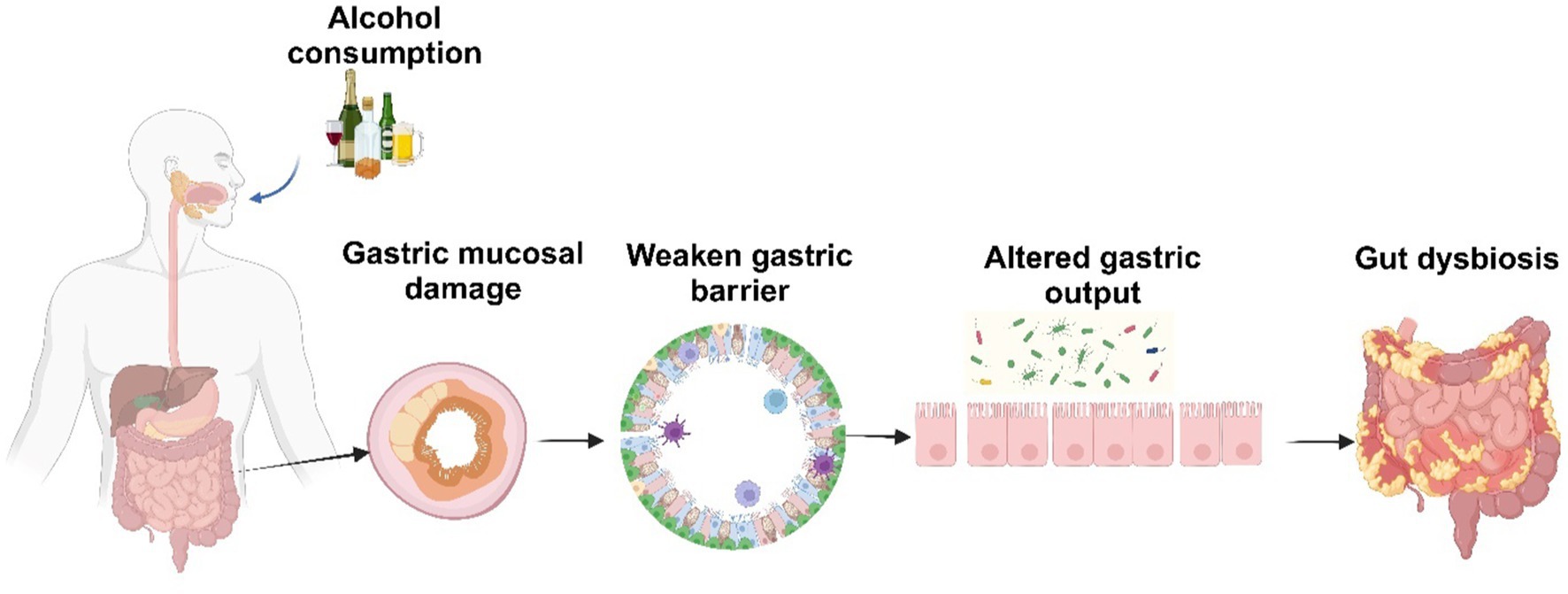
Figure 6. Alcohol-induced alterations in the gastric environment. Created with biorender.com.
Furthermore, different drinking patterns, dose or experimental subjects may show varying effects and even completely different changes in the gut microbiota (93, 130, 137–144). Interestingly, alcohol consumption induces distinct changes in the gut composition that varies with short-term low-dose versus high-dose intake. In addition, while the acute alcohol-induced changes to the gut microbiota are found to be reversible (142), chronic alcohol consumption can lead to more drastic changes and have a more serious effect on the gut microbiota (137). The effects of short-term, low-dose alcohol consumption could be mitigated with appropriate probiotics and interventions like fermented rice liquors, red wine polyphenols or short chain fatty acid produced by protective gut bacteria (138, 141, 145, 146). Recovery from gut microbiota disruption caused by high-dose alcohol consumption requires longer and more complex interventions (146).
With regards to MASLD, the reader is referred to a recent review on changes in microbiota in MASLD patients (147). A noteworthy study revealed reduced gut microbiota diversity in MASLD patients compared to healthy individuals that leads to the disruption of the intestinal barrier in association with liver disease progression in the patient population (148). Furthermore, the severity of MASLD is linked to gut microbiota dysbiosis and alterations in the metabolic functions of the gastrointestinal microbiota. Notably, Bacteroidetes have been independently associated with MASH, while Ruminococcus is linked to significant fibrosis (149). Gut microbiota contribute to MASLD development through various mechanisms, including disruption of liver choline metabolism (necessary for VLDL synthesis and liver lipid export) (150), alterations in bile acid synthesis (151, 152), ethanol production (153), and increased lipopolysaccharides (LPS), which results in liver inflammation (154–156). These findings highlight the critical role of gut microbiota in the pathogenesis of MASLD and underscore the potential of microbiota-targeted therapies in managing the disease. Given the role of altered gut-liver axis in promoting liver ALD and MASLD, betaine has attracted attention as a potential therapeutic agent that supports gut health and protects the microbiome and intestinal barrier function (20, 157–159).
Betaine, a functional nutrient
In recent years, there has been a global trend towards the use of natural substances existing in fruits, vegetables, and herbs as antioxidants and functional nutrients (20). One such compound is betaine (trimethylglycine), which is a short chain, non-essential amino acid derivative and a naturally occurring compound found in animals, plants, and microorganisms. Its rich dietary sources include seafood, especially marine invertebrates; wheat germ/bran; spinach and sugar beets (22, 160–162). While betaine can be obtained externally through nutrition (163), it is also endogenously generated through the metabolism of choline (164). Numerous organisms use betaine because of its vital biochemical roles, and they have developed distinct metabolic routes for both its biosynthesis and catabolism (20). This valuable compound has gained popularity as an ingredient in novel and functional foods due to its demonstrated health benefits. Cereals and cereal products are the main sources of betaine in human nutrition (165). Of the commonly consumed food, refined and whole grains are the best sources of betaine; however, there is 2–4 times higher betaine content in wholegrain products compared to refined grain products (165). Dietary betaine intake is generally less than 150 mg/day (165). Betaine can be synthesized chemically for use in secondary industries or extracted somewhat expensively from sugar beets or processed beet byproducts. Three types of commercial betaine are available: betaine hydrochloride, synthetic anhydrous betaine, and natural anhydrous betaine (157). Because natural betaine has better functional qualities than its synthetic counterpart, the pharmaceutical, cosmetic, and healthcare sectors prefer to employ it (157, 166).
Human plasma/serum typically contains 20–70 μmol/L betaine with the concentration being higher in the adult males than females (27). It is present in almost all tissues, with the highest concentrations in liver, kidney, and testes (28). Betaine insufficiency is associated with dysregulated lipid metabolism, diabetes, metabolic syndrome, and vascular diseases in patients (27, 28). Furthermore, patients with MASLD also exhibit betaine insufficiency (167). The depletion of hepatic betaine is also reported in animal models of ALD (168, 169). Betaine generally appears to be safe at a daily intake of 9–15 g (164, 170). Subacute and sub chronic rat studies determined that betaine is non-toxic at 1, 2 and 5% added to chow diet (171).
Betaine intake as a dietary supplement or through food has a similar bioavailability and is rapidly absorbed to participate in two main biological processes. In one, betaine acts as a donor of methyl groups in the methionine metabolic pathway (159, 172, 173) for remethylating and removing homocysteine (174, 175). In the second process, betaine acts as an osmolyte regulating cells’ adaptation to adverse osmotic environment and environmental stressors, such as low water levels, high salinity, and extreme temperatures (35, 176). In its role in remethylating homocysteine, betaine generates methionine, which upon enzymatic conversion to a key methyl donor, S-adenosylmethionine (SAM) is utilized in a variety of essential transmethylation reactions (22). The conversion of homocysteine to methionine is catalyzed by BHMT, an enzyme that was at first was thought to be present primarily in the liver (22). However, studies from several laboratory have reported the expression of BHMT in other important organs such as white adipose tissue and intestine (25, 34, 177). These results have prompted investigations into the potential health benefits of betaine (178). Several BHMT gene polymorphisms regulating its activity and protein level have been reported suggesting that loss-of-function polymorphisms by affecting betaine metabolism could adversely its efficacy in regulating homocysteine levels and related metabolic pathways (179, 180). However, studies on how genetic factors or population differences influence the efficiency of betaine utilization are few and limited to data collected from healthy population and only offer several speculative mechanisms of liver disease pathogenesis (179, 180). Interestingly, there is a complete loss of BHMT transcripts in liver tumors (181, 182).
Betaine and liver
Research from our laboratory has demonstrated that betaine exerts its protective effects through its role in the BHMT-catalyzed reaction, which not only remethylates and removes homocysteine but also eliminates its detrimental precursor, SAH (21, 29, 30, 183–185). Thus, by preventing the ethanol-induced lowering of SAM: SAH ratio that impairs the activity of several crucial methyltransferase, betaine prevents the development of hallmark features of ALD such as steatosis, apoptosis, accumulation of altered proteins, lysosomal and proteasome dysfunction (24, 29, 30, 184–186). In relation to the gut-liver axis, betaine administration upregulates anti-oxidant defense system and prevents the ethanol-induced increases in serum endotoxin, alanine aminotransferase (AST), aspartate aminotransferase levels (ALT) and liver inflammatory factors, including TNF-α, IFN-γ, interleukin (IL)-18, cyclooxygenase-2 (COX-2), nitric oxide synthase 2 (NOS2), toll-like receptor 4 (TLR4), cluster of differentiation 14 (CD14) (187, 188). However, an earlier study using the intragastric ethanol-feeding model failed to show any protection by betaine feeding in the ethanol-induced up-regulation of CD14 and TNF-α expression (189). Using the same model, however, other laboratories have shown that betaine feeding prevented the alcohol-induced upregulation of several genes including TLR2/4, Janus kinase 3, α-2-macroglobulin (190) which implies that there are multiple pathways that betaine could act on to protect against the development of inflammation during ALD progression.
Similarly in the context of MASLD, studies have reported that betaine prevents the development of MASLD and reduces indices of liver damage in animal models and in patients (31, 32, 177, 191–195).
Readers are encouraged to refer to other comprehensive reviews on the protective effects of betaine on the liver in the context of ALD and MAFLD (20, 160, 192, 196, 197), as these aspects are not covered in detail here. Instead, this review focuses on the alterations in the gut-liver interaction, which has emerged as a crucial factor in the progression of advanced liver injury in both ALD and MASLD.
Betaine and gut function
Intestinal enzyme activities are crucial for physiological processes (198). Betaine administration increases the activities of digestive enzymes, amylase, lipase, trypsin, and chymotrypsin, in the small intestine of stressed rats (35). Dietary betaine supplementation also increases the epithelial crypt-villus ratio in the guts of both healthy and challenged chicks, suggesting betaine’s protective role against coccidial infection is by stabilizing the mucosal structure (199). In addition to improving intestinal morphology and nutrient absorption in yellow-feathered broilers (200), dietary betaine reduces serum levels of the pro-inflammatory cytokines IL-1β, IL-6, TNF-α, and IFN-γ in goslings (201). Betaine not only regulates the osmotic microenvironment for microbes to survive but also provides extra sources of carbon and nitrogen for microbial growth. Furthermore, as a potent organic osmolyte, betaine can cooperate with endocrine hormones to regulate water and electrolyte balance and it plays an important role in improving intestinal functions by enhancing digestive enzymes, ameliorating damaged intestinal morphology, enriching intestinal microbiota (35) and enhancing intestinal barrier function (202).
Betaine and intestinal barrier function
The direct protective effect of betaine on intestinal TJs and barrier integrity was revealed by an in vitro study using Caco-2 cells. The authors reported that co-treatment of these intestinal epithelial cells with betaine prevented both ethanol-and high SAH-induced TJ disruption to maintain epithelial barrier integrity (34). Many other studies have demonstrated the efficacy of betaine in other models of liver injury such as acute liver failure (ALF) showing that betaine protection occurred by an enhanced expression of TJ proteins, ZO-1 and occludin, thus modulating the TLR4 signaling pathway (203). Similarly, betaine treatment reversed LPS-induced downregulation of TJ proteins in intestinal porcine epithelial cells, thereby preventing barrier disruption (202). Furthermore, another study demonstrated that betaine pretreatment alleviates the inflammatory response and improves intestinal barrier function by enhancing the expression of TJ proteins, occludin and ZO-1 in a dextran sulfate sodium-induced colitis model (204). Overall, these studies highlight the significant therapeutic potential of betaine in protecting intestinal health by enhancing production of TJ proteins and their interaction, thereby mitigating gastrointestinal disorders and inflammatory diseases.
Betaine and gut microbiota
Betaine administration changed the intestinal bacterial communities to promote the abundance of the commensals while reducing the pathogenic bacteria as shown in Table 1. There is yet no reports or publications related to modulation of the fungal or viral communities by betaine treatment in any animal models or patient studies.
Mechanism (s) of betaine protection against the development of chronic liver diseases
Several publications indicate that the mechanism of betaine protection against ALD and MASLD development is through remethylating homocysteine that by removing SAH preserves the methionine metabolic pathway and methylation pathways in the liver (20, 21, 24–26, 29, 30, 177, 183–185, 189, 191, 192, 195, 205–209). Despite extensive documentation on betaine’s effect on the liver, as shown in Table 2, only a few studies have been conducted that demonstrates its efficacy in preserving adipose and gut function in cell/animal models of MASLD or ALD. However, these limited studies also indicate that the protective effect of betaine is by preserving the methionine metabolic pathway via reducing SAH and/or homocysteine and rectifying the hypomethylation state (20, 34, 177, 210). Regarding gut-liver axis, several studies have indeed established that the trigger that causes systemic/liver inflammation to exacerbate liver injury (211) is prevented by betaine through stabilizing TJs between enterocytes and preventing the translocation of harmful gut-derived toxins/pathogens to the liver (34, 35, 202, 203). In addition, betaine modulates the gut microbiota, promoting a balanced microbial composition that supports healthy digestion and production of protective factors such as short chain fatty acids (SCFA), vitamins etc. (33, 212). These metabolites, in turn, regulate intestinal inflammation and barrier function (164, 213). Additional studies reveal that betaine influences bile acid metabolism, which is critical in regulating gut microbiota composition and intestinal permeability (214). This modulation helps maintain gut-liver homeostasis and reduces liver inflammation (203, 215). Another mechanism of betaine protection is by influencing gut osmolality and potentially altering intestinal pH, which enhancing digestive enzyme activity, restoring intestinal morphology, and increasing microbiota diversity (35). Further, this modulation of the microbiota can decrease the production of harmful metabolites and inflammatory mediators, thus preventing liver and systemic inflammation (13, 204).
Betaine supplementation maintains the stability of the intestinal morphological structure by preventing cellular water loss (173), increasing the villus height, crypt depth and villus height/crypt depth ratio in the gut which prevent bacterial translocations (216). Betaine modulates the gut microbiota by increasing the abundance of commensals Prevotella, Ruminococcus, Oscillospira, Bifidobacterium, Akkermansia muciniphila, Lactobacillus, Dorea, Bacteroidaceae, Bacteroides, Parabacteroides and decrease the abundance of pathobionts including Desulfovibrio, Mucispirillum schaedleri, Coriobacteriaceae, Lachnospiraceae, Enterorhabdus, Coriobacteriales (33). Betaine increases the level of secretory immunoglobulin A (sIgA), which plays an important role in the clearance of pathogens and harmful substances, and also up-regulates IL-4 and down-regulated TNF-α in small intestinal mucosa (216). Betaine prevents nitric oxide (NO) generation markedly by inhibiting the expression of NO synthase, a principal enzyme for NO formation, consequently repressing inflammation in the intestinal wall (164). Betaine decreases malondialdehyde and increases glutathione and glutathione peroxidase activities in the small intestinal mucosa, thereby improving the antioxidant capacity of small intestine (217). Betaine supplementation up-regulates the gene expression of solute carrier family 7, member 6 (SLC7A6) solute carrier family 6, member 20 (SLC6A20), solute carrier family 38, member (SLC38A2) in the intestine. The consequent activation of mammalian target of rapamycin (mTOR), down-regulate the gene expression of pro-inflammatory factors by activating the phosphorylation state of downstream signaling factors 4EBP-2 and P70S6k1, to alleviate intestinal inflammation and maintain intestinal health (216). Figure 7 depicts the multiple pathways by which betaine modulates the gut homeostasis. While there is indication that the regulation of intestinal barrier function is through maintaining the methylation state (34), there has been no investigation till date on the mechanism by which betaine can maintain the commensal microbiota. Is it by regulating the intestinal pH, sIgA or by some other yet unknown mechanism? This area of research is highly underdeveloped. There is a need for characterizing how betaine regulates the gut microbiome and the further exploration of its role in maintaining intestinal barrier to prevent the development of not only common chronic liver diseases, but also inflammatory diseases of the gastrointestinal system.
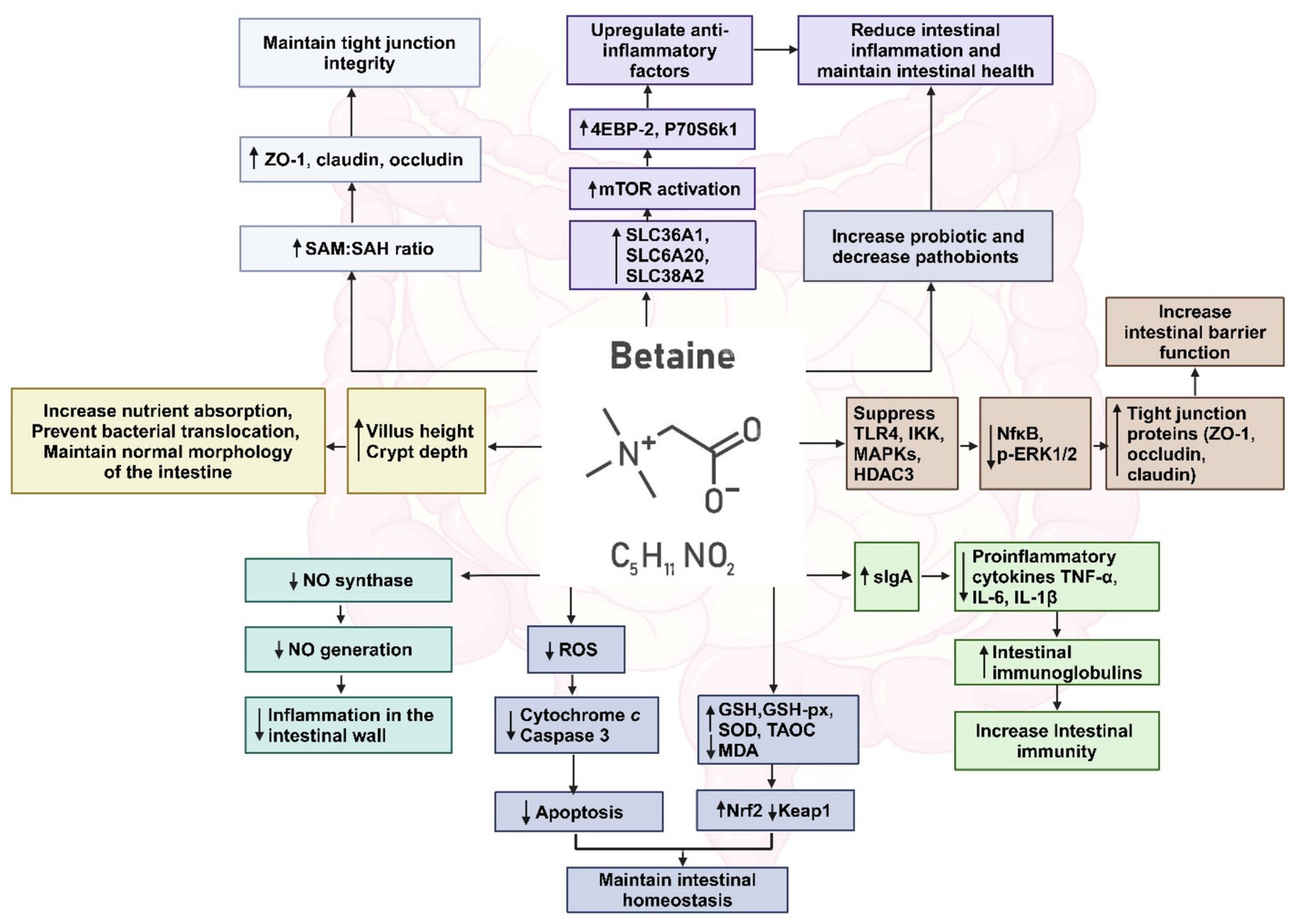
Figure 7. Multiple pathways by which betaine restores gut homeostasis. Created with biorender.com.
Betaine’s efficacy relative to other common therapeutic agents
Betaine exerts significant therapeutic and biological effects that are potentially beneficial for alleviating a diverse range of diseases (20). Probiotics have gained popularity in the last decade by its ability to restore the composition of the gut microbiome and introduce beneficial functions to gut microbial communities, resulting in the amelioration or prevention of gut inflammation and other intestinal or systemic disease phenotypes including liver diseases (218, 219). The major effects of probiotics include the restoration of commensal intestinal microbial communities, suppression of pathogenic bacterial communities, immunomodulation, stimulation of epithelial cell proliferation, and fortification of the intestinal barrier (220). These protective effects of probiotics enhance intestinal epithelial cell function and provide protection against physiological stress (220, 221). The most widely used probiotics like Lactobacillus and Bifidobacterium modulate gut microbiota composition and restore balance (222). Probiotics also enhance SCFA production and improve gut barrier integrity, thereby reducing systemic inflammation (33). Hajirezaee et al. (223) reported significant growth enhancement, digestive enzyme activities, antioxidant capacity, and immune performance in fish receiving a combination of the probiotic Lactobacillus rhamnosus and betaine therapy. In comparison with probiotics, betaine alone can directly protect liver, adipose, intestinal barrier integrity and restore the commensal microbiota and SCFA production (33) and thus in comparison functions to prevent liver disease and other inflammatory condition without any adjunct probiotic use.
Antioxidants like N-acetylcysteine and glutathione effectively mitigate oxidative stress and inflammation, primarily through scavenging reactive oxygen species (224, 225). However, betaine also possesses antioxidant properties by not only reducing oxidative stress via homocysteine remethylation but also by stabilizing the gut barrier and reducing LPS-mediated inflammation (164). Unlike probiotics or traditional antioxidants, betaine addresses both gut microbiota modulation and systemic inflammation through dual pathways: enhancing methylation processes and regulating osmoregulation in gut epithelial cells. This dual role is especially critical in the gut-liver axis, where betaine prevents endotoxin translocation and reduces hepatic inflammation (20).
There are many studies that have illustrated the therapeutic potential of betaine in combination with other compounds for improving health outcomes in both animals and human studies across a range of conditions ranging from inflammation, metabolic stressors, infections to metabolic regulation and immune support (226–232). While these combination therapies exploited betaine’s efficacy in reducing oxidative stress, preventing inflammation and, enhancing methylation processes to improve outcomes, none were related to maintaining gut-liver interaction to prevent chronic liver diseases. Hence discussion of these combinational therapies is beyond the scope of this review.
Limitations and challenges of using betaine as a therapeutic agent
Betaine helps to maintain health levels of stomach acids, increase nutrient absorption and support digestion. Betaine helps to manage harmful microbes and reduce the bacterial infections in the gut. Betaine reduces insulin resistance, lipid synthesis, inflammation, endoplasmic reticulum stress, hepatic oxidative stress, promoting fatty acid oxidation, reshape the intestinal microbiota, prevent liver steatosis. Despite the efficacy of betaine in multiple preclinical studies in promoting overall health by its effect in the gut, liver, adipose, there are several limitations and challenges. One minor challenge is that betaine supplementation causes gastrointestinal discomfort, including bloating or diarrhea in some individuals, particularly when consumed in high amounts. In addition to these mild adverse events, it is likely that loss-of-function BHMT polymorphisms may also pose a challenge in conducting human trials. However, prior selection of the participants can overcome this obstacle. The biggest challenge in its use in clinical settings is the lack of interest by pharmaceuticals to fund these studies. This is because betaine is easily accessible, orally bioavailable, very soluble and a naturally occurring metabolite in our body. It therefore falls on the investigator-initiated efforts to solicit funds for conducting robust clinical trials to validate betaine efficacy outcomes in individuals with inherent differences in gut microbiota composition, dietary habits, metabolic profiles, and liver disease severity. Further, collaborative research involving multi-omics approaches (genomics, metabolomics, and microbiomics) could provide insights into individual variability and enhance the predictive power of preclinical findings. Future research must address these limitations through well-designed studies exploring betaine dose, molecular mechanisms, and long-term safety to harness its potential in promoting gut and overall health in preventing chronic liver diseases.
Despite the challenges mentioned above, there are a few randomized clinical trials conducted using betaine supplementation demonstrating its potential therapeutic applications in a range of diseases and conditions such as cardiovascular diseases, metabolic disorder during pregnancy, inflammatory disorders etc. (NCT01371357, NCT00102843, NCT00126347, NCT01950039, NCT04633044, and NCT06042270). However, none of these clinical trials were related to gut-liver axis modulation in relation to chronic liver diseases, hence no details are included in this review. The reader can refer to the NCT numbers for details on these clinical trials and outcomes.
Translation from preclinical findings to clinical applications
Despite the limitations and challenges, the development of therapeutic protocols to ensure the translational potential of betaine from preclinical to clinical studies are important. The primary goal is to conduct advanced preclinical studies using diverse animal model studies that mimic human conditions to understand the mechanism of betaine’s protective effect on not only gut biology but also other organ systems such as the brain. Another important goal is to validate the preclinical data by (1) conducting well organized early phase clinical trials to assess safety, optimal dosages, and initial efficacy of betaine in humans; (2) incorporating different layers of patient criteria such as genetic polymorphisms, gut microbiota composition and metabolic profiles to understand how individual differences may affect betaine’s efficacy; and (3) conducting long-term observational studies and randomized controlled trials in diverse populations to validate betaine’s effectiveness in modulating gut-liver axis for treating chronic liver and other inflammation-related diseases. These steps will bridge the gap between preclinical research and clinical application of betaine in preventing the development of chronic liver diseases.
Conclusion
In conclusion, gastrointestinal alterations play a significant role in the development and progression of chronic liver diseases such as ALD and MASLD. Disruption of gut homeostasis, including alterations in gut microbiota composition, impaired intestinal barrier function and increased microbial translocation, initiates a cascade of immune activation and inflammatory responses that promotes advanced liver damage. Many therapeutic approaches targeting chronic liver diseases focus on modulating gut dysbiosis and enhancing barrier function. Betaine, through its ability to regulate the protective gut microbiota and maintain intestinal barrier integrity, emerges as a promising therapeutic agent for preventing the development of chronic liver diseases, including ALD and MASLD. Betaine protection is mediated via donating a methyl group in methionine metabolic pathway to remove homocysteine and SAH and preserves cellular methylation potential, thereby safeguarding the gut microbiota and intestinal barrier function. Further exploration of the molecular mechanisms of betaine action in preclinical and clinical studies will enhance our understanding of its therapeutic potential and pave the way for targeted interventions in the management of liver diseases and other inflammatory bowel diseases or systemic inflammatory conditions.
Author contributions
SP: Conceptualization, Resources, Visualization, Writing – original draft, Writing – review & editing. MA: Conceptualization, Writing – original draft, Writing – review & editing. NO: Supervision, Writing – review & editing. KR: Supervision, Writing – review & editing. KK: Conceptualization, Funding acquisition, Project administration, Resources, Supervision, Visualization, Writing – review & editing.
Funding
The author(s) declare that financial support was received for the research and/or publication of this article. This work was supported by the National Institute of Health grants R01 AA026723 (KK), R01 AA028504 (KR), P50 AA030407-1531 (KK), and Merit Review grant I01BX006064 (KK) from the US Department of Veterans Affairs, Biomedical Laboratory Research, and Development Service.
Conflict of interest
The authors declare that the research was conducted in the absence of any commercial or financial relationships that could be construed as a potential conflict of interest.
The author(s) declared that they were an editorial board member of Frontiers, at the time of submission. This had no impact on the peer review process and the final decision.
Publisher’s note
All claims expressed in this article are solely those of the authors and do not necessarily represent those of their affiliated organizations, or those of the publisher, the editors and the reviewers. Any product that may be evaluated in this article, or claim that may be made by its manufacturer, is not guaranteed or endorsed by the publisher.
References
1. Albuquerque-Souza, E, and Sahingur, SE. Periodontitis, chronic liver diseases, and the emerging oral-gut-liver axis. Periodontol. (2022) 89:125–41. doi: 10.1111/prd.12427
2. Asrani, SK, Devarbhavi, H, Eaton, J, and Kamath, PS. Burden of liver diseases in the world. J Hepatol. (2019) 70:151–71. doi: 10.1016/j.jhep.2018.09.014
3. Trefts, E, Gannon, M, and Wasserman, DH. The liver. Curr Biol. (2017) 27:R1147–51. doi: 10.1016/j.cub.2017.09.019
4. Alvarez-Mercado, AI, Navarro-Oliveros, M, Robles-Sanchez, C, Plaza-Diaz, J, Saez-Lara, MJ, Munoz-Quezada, S, et al. Microbial population changes and their relationship with human health and disease. Microorganisms. (2019) 7:68. doi: 10.3390/microorganisms7030068
5. Cornide-Petronio, ME, Alvarez-Mercado, AI, Jimenez-Castro, MB, and Peralta, C. Current knowledge about the effect of nutritional status, supplemented nutrition diet, and gut microbiota on hepatic ischemia-reperfusion and regeneration in liver surgery. Nutrients. (2020) 12:284. doi: 10.3390/nu12020284
6. Petronini, PG, De Angelis, EM, Borghetti, P, Borghetti, AF, and Wheeler, KP. Modulation by betaine of cellular responses to osmotic stress. Biochem J. (1992) 282:69–73. doi: 10.1042/bj2820069
7. Sharma, A, and Nagalli, S. Chronic liver disease In: Stat pearls. Treasure Island, FL: StatPearls Publishing LLC. (2024).
8. Trovato, GM, Catalano, D, Martines, GF, Pirri, C, and Trovato, FM. Western dietary pattern and sedentary life: independent effects of diet and physical exercise intensity on NAFLD. Am J Gastroenterol. (2013) 108:1932–3. doi: 10.1038/ajg.2013.356
9. Albillos, A, De Gottardi, A, and Rescigno, M. The gut-liver axis in liver disease: pathophysiological basis for therapy. J Hepatol. (2020) 72:558–77. doi: 10.1016/j.jhep.2019.10.003
10. Konturek, PC, Harsch, IA, Konturek, K, Schink, M, Konturek, T, Neurath, MF, et al. Gut(−)liver Axis: how Do gut Bacteria influence the liver? Med Sci. (2018) 6:79. doi: 10.3390/medsci6030079
11. Song, Q, and Zhang, X. The role of gut-liver axis in gut microbiome dysbiosis associated NAFLD and NAFLD-HCC. Biomedicines. (2022) 10:524. doi: 10.3390/biomedicines10030524
12. Stolfi, C, Maresca, C, Monteleone, G, and Laudisi, F. Implication of intestinal barrier dysfunction in gut Dysbiosis and diseases. Biomedicines. (2022) 10:289. doi: 10.3390/biomedicines10020289
13. Wang, L, Cao, ZM, Zhang, LL, Li, JM, and Lv, WL. The role of gut microbiota in some liver diseases: from an immunological perspective. Front Immunol. (2022) 13:923599. doi: 10.3389/fimmu.2022.923599
14. Nicoletti, A, Ponziani, FR, Biolato, M, Valenza, V, Marrone, G, Sganga, G, et al. Intestinal permeability in the pathogenesis of liver damage: from non-alcoholic fatty liver disease to liver transplantation. World J Gastroenterol. (2019) 25:4814–34. doi: 10.3748/wjg.v25.i33.4814
15. Artis, D. Epithelial-cell recognition of commensal bacteria and maintenance of immune homeostasis in the gut. Nat Rev Immunol. (2008) 8:411–20. doi: 10.1038/nri2316
16. Varol, C, Zigmond, E, and Jung, S. Securing the immune tightrope: mononuclear phagocytes in the intestinal lamina propria. Nat Rev Immunol. (2010) 10:415–26. doi: 10.1038/nri2778
17. Crispe, IN. The liver as a lymphoid organ. Annu Rev Immunol. (2009) 27:147–63. doi: 10.1146/annurev.immunol.021908.132629
18. Wiest, R, Albillos, A, Trauner, M, Bajaj, JS, and Jalan, R. Targeting the gut-liver axis in liver disease. J Hepatol. (2017) 67:1084–103. doi: 10.1016/j.jhep.2017.05.007
19. Yang, X, Lu, D, Zhuo, J, Lin, Z, Yang, M, and Xu, X. The gut-liver Axis in immune remodeling: new insight into liver diseases. Int J Biol Sci. (2020) 16:2357–66. doi: 10.7150/ijbs.46405
20. Arumugam, MK, Paal, MC, Donohue, TM, Ganesan, M, Osna, NA, and Kharbanda, KK. Beneficial effects of betaine: a comprehensive review. Biology. (2021) 10:456. doi: 10.3390/biology10060456
21. Barak, AJ, Beckenhauer, HC, Mailliard, ME, Kharbanda, KK, and Tuma, DJ. Betaine lowers elevated s-adenosylhomocysteine levels in hepatocytes from ethanol-fed rats. J Nutr. (2003) 133:2845–8. doi: 10.1093/jn/133.9.2845
22. Craig, SA. Betaine in human nutrition. Am J Clin Nutr. (2004) 80:539–49. doi: 10.1093/ajcn/80.3.539
23. Ji, C, Shinohara, M, Kuhlenkamp, J, Chan, C, and Kaplowitz, N. Mechanisms of protection by the betaine-homocysteine methyltransferase/betaine system in HepG2 cells and primary mouse hepatocytes. Hepatology. (2007) 46:1586–96. doi: 10.1002/hep.21854
24. Arumugam, MK, Chava, S, Perumal, SK, Paal, MC, Rasineni, K, Ganesan, M, et al. Acute ethanol-induced liver injury is prevented by betaine administration. Front Physiol. (2022) 13:940148. doi: 10.3389/fphys.2022.940148
25. Arumugam, MK, Chava, S, Rasineni, K, Paal, MC, Donohue, TM Jr, Osna, NA, et al. Elevated S-adenosylhomocysteine induces adipocyte dysfunction to promote alcohol-associated liver steatosis. Sci Rep. (2021) 11:14693. doi: 10.1038/s41598-021-94180-x
26. Kharbanda, KK, Rogers, DD 2nd, Mailliard, ME, Siford, GL, Barak, AJ, Beckenhauer, HC, et al. Role of elevated S-adenosylhomocysteine in rat hepatocyte apoptosis: protection by betaine. Biochem Pharmacol. (2005) 70:1883–90. doi: 10.1016/j.bcp.2005.09.021
27. Lever, M, and Slow, S. The clinical significance of betaine, an osmolyte with a key role in methyl group metabolism. Clin Biochem. (2010) 43:732–44. doi: 10.1016/j.clinbiochem.2010.03.009
28. Slow, S, Lever, M, Chambers, ST, and George, PM. Plasma dependent and independent accumulation of betaine in male and female rat tissues. Physiol Res. (2009) 58:403–10. doi: 10.33549/physiolres.931569
29. Kharbanda, KK, Mailliard, ME, Baldwin, CR, Beckenhauer, HC, Sorrell, MF, and Tuma, DJ. Betaine attenuates alcoholic steatosis by restoring phosphatidylcholine generation via the phosphatidylethanolamine methyltransferase pathway. J Hepatol. (2007) 46:314–21. doi: 10.1016/j.jhep.2006.08.024
30. Kharbanda, KK, Mailliard, ME, Baldwin, CR, Sorrell, MF, and Tuma, DJ. Accumulation of proteins bearing atypical isoaspartyl residues in livers of alcohol-fed rats is prevented by betaine administration: effects on protein-L-isoaspartyl methyltransferase activity. J Hepatol. (2007) 46:1119–25. doi: 10.1016/j.jhep.2007.01.026
31. Patrick, L. Non-alcoholic fatty liver disease: relationship to insulin sensitivity and oxidative stress. Treatment approaches using vitamin E, magnesium, and betaine. Altern Med Rev. (2002) 7:276–91.
32. Xu, L, Huang, D, Hu, Q, Wu, J, Wang, Y, and Feng, J. Betaine alleviates hepatic lipid accumulation via enhancing hepatic lipid export and fatty acid oxidation in rats fed with a high-fat diet. Br J Nutr. (2015) 113:1835–43. doi: 10.1017/S0007114515001130
33. Du, J, Zhang, P, Luo, J, Shen, L, Zhang, S, Gu, H, et al. Dietary betaine prevents obesity through gut microbiota-drived microRNA-378a family. Gut Microbes. (2021) 13:1–19. doi: 10.1080/19490976.2020.1862612
34. Thomes, PG, Osna, NA, Bligh, SM, Tuma, DJ, and Kharbanda, KK. Role of defective methylation reactions in ethanol-induced dysregulation of intestinal barrier integrity. Biochem Pharmacol. (2015) 96:30–8. doi: 10.1016/j.bcp.2015.04.018
35. Wang, H, Li, S, Fang, S, Yang, X, and Feng, J. Betaine improves intestinal functions by enhancing digestive enzymes, ameliorating intestinal morphology, and enriching intestinal microbiota in high-salt stressed rats. Nutrients. (2018) 10:907. doi: 10.3390/nu10070907
36. Chen, M, Zhong, W, and Xu, W. Alcohol and the mechanisms of liver disease. J Gastroenterol Hepatol. (2023) 38:1233–40. doi: 10.1111/jgh.16282
37. Torruellas, C, French, SW, and Medici, V. Diagnosis of alcoholic liver disease. World J Gastroenterol. (2014) 20:11684–99. doi: 10.3748/wjg.v20.i33.11684
38. Wu, X, Fan, X, Miyata, T, Kim, A, Cajigas-Du Ross, CK, Ray, S, et al. Recent advances in understanding of pathogenesis of alcohol-associated liver disease. Annu Rev Pathol. (2023) 18:411–38. doi: 10.1146/annurev-pathmechdis-031521-030435
39. Elamin, E, Masclee, A, Juuti-Uusitalo, K, Van Ijzendoorn, S, Troost, F, Pieters, HJ, et al. Fatty acid ethyl esters induce intestinal epithelial barrier dysfunction via a reactive oxygen species-dependent mechanism in a three-dimensional cell culture model. PLoS One. (2013) 8:e58561. doi: 10.1371/journal.pone.0058561
40. Elamin, EE, Masclee, AA, Dekker, J, and Jonkers, DM. Ethanol metabolism and its effects on the intestinal epithelial barrier. Nutr Rev. (2013) 71:483–99. doi: 10.1111/nure.12027
41. Mackowiak, B, Fu, Y, Maccioni, L, and Gao, B. Alcohol-associated liver disease. J Clin Invest. (2024) 134:6345. doi: 10.1172/JCI176345
42. Seth, D, Haber, PS, Syn, WK, Diehl, AM, and Day, CP. Pathogenesis of alcohol-induced liver disease: classical concepts and recent advances. J Gastroenterol Hepatol. (2011) 26:1089–105. doi: 10.1111/j.1440-1746.2011.06756.x
43. Lieber, CS. Role of oxidative stress and antioxidant therapy in alcoholic and non-alcoholic liver diseases. Adv Pharmacol. (1997) 38:601–28.
44. Rocco, A, Compare, D, Angrisani, D, Sanduzzi Zamparelli, M, and Nardone, G. Alcoholic disease: liver and beyond. World J Gastroenterol. (2014) 20:14652–9. doi: 10.3748/wjg.v20.i40.14652
45. Osna, NA, Donohue, TM Jr, and Kharbanda, KK. Alcoholic liver disease: pathogenesis and current management. Alcohol Res. (2017) 38:147–61.
46. Casanova, J, and Bataller, R. Alcoholic hepatitis: prognosis and treatment. Gastroenterol Hepatol. (2014) 37:262–8. doi: 10.1016/j.gastrohep.2014.02.001
47. Chacko, KR, and Reinus, J. Spectrum of alcoholic liver disease. Clin Liver Dis. (2016) 20:419–27. doi: 10.1016/j.cld.2016.02.002
48. Prado, V, Caballeria, J, Vargas, V, Bataller, R, and Altamirano, J. Alcoholic hepatitis: how far are we and where are we going? Ann Hepatol. (2016) 15:463–73. doi: 10.5604/16652681.1202885
49. Gopal, T, Ai, W, Casey, CA, Donohue, TM Jr, and Saraswathi, V. A review of the role of ethanol-induced adipose tissue dysfunction in alcohol-associated liver disease. Alcohol Clin Exp Res. (2021) 45:1927–39. doi: 10.1111/acer.14698
50. Hsu, CL, and Schnabl, B. The gut-liver axis and gut microbiota in health and liver disease. Nat Rev Microbiol. (2023) 21:719–33. doi: 10.1038/s41579-023-00904-3
51. Luo, L, Chang, Y, and Sheng, L. Gut-liver axis in the progression of non-alcoholic fatty liver disease: from the microbial derivatives-centered perspective. Life Sci. (2023) 321:121614. doi: 10.1016/j.lfs.2023.121614
52. Maccioni, L, Gao, B, Leclercq, S, Pirlot, B, Horsmans, Y, De Timary, P, et al. Intestinal permeability, microbial translocation, changes in duodenal and fecal microbiota, and their associations with alcoholic liver disease progression in humans. Gut Microbes. (2020) 12:1782157. doi: 10.1080/19490976.2020.1782157
53. Mak, KM, Kee, D, and Shin, DW. Alcohol-associated capillarization of sinusoids: a critique since the discovery by Schaffner and popper in 1963. Anat Rec (Hoboken). (2022) 305:1592–610. doi: 10.1002/ar.24829
54. Bessone, F, Razori, MV, and Roma, MG. Molecular pathways of non-alcoholic fatty liver disease development and progression. Cell Mol Life Sci. (2019) 76:99–128. doi: 10.1007/s00018-018-2947-0
55. Angulo, P. Non-alcoholic fatty liver disease. N Engl J Med. (2002) 346:1221–31. doi: 10.1056/NEJMra011775
56. Younossi, Z, Anstee, QM, Marietti, M, Hardy, T, Henry, L, Eslam, M, et al. Global burden of NAFLD and NASH: trends, predictions, risk factors and prevention. Nat Rev Gastroenterol Hepatol. (2018) 15:11–20. doi: 10.1038/nrgastro.2017.109
57. Younossi, ZM, Koenig, AB, Abdelatif, D, Fazel, Y, Henry, L, and Wymer, M. Global epidemiology of non-alcoholic fatty liver disease-Meta-analytic assessment of prevalence, incidence, and outcomes. Hepatology. (2016) 64:73–84. doi: 10.1002/hep.28431
58. Musso, G, Gambino, R, De Michieli, F, Cassader, M, Rizzetto, M, Durazzo, M, et al. Dietary habits and their relations to insulin resistance and postprandial lipemia in non-alcoholic steatohepatitis. Hepatology. (2003) 37:909–16. doi: 10.1053/jhep.2003.50132
59. Clark, JM, Brancati, FL, and Diehl, AM. The prevalence and etiology of elevated aminotransferase levels in the United States. Am J Gastroenterol. (2003) 98:960–7. doi: 10.1111/j.1572-0241.2003.07486.x
60. Guo, X, Yin, X, Liu, Z, and Wang, J. Non-alcoholic fatty liver disease (NAFLD) pathogenesis and natural products for prevention and treatment. Int J Mol Sci. (2022) 23:5489. doi: 10.3390/ijms232415489
61. Singh, S, Osna, NA, and Kharbanda, KK. Treatment options for alcoholic and non-alcoholic fatty liver disease: a review. World J Gastroenterol. (2017) 23:6549–70. doi: 10.3748/wjg.v23.i36.6549
62. Leoni, S, Tovoli, F, Napoli, L, Serio, I, Ferri, S, and Bolondi, L. Current guidelines for the management of non-alcoholic fatty liver disease: a systematic review with comparative analysis. World J Gastroenterol. (2018) 24:3361–73. doi: 10.3748/wjg.v24.i30.3361
63. Sheka, AC, Adeyi, O, Thompson, J, Hameed, B, Crawford, PA, and Ikramuddin, S. Non-alcoholic steatohepatitis: a review. JAMA. (2020) 323:1175–83. doi: 10.1001/jama.2020.2298
64. Day, CP, and James, OF. Steatohepatitis: a tale of two “hits?”. Gastroenterology. (1998) 114:842–5. doi: 10.1016/S0016-5085(98)70599-2
65. Aubert, J, Begriche, K, Knockaert, L, Robin, MA, and Fromenty, B. Increased expression of cytochrome P450 2E1 in non-alcoholic fatty liver disease: mechanisms and pathophysiological role. Clin Res Hepatol Gastroenterol. (2011) 35:630–7. doi: 10.1016/j.clinre.2011.04.015
66. Arslan, N. Obesity, fatty liver disease and intestinal microbiota. World J Gastroenterol. (2014) 20:16452–63. doi: 10.3748/wjg.v20.i44.16452
67. Brunt, EM. Grading and staging the histopathological lesions of chronic hepatitis: the Knodell histology activity index and beyond. Hepatology. (2000) 31:241–6. doi: 10.1002/hep.510310136
68. Wree, A, Broderick, L, Canbay, A, Hoffman, HM, and Feldstein, AE. From NAFLD to NASH to cirrhosis-new insights into disease mechanisms. Nat Rev Gastroenterol Hepatol. (2013) 10:627–36. doi: 10.1038/nrgastro.2013.149
69. Pabst, O, Hornef, MW, Schaap, FG, Cerovic, V, Clavel, T, and Bruns, T. Gut-liver axis: barriers and functional circuits. Nat Rev Gastroenterol Hepatol. (2023) 20:447–61. doi: 10.1038/s41575-023-00771-6
70. Ramadori, G, Moriconi, F, Malik, I, and Dudas, J. Physiology and pathophysiology of liver inflammation, damage and repair. J Physiol Pharmacol. (2008) 59:107–17.
71. Tilg, H, Adolph, TE, and Trauner, M. Gut-liver axis: pathophysiological concepts and clinical implications. Cell Metab. (2022) 34:1700–18. doi: 10.1016/j.cmet.2022.09.017
72. Seki, E, and Schnabl, B. Role of innate immunity and the microbiota in liver fibrosis: crosstalk between the liver and gut. J Physiol. (2012) 590:447–58. doi: 10.1113/jphysiol.2011.219691
73. Tsuchida, T, and Friedman, SL. Mechanisms of hepatic stellate cell activation. Nat Rev Gastroenterol Hepatol. (2017) 14:397–411. doi: 10.1038/nrgastro.2017.38
74. Verbeke, L, Nevens, F, and Laleman, W. Bench-to-beside review: acute-on-chronic liver failure – linking the gut, liver and systemic circulation. Crit Care. (2011) 15:233. doi: 10.1186/cc10424
75. Rao, R. Endotoxemia and gut barrier dysfunction in alcoholic liver disease. Hepatology. (2009) 50:638–44. doi: 10.1002/hep.23009
76. Raya Tonetti, F, Eguileor, A, Mrdjen, M, Pathak, V, Travers, J, Nagy, LE, et al. Gut-liver axis: recent concepts in pathophysiology in alcohol-associated liver disease. Hepatology. (2024) 80:1342–71. doi: 10.1097/HEP.0000000000000924
77. Shao, T, Zhao, C, Li, F, Gu, Z, Liu, L, Zhang, L, et al. Intestinal HIF-1alpha deletion exacerbates alcoholic liver disease by inducing intestinal dysbiosis and barrier dysfunction. J Hepatol. (2018) 69:886–95. doi: 10.1016/j.jhep.2018.05.021
78. Starkel, P, Leclercq, S, De Timary, P, and Schnabl, B. Intestinal dysbiosis and permeability: the yin and yang in alcohol dependence and alcoholic liver disease. Clin Sci. (2018) 132:199–212. doi: 10.1042/CS20171055
79. Luissint, AC, Parkos, CA, and Nusrat, A. Inflammation and the intestinal barrier: leukocyte-epithelial cell interactions, cell junction remodeling, and mucosal repair. Gastroenterology. (2016) 151:616–32. doi: 10.1053/j.gastro.2016.07.008
80. Van Itallie, CM, and Anderson, JM. Architecture of tight junctions and principles of molecular composition. Semin Cell Dev Biol. (2014) 36:157–65. doi: 10.1016/j.semcdb.2014.08.011
81. Hugon, P, Dufour, JC, Colson, P, Fournier, PE, Sallah, K, and Raoult, D. A comprehensive repertoire of prokaryotic species identified in human beings. Lancet Infect Dis. (2015) 15:1211–9. doi: 10.1016/S1473-3099(15)00293-5
82. Pastorelli, L, De Salvo, C, Mercado, JR, Vecchi, M, and Pizarro, TT. Central role of the gut epithelial barrier in the pathogenesis of chronic intestinal inflammation: lessons learned from animal models and human genetics. Front Immunol. (2013) 4:280. doi: 10.3389/fimmu.2013.00280
83. Assimakopoulos, SF, Triantos, C, Thomopoulos, K, Fligou, F, Maroulis, I, Marangos, M, et al. Gut-origin sepsis in the critically ill patient: pathophysiology and treatment. Infection. (2018) 46:751–60. doi: 10.1007/s15010-018-1178-5
84. Anderson, JM, and Van Itallie, CM. Tight junctions and the molecular basis for regulation of paracellular permeability. Am J Phys. (1995) 269:G467–75. doi: 10.1152/ajpgi.1995.269.4.G467
85. Heinemann, U, and Schuetz, A. Structural features of tight-junction proteins. Int J Mol Sci. (2019) 20:6020. doi: 10.3390/ijms20236020
86. Turner, JR. Intestinal mucosal barrier function in health and disease. Nat Rev Immunol. (2009) 9:799–809. doi: 10.1038/nri2653
87. Zihni, C, Mills, C, Matter, K, and Balda, MS. Tight junctions: from simple barriers to multifunctional molecular gates. Nat Rev Mol Cell Biol. (2016) 17:564–80. doi: 10.1038/nrm.2016.80
88. Gill, SR, Pop, M, Deboy, RT, Eckburg, PB, Turnbaugh, PJ, Samuel, BS, et al. Metagenomic analysis of the human distal gut microbiome. Science. (2006) 312:1355–9. doi: 10.1126/science.1124234
89. Ng, KM, Ferreyra, JA, Higginbottom, SK, Lynch, JB, Kashyap, PC, Gopinath, S, et al. Microbiota-liberated host sugars facilitate post-antibiotic expansion of enteric pathogens. Nature. (2013) 502:96–9. doi: 10.1038/nature12503
90. Quigley, EMM. Gut microbiome as a clinical tool in gastrointestinal disease management: are we there yet? Nat Rev Gastroenterol Hepatol. (2017) 14:315–20. doi: 10.1038/nrgastro.2017.29
91. Eckburg, PB, Bik, EM, Bernstein, CN, Purdom, E, Dethlefsen, L, Sargent, M, et al. Diversity of the human intestinal microbial flora. Science. (2005) 308:1635–8. doi: 10.1126/science.1110591
92. Thursby, E, and Juge, N. Introduction to the human gut microbiota. Biochem J. (2017) 474:1823–36. doi: 10.1042/BCJ20160510
93. Bajaj, JS. Alcohol, liver disease and the gut microbiota. Nat Rev Gastroenterol Hepatol. (2019) 16:235–46. doi: 10.1038/s41575-018-0099-1
94. Belkaid, Y, and Hand, TW. Role of the microbiota in immunity and inflammation. Cell. (2014) 157:121–41. doi: 10.1016/j.cell.2014.03.011
95. Hou, K, Wu, ZX, Chen, XY, Wang, JQ, Zhang, D, Xiao, C, et al. Microbiota in health and diseases. Signal Transduct Target Ther. (2022) 7:135. doi: 10.1038/s41392-022-00974-4
96. Vancamelbeke, M, and Vermeire, S. The intestinal barrier: a fundamental role in health and disease. Expert Rev Gastroenterol Hepatol. (2017) 11:821–34. doi: 10.1080/17474124.2017.1343143
97. Brun, P, Castagliuolo, I, Di Leo, V, Buda, A, Pinzani, M, Palu, G, et al. Increased intestinal permeability in obese mice: new evidence in the pathogenesis of non-alcoholic steatohepatitis. Am J Physiol Gastrointest Liver Physiol. (2007) 292:G518–25. doi: 10.1152/ajpgi.00024.2006
98. Cui, Y, Wang, Q, Chang, R, Zhou, X, and Xu, C. Intestinal barrier function-non-alcoholic fatty liver disease interactions and possible role of gut microbiota. J Agric Food Chem. (2019) 67:2754–62. doi: 10.1021/acs.jafc.9b00080
99. Groschwitz, KR, and Hogan, SP. Intestinal barrier function: molecular regulation and disease pathogenesis. J Allergy Clin Immunol. (2009) 124:3–20. doi: 10.1016/j.jaci.2009.05.038
100. Han, R, Qiu, H, Zhong, J, Zheng, N, Li, B, Hong, Y, et al. Si Miao formula attenuates non-alcoholic fatty liver disease by modulating hepatic lipid metabolism and gut microbiota. Phytomedicine. (2021) 85:153544. doi: 10.1016/j.phymed.2021.153544
101. Liu, L, Fu, Q, Li, T, Shao, K, Zhu, X, Cong, Y, et al. Gut microbiota and butyrate contribute to non-alcoholic fatty liver disease in premenopause due to estrogen deficiency. PLoS One. (2022) 17:e0262855. doi: 10.1371/journal.pone.0262855
102. Liu, X, Sun, R, Li, Z, Xiao, R, Lv, P, Sun, X, et al. Luteolin alleviates non-alcoholic fatty liver disease in rats via restoration of intestinal mucosal barrier damage and microbiota imbalance involving in gut-liver axis. Arch Biochem Biophys. (2021) 711:109019. doi: 10.1016/j.abb.2021.109019
103. Miele, L, Valenza, V, La Torre, G, Montalto, M, Cammarota, G, Ricci, R, et al. Increased intestinal permeability and tight junction alterations in non-alcoholic fatty liver disease. Hepatology. (2009) 49:1877–87. doi: 10.1002/hep.22848
104. Parlesak, A, Schafer, C, Schutz, T, Bode, JC, and Bode, C. Increased intestinal permeability to macromolecules and endotoxemia in patients with chronic alcohol abuse in different stages of alcohol-induced liver disease. J Hepatol. (2000) 32:742–7. doi: 10.1016/S0168-8278(00)80242-1
105. Rao, RK, Seth, A, and Sheth, P. Recent advances in alcoholic liver disease I. Role of intestinal permeability and endotoxemia in alcoholic liver disease. Am J Physiol Gastrointest Liver Physiol. (2004) 286:G881–4. doi: 10.1152/ajpgi.00006.2004
106. Tranah, TH, Vijay, GK, Ryan, JM, and Shawcross, DL. Systemic inflammation and ammonia in hepatic encephalopathy. Metab Brain Dis. (2013) 28:1–5. doi: 10.1007/s11011-012-9370-2
107. Xu, Y, Huang, X, Huangfu, B, Hu, Y, Xu, J, Gao, R, et al. Sulforaphane ameliorates non-alcoholic fatty liver disease induced by high-fat and high-fructose diet via LPS/TLR4 in the gut-liver Axis. Nutrients. (2023) 15:743. doi: 10.3390/nu15030743
108. Xue, J, Zhao, M, Liu, Y, Jia, X, Zhang, X, Gu, Q, et al. Hydrogen inhalation ameliorates hepatic inflammation and modulates gut microbiota in rats with high-fat diet-induced non-alcoholic fatty liver disease. Eur J Pharmacol. (2023) 947:175698. doi: 10.1016/j.ejphar.2023.175698
109. Yang, T, Yang, H, Heng, C, Wang, H, Chen, S, Hu, Y, et al. Amelioration of non-alcoholic fatty liver disease by sodium butyrate is linked to the modulation of intestinal tight junctions in db/db mice. Food Funct. (2020) 11:10675–89. doi: 10.1039/D0FO01954B
110. Elamin, E, Masclee, A, Troost, F, Pieters, HJ, Keszthelyi, D, Aleksa, K, et al. Ethanol impairs intestinal barrier function in humans through mitogen activated protein kinase signaling: a combined in vivo and in vitro approach. PLoS One. (2014) 9:e107421. doi: 10.1371/journal.pone.0107421
111. Li, X, Wang, C, Nie, J, Lv, D, Wang, T, and Xu, Y. Toll-like receptor 4 increases intestinal permeability through up-regulation of membrane PKC activity in alcoholic steatohepatitis. Alcohol. (2013) 47:459–65. doi: 10.1016/j.alcohol.2013.05.004
112. Rao, RK. Acetaldehyde-induced increase in paracellular permeability in Caco-2 cell monolayer. Alcohol Clin Exp Res. (1998) 22:1724–30. doi: 10.1111/j.1530-0277.1998.tb03972.x
113. Rao, RK. Acetaldehyde-induced barrier disruption and paracellular permeability in Caco-2 cell monolayer. Methods Mol Biol. (2008) 447:171–83. doi: 10.1007/978-1-59745-242-7_13
114. Zhong, W, Li, Q, Zhang, W, Sun, Q, Sun, X, and Zhou, Z. Modulation of intestinal barrier and bacterial endotoxin production contributes to the beneficial effect of nicotinic acid on alcohol-induced endotoxemia and hepatic inflammation in rats. Biomol Ther. (2015) 5:2643–58. doi: 10.3390/biom5042643
115. Zhong, W, Mcclain, CJ, Cave, M, Kang, YJ, and Zhou, Z. The role of zinc deficiency in alcohol-induced intestinal barrier dysfunction. Am J Physiol Gastrointest Liver Physiol. (2010) 298:G625–33. doi: 10.1152/ajpgi.00350.2009
116. Atkinson, KJ, and Rao, RK. Role of protein tyrosine phosphorylation in acetaldehyde-induced disruption of epithelial tight junctions. Am J Physiol Gastrointest Liver Physiol. (2001) 280:G1280–8. doi: 10.1152/ajpgi.2001.280.6.G1280
117. Dunagan, M, Chaudhry, K, Samak, G, and Rao, RK. Acetaldehyde disrupts tight junctions in Caco-2 cell monolayers by a protein phosphatase 2A-dependent mechanism. Am J Physiol Gastrointest Liver Physiol. (2012) 303:G1356–64. doi: 10.1152/ajpgi.00526.2011
118. Sheth, P, Seth, A, Atkinson, KJ, Gheyi, T, Kale, G, Giorgianni, F, et al. Acetaldehyde dissociates the PTP1B-E-cadherin-beta-catenin complex in Caco-2 cell monolayers by a phosphorylation-dependent mechanism. Biochem J. (2007) 402:291–300. doi: 10.1042/BJ20060665
119. Li, Y, Liu, T, Yan, C, Xie, R, Guo, Z, Wang, S, et al. Diammonium glycyrrhizinate protects against non-alcoholic fatty liver disease in mice through modulation of gut microbiota and restoration of intestinal barrier. Mol Pharm. (2018) 15:3860–70. doi: 10.1021/acs.molpharmaceut.8b00347
120. Kaushal, K, Agarwal, S, Sharma, S, Goswami, P, Singh, N, Sachdev, V, et al. Demonstration of gut-barrier dysfunction in early stages of non-alcoholic fatty liver disease: a proof-of-concept study. J Clin Exp Hepatol. (2022) 12:1102–13. doi: 10.1016/j.jceh.2022.01.006
121. Dubinkina, VB, Tyakht, AV, Odintsova, VY, Yarygin, KS, Kovarsky, BA, Pavlenko, AV, et al. Links of gut microbiota composition with alcohol dependence syndrome and alcoholic liver disease. Microbiome. (2017) 5:141. doi: 10.1186/s40168-017-0359-2
122. Hartmann, P, Seebauer, CT, and Schnabl, B. Alcoholic liver disease: the gut microbiome and liver cross talk. Alcohol Clin Exp Res. (2015) 39:763–75. doi: 10.1111/acer.12704
123. Weiss, GA, and Hennet, T. Mechanisms and consequences of intestinal dysbiosis. Cell Mol Life Sci. (2017) 74:2959–77. doi: 10.1007/s00018-017-2509-x
124. Vassallo, G, Mirijello, A, Ferrulli, A, Antonelli, M, Landolfi, R, Gasbarrini, A, et al. Review article: alcohol and gut microbiota – the possible role of gut microbiota modulation in the treatment of alcoholic liver disease. Aliment Pharmacol Ther. (2015) 41:917–27. doi: 10.1111/apt.13164
125. Boltin, D, and Niv, Y. Pharmacological and alimentary alteration of the gastric barrier. Best Pract Res Clin Gastroenterol. (2014) 28:981–94. doi: 10.1016/j.bpg.2014.09.001
126. Franke, A, Teyssen, S, and Singer, MV. Alcohol-related diseases of the esophagus and stomach. Dig Dis. (2005) 23:204–13. doi: 10.1159/000090167
127. Teyssen, S, Gonzalez-Calero, G, Schimiczek, M, and Singer, MV. Maleic acid and succinic acid in fermented alcoholic beverages are the stimulants of gastric acid secretion. J Clin Invest. (1999) 103:707–13. doi: 10.1172/JCI3620
128. Brenner, H, Rothenbacher, D, Bode, G, and Adler, G. Relation of smoking and alcohol and coffee consumption to active Helicobacter pylori infection: cross sectional study. BMJ. (1997) 315:1489–92. doi: 10.1136/bmj.315.7121.1489
129. Capurso, G, and Lahner, E. The interaction between smoking, alcohol and the gut microbiome. Best Pract Res Clin Gastroenterol. (2017) 31:579–88. doi: 10.1016/j.bpg.2017.10.006
130. Yan, AW, Fouts, DE, Brandl, J, Starkel, P, Torralba, M, Schott, E, et al. Enteric dysbiosis associated with a mouse model of alcoholic liver disease. Hepatology. (2011) 53:96–105. doi: 10.1002/hep.24018
131. Chen, G, Shi, F, Yin, W, Guo, Y, Liu, A, Shuai, J, et al. Gut microbiota dysbiosis: the potential mechanisms by which alcohol disrupts gut and brain functions. Front Microbiol. (2022) 13:916765. doi: 10.3389/fmicb.2022.916765
132. Wang, Y, Kirpich, I, Liu, Y, Ma, Z, Barve, S, Mcclain, CJ, et al. Lactobacillus rhamnosus GG treatment potentiates intestinal hypoxia-inducible factor, promotes intestinal integrity and ameliorates alcohol-induced liver injury. Am J Pathol. (2011) 179:2866–75. doi: 10.1016/j.ajpath.2011.08.039
133. Wang, Y, Liu, Y, Sidhu, A, Ma, Z, Mcclain, C, and Feng, W. Lactobacillus rhamnosus GG culture supernatant ameliorates acute alcohol-induced intestinal permeability and liver injury. Am J Physiol Gastrointest Liver Physiol. (2012) 303:G32–41. doi: 10.1152/ajpgi.00024.2012
134. Zhao, H, Zhao, C, Dong, Y, Zhang, M, Wang, Y, Li, F, et al. Inhibition of miR122a by Lactobacillus rhamnosus GG culture supernatant increases intestinal occludin expression and protects mice from alcoholic liver disease. Toxicol Lett. (2015) 234:194–200. doi: 10.1016/j.toxlet.2015.03.002
135. Meroni, M, Longo, M, and Dongiovanni, P. Alcohol or gut microbiota: who is the guilty? Int J Mol Sci. (2019) 20:4568. doi: 10.3390/ijms20184568
136. Philips, CA, Pande, A, Shasthry, SM, Jamwal, KD, Khillan, V, Chandel, SS, et al. Healthy donor fecal microbiota transplantation in steroid-ineligible severe alcoholic hepatitis: a pilot study. Clin Gastroenterol Hepatol. (2017) 15:600–2. doi: 10.1016/j.cgh.2016.10.029
137. Bjorkhaug, ST, Aanes, H, Neupane, SP, Bramness, JG, Malvik, S, Henriksen, C, et al. Characterization of gut microbiota composition and functions in patients with chronic alcohol overconsumption. Gut Microbes. (2019) 10:663–75. doi: 10.1080/19490976.2019.1580097
138. Bull-Otterson, L, Feng, W, Kirpich, I, Wang, Y, Qin, X, Liu, Y, et al. Metagenomic analyses of alcohol induced pathogenic alterations in the intestinal microbiome and the effect of Lactobacillus rhamnosus GG treatment. PLoS One. (2013) 8:e53028. doi: 10.1371/journal.pone.0053028
139. Hartmann, P, Chen, WC, and Schnabl, B. The intestinal microbiome and the leaky gut as therapeutic targets in alcoholic liver disease. Front Physiol. (2012) 3:402. doi: 10.3389/fphys.2012.00402
140. Lang, S, Fairfied, B, Gao, B, Duan, Y, Zhang, X, Fouts, DE, et al. Changes in the fecal bacterial microbiota associated with disease severity in alcoholic hepatitis patients. Gut Microbes. (2020) 12:1785251. doi: 10.1080/19490976.2020.1785251
141. Lin, D, Jiang, X, Zhao, Y, Zhai, X, and Yang, X. Komagataeibacter hansenii CGMCC 3917 alleviates alcohol-induced liver injury by regulating fatty acid metabolism and intestinal microbiota diversity in mice. Food Funct. (2020) 11:4591–604. doi: 10.1039/C9FO02040C
142. Ming, L, Qiao, X, Yi, L, Siren, D, He, J, Hai, L, et al. Camel milk modulates ethanol-induced changes in the gut microbiome and transcriptome in a mouse model of acute alcoholic liver disease. J Dairy Sci. (2020) 103:3937–49. doi: 10.3168/jds.2019-17247
143. Mutlu, E, Keshavarzian, A, Engen, P, Forsyth, CB, Sikaroodi, M, and Gillevet, P. Intestinal dysbiosis: a possible mechanism of alcohol-induced endotoxemia and alcoholic steatohepatitis in rats. Alcohol Clin Exp Res. (2009) 33:1836–46. doi: 10.1111/j.1530-0277.2009.01022.x
144. Mutlu, EA, Gillevet, PM, Rangwala, H, Sikaroodi, M, Naqvi, A, Engen, PA, et al. Colonic microbiome is altered in alcoholism. Am J Physiol Gastrointest Liver Physiol. (2012) 302:G966–78. doi: 10.1152/ajpgi.00380.2011
145. Cresci, GA, Bush, K, and Nagy, LE. Tributyrin supplementation protects mice from acute ethanol-induced gut injury. Alcohol Clin Exp Res. (2014) 38:1489–501. doi: 10.1111/acer.12428
146. Lee, JE, Ha, JS, Park, HY, and Lee, E. Alteration of gut microbiota composition by short-term low-dose alcohol intake is restored by fermented rice liquor in mice. Food Res Int. (2020) 128:108800. doi: 10.1016/j.foodres.2019.108800
147. Kobayashi, T, Iwaki, M, Nakajima, A, Nogami, A, and Yoneda, M. Current research on the pathogenesis of NAFLD/NASH and the gut-liver axis: gut microbiota, dysbiosis, and leaky-gut syndrome. Int J Mol Sci. (2022) 23:1689. doi: 10.3390/ijms231911689
148. Rau, M, Rehman, A, Dittrich, M, Groen, AK, Hermanns, HM, Seyfried, F, et al. Fecal SCFAs and SCFA-producing bacteria in gut microbiome of human NAFLD as a putative link to systemic T-cell activation and advanced disease. United European Gastroenterol J. (2018) 6:1496–507. doi: 10.1177/2050640618804444
149. Boursier, J, Mueller, O, Barret, M, Machado, M, Fizanne, L, Araujo-Perez, F, et al. The severity of non-alcoholic fatty liver disease is associated with gut dysbiosis and shift in the metabolic function of the gut microbiota. Hepatology. (2016) 63:764–75. doi: 10.1002/hep.28356
150. Zhang, R, Yan, Z, Zhong, H, Luo, R, Liu, W, Xiong, S, et al. Gut microbial metabolites in MASLD: implications of mitochondrial dysfunction in the pathogenesis and treatment. Hepatol Commun. (2024) 8:484. doi: 10.1097/HC9.0000000000000484
151. Monga Kravetz, A, Testerman, T, Galuppo, B, Graf, J, Pierpont, B, Siebel, S, et al. Effect of gut microbiota and PNPLA3 rs738409 variant on non-alcoholic fatty liver disease (NAFLD) in obese youth. J Clin Endocrinol Metab. (2020) 105:e3575–85. doi: 10.1210/clinem/dgaa382
152. Zhuge, A, Li, S, Lou, P, Wu, W, Wang, K, Yuan, Y, et al. Longitudinal 16S rRNA sequencing reveals relationships among alterations of gut microbiota and non-alcoholic fatty liver disease progression in mice. Microbiol Spectr. (2022) 10:e0004722. doi: 10.1128/spectrum.00047-22
153. Yuan, J, Chen, C, Cui, J, Lu, J, Yan, C, Wei, X, et al. Fatty liver disease caused by high-alcohol-producing Klebsiella pneumoniae. Cell Metab. (2019) 30:e677. doi: 10.1016/j.cmet.2019.11.006
154. Ferro, D, Baratta, F, Pastori, D, Cocomello, N, Colantoni, A, Angelico, F, et al. New insights into the pathogenesis of non-alcoholic fatty liver disease: gut-derived lipopolysaccharides and oxidative stress. Nutrients. (2020) 12:2762. doi: 10.3390/nu12092762
155. Kirpich, IA, Marsano, LS, and Mcclain, CJ. Gut-liver axis, nutrition, and non-alcoholic fatty liver disease. Clin Biochem. (2015) 48:923–30. doi: 10.1016/j.clinbiochem.2015.06.023
156. Poeta, M, Pierri, L, and Vajro, P. Gut-liver Axis derangement in non-alcoholic fatty liver disease. Children. (2017) 4:66. doi: 10.3390/children4080066
157. Dobrijevic, D, Pastor, K, Nastic, N, Ozogul, F, Krulj, J, Kokic, B, et al. Betaine as a functional ingredient: metabolism, health-promoting attributes, food sources, applications and analysis methods. Molecules. (2023) 28:4824. doi: 10.3390/molecules28124824
158. Ghosh, S, Whitley, CS, Haribabu, B, and Jala, VR. Regulation of intestinal barrier function by microbial metabolites. Cell Mol Gastroenterol Hepatol. (2021) 11:1463–82. doi: 10.1016/j.jcmgh.2021.02.007
159. Kim, DH, Sung, B, Kang, YJ, Jang, JY, Hwang, SY, Lee, Y, et al. Anti-inflammatory effects of betaine on AOM/DSS-induced colon tumorigenesis in ICR male mice. Int J Oncol. (2014) 45:1250–6. doi: 10.3892/ijo.2014.2515
160. Day, CR, and Kempson, SA. Betaine chemistry, roles, and potential use in liver disease. Biochim Biophys Acta. (2016) 1860:1098–106. doi: 10.1016/j.bbagen.2016.02.001
161. Sakamoto, A, Ono, H, Mizoguchi, N, and Sakura, N. Betaine and homocysteine concentrations in infant formulae and breast milk. Pediatr Int. (2001) 43:637–40. doi: 10.1046/j.1442-200X.2001.01465.x
162. Zhao, N, Yang, S, Jia, Y, Sun, B, He, B, and Zhao, R. Maternal betaine supplementation attenuates glucocorticoid-induced hepatic lipid accumulation through epigenetic modification in adult offspring rats. J Nutr Biochem. (2018) 54:105–12. doi: 10.1016/j.jnutbio.2017.12.003
163. Willingham, BD, Ragland, TJ, and Ormsbee, MJ. Betaine supplementation may improve heat tolerance: potential mechanisms in humans. Nutrients. (2020) 12:2939. doi: 10.3390/nu12102939
164. Zhao, G, He, F, Wu, C, Li, P, Li, N, Deng, J, et al. Betaine in inflammation: mechanistic aspects and applications. Front Immunol. (2018) 9:1070. doi: 10.3389/fimmu.2018.01070
165. Ross, AB, Zangger, A, and Guiraud, SP. Cereal foods are the major source of betaine in the Western diet--analysis of betaine and free choline in cereal foods and updated assessments of betaine intake. Food Chem. (2014) 145:859–65. doi: 10.1016/j.foodchem.2013.08.122
166. Altinisik, S, Zeidan, H, Yilmaz, MD, and Marti, ME. Reactive extraction of betaine from Sugar beet processing byproducts. ACS Omega. (2023) 8:11029–38. doi: 10.1021/acsomega.2c07845
167. Sookoian, S, Puri, P, Castano, GO, Scian, R, Mirshahi, F, Sanyal, AJ, et al. Non-alcoholic steatohepatitis is associated with a state of betaine-insufficiency. Liver Int. (2017) 37:611–9. doi: 10.1111/liv.13249
168. Barak, AJ, Baker, H, and Tuma, DJ. Influence of ethanol on in-vivo levels of hepatic Methylators betaine and N5-Methyltetrahydrofolate in the rat. IRCS Med Sci. (1981) 9:527–8.
169. Barak, AJ, Beckenhauer, HC, Tuma, DJ, and Badakhsh, S. Effects of prolonged ethanol feeding on methionine metabolism in rat liver. Biochem Cell Biol. (1987) 65:230–3. doi: 10.1139/o87-029
170. Ganesan, B, Anandan, R, and Lakshmanan, PT. Studies on the protective effects of betaine against oxidative damage during experimentally induced restraint stress in Wistar albino rats. Cell Stress Chaperones. (2011) 16:641–52. doi: 10.1007/s12192-011-0276-4
171. Hayes, KC, Pronczuk, A, Cook, MW, and Robbins, MC. Betaine in sub-acute and sub-chronic rat studies. Food Chem Toxicol. (2003) 41:1685–700. doi: 10.1016/S0278-6915(03)00196-0
172. Ducker, GS, and Rabinowitz, JD. One-carbon metabolism in health and disease. Cell Metab. (2017) 25:27–42. doi: 10.1016/j.cmet.2016.08.009
173. Ratriyanto, A, and Mosenthin, R. Osmoregulatory function of betaine in alleviating heat stress in poultry. J Anim Physiol Anim Nutr. (2018) 102:1634–50. doi: 10.1111/jpn.12990
174. Ueland, PM. Choline and betaine in health and disease. J Inherit Metab Dis. (2011) 34:3–15. doi: 10.1007/s10545-010-9088-4
175. Zeisel, SH, and Blusztajn, JK. Choline and human nutrition. Annu Rev Nutr. (1994) 14:269–96. doi: 10.1146/annurev.nu.14.070194.001413
176. Teixido, N, Canamas, TP, Usall, J, Torres, R, Magan, N, and Vinas, I. Accumulation of the compatible solutes, glycine-betaine and ectoine, in osmotic stress adaptation and heat shock cross-protection in the biocontrol agent Pantoea agglomerans CPA-2. Lett Appl Microbiol. (2005) 41:248–52. doi: 10.1111/j.1472-765X.2005.01757.x
177. Wang, Z, Yao, T, Pini, M, Zhou, Z, Fantuzzi, G, and Song, Z. Betaine improved adipose tissue function in mice fed a high-fat diet: a mechanism for hepatoprotective effect of betaine in non-alcoholic fatty liver disease. Am J Physiol Gastrointest Liver Physiol. (2010) 298:G634–42. doi: 10.1152/ajpgi.00249.2009
178. Ismaeel, A. Effects of betaine supplementation on muscle strength and power: a systematic review. J Strength Cond Res. (2017) 31:2338–46. doi: 10.1519/JSC.0000000000001959
179. Feng, Q, Kalari, K, Fridley, BL, Jenkins, G, Ji, Y, Abo, R, et al. Betaine-homocysteine methyltransferase: human liver genotype-phenotype correlation. Mol Genet Metab. (2011) 102:126–33. doi: 10.1016/j.ymgme.2010.10.010
180. Li, F, Feng, Q, Lee, C, Wang, S, Pelleymounter, LL, Moon, I, et al. Human betaine-homocysteine methyltransferase (BHMT) and BHMT2: common gene sequence variation and functional characterization. Mol Genet Metab. (2008) 94:326–35. doi: 10.1016/j.ymgme.2008.03.013
181. Pellanda, H. Betaine homocysteine methyltransferase (BHMT)-dependent remethylation pathway in human healthy and tumoral liver. Clin Chem Lab Med. (2013) 51:617–21. doi: 10.1515/cclm-2012-0689
182. Pellanda, H, Namour, F, Fofou-Caillierez, M, Bressenot, A, Alberto, JM, Chery, C, et al. A splicing variant leads to complete loss of function of betaine-homocysteine methyltransferase (BHMT) gene in hepatocellular carcinoma. Int J Biochem Cell Biol. (2012) 44:385–92. doi: 10.1016/j.biocel.2011.11.014
183. Barak, AJ, Beckenhauer, HC, Kharbanda, KK, and Tuma, DJ. Chronic ethanol consumption increases homocysteine accumulation in hepatocytes. Alcohol. (2001) 25:77–81. doi: 10.1016/S0741-8329(01)00168-9
184. Kharbanda, KK. Alcoholic liver disease and methionine metabolism. Semin Liver Dis. (2009) 29:155–65. doi: 10.1055/s-0029-1214371
185. Kharbanda, KK, Todero, SL, Ward, BW, Cannella, JJ, and Tuma, DJ. Betaine administration corrects ethanol-induced defective VLDL secretion. Mol Cell Biochem. (2009) 327:75–8. doi: 10.1007/s11010-009-0044-2
186. Osna, NA, White, RL, Donohue, TM Jr, Beard, MR, Tuma, DJ, and Kharbanda, KK. Impaired methylation as a novel mechanism for proteasome suppression in liver cells. Biochem Biophys Res Commun. (2010) 391:1291–6. doi: 10.1016/j.bbrc.2009.12.074
187. Jung, YS, Kim, SJ, Kwon Do, Y, Ahn, CW, Kim, YS, Choi, DW, et al. Alleviation of alcoholic liver injury by betaine involves an enhancement of antioxidant defense via regulation of sulfur amino acid metabolism. Food Chem Toxicol. (2013) 62:292–8. doi: 10.1016/j.fct.2013.08.049
188. Shi, QZ, Wang, LW, Zhang, W, and Gong, ZJ. Betaine inhibits toll-like receptor 4 expression in rats with ethanol-induced liver injury. World J Gastroenterol. (2010) 16:897–903. doi: 10.3748/wjg.v16.i7.897
189. Ji, C, and Kaplowitz, N. Betaine decreases hyperhomocysteinemia, endoplasmic reticulum stress, and liver injury in alcohol-fed mice. Gastroenterology. (2003) 124:1488–99. doi: 10.1016/S0016-5085(03)00276-2
190. Li, J, Li, XM, Caudill, M, Malysheva, O, Bardag-Gorce, F, Oliva, J, et al. Betaine feeding prevents the blood alcohol cycle in rats fed alcohol continuously for 1 month using the rat intragastric tube feeding model. Exp Mol Pathol. (2011) 91:540–7. doi: 10.1016/j.yexmp.2011.05.009
191. Kathirvel, E, Morgan, K, Nandgiri, G, Sandoval, BC, Caudill, MA, Bottiglieri, T, et al. Betaine improves non-alcoholic fatty liver and associated hepatic insulin resistance: a potential mechanism for hepatoprotection by betaine. Am J Physiol Gastrointest Liver Physiol. (2010) 299:G1068–77. doi: 10.1152/ajpgi.00249.2010
192. Mukherjee, S, Bernard, T, Kharbanda, K, Barak, AJ, Sorrell, MF, and Tuma, DJ. Impact of betaine on hepatic fibrosis and homocysteine in non-alcoholic steatohepatitis-a prospective cohort study. Open Transl. J. (2011) 3:1–4. doi: 10.2174/1876399501103010001
193. Song, Z, Deaciuc, I, Zhou, Z, Song, M, Chen, T, Hill, D, et al. Involvement of AMP-activated protein kinase in beneficial effects of betaine on high-sucrose diet-induced hepatic steatosis. Am J Physiol Gastrointest Liver Physiol. (2007) 293:G894–902. doi: 10.1152/ajpgi.00133.2007
194. Tokar, JL, and Berg, CL. Therapeutic options in non-alcoholic fatty liver disease. Curr Treat Options Gastroenterol. (2002) 5:425–36. doi: 10.1007/s11938-002-0030-1
195. Zhang, W, Wang, LW, Wang, LK, Li, X, Zhang, H, Luo, LP, et al. Betaine protects against high-fat-diet-induced liver injury by inhibition of high-mobility group box 1 and toll-like receptor 4 expression in rats. Dig Dis Sci. (2013) 58:3198–206. doi: 10.1007/s10620-013-2775-x
196. Rehman, A, and Mehta, KJ. Betaine in ameliorating alcohol-induced hepatic steatosis. Eur J Nutr. (2022) 61:1167–76. doi: 10.1007/s00394-021-02738-2
197. Thomes, PG, Bligh, SM, and Kharbanda, KK. Multiple roles of betaine against alcohol-induced liver injury In: VR Preedy, editor. Betaine, chemistry, analysis, function and effects. UK: Royal Society of Chemistry (2015). 285–310.
198. Cho, I, Yamanishi, S, Cox, L, Methe, BA, Zavadil, J, Li, K, et al. Antibiotics in early life alter the murine colonic microbiome and adiposity. Nature. (2012) 488:621–6. doi: 10.1038/nature11400
199. Kettunen, H, Tiihonen, K, Peuranen, S, Saarinen, MT, and Remus, JC. Dietary betaine accumulates in the liver and intestinal tissue and stabilizes the intestinal epithelial structure in healthy and coccidia-infected broiler chicks. Comp Biochem Physiol A Mol Integr Physiol. (2001) 130:759–69. doi: 10.1016/S1095-6433(01)00410-X
200. Liu, W, Yuan, Y, Sun, C, Balasubramanian, B, Zhao, Z, and An, L. Effects of dietary betaine on growth performance, digestive function, carcass traits, and meat quality in indigenous yellow-feathered broilers under long-term heat stress. Animals. (2019) 9:506. doi: 10.3390/ani9080506
201. Yang, Z, Yang, JJ, Zhu, PJ, Han, HM, Wan, XL, Yang, HM, et al. Effects of betaine on growth performance, intestinal health, and immune response of goslings challenged with lipopolysaccharide. Poult Sci. (2022) 101:102153. doi: 10.1016/j.psj.2022.102153
202. Wu, J, He, C, Bu, J, Luo, Y, Yang, S, Ye, C, et al. Betaine attenuates LPS-induced downregulation of Occludin and Claudin-1 and restores intestinal barrier function. BMC Vet Res. (2020) 16:75. doi: 10.1186/s12917-020-02298-3
203. Chen, Q, Wang, Y, Jiao, F, Shi, C, Pei, M, Wang, L, et al. Betaine inhibits toll-like receptor 4 responses and restores intestinal microbiota in acute liver failure mice. Sci Rep. (2020) 10:21850. doi: 10.1038/s41598-020-78935-6
204. Zhao, N, Yang, Y, Chen, C, Jing, T, Hu, Y, Xu, H, et al. Betaine supplementation alleviates dextran sulfate sodium-induced colitis via regulating the inflammatory response, enhancing the intestinal barrier, and altering gut microbiota. Food Funct. (2022) 13:12814–26. doi: 10.1039/D2FO02942A
205. Kharbanda, KK. Role of transmethylation reactions in alcoholic liver disease. World J Gastroenterol. (2007) 13:4947–54. doi: 10.3748/wjg.v13.i37.4947
206. Kharbanda, KK, Rogers, DD, Mailliard, ME, Siford, GL, Barak, AJ, Beckenhauer, HC, et al. A comparison of the effects of betaine and S-adenosylmethionine on ethanol-induced changes in methionine metabolism and steatosis in rat hepatocytes. J Nutr. (2005) 135:519–24. doi: 10.1093/jn/135.3.519
207. Kharbanda, KK, Ronis, MJJ, Shearn, CT, Petersen, DR, Zakhari, S, Warner, DR, et al. Role of nutrition in alcoholic liver disease: summary of the symposium at the ESBRA 2017 congress. Biomol Ther. (2018) 8:8. doi: 10.3390/biom8020016
208. Kharbanda, KK, Todero, SL, King, AL, Osna, NA, Mcvicker, BL, Tuma, DJ, et al. Betaine treatment attenuates chronic ethanol-induced hepatic steatosis and alterations to the mitochondrial respiratory chain proteome. Int J Hepatol. (2012) 2012:962183:1–10. doi: 10.1155/2012/962183
209. Mukherjee, S. Betaine and non-alcoholic steatohepatitis: back to the future? World J Gastroenterol. (2011) 17:3663–4. doi: 10.3748/wjg.v17.i32.3663
210. Dou, X, Xia, Y, Chen, J, Qian, Y, Li, S, Zhang, X, et al. Rectification of impaired adipose tissue methylation status and lipolytic response contributes to hepatoprotective effect of betaine in a mouse model of alcoholic liver disease. Br J Pharmacol. (2014) 171:4073–86. doi: 10.1111/bph.12765
211. Chopyk, DM, and Grakoui, A. Contribution of the intestinal microbiome and gut barrier to hepatic disorders. Gastroenterology. (2020) 159:849–63. doi: 10.1053/j.gastro.2020.04.077
212. Alvarenga, L, Ferreira, MS, Kemp, JA, and Mafra, D. The role of betaine in patients with chronic kidney disease: a narrative review. Curr Nutr Rep. (2022) 11:395–406. doi: 10.1007/s13668-022-00426-z
213. Ferenc, K, Sokal-Dembowska, A, Helma, K, Motyka, E, Jarmakiewicz-Czaja, S, and Filip, R. Modulation of the gut microbiota by nutrition and its relationship to epigenetics. Int J Mol Sci. (2024) 25:1228. doi: 10.3390/ijms25021228
214. Li, S, Xu, S, Zhao, Y, Wang, H, and Feng, J. Dietary betaine addition promotes hepatic cholesterol synthesis, bile acid conversion, and export in rats. Nutrients. (2020) 12:1399. doi: 10.3390/nu12051399
215. Zhao, N, Yang, S, Feng, Y, Sun, B, and Zhao, R. Enhanced hepatic cholesterol accumulation induced by maternal betaine exposure is associated with hypermethylation of CYP7A1 gene promoter. Endocrine. (2019) 64:544–51. doi: 10.1007/s12020-019-01906-z
216. Li, Z, Pu, J, Chen, X, Chen, Y, Peng, X, Cai, J, et al. Betaine addition to the diet alleviates intestinal injury in growing rabbits during the summer heat through the AAT/mTOR pathway. J Anim Sci Biotechnol. (2024) 15:41. doi: 10.1186/s40104-024-00998-6
217. Chen, X, Li, Z, Pu, J, Cai, J, Zhao, H, Jia, G, et al. Dietary betaine improves the intestinal health and growth performance of heat-stressed growing rabbits in summer. J Anim Sci. (2023) 101:363. doi: 10.1093/jas/skad363
218. Hemarajata, P, and Versalovic, J. Effects of probiotics on gut microbiota: mechanisms of intestinal immunomodulation and neuromodulation. Ther Adv Gastroenterol. (2013) 6:39–51. doi: 10.1177/1756283X12459294
219. Maftei, NM, Raileanu, CR, Balta, AA, Ambrose, L, Boev, M, Marin, DB, et al. The potential impact of probiotics on human health: An update on their health-promoting properties. Microorganisms. (2024) 12:234. doi: 10.3390/microorganisms12020234
220. Thomas, CM, and Versalovic, J. Probiotics-host communication: modulation of signaling pathways in the intestine. Gut Microbes. (2010) 1:148–63. doi: 10.4161/gmic.1.3.11712
221. Cani, PD, and Delzenne, NM. The role of the gut microbiota in energy metabolism and metabolic disease. Curr Pharm Des. (2009) 15:1546–58. doi: 10.2174/138161209788168164
222. Choi, MY, Fitzpatrick, RD, Buhler, K, Mahler, M, and Fritzler, MJ. A review and meta-analysis of anti-ribosomal P autoantibodies in systemic lupus erythematosus. Autoimmun Rev. (2020) 19:102463. doi: 10.1016/j.autrev.2020.102463
223. Hajirezaee, S, Ramezani, S, and Ramezani, S. Betaine and the probiotic, Lactobacillus rhamnosus in the diet of the common carp, Cyprinus carpio: effects on growth, digestive enzyme activities, antioxidant system, humoral and mucosal immunity and resistance to Streptococcus iniae. Aquacult. Rep. (2024) 38:102282. doi: 10.1016/j.aqrep.2024.102282
224. Kerksick, C, and Willoughby, D. The antioxidant role of glutathione and N-acetyl-cysteine supplements and exercise-induced oxidative stress. J Int Soc Sports Nutr. (2005) 2:38–44. doi: 10.1186/1550-2783-2-2-38
225. Sen, CK, Khanna, S, Reznick, AZ, Roy, S, and Packer, L. Glutathione regulation of tumor necrosis factor-alpha-induced NF-kappa B activation in skeletal muscle-derived L6 cells. Biochem Biophys Res Commun. (1997) 237:645–9. doi: 10.1006/bbrc.1997.7206
226. Jewell, DE, and Jackson, MI. Dietary betaine interacts with very long chain n-3 polyunsaturated fatty acids to influence fat metabolism and circulating single carbon status in the cat. Animals. (2022) 12:2837. doi: 10.3390/ani12202837
227. Jewell, DE, Tavener, SK, Creech, R, and Panickar, KS. Betaine and L-carnitine synergistically influence the metabolome and immune response in dogs. Animals. (2024) 14:357. doi: 10.3390/ani14030357
228. Lattef, FA, and Khudair, KK. Effects of combination of betaine and Kaempferol on induced methionine overload in rats: lipid profile and oxidative stress. Rev. Latinoamericana Hipertensión. (2024) 19:183–90. doi: 10.5281/zenodo.11261767
229. Lee, SY, and Ko, KS. Effects of S-adenosylmethionine and its combinations with taurine and/or betaine on glutathione homeostasis in ethanol-induced acute hepatotoxicity. J Cancer Prev. (2016) 21:164–72. doi: 10.15430/JCP.2016.21.3.164
230. Lee, SY, and Ko, KS. Protective effects of S-adenosylmethionine and its combinations with taurine and/or betaine against lipopolysaccharide or polyinosinic-polycytidylic acid-induced acute hepatotoxicity. J Cancer Prev. (2016) 21:152–63. doi: 10.15430/JCP.2016.21.3.152
231. Li, Y, Yuan, J, Sun, S, Ma, F, Xiong, Y, and He, S. Optimizing growth and antioxidant function in heat-stressed broilers with vitamin C and betaine supplementation. Int J Biometeorol. (2024) 68:1953–60. doi: 10.1007/s00484-024-02717-2
232. Saleh, AA, El-Tahan, HM, Shaban, M, Morsy, WA, Genedy, S, Alzawqari, MH, et al. Effect of dietary supplementation of betaine and organic minerals on growth performance, serum biochemical parameters, nutrients digestibility, and growth-related genes in broilers under heat stress. Poult Sci. (2023) 102:103051. doi: 10.1016/j.psj.2023.103051
233. Sun, L, Tan, X, Liang, X, Chen, H, Ou, Q, Wu, Q, et al. Maternal betaine supplementation mitigates maternal high fat diet-induced NAFLD in offspring mice through gut microbiota. Nutrients. (2023) 15:15. doi: 10.3390/nu15020284
234. Augustine, PC, Mcnaughton, JL, Virtanen, E, and Rosi, L. Effect of betaine on the growth performance of chicks inoculated with mixed cultures of avian Eimeria species and on invasion and development of Eimeria tenella and Eimeria acervulina in vitro and in vivo. Poult Sci. (1997) 76:802–9. doi: 10.1093/ps/76.6.802
235. Wang, W, Liu, A, Chen, X, Zheng, X, Fu, W, Wang, G, et al. The potential role of betaine in enhancement of microbial-assisted phytoremediation of benzophenone-3 contaminated soil. Chemosphere. (2022) 307:135783. doi: 10.1016/j.chemosphere.2022.135783
236. Abu Hafsa, SH, Centoducati, G, Hassan, AA, Maggiolino, A, Elghandour, M, and Salem, AZM. Effects of dietary supplementations of vitamin C, organic selenium, betaine, and pomegranate Peel on alleviating the effect of heat stress on growing rabbits. Animals. (2024) 14:950. doi: 10.3390/ani14060950
237. Wahid, ZA, Pradista, LA, Prastowo, S, and Ratriyanto, A (2024). Betaine Alter SCFA-producing Bacteria population in laying pullet reared in tropical climate. IOP Conference Series: Earth and Environmental Science 1341.
238. Yang, W, Huang, L, Gao, J, Wen, S, Tai, Y, Chen, M, et al. Betaine attenuates chronic alcohol-induced fatty liver by broadly regulating hepatic lipid metabolism. Mol Med Rep. (2017) 16:5225–34. doi: 10.3892/mmr.2017.7295
239. Balkan, J, Oztezcan, S, Kucuk, M, Cevikbas, U, Kocak-Toker, N, and Uysal, M. The effect of betaine treatment on triglyceride levels and oxidative stress in the liver of ethanol-treated guinea pigs. Exp Toxicol Pathol. (2004) 55:505–9. doi: 10.1078/0940-2993-00347
240. Kanbak, G, Arslan, OC, Dokumacioglu, A, Kartkaya, K, and Inal, ME. Effects of chronic ethanol consumption on brain synaptosomes and protective role of betaine. Neurochem Res. (2008) 33:539–44. doi: 10.1007/s11064-007-9472-0
241. Oliva, J, Bardag-Gorce, F, Tillman, B, and French, SW. Protective effect of quercetin, EGCG, catechin and betaine against oxidative stress induced by ethanol in vitro. Exp Mol Pathol. (2011) 90:295–9. doi: 10.1016/j.yexmp.2011.02.006
242. Kwon, DY, Jung, YS, Kim, SJ, Park, HK, Park, JH, and Kim, YC. Impaired sulfur-amino acid metabolism and oxidative stress in non-alcoholic fatty liver are alleviated by betaine supplementation in rats. J Nutr. (2009) 139:63–8. doi: 10.3945/jn.108.094771
243. Du, J, Shen, L, Tan, Z, Zhang, P, Zhao, X, Xu, Y, et al. Betaine supplementation enhances lipid metabolism and improves insulin resistance in mice fed a high-fat diet. Nutrients. (2018) 10:131. doi: 10.3390/nu10020131
244. Li, L, Sun, L, Liang, X, Ou, Q, Tan, X, Li, F, et al. Maternal betaine supplementation ameliorates fatty liver disease in offspring mice by inhibiting hepatic NLRP3 inflammasome activation. Nutr Res Pract. (2023) 17:1084–98. doi: 10.4162/nrp.2023.17.6.1084
245. Abu Ahmad, N, Raizman, M, Weizmann, N, Wasek, B, Arning, E, Bottiglieri, T, et al. Betaine attenuates pathology by stimulating lipid oxidation in liver and regulating phospholipid metabolism in brain of methionine-choline-deficient rats. FASEB J. (2019) 33:9334–49. doi: 10.1096/fj.201802683R
246. Veskovic, M, Mladenovic, D, Milenkovic, M, Tosic, J, Borozan, S, Gopcevic, K, et al. Betaine modulates oxidative stress, inflammation, apoptosis, autophagy, and Akt/mTOR signaling in methionine-choline deficiency-induced fatty liver disease. Eur J Pharmacol. (2019) 848:39–48. doi: 10.1016/j.ejphar.2019.01.043
247. Veskovic, M, Labudovic-Borovic, M, Mladenovic, D, Jadzic, J, Jorgacevic, B, Vukicevic, D, et al. Effect of betaine supplementation on liver tissue and ultrastructural changes in methionine-choline-deficient diet-induced NAFLD. Microsc Microanal. (2020) 26:997–1006. doi: 10.1017/S1431927620024265
248. Abdelmalek, MF, Angulo, P, Jorgensen, RA, Sylvestre, PB, and Lindor, KD. Betaine, a promising new agent for patients with non-alcoholic steatohepatitis: results of a pilot study. Am J Gastroenterol. (2001) 96:2711–7. doi: 10.1111/j.1572-0241.2001.04129.x
249. Abdelmalek, MF, Sanderson, SO, Angulo, P, Soldevila-Pico, C, Liu, C, Peter, J, et al. Betaine for non-alcoholic fatty liver disease: results of a randomized placebo-controlled trial. Hepatology. (2009) 50:1818–26. doi: 10.1002/hep.23239
250. Miglio, F, Rovati, LC, Santoro, A, and Setnikar, I. Efficacy and safety of oral betaine glucuronate in non-alcoholic steatohepatitis. A double-blind, randomized, parallel-group, placebo-controlled prospective clinical study. Arzneimittelforschung. (2000) 50:722–7.
251. Steenge, GR, Verhoef, P, and Katan, MB. Betaine supplementation lowers plasma homocysteine in healthy men and women. J Nutr. (2003) 133:1291–5. doi: 10.1093/jn/133.5.1291
252. Smolin, LA, Benevenga, NJ, and Berlow, S. The use of betaine for the treatment of homocystinuria. J Pediatr. (1981) 99:467–72. doi: 10.1016/S0022-3476(81)80352-6
253. Kim, SK, and Kim, YC. Attenuation of bacterial lipopolysaccharide-induced hepatotoxicity by betaine or taurine in rats. Food Chem Toxicol. (2002) 40:545–9. doi: 10.1016/S0278-6915(01)00102-8
254. Park, SO, and Kim, WK. Effects of betaine on biological functions in meat-type ducks exposed to heat stress. Poult Sci. (2017) 96:1212–8. doi: 10.3382/ps/pew359
255. Ali, BH, Al-Qarawi, AA, and Mousa, HM. Stress associated with road transportation in desert sheep and goats, and the effect of pretreatment with xylazine or sodium betaine. Res Vet Sci. (2006) 80:343–8. doi: 10.1016/j.rvsc.2005.07.012
256. Qu, Y, Zhang, K, Pu, Y, Chang, L, Wang, S, Tan, Y, et al. Betaine supplementation is associated with the resilience in mice after chronic social defeat stress: a role of brain-gut-microbiota axis. J Affect Disord. (2020) 272:66–76. doi: 10.1016/j.jad.2020.03.095
Keywords: betaine, liver, alcohol-associated liver disease, metabolic dysfunction-associated steatotic liver disease, gut-liver axis, intestinal barrier integrity, gut microbiome
Citation: Perumal SK, Arumugam MK, Osna NA, Rasineni K and Kharbanda KK (2025) Betaine regulates the gut-liver axis: a therapeutic approach for chronic liver diseases. Front. Nutr. 12:1478542. doi: 10.3389/fnut.2025.1478542
Edited by:
Jingjing Du, Max Planck Institute for Heart and Lung Research, GermanyReviewed by:
Qi Wang, Southern Medical University, ChinaSilvio Pires Gomes, University of São Paulo, Brazil
Copyright © 2025 Perumal, Arumugam, Osna, Rasineni and Kharbanda. This is an open-access article distributed under the terms of the Creative Commons Attribution License (CC BY). The use, distribution or reproduction in other forums is permitted, provided the original author(s) and the copyright owner(s) are credited and that the original publication in this journal is cited, in accordance with accepted academic practice. No use, distribution or reproduction is permitted which does not comply with these terms.
*Correspondence: Kusum K. Kharbanda, a2toYXJiYW5kYUB1bm1jLmVkdQ==
†These authors share first authorship
‡These authors share senior authorship