- 1Department of Critical Care Medicine of the Third Affiliated Hospital (The First People's Hospital of Zunyi), Zunyi Medical University, Zunyi, China
- 2Department of Nursing of Affiliated Hospital, Zunyi Medical University, Zunyi, China
- 3Department of Molecular and Cellular Biology, Baylor College of Medicine, Houston, TX, United States
- 4Department of Anesthesiology, The Second Affiliated Hospital, Hengyang Medical School, University of South China, Hengyang, China
- 5Department of Critical Care Medicine, The Second Affiliated Hospital, Hengyang Medical School, University of South China, Hengyang, China
Background: Sepsis is the body’s extreme response to an infection leading to organ dysfunction. Sepsis-related acquired weakness (SAW), a critical illness closely related to metabolic disorders, is characterized by generalized sepsis-induced skeletal muscle weakness, mainly manifesting as symmetrical atrophy of respiratory and limb muscles. Muscle accounts for 40% of the body’s total mass and is one of the major sites of glucose and energy absorption. Diet affects skeletal muscle metabolism, which further impacts physiology and signaling pathways. The ketogenic diet (KD) is a high-fat, low-carbohydrate diet that has shown benefits in patients with a variety of neuromuscular disorders. Patients with SAW are in a hypermetabolic state and can consume approximately 1% of total body muscle mass in a day. Due to the decreased total body energy expenditure secondary to starvation, skeletal muscles enter a low metabolic state, with reduced gluconeogenesis and protein consumption and elevated levels of ketone bodies. The latest research suggests that KD may be a new strategy for SAW prevention and treatment, but its mechanism is still unclear.
Objective: Our article aims to explore the effect and mechanism of KD on SAW. And we hope that our review will inspire further research on the KD and foster the exploration of novel strategies for combating SAW.
Methods: Search medical databases and related academic websites, using keywords such as “Sepsis-related acquired weakness,” “ketogenic diet,” and “skeletal muscle,” and select representative literature. Using the method of induction and summary, analyze the effect and mechanism of KD on SAW.
Results: Compared with early nutrition, KD has a more protective effect on SAW, but its mechanism is complex. Firstly, KD can alter energy metabolism substrates to affect SAW’s energy metabolism; Secondly, KD can directly act as a signaling molecule to improve mitochondrial function in skeletal muscle and stimulate skeletal muscle regeneration signaling molecules; Thirdly, KD can affect the gut microbiota to exert anti-inflammatory effects, enhance immunity, and thus protect SAW.
Conclusion: KD has a protective effect on SAW, which includes improving energy metabolism, stimulating muscle regeneration signals, optimizing gut microbiota composition, and reducing inflammation and oxidative stress.
Introduction
ICU-acquired weakness (ICU-AW) is a neuromuscular dysfunction that has no other reasonable etiology besides critical illness and its treatment (1). ICU-acquired weakness is typically generalized, symmetrical, and affects limb (proximal more than distal) and respiratory muscles, whereas facial and ocular muscles are spared (2). According to statistics, globally, about 13–20 million patients receive life-supporting treatment in ICUs every year, and more than 1 million critically ill patients experience ICU-AW each year (3). Comorbid sepsis, prolonged motor inhibition, the application of mechanical ventilation, and malnutrition are risk factors for ICU-AW in critically ill patients (4), among which sepsis is an independent risk factor for ICU-AW. Up to 60–100% of patients with sepsis develop ICU-AW, which is known as sepsis-related acquired weakness (SAW) (5). SAW is a critical illness with unique metabolisms (6), for which there has been no effective clinical treatment (6). It often results in prolonged mechanical ventilatory support and hospitalization, increasing patient mortality and seriously affecting patients’ quality of life after discharge (7). Therefore, there is an urgent need for effective measures to prevent the occurrence and development of SAW, and to improve the survival rate and quality of life of critically ill patients.
Muscle accounts for 40% of the body’s total mass and is the primary site of glucose and energy absorption. For organisms that have high energy requirements, sufficient adenosine triphosphate (ATP) production is essential for muscle contraction and the maintenance of muscle function (8). Variations in diets affect not only muscle metabolism (9) but also signaling pathways for growth, survival, and other functions in skeletal muscle cells. Metabolic flexibility in response to a variety of stimuli, including dietary changes, is an important feature of muscle energetics. Changes in dietary metabolism impact muscle tissues not only through energy supply but also through metabolic intermediates. Specific changes in metabolism, such as activation of ketogenic metabolism, may have protective effects against SAW (10).
The ketogenic diet (KD) is formulated with a high percentage of fats, a low percentage of carbohydrates, and adequate proteins and other nutrients. It has been used for more than 100 years since its initial introduction in 1921 by Dr. Wilder at the Mayo Clinic (11). The KD, which was originally used for controlling epilepsy, especially hard-to-control epilepsy (12), is now being used in the management of obesity (13), polycystic ovary syndrome (14), cancer (15), diabetes mellitus (16), and traumatic brain injury (17), as well as amyotrophic lateral sclerosis (18), mitochondrial myopathies (19), primary sarcopenia (20), and other pathologic conditions (21). Recent studies have demonstrated that the KD may be a novel strategy for the treatment of SAW (22), but the mechanism is still unclear. In addition, KD has great plasticity in the prevention and treatment of muscle atrophy, but a lot of work is needed to clarify the overall situation. Therefore, this review will review the role and mechanism of KD in SAW, providing new methods and avenues for the prevention or treatment of SAW.
The KD and early nutritional support
The KD
The main types of KDs include the classic KD, the medium-chain triglyceride KD, the modified Atkins diet, and the low-blood-sugar KD. The classic KD: Fat accounts for 70–75% of total calories, protein accounts for 20–25%, carbohydrates account for about 5%. Strictly controlling the intake of carbohydrates can promote the body to enter a ketotic state, relying on fat metabolism to produce ketone bodies for energy supply, which can effectively control epileptic seizures, especially for children with refractory epilepsy; The medium-chain triglyceride KD: emphasizes increasing the intake of medium chain triglycerides (MCT), which can be quickly absorbed by the body and converted into ketone bodies. Compared to ordinary fats, MCT is more likely to produce ketone bodies, making it more suitable for people who need to quickly enter a ketogenic state, such as patients with certain neurological diseases; The modified Atkins diet: Compared to the classic ketogenic diet, the restrictions on carbohydrates are relatively relaxed, allowing for a daily intake of 10–20 grams of net carbohydrates. The fat ratio is relatively reduced, and the protein ratio can be appropriately increased. This is suitable for epilepsy patients who are unwilling to accept a strict ketogenic diet and may also have certain benefits in weight loss and improving metabolic indicators; The low-blood-sugar KD: Pay attention to the glycemic index (GI) of food, choose low GI foods with a balanced ratio of fat and protein, which helps stabilize blood sugar levels, reduce blood sugar fluctuations, and improve insulin sensitivity to some extent (23). After metabolizing the KD in vivo, the levels of ketone bodies (KBs) (i.e., acetoacetic acid, β-hydroxybutyric acid [β-HB], and acetone) and fatty acids significantly increase. The synthesis of KBs, also known as ketogenesis, predominantly occurs in hepatocytes and less in astrocytes or renal cells (24). KB utilization occurs in the heart, skeletal muscles, and brain (Figure 1) (25). When there is a deficiency or decrease in dietary carbohydrates, the plasma insulin level decreases while the glucagon level increases, thereby promoting hepatic glycogenolysis and gluconeogenesis, as well as lipolysis in adipose tissue, which is mediated by hormone-sensitive lipase. Restricting carbohydrate intake for 4–7 days leads to failed glycogenolysis and increased ketogenesis with elevated levels of free fatty acids (FFA), acetyl coenzyme A (acetyl-CoA), and KBs (26). When glucose levels are low or carbohydrate consumption is insufficient, KBs become the primary source of energy and mediate cell signaling, post-translational modifications, inflammation, oxidative stress, and the synthesis of lipids like myelin and cholesterol (27).
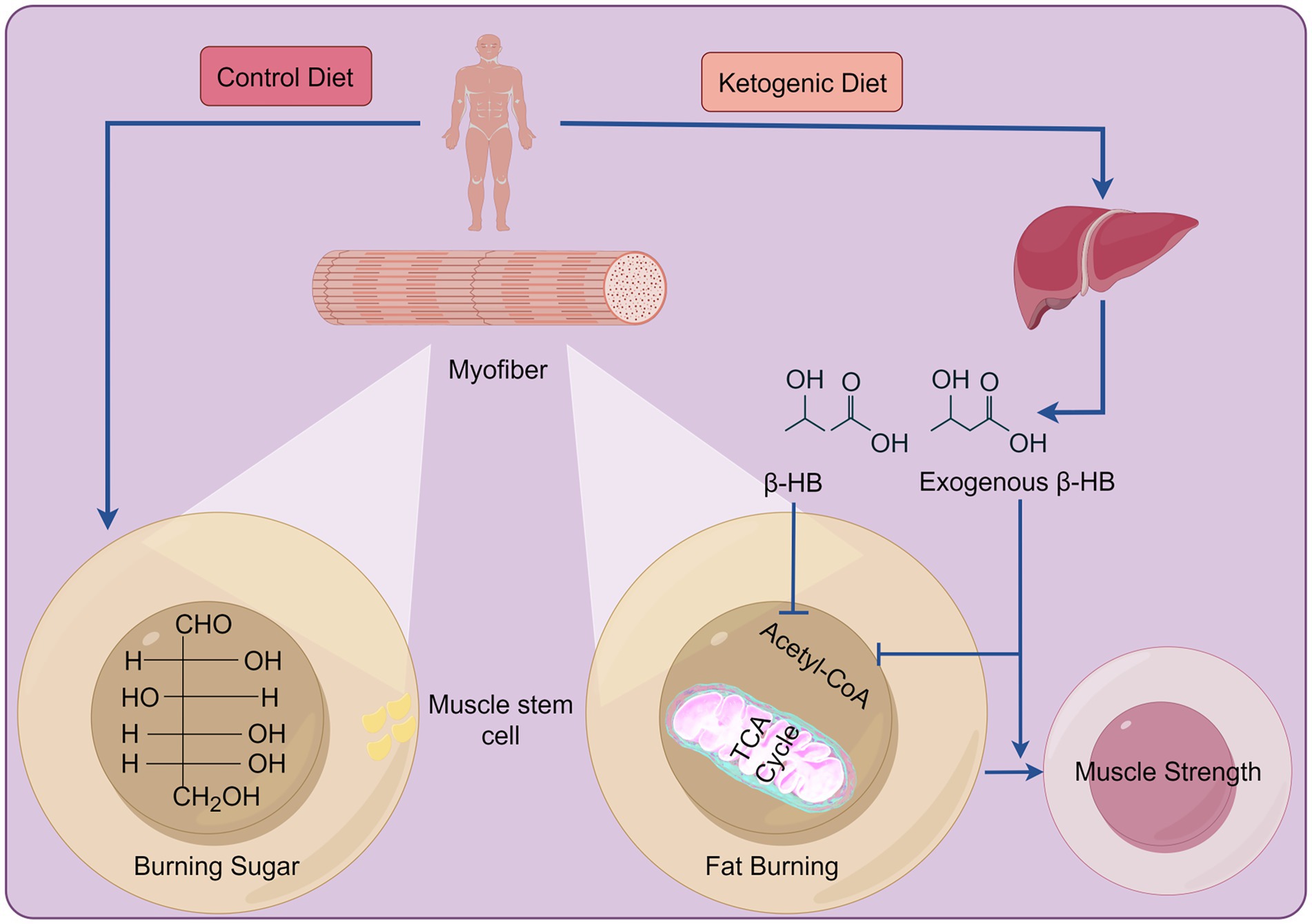
Figure 1. The differences between the KD and a standard diet in energy production. In a standard diet, carbohydrates serve as the primary energy source post-consumption. In contrast, the KD mainly relies on KBs for energy production. These ingested KBs, alongside the ones produced by the liver, enter the tricarboxylic acid cycle to generate energy. Created with Figdraw.com.
Early nutritional support
Inflammatory mediators can potently induce catabolism during sepsis and play a key role in catabolizing muscle proteins and driving lipolysis in adipocytes. Endogenous proteolysis associated with inflammation in skeletal muscles can rapidly progress to SAW (28). Early nutrition for critically ill patients refers to providing nutrition to patients in the early stages (within 48 h) after the onset of critical illness (29). Traditionally, providing patients with 80% full-calorie and protein (1.2–2.0 g/kg/d) feeds during the first week in the ICU optimizes their outcomes (30). However, the recent CALORIES and NUTRIREA-2 trials have shown that early nutritional support (enteral versus parenteral nutrition) does not alter the survival rate (31), and intentional undernutrition (low-volume enteral nutrition) in the first week of ICU stay has the same effects as early nutritional support (32). In addition, early nutritional support may create an extra metabolic burden, leading not only to higher blood glucose levels, aggravated infections (33), and adverse gastrointestinal reactions, but also to increased mechanical ventilation dependence, prolonged ICU stays, and hindered recovery from SAW (34). Overall, these studies have shown that early nutritional support in SAW is ineffective and may even cause harm (Table 1).
The most recent clinical guidelines for intensive care recommend a protein intake of 1.2–2.0 g/kg/day (30), which is associated with lower mortality rates (35). A protein intake that is higher than the recommended level is associated with negative outcomes, such as delayed recovery (33). Meta-analyses have shown that high-protein nutrition does not benefit critically ill patients (36). An observational study showed that a high protein diet only benefits the survival of non-septic patients and tends to increase mortality in septic patients (37). This is because a high-protein intake can not only lead to hypernatremia (38) but can also inhibit autophagy (39). Autophagy is a cell-regulating mechanism that clears unfolded or misfolded proteins and damaged organelles, allowing for nutrient recycling and cell survival. In a mouse model of SAW, autophagy was shown to have a protective effect that improves survival and organ function (40). Inhibition of autophagy aggravates SAW, and enhancement of autophagy ameliorates SAW (41). Thus, focusing on nitrogen balance alone to guide dietary protein interventions may delay SAW recovery. The dietary protein intake level needs to be further determined based on the type of disease, the severity of protein catabolism, the energy state, and the timing and dosage of supplementation. However, KD has been shown to enhance autophagy in skeletal muscle (42), and ketolipids can treat sepsis and prevent saws (43).
It is generally believed that carbohydrates are one of the main sources of energy for the human body and play an important role in the normal function of skeletal muscles. Patients with sepsis manifest a significant shift in overall metabolism from glucose oxidation to fatty acid oxidation (FAO), with critically ill patients having a lower level of glucose oxidation (44) and enhanced lipid metabolism (45). Significant differences in glucose metabolism and fatty acid β-oxidation pathways were found between sepsis survivors and non-survivors (46). The mortality rate of sepsis patients with high blood sugar is increased (47). Research has found that high carbohydrate nutrition increases the susceptibility of drosophilae to certain bacterial infections. Drosophilae with high carbohydrate nutrition may develop hyperglycemia, and some pathogens may use excess sugar in the host’s body to promote growth during infection (48). In addition, obese mice fed high carbohydrate diets can exacerbate skeletal muscle damage in sepsis (49). The commercially available enteral feeds are often very high in carbohydrates. Many intravenous drugs are administered as glucose-containing solutions. Parenteral nutrition solutions also contain large amounts of glucose. The resulting high carbohydrate load leads to an increased requirement for insulin and the inhibition of FAO and ketogenesis (50). Therefore, alternative dietary management for patients with SAW may include limiting the glycemic load or changing the dietary composition to allow sufficient fasting time to enhance FAO and ketogenesis. A study found that sepsis patients receiving KD improved hyperglycemia, achieved stable ketosis, and reduced immune dysregulation compared to those receiving high carbohydrate nutrition, and were associated with improvements in clinical indicators (51).
Intermittent fasting is a major mechanism for promoting longevity and mitigating diseases (52). It can effectively activate cytoprotective and cell-repairing pathways, including autophagy, mitochondrial biogenesis, and antioxidant defense (53). However, prolonged fasting ultimately comes at the cost of weight loss (54). Therefore, the beneficial pathways facilitated by fasting can be similarly activated through modified nutritional strategies, such as ketone supplementation (55). The finding that obese patients with sepsis have a lower incidence of SAW than lean patients with sepsis suggests that obesity has a protective effect on SAW, which may be attributed to the presence of KBs (22). Plasma concentrations of β-HB, a type of KB, are increased in pediatric mice with early childhood macronutrient deficiencies in the ICU (56). In a mouse model of sepsis, β-HB treatment was shown to ameliorate SAW (22), suggesting that it has a direct effect on muscle strength. However, the protective effects of the KD or KBs are not solely attributed to their role as energy substrates but also to their function as signaling molecules that impact regenerative pathways (57).
Mechanism of action of the KD on SAW
Energy metabolism
Skeletal muscles require energy for various activities (58), and ATP is required to support muscle contraction. Typically, the majority of ATP is generated through mitochondrial respiration, with anaerobic glycolysis contributing to ≤2% of total ATP production. SAW can lead to systemic hypoxia and inflammation, increased reactive oxygen species (ROS), impaired glucose and fatty acid utilization, diminished mitochondrial number and function (59, 60), increased ATP demand, and elevated adenosine monophosphate kinase (AMPK) (60) (Figure 1).
Carbohydrates are the preferred substrate for energy production under healthy conditions. However, in the critically ill population, carbohydrates can cause hyperglycemia, increase mitochondrial oxygen consumption, and increase ROS production, thereby triggering a vicious cycle of mitochondrial damage (61). The impaired translocation of glucose transporter-4 and increased insulin resistance both exacerbate the deleterious effects of hyperglycemia (62). In addition, the metabolic characteristics of SAW patients are impaired ketone production and reduced fatty acid metabolism in the liver and muscles (22). Research has found that in SAW patients, β-HB is preferentially absorbed by muscles and metabolized into cholesterol precursor mevalonate, rather than TCA metabolites (57). Fat, as an energy substrate, can alter muscle substrate metabolism, from using glycogen to β-HB (63). KD treatment of SAW can increase the release and metabolism of fatty acids into ketone bodies, thereby preventing and treating SAW (22).
Pyruvate is transferred to mitochondria under aerobic conditions and oxidized to acetyl-CoA via the pyruvate dehydrogenase complex (PDHC) to accelerate aerobic oxidation. The PDHC activity of mononuclear cells in the peripheral blood was significantly lower in patients with sepsis than in healthy controls (64). In addition, rats with sepsis had a 70% reduction in PDHC activity and decreased acetyl-CoA production in skeletal muscle cells, resulting in hypoxia and dysfunction in skeletal muscle cells (Figure 2) (65). KD has been shown to increase pyruvate dehydrogenase levels (66). In mice fed with a KD, pyruvate was oxidatively decarboxylated to acetyl-CoA, catalyzed by pyruvate dehydrogenase (67), and the KD led to increased levels of pyruvate in skeletal muscles (68).
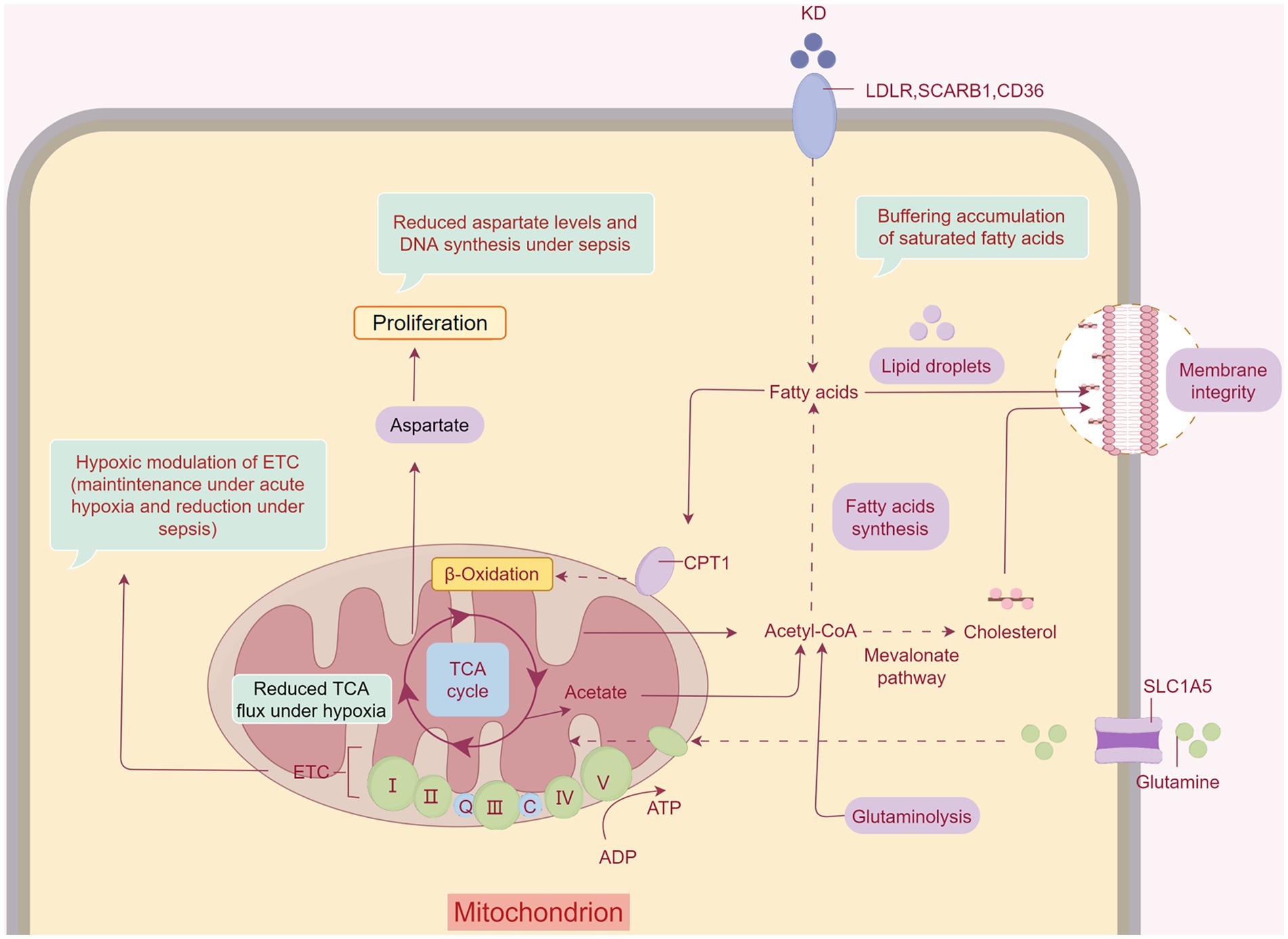
Figure 2. The KD ameliorates SAW by changing the energy-supplying pattern. The KD ameliorates inflammation and hypoxia in muscles during sepsis. Fatty acids provide direct relief from SAW. KBs also have therapeutic effects on SAW by affecting the tricarboxylic acid cycle and subsequently enhancing mitochondrial function. Created with Figdraw.com.
Muscle signaling molecules
Wallace et al. demonstrated the efficacy of the long-term application of the KD in alleviating sarcopenia (20). The KD increases body weight and fat mass (69) but does not change body mass or muscle mass in mice (70). This may be related to its role in providing signaling molecules rather than being solely a metabolic substrate (43). The KD not only promotes the conversion of type IIb skeletal muscle fibers to type IIa fibers and increases the levels of neuromuscular junction remodeling markers, but also facilitates mitochondrial biogenesis, reduces endoplasmic reticulum stress, and enhances protein synthesis and proteasome activity (20). In addition, the KD upregulates genes related to muscle atrophy, such as Mafbx, Murf1, Foxo3, Lc3b, and Klf15, to reduce muscle mass, fiber cross-sectional area, and grip strength (67).
In humans, a KD can modulate tryptophan metabolism, thereby increasing mitoprotective metabolites. In addition, the anti-inflammatory fatty acids eicosatetraenoic acid and docosahexaenoic acid may be increased. During a KD, there may be increased utilization of carnitine and changes in the tryptophan pathway with decreased quinolinic acid and increased kynurenic acid concentrations. Kynurenic acid is thought to have protective effects on mitochondrial respiration, whereas accumulation of quinolinic acid is associated with mitochondrial dysfunction (71).
Animal studies have found that the administration of β-HB in combination with parenteral nutrition reduces muscle weakness (43). Moreover, β-HB was shown to increase the levels of muscle regeneration markers and decrease the expression of histone deacetylase (HDAC) 4 and 5, which are inhibitors of the muscle regeneration pathways (43). β-OHB injections in healthy adults reduce leucine oxidation and enhance the incorporation of leucine into the skeletal muscles (72). In addition, supplementing SAW mice with β-HB increased markers of early muscle regeneration and reduced the expression of class IIa histone deacetylases (22), which are known factors that inhibit the regeneration pathway by suppressing myocyte enhancer factor 2 (73).
Immunity and the gut microbiota
Immunometabolism plays a critical role in host defense (74). The metabolic determinants of the host response are complex and specific to the types of infection and immune cells involved. A patient’s nutritional status alters their gut microbiota and intestinal integrity, affecting inflammation and their host response to sepsis (75). Therefore, it is necessary to consider the balance of these potentially conflicting metabolic demands when designing nutritional interventions.
Sepsis changes the gut microbiota and metabolites. Specifically, the beneficial microorganisms are replaced by the pathogenic ones (76). The gut microbiota is closely intertwined with the host immune system (77) and serves an essential function in host metabolism and resistance against pathogen colonization (78). The disturbance of the gut microbiota occurs in a variety of diseases, including infections and sepsis (79). The gut microbiota may potentially link the immune system and sepsis (80). Recent preclinical evidence has suggested a complex crosstalk between the gut microbial environment and skeletal muscles (81). Lack of gut microbiota found in animal studies can lead to muscle mass loss (82). An increase in the Rikenellaceae of elderly mice was found to be associated with muscle atrophy due to the presence of Riederia bacteria (83). In addition, compared with healthy adult rats, muscle atrophy rats have a higher ratio of Sutterella to Barnesiella, reduced size of gastrocnemius and triceps, and altered immune function (84). Luckily, the KD can maintain gut microbiota homeostasis. For instance, it increases the beneficial gut microbiota, such as Akkermansia muciniphila and Lactobacillus (85). Another study in mice and humans found that a KD led to a reduction in Bifidobacterium and a decrease in pro-inflammatory Th17 cells in the gut and visceral fat (86). In addition, a KD maintains immune cell homeostasis and promotes cell survival after bacterial infections (86). For instance, it promotes macrophage polarization (87), resulting in a 50% increase in M2-type macrophages and a 50% decrease in M1-type macrophages (88).
Inflammation and gut microbiota
Persistent inflammation is one of the main mechanisms leading to the loss of skeletal muscle mass and function. In the clinical-translational setting, the fundamental importance of ketogenic metabolism for human T cells has already been demonstrated in the context of critically ill COVID-19 patients and, in particular, the improvement of T cell immune metabolism by ketone bodies has been demonstrated in compromised T cells of intensive care patients (89). Due to the profound change in macronutrient composition, a ketogenic diet also has significant effects on the cerebrospinal fluid metabolome, which indirectly exerts additional modulating influences on immune cell populations (90).
Intestinal disorders and the loss of gut microbiota diversity impair the integrity of the intestinal barrier and can allow harmful microbial products, such as lipopolysaccharides, to enter the bloodstream, leading to systemic inflammation and metabolic disorders, which can weaken muscle function and reduce muscle mass (91) (Figure 3). The gut microbiota promotes metabolic homeostasis and immune function by strengthening the intestinal barrier. The loss of gut microbiota diseases and diversity can damage the integrity of the intestinal barrier, allowing harmful microbial products such as lipopolysaccharides (LPS) to enter the bloodstream. These harmful substances can cause systemic inflammation, leading to metabolic disorders and decreased muscle function and quality (91). When the intestinal epithelial barrier function is impaired, under lipopolysaccharide stimulation, nuclear factor kappa B (NF-kB) translocates from the cytoplasm to the nucleus, and stimulates dendritic cells and macrophages to produce pro-inflammatory cytokines and mediators, such as cyclooxygenase-2, TNF-α, inducible nitric oxide synthase, and IL-6, which regulate intestinal and systemic inflammation (92). And TNF-α regulates the activation of NF-kB signaling pathway by expressing atrophy related genes, and promotes protein degradation through transcription of ubiquitin proteasome E3 ligase (93). It was reported that the expression level of SOCS3, the target gene of IL-6, was elevated in the skeletal muscles of patients with SAW and that L-6 mediates sepsis-induced muscle atrophy through the gp130/JAK2/STAT3 pathway (94). Research has found that KD not only alleviates TNF α-induced apoptosis and inflammation of intestinal cells (95), but also reduces the increase in IL-6 levels caused by gut microbiota disorders (96).
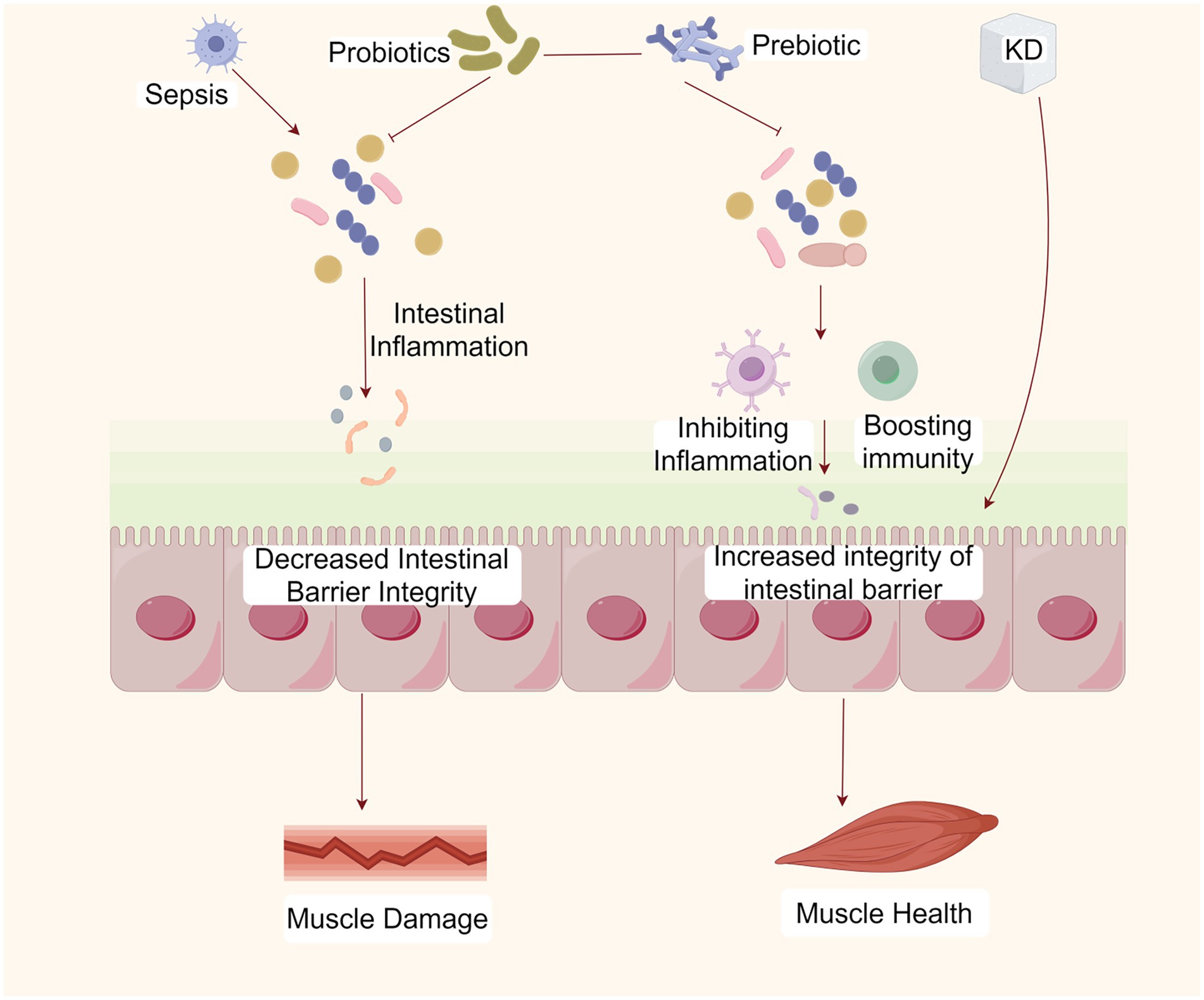
Figure 3. The KD ameliorates SAW by maintaining gut microbiota homeostasis. The KD can maintain the homeostasis of the gut microbiota, the integrity of the intestinal barrier, and immune homeostasis, thereby inhibiting inflammation and ameliorating SAW. Created with Figdraw.com.
Insulin resistance and reduction in skeletal muscles are associated with increased Toll-like receptor 4 (TLR4) expression and signaling in an aging model, which may be due to the development of secondary endotoxemia (97). Activation of the TLR4 signaling pathway results in significant increases in the NF-κB protein level and the phosphorylation level of c-Jun N-terminal kinase (97). The TLR4 signaling pathway can also induce a systemic inflammatory response by upregulating pro-inflammatory cytokine (IL-6 and TNF-α) levels through a cascade reaction (98). These inflammatory cytokines are involved in regulating muscle atrophy. Inhibition of the production of these inflammatory cytokines by modulating the gut microbiota can alleviate muscle atrophy (99). Many studies have now confirmed that a KD improves the inflammatory environment by regulating the gut microbiota (Table 2). A KD upregulates antioxidant and anti-inflammatory pathways (100). For example, Ketones are anti-inflammatory and can suppress chronic low-grade inflammation by inhibiting the NLRP3 inflammasome (101). At the same time, however, the antiviral immune response of gamma/delta T cells is enhanced by a ketogenic diet, and the use of ketone bodies has been postulated as an antiviral therapy option (102). And β-HB inhibits the formation of NLRP3 inflammatory vesicles and prevents the release of pro-inflammatory cytokines (103).
Conclusion and outlook
SAW involves unique metabolic entities compared to other critical illnesses. Therefore, optimal metabolic and nutritional management strategies may differ between critically ill patients with SAW and non-SAW. Increasing evidence suggests that transitioning from a carbohydrate-centric metabolism to one that prioritizes lipid metabolism may offer protective effects against SAW. This involves mechanisms like maintaining mitochondrial homeostasis, producing anti-inflammatory effects, and regulating immune homeostasis and gut microbiota. However, the benefits of a KD on SAW remain controversial and need validation with further clinical and basic studies. In addition, this article is a narrative review and lacks a systematic retrieval strategy in the literature collection process, requiring more systematic evidence summary and analysis.
Author contributions
YM: Conceptualization, Data curation, Investigation, Methodology, Project administration, Software, Supervision, Writing – original draft, Writing – review & editing. LX: Conceptualization, Methodology, Project administration. SC: Project administration, Supervision, Writing – review & editing. XZ: Investigation, Writing – original draft. WL: Data curation, Resources, Writing – review & editing. PX: Formal analysis, Funding acquisition, Supervision, Writing – review & editing.
Funding
The author(s) declare that financial support was received for the research, authorship, and/or publication of this article. This work was supported by the National Natural Science Foundation (Grant Nos. 82060359 and 82360382) of China; Guizhou Province Social Development Project: Qiankehe [2021] General 088; Key Project of Guizhou Natural Science Foundation: Qiankehe Fundamentals ZK [2022] Key 049; Guizhou Province Excellent Youth Science and Technology Talent Project: Qiankehe Platform Talent [2021] No. 5648. Hunan innovation platform and talent plan: 2023SK4014.
Acknowledgments
The authors would like to thank Xiaoming Zhang for his help in reviewing and proofreading this paper. The authors acknowledge the use of Figdraw (www.figdraw.com) to create all figures.
Conflict of interest
The authors declare that the research was conducted in the absence of any commercial or financial relationships that could be construed as a potential conflict of interest.
Publisher’s note
All claims expressed in this article are solely those of the authors and do not necessarily represent those of their affiliated organizations, or those of the publisher, the editors and the reviewers. Any product that may be evaluated in this article, or claim that may be made by its manufacturer, is not guaranteed or endorsed by the publisher.
Abbreviations
SAW, Sepsis-related acquired weakness; KD, Ketogenic diet; ICU-AW, ICU-acquired weakness; ATP, Adenosine triphosphate; MCT, Medium chain triglycerides; GI, Glycemic index; KB, Ketone bodies; FFA, Free fatty acid; acetyl-CoA, Acetyl coenzyme A; FAO, Fatty acid oxidation; ROS, Reactive oxygen species; AMPK, Adenosine monophosphate kinase; TCA, Tricarboxylic acid cycle; PDHC, Pyruvate dehydrogenase complex; HDAC, Histone deacetylase; COVID-19, Corona Virus Disease 2019; NF-kB, Nuclear factor kappa B; TLR4, Toll-like receptor 4; NLRP3, NLR family, pyrin domain containing protein 3; β-HB, β-hydroxybutyric.
References
1. Stevens, RD, Marshall, SA, Cornblath, DR, Hoke, A, Needham, DM, de Jonghe, B, et al. A framework for diagnosing and classifying intensive care unit-acquired weakness. Crit Care Med. (2009) 37:S299–308. doi: 10.1097/CCM.0b013e3181b6ef67
2. Piva, S, Fagoni, N, and Latronico, N. Intensive care unit-acquired weakness: unanswered questions and targets for future research. F1000Res. (2019):F1000 Faculty Rev-508:8. doi: 10.12688/f1000research.17376.1
3. Fan, E, Cheek, F, Chlan, L, Gosselink, R, Hart, N, Herridge, MS, et al. An official American Thoracic Society clinical practice guideline: the diagnosis of intensive care unit-acquired weakness in adults. Am J Respir Crit Care Med. (2014) 190:1437–46. doi: 10.1164/rccm.201411-2011ST
4. Ozdemir, M, Bomkamp, MP, Hyatt, HW, Smuder, AJ, and Powers, SK. Intensive care unit acquired weakness is associated with rapid changes to skeletal muscle Proteostasis. Cells (Basel). (2022) 11:4005. doi: 10.3390/cells11244005
5. Mitobe, Y, Morishita, S, Ohashi, K, Sakai, S, Uchiyama, M, Abeywickrama, H, et al. Skeletal muscle index at intensive care unit admission is a predictor of intensive care unit-acquired weakness in patients with Sepsis. J Clin Med Res. (2019) 11:834–41. doi: 10.14740/jocmr4027
6. Koutroulis, I, Batabyal, R, McNamara, B, Ledda, M, Hoptay, C, and Freishtat, RJ. Sepsis Immunometabolism: from defining Sepsis to understanding how energy production affects immune response. Crit Care Explor. (2019) 1:e61:e0061. doi: 10.1097/CCE.0000000000000061
7. Tortuyaux, R, Davion, JB, and Jourdain, M. Intensive care unit-acquired weakness: questions the clinician should ask. Rev Neurol (Paris). (2022) 178:84–92. doi: 10.1016/j.neurol.2021.12.007
8. Chadt, A, and Al-Hasani, H. Glucose transporters in adipose tissue, liver, and skeletal muscle in metabolic health and disease. Pflugers Arch. (2020) 472:1273–98. doi: 10.1007/s00424-020-02417-x
9. Yakupova, EI, Bocharnikov, AD, and Plotnikov, EY. Effects of ketogenic diet on muscle metabolism in health and disease. Nutrients. (2022) 14:14. doi: 10.3390/nu14183842
10. Zhu, H, Bi, D, Zhang, Y, Kong, C, Du, J, Wu, X, et al. Ketogenic diet for human diseases: the underlying mechanisms and potential for clinical implementations. Signal Transduct Target Ther. (2022) 7:11. doi: 10.1038/s41392-021-00831-w
12. Wells, J, Swaminathan, A, Paseka, J, and Hanson, C. Efficacy and safety of a ketogenic diet in children and adolescents with refractory epilepsy—a review. Nutrients. (2020) 12:1809. doi: 10.3390/nu12061809
13. Bruci, A, Tuccinardi, D, Tozzi, R, Balena, A, Santucci, S, Frontani, R, et al. Very low-calorie ketogenic diet: a safe and effective tool for weight loss in patients with obesity and mild kidney failure. Nutrients. (2020) 12:12. doi: 10.3390/nu12020333
14. Mavropoulos, JC, Yancy, WS, Hepburn, J, and Westman, EC. The effects of a low-carbohydrate, ketogenic diet on the polycystic ovary syndrome: a pilot study. Nutr Metab (Lond). (2005) 2:35. doi: 10.1186/1743-7075-2-35
15. Yang, L, TeSlaa, T, Ng, S, Nofal, M, Wang, L, Lan, T, et al. Ketogenic diet and chemotherapy combine to disrupt pancreatic cancer metabolism and growth. Med. (2022) 3:119–136.e8. doi: 10.1016/j.medj.2021.12.008
16. Accurso, A, Bernstein, RK, Dahlqvist, A, Draznin, B, Feinman, RD, Fine, EJ, et al. Dietary carbohydrate restriction in type 2 diabetes mellitus and metabolic syndrome: time for a critical appraisal. Nutr Metab (Lond). (2008) 5:9. doi: 10.1186/1743-7075-5-9
17. Wood, TR, Stubbs, BJ, and Juul, SE. Exogenous ketone bodies as promising neuroprotective agents for developmental brain injury. Dev Neurosci (Basel). (2019) 40:451–62. doi: 10.1159/000499563
18. Caplliure Llopis, J, Peralta Chamba, T, Carrera Juliá, S, Cuerda Ballester, M, Drehmer Rieger, E, López Rodriguez, MM, et al. Therapeutic alternative of the ketogenic Mediterranean diet to improve mitochondrial activity in amyotrophic lateral sclerosis (ALS): a comprehensive review. Food Sci Nutr. (2019) 8:23–35. doi: 10.1002/fsn3.1324
19. Ahola-Erkkilä, S, Carroll, CJ, Peltola-Mjösund, K, Tulkki, V, Mattila, I, Seppänen-Laakso, T, et al. Ketogenic diet slows down mitochondrial myopathy progression in mice. Hum Mol Genet. (2010) 19:1974–84. doi: 10.1093/hmg/ddq076
20. Wallace, MA, Aguirre, NW, Marcotte, GR, Marshall, AG, Baehr, LM, Hughes, DC, et al. The ketogenic diet preserves skeletal muscle with aging in mice. Aging Cell. (2021) 20:e13322. doi: 10.1111/acel.13322
21. Paoli, A, Grimaldi, K, Toniolo, L, Canato, M, Bianco, A, and Fratter, A. Nutrition and acne: therapeutic potential of ketogenic diets. Skin Pharmacol Phys. (2012) 25:111–7. doi: 10.1159/000336404
22. Goossens, C, Weckx, R, Derde, S, Dufour, T, Vander Perre, S, Pauwels, L, et al. Adipose tissue protects against sepsis-induced muscle weakness in mice: from lipolysis to ketones. Crit Care. (2019) 23:236. doi: 10.1186/s13054-019-2506-6
23. Barzegar, M, Afghan, M, Tarmahi, V, Behtari, M, Rahimi Khamaneh, S, and Raeisi, S. Ketogenic diet: overview, types, and possible anti-seizure mechanisms. Nutr Neurosci. (2021) 24:307–16. doi: 10.1080/1028415X.2019.1627769
24. Puchalska, P, and Crawford, PA. Multi-dimensional roles of ketone bodies in fuel metabolism, signaling, and therapeutics. Cell Metab. (2017) 25:262–84. doi: 10.1016/j.cmet.2016.12.022
25. Bentourkia, M, Tremblay, S, Pifferi, F, Rousseau, J, Lecomte, R, and Cunnane, S. PET study of 11C-acetoacetate kinetics in rat brain during dietary treatments affecting ketosis. Am J Physiol Endocrinol Metab. (2009) 296:E796–801. doi: 10.1152/ajpendo.90644.2008
26. Paoli, A . Ketogenic diet for obesity: friend or foe? Int J Environ Res Public Health. (2014) 11:2092–107. doi: 10.3390/ijerph110202092
27. Barry, D, Ellul, S, Watters, L, Lee, D, Haluska, RJ, and White, R. The ketogenic diet in disease and development. Int J Dev Neurosci. (2018) 68:53–8. doi: 10.1016/j.ijdevneu.2018.04.005
28. Reid, CL, Campbell, IT, and Little, RA. Muscle wasting and energy balance in critical illness. Clin Nutr. (2004) 23:273–80. doi: 10.1016/S0261-5614(03)00129-8
29. Reintam, BA, Starkopf, J, Alhazzani, W, Berger, MM, Casaer, MP, Deane, AM, et al. Early enteral nutrition in critically ill patients: ESICM clinical practice guidelines. Intensive Care Med. (2017) 43:380–98. doi: 10.1007/s00134-016-4665-0
30. Heyland, DK, Cahill, N, and Day, AG. Optimal amount of calories for critically ill patients: depends on how you slice the cake! Crit Care Med. (2011) 39:2619–26. doi: 10.1097/CCM.0b013e318226641d
31. Harvey, SE, Parrott, F, Harrison, DA, Bear, DE, Segaran, E, Beale, R, et al. Trial of the route of early nutritional support in critically ill adults. N Engl J Med. (2014) 371:1673–84. doi: 10.1056/NEJMoa1409860
32. Rice, TW, Mogan, S, Hays, MA, Bernard, GR, Jensen, GL, and Wheeler, AP. Randomized trial of initial trophic versus full-energy enteral nutrition in mechanically ventilated patients with acute respiratory failure. Crit Care Med. (2011) 39:967–74. doi: 10.1097/CCM.0b013e31820a905a
33. Heidegger, CP, Berger, MM, Graf, S, Zingg, W, Darmon, P, Costanza, MC, et al. Optimisation of energy provision with supplemental parenteral nutrition in critically ill patients: a randomised controlled clinical trial. Lancet. (2013) 381:385–93. doi: 10.1016/S0140-6736(12)61351-8
34. Reintam Blaser, A, Rooyackers, O, and Bear, DE. How to avoid harm with feeding critically ill patients: a synthesis of viewpoints of a basic scientist, dietitian and intensivist. Crit Care. (2023) 27:258. doi: 10.1186/s13054-023-04543-1
35. Weijs, PJ, Looijaard, WG, Beishuizen, A, Girbes, AR, and Oudemans-van, SH. Early high protein intake is associated with low mortality and energy overfeeding with high mortality in non-septic mechanically ventilated critically ill patients. Crit Care. (2014) 18:701. doi: 10.1186/s13054-014-0701-z
36. Lee, ZY, Yap, C, Hasan, MS, Engkasan, JP, Barakatun-Nisak, MY, Day, AG, et al. The effect of higher versus lower protein delivery in critically ill patients: a systematic review and meta-analysis of randomized controlled trials. Crit Care. (2021) 25:260. doi: 10.1186/s13054-021-03693-4
37. Weijs, PJ, Stapel, SN, de Groot, SD, Driessen, RH, de Jong, E, Girbes, AR, et al. Optimal protein and energy nutrition decreases mortality in mechanically ventilated, critically ill patients: a prospective observational cohort study. JPEN J Parenter Enteral Nutr. (2012) 36:60–8. doi: 10.1177/0148607111415109
38. Lindner, G, Funk, GC, Schwarz, C, Kneidinger, N, Kaider, A, Schneeweiss, B, et al. Hypernatremia in the critically ill is an independent risk factor for mortality. Am J Kidney Dis. (2007) 50:952–7. doi: 10.1053/j.ajkd.2007.08.016
39. Gunst, J, Vanhorebeek, I, Thiessen, SE, and Van den Berghe, G. Amino acid supplements in critically ill patients. Pharmacol Res. (2018) 130:127–31. doi: 10.1016/j.phrs.2017.12.007
40. Li, F, Lang, F, Zhang, H, Xu, L, Wang, Y, and Hao, E. Role of TFEB mediated autophagy, oxidative stress, inflammation, and cell death in endotoxin induced myocardial toxicity of young and aged mice. Oxidative Med Cell Longev. (2016) 2016:5380319. doi: 10.1155/2016/5380319
41. Hermans, G, Casaer, MP, Clerckx, B, Guiza, F, Vanhullebusch, T, Derde, S, et al. Effect of tolerating macronutrient deficit on the development of intensive-care unit acquired weakness: a subanalysis of the EPaNIC trial. Lancet Respir Med. (2013) 1:621–9. doi: 10.1016/S2213-2600(13)70183-8
42. Zhang, J, Chen, B, and Zou, K. Effect of ketogenic diet on exercise tolerance and transcriptome of gastrocnemius in mice. Open Life Sci. (2023) 18:20220570. doi: 10.1515/biol-2022-0570
43. Weckx, R, Goossens, C, Derde, S, Pauwels, L, Vander, PS, Van den Berghe, G, et al. Efficacy and safety of ketone ester infusion to prevent muscle weakness in a mouse model of sepsis-induced critical illness. Sci Rep. (2022) 12:10591. doi: 10.1038/s41598-022-14961-w
44. White, RH, Frayn, KN, Little, RA, Threlfall, CJ, Stoner, HB, and Irving, MH. Hormonal and metabolic responses to glucose infusion in sepsis studied by the hyperglycemic glucose clamp technique. JPEN J Parenter Enteral Nutr. (1987) 11:345–53. doi: 10.1177/0148607187011004345
45. Giovannini, I, Boldrini, G, Castagneto, M, Sganga, G, Nanni, G, Pittiruti, M, et al. Respiratory quotient and patterns of substrate utilization in human sepsis and trauma. JPEN J Parenter Enteral Nutr. (1983) 7:226–30. doi: 10.1177/0148607183007003226
46. Langley, RJ, Tsalik, EL, van Velkinburgh, JC, Glickman, SW, Rice, BJ, Wang, C, et al. An integrated clinico-metabolomic model improves prediction of death in sepsis. Sci Transl Med. (2013) 5:195ra95. doi: 10.1126/scitranslmed.3005893
47. Gill, SK, Hui, K, Farne, H, Garnett, JP, Baines, DL, Moore, LS, et al. Increased airway glucose increases airway bacterial load in hyperglycaemia. Sci Rep. (2016) 6:27636. doi: 10.1038/srep27636
48. Darby, AM, Okoro, DO, Aredas, S, Frank, AM, Pearson, WH, Dionne, MS, et al. High sugar diets can increase susceptibility to bacterial infection in Drosophila melanogaster. PLoS Pathog. (2024) 20:e1012447. doi: 10.1371/journal.ppat.1012447
49. Petronilho, F, Giustina, AD, Nascimento, DZ, Zarbato, GF, Vieira, AA, Florentino, D, et al. Obesity exacerbates Sepsis-induced oxidative damage in organs. Inflammation. (2016) 39:2062–71. doi: 10.1007/s10753-016-0444-x
50. Beylot, M, Guiraud, M, Grau, G, and Bouletreau, P. Regulation of ketone body flux in septic patients. Am J Phys. (1989) 257:E665–74. doi: 10.1152/ajpendo.1989.257.5.E665
51. Rahmel, T, Effinger, D, Bracht, T, Griep, L, Koos, B, Sitek, B, et al. An open-label, randomized controlled trial to assess a ketogenic diet in critically ill patients with sepsis. Sci Transl Med. (2024) 16:n9285. doi: 10.1126/scitranslmed.adn9285
52. de Cabo, R, and Mattson, MP. Effects of intermittent fasting on health, aging, and disease. N Engl J Med. (2019) 381:2541–51. doi: 10.1056/NEJMra1905136
53. Dong, TA, Sandesara, PB, Dhindsa, DS, Mehta, A, Arneson, LC, Dollar, AL, et al. Intermittent fasting: a heart healthy dietary pattern? Am J Med. (2020) 133:901–7. doi: 10.1016/j.amjmed.2020.03.030
54. Dulloo, AG, Jacquet, J, and Montani, J. How dieting makes some fatter: from a perspective of human body composition autoregulation. Proc Nutr Soc. (2012) 71:379–89. doi: 10.1017/S0029665112000225
55. Danial, NN, Hartman, AL, Stafstrom, CE, and Thio, LL. How does the ketogenic diet work? Four potential mechanisms. J Child Neurol. (2013) 28:1027–33. doi: 10.1177/0883073813487598
56. De Bruyn, A, Langouche, L, Vander, PS, Gunst, J, and Van den Berghe, G. Impact of withholding early parenteral nutrition in adult critically ill patients on ketogenesis in relation to outcome. Crit Care. (2021) 25:102. doi: 10.1186/s13054-021-03519-3
57. Goossens, C, Weckx, R, Derde, S, Vander, PS, Derese, I, Van Veldhoven, PP, et al. Altered cholesterol homeostasis in critical illness-induced muscle weakness: effect of exogenous 3-hydroxybutyrate. Crit Care. (2021) 25:252. doi: 10.1186/s13054-021-03688-1
58. Westerblad, H, Bruton, JD, and Katz, A. Skeletal muscle: energy metabolism, fiber types, fatigue and adaptability. Exp Cell Res. (2010) 316:3093–9. doi: 10.1016/j.yexcr.2010.05.019
59. Puthucheary, ZA, Rawal, J, McPhail, M, Connolly, B, Ratnayake, G, Chan, P, et al. Acute skeletal muscle wasting in critical illness. JAMA. (2013) 310:1591. doi: 10.1001/jama.2013.278481
60. Friedrich, O, Reid, MB, Van den Berghe, G, Vanhorebeek, I, Hermans, G, Rich, MM, et al. The sick and the weak: neuropathies/myopathies in the critically ill. Physiol Rev. (2015) 95:1025–109. doi: 10.1152/physrev.00028.2014
61. McClave, SA, Wischmeyer, PE, Miller, KR, and van Zanten, A. Mitochondrial dysfunction in critical illness: implications for nutritional therapy. Curr Nutr Rep. (2019) 8:363–73. doi: 10.1007/s13668-019-00296-y
62. Marik, PE, and Bellomo, R. Stress hyperglycemia: an essential survival response! Crit Care. (2013) 17:305. doi: 10.1186/cc12514
63. Cox, PJ, Kirk, T, Ashmore, T, Willerton, K, Evans, R, Smith, A, et al. Nutritional ketosis alters fuel preference and thereby endurance performance in athletes. Cell Metab. (2016) 24:256–68. doi: 10.1016/j.cmet.2016.07.010
64. Nuzzo, E, Berg, KM, Andersen, LW, Balkema, J, Montissol, S, Cocchi, MN, et al. Pyruvate dehydrogenase activity is decreased in the peripheral blood mononuclear cells of patients with Sepsis. A prospective observational trial. Ann Am Thorac Soc. (2015) 12:1662–6. doi: 10.1513/AnnalsATS.201505-267BC
65. Vary, TC . Sepsis-induced alterations in pyruvate dehydrogenase complex activity in rat skeletal muscle: effects on plasma lactate. Shock. (1996) 6:89–94. doi: 10.1097/00024382-199608000-00002
66. Inui, T, Wada, Y, Shibuya, M, Arai-Ichinoi, N, Okubo, Y, Endo, W, et al. Intravenous ketogenic diet therapy for neonatal-onset pyruvate dehydrogenase complex deficiency. Brain and Development. (2022) 44:244–8. doi: 10.1016/j.braindev.2021.11.005
67. Nakao, R, Abe, T, Yamamoto, S, and Oishi, K. Ketogenic diet induces skeletal muscle atrophy via reducing muscle protein synthesis and possibly activating proteolysis in mice. Sci Rep. (2019) 9:19652. doi: 10.1038/s41598-019-56166-8
68. Spriet, LL, Tunstall, RJ, Watt, MJ, Mehan, KA, Hargreaves, M, and Cameron-Smith, D. Pyruvate dehydrogenase activation and kinase expression in human skeletal muscle during fasting. J Appl Physiol (1985). (2004) 96:2082–7. doi: 10.1152/japplphysiol.01318.2003
69. Parker, BA, Walton, CM, Carr, ST, Andrus, JL, Cheung, E, Duplisea, MJ, et al. beta-Hydroxybutyrate elicits favorable mitochondrial changes in skeletal muscle. Int J Mol Sci. (2018) 19:19. doi: 10.3390/ijms19082247
70. Saito, H, Wada, N, and Iida, K. Isonitrogenous low-carbohydrate diet elicits specific changes in metabolic gene expression in the skeletal muscle of exercise-trained mice. PLoS One. (2022) 17:e262875:e0262875. doi: 10.1371/journal.pone.0262875
71. Effinger, D, Hirschberger, S, Yoncheva, P, Schmid, A, Heine, T, Newels, P, et al. A ketogenic diet substantially reshapes the human metabolome. Clin Nutr. (2023) 42:1202–12. doi: 10.1016/j.clnu.2023.04.027
72. Nair, KS, Welle, SL, Halliday, D, and Campbell, RG. Effect of beta-hydroxybutyrate on whole-body leucine kinetics and fractional mixed skeletal muscle protein synthesis in humans. J Clin Invest. (1988) 82:198–205. doi: 10.1172/JCI113570
73. Berkes, CA, and Tapscott, SJ. MyoD and the transcriptional control of myogenesis. Semin Cell Dev Biol. (2005) 16:585–95. doi: 10.1016/j.semcdb.2005.07.006
74. Lercher, A, Baazim, H, and Bergthaler, A. Systemic Immunometabolism: challenges and opportunities. Immunity. (2020) 53:496–509. doi: 10.1016/j.immuni.2020.08.012
75. Otani, S, and Coopersmith, CM. Gut integrity in critical illness. J Intensive Care. (2019) 7:17. doi: 10.1186/s40560-019-0372-6
76. Zhou, Y, Luo, Y, Wang, X, Luan, F, Peng, Y, Li, Y, et al. Early gut microbiological changes and metabolomic changes in patients with sepsis: a preliminary study. Int Microbiol. (2023) 26:1131–42. doi: 10.1007/s10123-023-00363-z
77. Kau, AL, Ahern, PP, Griffin, NW, Goodman, AL, and Gordon, JI. Human nutrition, the gut microbiome, and immune system: envisioning the future. Nature (London). (2011) 474:327–36. doi: 10.1038/nature10213
78. Hooper, LV, Littman, DR, and Macpherson, AJ. Interactions between the microbiota and the immune system. Science. (2012) 336:1268–73. doi: 10.1126/science.1223490
79. Zeng, MY, Inohara, N, and Nunez, G. Mechanisms of inflammation-driven bacterial dysbiosis in the gut. Mucosal Immunol. (2017) 10:18–26. doi: 10.1038/mi.2016.75
80. Karlsson, FH, Fak, F, Nookaew, I, Tremaroli, V, Fagerberg, B, Petranovic, D, et al. Symptomatic atherosclerosis is associated with an altered gut metagenome. Nat Commun. (2012) 3:1245. doi: 10.1038/ncomms2266
81. Ticinesi, A, Nouvenne, A, Cerundolo, N, Catania, P, Prati, B, Tana, C, et al. Gut microbiota, muscle mass and function in aging: a focus on physical frailty and sarcopenia. Nutrients. (2019) 11:11. doi: 10.3390/nu11071633
82. Blanton, LV, Charbonneau, MR, Salih, T, Barratt, MJ, Venkatesh, S, Ilkaveya, O, et al. Gut bacteria that prevent growth impairments transmitted by microbiota from malnourished children. Science. (2016) 351:351. doi: 10.1126/science.aad3311
83. Langille, MG, Meehan, CJ, Koenig, JE, Dhanani, AS, Rose, RA, Howlett, SE, et al. Microbial shifts in the aging mouse gut. Microbiome. (2014) 2:50. doi: 10.1186/s40168-014-0050-9
84. Siddharth, J, Chakrabarti, A, Pannerec, A, Karaz, S, Morin-Rivron, D, Masoodi, M, et al. Aging and sarcopenia associate with specific interactions between gut microbes, serum biomarkers and host physiology in rats. Aging (Albany NY). (2017) 9:1698–720. doi: 10.18632/aging.101262
85. Ma, D, Wang, AC, Parikh, I, Green, SJ, Hoffman, JD, Chlipala, G, et al. Ketogenic diet enhances neurovascular function with altered gut microbiome in young healthy mice. Sci Rep. (2018) 8:6670. doi: 10.1038/s41598-018-25190-5
86. Rahmel, T, Hübner, M, Koos, B, Wolf, A, Willemsen, K, Strauß, G, et al. Impact of carbohydrate-reduced nutrition in septic patients on ICU: study protocol for a prospective randomised controlled trial. BMJ Open. (2020) 10:e38532:e038532. doi: 10.1136/bmjopen-2020-038532
87. Lin, J, Huang, Z, Liu, J, Huang, Z, Liu, Y, Liu, Q, et al. Neuroprotective effect of ketone metabolism on inhibiting inflammatory response by regulating macrophage polarization after acute cervical spinal cord injury in rats. Front Neurosci. (2020) 14:583611. doi: 10.3389/fnins.2020.583611
88. Kesarwani, P, Kant, S, Zhao, Y, Miller, CR, and Chinnaiyan, P. The influence of the ketogenic diet on the immune tolerant microenvironment in glioblastoma. Cancers. (2022) 14:5550. doi: 10.3390/cancers14225550
89. Hirschberger, S, Gellert, L, Effinger, D, Muenchhoff, M, Herrmann, M, Briegel, JM, et al. Ketone bodies improve human CD8(+) cytotoxic T-cell immune response during COVID-19 infection. Front Med (Lausanne). (2022) 9:923502. doi: 10.3389/fmed.2022.923502
90. Masino, SA, Ruskin, DN, Freedgood, NR, Lindefeldt, M, and Dahlin, M. Differential ketogenic diet-induced shift in CSF lipid/carbohydrate metabolome of pediatric epilepsy patients with optimal vs. no anticonvulsant response: a pilot study. Nutr Metab (Lond). (2021) 18:23. doi: 10.1186/s12986-020-00524-1
91. Grosicki, GJ, Fielding, RA, and Lustgarten, MS. Gut microbiota contribute to age-related changes in skeletal muscle size, composition, and function: biological basis for a gut-muscle Axis. Calcif Tissue Int. (2018) 102:433–42. doi: 10.1007/s00223-017-0345-5
92. Wu, XX, Huang, XL, Chen, RR, Li, T, Ye, HJ, Xie, W, et al. Paeoniflorin prevents intestinal barrier disruption and inhibits lipopolysaccharide (LPS)-induced inflammation in Caco-2 cell monolayers. Inflammation. (2019) 42:2215–25. doi: 10.1007/s10753-019-01085-z
93. Cohen, S, Nathan, JA, and Goldberg, AL. Muscle wasting in disease: molecular mechanisms and promising therapies. Nat Rev Drug Discov. (2015) 14:58–74. doi: 10.1038/nrd4467
94. Zanders, L, Kny, M, Hahn, A, Schmidt, S, Wundersitz, S, Todiras, M, et al. Sepsis induces interleukin 6, gp130/JAK2/STAT3, and muscle wasting. J Cachexia Sarcopenia Muscle. (2022) 13:713–27. doi: 10.1002/jcsm.12867
95. Kim, JT, Napier, DL, Kim, J, Li, C, Lee, EY, Weiss, HL, et al. Ketogenesis alleviates TNFalpha-induced apoptosis and inflammatory responses in intestinal cells. Free Radic Biol Med. (2021) 172:90–100. doi: 10.1016/j.freeradbiomed.2021.05.032
96. Alsharairi, NA . The therapeutic role of short-chain fatty acids mediated very low-calorie ketogenic diet-gut microbiota relationships in Paediatric inflammatory bowel diseases. Nutrients. (2022) 14:14. doi: 10.3390/nu14194113
97. Ghosh, S, Lertwattanarak, R, Garduno, JJ, Galeana, JJ, Li, J, Zamarripa, F, et al. Elevated muscle TLR4 expression and metabolic endotoxemia in human aging. J Gerontol A Biol Sci Med Sci. (2015) 70:232–46. doi: 10.1093/gerona/glu067
98. Thevaranjan, N, Puchta, A, Schulz, C, Naidoo, A, Szamosi, JC, Verschoor, CP, et al. Age-associated microbial Dysbiosis promotes intestinal permeability, systemic inflammation, and macrophage dysfunction. Cell Host Microbe. (2017) 21:455–466.e4. doi: 10.1016/j.chom.2017.03.002
99. Sartori, R, Romanello, V, and Sandri, M. Mechanisms of muscle atrophy and hypertrophy: implications in health and disease. Nat Commun. (2021) 12:330. doi: 10.1038/s41467-020-20123-1
100. Manolis, AS, Manolis, TA, and Manolis, AA. Ketone bodies and cardiovascular disease: an alternate fuel source to the rescue. Int J Mol Sci. (2023) 24:24. doi: 10.3390/ijms24043534
101. Goldberg, EL, Asher, JL, Molony, RD, Shaw, AC, Zeiss, CJ, Wang, C, et al. beta-Hydroxybutyrate deactivates neutrophil NLRP3 Inflammasome to relieve gout flares. Cell Rep. (2017) 18:2077–87. doi: 10.1016/j.celrep.2017.02.004
102. Stubbs, BJ, Koutnik, AP, Goldberg, EL, Upadhyay, V, Turnbaugh, PJ, Verdin, E, et al. Investigating ketone bodies as Immunometabolic countermeasures against respiratory viral infections. Med. (2020) 1:43–65. doi: 10.1016/j.medj.2020.06.008
103. Choi, IY, Piccio, L, Childress, P, Bollman, B, Ghosh, A, Brandhorst, S, et al. A diet mimicking fasting promotes regeneration and reduces autoimmunity and multiple sclerosis symptoms. Cell Rep. (2016) 15:2136–46. doi: 10.1016/j.celrep.2016.05.009
104. Olson, CA, Iniguez, AJ, Yang, GE, Fang, P, Pronovost, GN, Jameson, KG, et al. Alterations in the gut microbiota contribute to cognitive impairment induced by the ketogenic diet and hypoxia. Cell Host Microbe. (2021) 29:1378–1392.e6. doi: 10.1016/j.chom.2021.07.004
105. Li, C, Pan, J, Sun, P, Wang, S, Wang, S, Feng, W, et al. Ketogenic diet alleviates hypoglycemia-induced Neuroinflammation via modulation the gut microbiota in mice. Mol Nutr Food Res. (2023) 67:e2200711. doi: 10.1002/mnfr.202200711
106. Ang, QY, Alexander, M, Newman, JC, Tian, Y, Cai, J, Upadhyay, V, et al. Ketogenic diets Alter the gut microbiome resulting in decreased intestinal Th17 cells. Cell. (2020) 181:1263–1275.e16. doi: 10.1016/j.cell.2020.04.027
107. Jiang, Z, Wang, X, Zhang, H, Yin, J, Zhao, P, Yin, Q, et al. Ketogenic diet protects MPTP-induced mouse model of Parkinson's disease via altering gut microbiota and metabolites. MedComm. (2023) 4:e268. doi: 10.1002/mco2.268
108. Shan, T, Huang, Y, Zhao, Z, Li, F, Wang, Y, Ye, C, et al. Ketogenic diet restrains herpes simplex encephalitis via gut microbes. Microbes Infect. (2023) 25:105061. doi: 10.1016/j.micinf.2022.105061
109. Park, S, Zhang, T, Wu, X, and Yi, QJ. Ketone production by ketogenic diet and by intermittent fasting has different effects on the gut microbiota and disease progression in an Alzheimer's disease rat model. J Clin Biochem Nutr. (2020) 67:188–98. doi: 10.3164/jcbn.19-87
110. Rohwer, N, El, HR, Smyl, C, Ocvirk, S, Goris, T, Grune, T, et al. Ketogenic diet has moderate effects on the fecal microbiota of wild-type mice. Nutrients. (2023) 15:15. doi: 10.3390/nu15214629
111. Xia, H, Guo, J, Shen, J, Jiang, S, Han, S, and Li, L. Butyrate ameliorated the intestinal barrier dysfunction and attenuated acute pancreatitis in mice fed with ketogenic diet. Life Sci. (2023) 334:122188. doi: 10.1016/j.lfs.2023.122188
112. Dahlin, M, Singleton, SS, David, JA, Basuchoudhary, A, Wickstrom, R, Mazumder, R, et al. Higher levels of Bifidobacteria and tumor necrosis factor in children with drug-resistant epilepsy are associated with anti-seizure response to the ketogenic diet. EBioMedicine. (2022) 80:104061. doi: 10.1016/j.ebiom.2022.104061
113. Quan, R, Chen, C, Yan, W, Zhang, Y, Zhao, X, and Fu, Y. BAFF blockade attenuates inflammatory responses and intestinal barrier dysfunction in a murine Endotoxemia model. Front Immunol. (2020) 11:570920. doi: 10.3389/fimmu.2020.570920
114. Xu, J, Kong, X, Li, J, Mao, H, Zhu, Y, Zhu, X, et al. Pediatric intensive care unit treatment alters the diversity and composition of the gut microbiota and antimicrobial resistance gene expression in critically ill children. Front Microbiol. (2023) 14:1237993. doi: 10.3389/fmicb.2023.1237993
Keywords: gut microbiota, ICU-acquired weakness, ketone bodies, ketogenic diet, sepsis-related acquired weakness
Citation: Miao Y, Xie L, Chen S, Zhang X, Liu W and Xie P (2024) Ketogenic diet in treating sepsis-related acquired weakness: is it friend or foe? Front. Nutr. 11:1484856. doi: 10.3389/fnut.2024.1484856
Edited by:
Le Li, Wuhan University, ChinaReviewed by:
Chang Hu, Zhongnan Hospital of Wuhan University, ChinaGeorgia Damoraki, National and Kapodistrian University of Athens, Greece
Yaya Xu, Shanghai Jiao Tong University, China
Copyright © 2024 Miao, Xie, Chen, Zhang, Liu and Xie. This is an open-access article distributed under the terms of the Creative Commons Attribution License (CC BY). The use, distribution or reproduction in other forums is permitted, provided the original author(s) and the copyright owner(s) are credited and that the original publication in this journal is cited, in accordance with accepted academic practice. No use, distribution or reproduction is permitted which does not comply with these terms.
*Correspondence: Wenjie Liu, d2VuamllbGl1OTZAMTYzLmNvbQ==; Peng Xie, MjAyMzAyMDIyMUB1c2MuZWR1LmNu
†These authors have contributed equally to this work