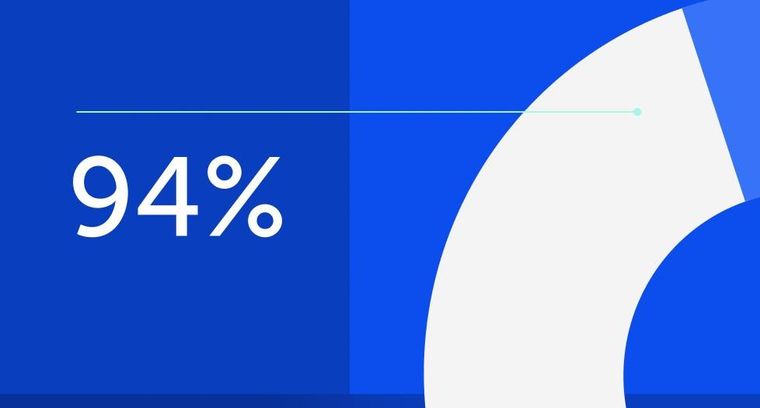
94% of researchers rate our articles as excellent or good
Learn more about the work of our research integrity team to safeguard the quality of each article we publish.
Find out more
REVIEW article
Front. Nutr., 01 August 2024
Sec. Nutrition, Psychology and Brain Health
Volume 11 - 2024 | https://doi.org/10.3389/fnut.2024.1425839
This article is part of the Research TopicIntervention Effects of Food-derived Polyphenols and Bioactive Peptides on Chronic InflammationView all 14 articles
Catechins, a class of phytochemicals found in various fruits and tea leaves, have garnered attention for their diverse health-promoting properties, including their potential in combating neurodegenerative diseases. Among these catechins, (−)-epigallocatechin-3-gallate (EGCG), the most abundant polyphenol in green tea, has emerged as a promising therapeutic agent due to its potent antioxidant and anti-inflammatory effects. Chronic neuroinflammation and oxidative stress are key pathological mechanisms in neurodegenerative diseases such as Alzheimer’s disease (AD) and Parkinson’s disease (PD). EGCG has neuroprotective efficacy due to scavenging free radicals, reducing oxidative stress and attenuating neuroinflammatory processes. This review discusses the molecular mechanisms of EGCG’s anti-oxidative stress and chronic neuroinflammation, emphasizing its effects on autoimmune responses, neuroimmune system interactions, and focusing on the related effects on AD and PD. By elucidating EGCG’s mechanisms of action and its impact on neurodegenerative processes, this review underscores the potential of EGCG as a therapeutic intervention for AD, PD, and possibly other neurodegenerative diseases. Overall, EGCG emerges as a promising natural compound for combating chronic neuroinflammation and oxidative stress, offering novel avenues for neuroprotective strategies in the treatment of neurodegenerative disorders.
Catechins, a class of physiologically active phytochemicals, are commonly found in the fruits and leaves of various plants, including tea, apricots, cherries, peaches, blackberries, strawberries, blueberries, raspberries, and cocoa (1). Research indicates that catechins possess numerous health-promoting properties, notably benefiting cardiovascular disease, metabolic syndrome, diabetes, cancer, stroke, and neurodegenerative diseases (Figure 1) (2–9). As predominant polyphenols in tea, constituting approximately 30% of the dry mass of tea leaves, catechins serve as key functional components. Major green tea polyphenols encompass (−)-epicatechin (EC), (−)-epicatechin gallate (ECG), (−)-epigallocatechin (EGC), and (−)-epigallocatechin gallate (EGCG) (Figure 1) (10, 11). EGCG, the most abundant among green tea catechins at 60%, garners significant interest due to its broad spectrum of benefits elucidated in clinical trials, animal studies, and cell culture research (12). The molecular weight of EGCG is 442.37. Mechanisms underlying EGCG’s multifaceted health effects include antioxidant properties, anti-inflammatory activity, interactions with plasma membrane proteins, activation of second messenger and signaling pathways, modulation of metabolic enzymes, and promotion of autophagy (13–15).
Figure 1. The chemical structures of four common green tea catechins are depicted. Their potential as therapeutic agents for common diseases is discussed. Additionally, the absorption and metabolism of green tea catechins are explored, accompanied by diagrams illustrating the absorption process across various organs of the body. Molecular formula, molecular weight, number of phenoile OH groups of four common catechins. Comparison of the four common catechins in green tea catechin profiles, anti-inflammatory, radical scavengers, and reduced efficiency of lipid peroxidation effects.
Neurodegenerative diseases manifest through the gradual and progressive degeneration of nerve cells in defined regions of the brain and spinal cord, leading to functional impairment. Prominent examples encompass Alzheimer’s disease (AD), Parkinson’s disease (PD), Huntington’s disease (HD), and amyotrophic lateral sclerosis (ALS) (16–18). Although the specific cellular and molecular mechanisms vary across these diseases, common features include oxidative stress, mitochondrial dysfunction, DNA damage, protein aggregation, and neuroinflammation (18, 19). Notably, chronic neuroinflammation and oxidative damage represent shared pathological hallmarks among all neurodegenerative diseases (20, 21). Neuroinflammation serves as a common defense mechanism to protect the brain by removing or inhibiting various pathogens (22). This inflammatory response plays a crucial role in facilitating tissue repair and preserving tissue homeostasis (23). Typically, neuroinflammation abates upon successful tissue repair or pathogen clearance (22, 24). However, when the inflammatory stimulus persists, chronic neuroinflammation ensues (22, 25). Various factors contribute to sustained inflammatory responses, including protein aggregation, systemic infections, gut microbiota dysbiosis, aging, and genetic mutations. Prolonged activation of microglia and astrocytes, key players in neuroinflammation, can precipitate neurodegenerative diseases (26–28). Furthermore, neurons exhibit heightened susceptibility to oxidative damage, attributed to their elevated content of unsaturated fatty acids, rendering them susceptible to free radical attack and peroxidation. Additionally, increased levels of iron in specific brain regions further augment neuronal vulnerability to oxidative stress (29). Consequently, interventions targeting anti-neuroinflammatory and antioxidant pathways hold particular significance in the context of neurodegenerative diseases.
EGCG, a natural polyphenol abundant in green tea, exhibits promising neuroprotective properties attributed to its potent anti-inflammatory and antioxidant activities (12). Accumulating evidence underscores its therapeutic potential in the prevention and treatment of neuroinflammatory and neurodegenerative disorders (30). EGCG demonstrates notable neuroprotective efficacy by modulating signals implicated in autoimmune responses, enhancing interplay between the nervous and immune systems, and effectively attenuating inflammatory processes. Furthermore, EGCG exhibits iron chelation capabilities, scavenges free radicals, and exerts significant antioxidant effects, as evidenced by pertinent studies (31). Therefore, this review comprehensively explores the role of EGCG in various neurodegenerative conditions, particularly AD and PD, with a focus on elucidating its molecular mechanisms underlying anti-neuroinflammatory and antioxidant actions.
Multiple investigations have substantiated the beneficial impact of green tea on neurodegenerative disorders. For instance, Shinichi Kuriyama et al. studied 1,003 elderly individuals aged over 70 years to assess the influence of green tea intake on cognitive function (32). Their findings revealed that subjects consuming more than 100 mL of green tea twice daily exhibited reduced susceptibility to neurodegenerative diseases (32). Similarly, Hu et al. conducted a 13-year longitudinal study involving nearly 30,000 Finnish adults, demonstrating that individuals consistently consuming over 600 mL of green tea daily exhibited a diminished risk of developing PD (33). These observations underscore the association between green tea consumption and a lowered incidence of neurodegenerative conditions.
The health-promoting bioactive components of green tea catechins include a wide range of isomers, the most representative of which are mainly four (EGCG, ECG, EGC and EC), with EGCG accounting for the vast majority of green tea research (34, 35). The biological action of the molecule will be determined by its chemical structure. EGCG (C22H18O11) is a catechin flavanol, specifically a gallate ester formed by the condensation of gallic acid with the (3R)-hydroxyl group of (−)-epigallocatechin, labeled A, B, C, and D (Figure 2) (36). The pentacosanoyl group esterification on Carbon −3 of the C-ring, along with hydroxyl groups on Carbon −3′, −4′, and − 5′ of the B-ring, underlie EGCG’s robust antioxidant activity compared to other catechins. The D- and B-rings contribute to its reactive oxygen species (ROS) neutralizing properties, with the D-ring further enhancing its anticancer and anti-inflammatory attributes. EGCG has seven hydroxyl groups in its aromatic ring. The location and number of hydroxyl groups on the ring determines its biological activity, giving EGCG greater antioxidant properties than EGC or EC, as well as water solubility, making EGCG highly permeable to the blood–brain barrier (BBB) (37). EGCG has been reported to cross the BBB within 0.5 h. Moreover, EGCG features two structures—the ortho-3′,4′-dihydroxy moiety and the 4-keto, 3-hydroxyl, or 4-keto, and 5-hydroxyl moiety—that can chelate metal ions, thereby neutralizing their activity. In essence, EGCG’s distinctive chemical structure and composition confer potent antioxidant and anti-inflammatory properties, suggesting potential benefits in select neurodegenerative disorders (38).
Figure 2. A schematic representation elucidates the role of EGCG in neuroprotection. The diagram illustrates how EGCG exerts antioxidant, anti-inflammatory, and anti-apoptotic effects via various molecular mechanisms, thereby conferring protection against neurodegenerative diseases.
Following oral administration, EGCG undergoes limited absorption by the intestines, resulting in minimal entry into the bloodstream and tissues (39). The constrained bioavailability of orally administered EGCG arises from factors including extreme pH conditions, digestive enzymes, and EGCG’s restricted membrane permeability within the intestinal wall (9). Within the body, EGCG undergoes extensive biotransformation via sulfonation, glucuronidation, and methylation reactions (39). Its half-life is approximately 3.9 h, with complete metabolism occurring within 24 h (40). Furthermore, the biological effects of EGCG are contingent on concentration levels. Plasma concentrations ≤10 μM elicit antioxidant, anti-inflammatory, and insulin-sensitizing effects. Conversely, plasma EGCG levels exceeding 10 μM may induce pro-oxidant activity, augmenting autophagy and cell death, and are commonly employed in tumor therapy (41).
Neuroinflammation serves as a protective mechanism within the nervous or central nervous system (CNS) against various threats including infections, toxic metabolites, autoimmunity, and traumatic brain injury, with the aim of eliminating harmful substances and damaged tissues (42). This process entails the activation of glial cells, which serve as neuroprotective agents by removing endogenous and exogenous substances while safeguarding themselves from ROS (43). Notably, microglia, as ubiquitous innate immune cells in the CNS, are pivotal contributors to neuroinflammation, participating in both anti-inflammatory and pro-inflammatory responses (44). The anti-neuroinflammatory properties of EGCG primarily involve the inhibition of microglial activation and the modulation of pro-inflammatory cytokine expression (45). The pro-inflammatory or neuroprotective functions of microglia are contingent upon their activation status (46). Pathogens or cellular debris induce heightened expression of pro-inflammatory cytokines such as IFNs and LPS, prompting microglial activation from a resting state (47). Activated microglia upregulate pro-inflammatory mediators including IL-1β, IL-23, TNF-α, IL-6, NO, and SOC3 via NF-κB and STAT1 pathways (48). In neuroinflammation, activated microglia sustain the release of pro-inflammatory cytokines, perpetuating chronic inflammation and generating cytotoxic molecules such as ROS and RNS (49). Extensive scientific evidence underscores the role of persistent inflammation in promoting neurodegenerative disorders. Conversely, neuroprotective microglia activated by IL-13, IL-10, and IL-4 secrete various factors associated with neuroprotection and tissue repair, including TGF-β, Chi3l3, Arginase 1, Ym1, IGF-1, and Fzd1 (48).
The effects of EGCG on microglia encompass: (1) Modulation of microglial activation under inflammatory conditions, primarily within the M1/M2 spectrum (50). M1 microglia release neurotoxic and inflammatory factors such as IL-6, IL-1β, and TNF-α, contributing to neuronal damage and death, while M2 microglia secrete neurotrophic factors including BDNF, IL-4, and IL-10, fostering neuronal growth and protection (51). EGCG downregulates M1 markers (IL-6, TNF-α, and IL-1β) and upregulates M2 markers (IL-10 and NQO1) in microglia, thereby modulating the M1/M2 ratio and mitigating neurotoxicity and neuronal damage arising from microglial hyperactivity (13). (2) EGCG induces M1 polarization via various signaling pathways including TLR4/NF-κB, JAK2/STAT3, TLR2, TLR4, JNK/P38, thereby suppressing the activation of inflammatory vesicles and reducing microglial inflammation and neurotoxicity (13). (3) Voltage-gated proton channels play a pivotal role in microglial NADPH oxidase-dependent ROS generation (52). EGCG impedes proton channel function in microglia without affecting channel gating processes. This inhibition of proton channels constitutes a significant mechanism through which EGCG suppresses microglial activation and neurotoxicity (53). (4) Neuronal injury or neuroinflammation triggers microglial activation, leading to NO production. NO reacts with cysteine thiols, resulting in protein S-nitrosylation, which regulates various cell signaling and protein activities, including protein misfolding and mitochondrial apoptosis. EGCG attenuates protein S-nitrosylation in activated microglia (54). In summary, EGCG mitigates excessive inflammatory responses and neurotoxicity induced by inflammation by inhibiting inducible NO synthase activity, reducing oxidative stress levels, and modulating the M1/M2 ratio in microglia.
EGCG, a significant natural antioxidant, demonstrates efficacy in neutralizing ROS like hydrogen peroxide, superoxide anions, and hydroxyl radicals (55). Its antioxidant properties stem from the polyhydroxyl structure and gallic acid moiety, which facilitate free radical scavenging, while the presence of phenolic moieties can lead to quinone generation via oxidative sensitivity (56). EGCG exerts antioxidant effects through diverse mechanisms, including hydrogen atom transfer (HAT), electron transfer, and catalytic metal chelation (Figure 2) (57). ROS are metabolically generated by organelles such as mitochondria, peroxisomes, and the endoplasmic reticulum (58). Normally, the antioxidant system efficiently eliminates ROS. However, oxidative stress prompts a shift in signaling pathways, fostering inflammation via pathways like NF-κB, PKC, MAPK, Nrf-2, and PI3K/Akt (59). EGCG mitigates oxidative stress by modulating these pathways (38, 60).
Moreover, studies have indicated that EGCG exerts a direct antioxidant effect by chelating free transition metals such as iron and copper (61). EGCG functions as a free radical scavenger, acting through two mechanisms: HAT and single electron transfer reaction (SET), in relation to its one-electron reduction potential (62). Additionally, EGCG enhances the activity of phase II enzymes and detoxification enzymes, including catalase, glutathione peroxidase (GPX), superoxide dismutase (SOD), and glutathione S-transferase (63). The regulation of these enzymes is primarily governed by Nrf2, which binds to cis-acting regulatory elements to initiate the gene expression of antioxidant enzymes (64). Furthermore, EGCG attenuates excessive levels of NO generated by inducible nitric oxide synthase (iNOS) (65). NO plays a crucial role in various physiological processes at appropriate concentrations. However, under oxidative stress, NO can act as a pro-inflammatory mediator, generating reactive nitrogen species (RNS) such as peroxynitrite (66). Studies have demonstrated that EGCG inhibits iNOS activity, thereby enhancing the bioavailability of NO levels (67). Additionally, EGCG effectively suppresses the activity of xanthine oxidase, an enzyme involved in purine catabolism and uric acid formation, thereby mitigating the associated increase in ROS (68). Moreover, EGCG inhibits the expression of cyclooxygenase-2 (COX-2), an enzyme crucial for fatty acid metabolism that is upregulated during inflammation, particularly in activated macrophages (69).
Neurodegenerative disease is a common and growing cause of mortality and morbidity worldwide (70), with 152 million people expected to receive the effects of the disease by 2060 (71), including AD, PD, HD, ALS, and prion diseases (72). Among various forms of dementia, AD exhibits the highest prevalence, accounting for 62%, followed by PD (73). The pathology of AD is characterized by the accumulation of extracellular amyloid β (Aβ) plaques and the formation of intracellular neurofibrillary tangles composed of hyperphosphorylated tau protein (38). Clinical manifestations encompass memory loss, cognitive impairment, personality changes, and in severe cases, hallucinations and seizures (74). PD onset is marked by progressive degeneration of dopaminergic neurons within the substantia nigra, leading to diminished levels of striatal dopamine and its metabolites in the adult brain (75). Clinical features include motor dysfunction, bradykinesia, tremors, gait and balance disturbances, cognitive decline, and disorientation (76). ALS, commonly known as Lou Gehrig’s disease, represents an adult-onset progressive neurodegenerative disorder characterized by selective motor neuron degeneration (77). This degeneration progressively affects both upper and lower motor neurons within the brain and spinal cord. The etiology of ALS remains largely elusive in the majority of cases, with fewer than 10% attributed to specific genetic mutations involving genes such as SOD1, C9orf72, TDP43, and FUS (78). HD arises from an unstable polyglutamine repeat expansion within the first exon of the IT-15 gene, which encodes the 350 kDa huntingtin protein (79). The aggregation propensity of huntingtin fibers contributes to the progressive degeneration of cortical and striatal neurons, alongside the formation of neuronal inclusions containing aggregated huntingtin. Clinical manifestations encompass movement disorders and psychiatric symptoms including chorea, coordination deficits, depression, psychosis, and obsessive-compulsive disorder (80).
While the pathological and clinical presentations of neurodegenerative diseases vary, they share common features including specific pathological alterations within distinct brain regions and the degeneration of various neuronal subtypes. Key factors contributing to neurodegenerative processes encompass the dysregulation of pro-apoptotic proteins, oxidative stress damage, immune-mediated inflammation, mitochondrial dysfunction, and reduced expression of trophic factors (81–83). Here we focus on the crosstalk between EGCG and neurodegenerative diseases in terms of EGCG anti-neuroinflammation and oxidative stress. Neuroinflammation and oxidative stress are intertwined, as inflammation amplifies ROS production while ROS, in turn, exacerbate inflammation (84). ROS can directly activate the NF-kB transcription factor pathway, promoting the synthesis of inflammatory cytokines (85). Given the multifactorial nature of neurodegenerative pathologies, the emergence of novel therapeutic strategies is imperative. The antioxidant properties and neuroprotective effects of EGCG have garnered significant attention from researchers worldwide, positioning it as a promising treatment for neurological disorders and a cytoprotective agent. In this section, we delve into the role of EGCG in mitigating oxidative stress and chronic neuroinflammation in two prevalent neurodegenerative diseases: AD and PD.
Neurodegenerative disease affects an estimated 24 million individuals globally, with AD being the most prevalent disease (86). In developed Western nations, individuals aged over 85 exhibit an AD prevalence ranging from 24 to 33%, a figure that escalates with advancing age (87). Given the global aging demographic, AD is poised to become a substantial public health concern over the next two decades and has been identified as a research priority (86). The pathogenic mechanisms underlying AD encompass microglia-induced inflammation, elevated intracellular calcium levels, disruption of antioxidant defense systems, cholinergic dysfunction, overactivation of glutamate receptors, and amplification of the inflammatory response (88). Despite the availability of various medications for managing AD, a definitive treatment remains elusive (89), underscoring the pressing need for research into novel therapeutic approaches and adjunctive therapies. Optimal antioxidant levels in the body have been associated with cognitive preservation, and several studies have demonstrated the neuroprotective effects of catechins, highlighting their potential as adjunctive therapy in select neurodegenerative diseases. These effects rely on the anti-inflammatory and antioxidant properties of catechins (90). Moreover, multiple studies have established a correlation between tea consumption, reduced risk of severe cognitive impairment, and a lower prevalence of AD.
Moeko Noguchi-Shinohara et al. conducted a 2-year follow-up survey of 490 subjects over 60 years of age with cognitive performance and blood tests. Even after correcting for potential confounders, drinking green tea was found to significantly reduce the chance of cognitive deterioration (91). In a questionnaire-based study of 1,003 Japanese participants aged 70 or older, Shinichi Kuriyama et al. discovered a correlation between higher green tea drinking and a lower prevalence of cognitive impairment (32). A brief analysis of tea consumption and prevalence of AD in different country regions by Fernando et al. revealed that countries with higher intake of tea, such as Japan, China, and India, had lower prevalence of AD, whereas European and American countries with lower intake of tea had higher prevalence of AD (92). Although epidemiological data favorably show a negative relationship between drinking tea and the preponderance of AD in that part of the country, any correlation between tea consumption and AD prevalence should be evaluated with caution because the effects of racial differences, dietary preferences, and lifestyle cannot be excluded (92). Yang Yuhuan et al. conducted a questionnaire survey to gauge the cognitive function of seniors 60 years of age and older in the Huangshi community in order to better understand the prevalence of mild cognitive impairment (MCI) and its influencing factors (93). The survey data were tested by chi-square test and it was concluded that the prevalence of MCI was lower in occasional tea drinkers, which may be related to the caffeine and catechins contained in tea, caffeine can reduce the level of Aβ in the brain, which is beneficial for improving cognitive function, while catechins have strong antioxidant capacity, but the study did not prove the relationship between tea drinking and AD prevalence. Wang, Ziqi et al. performed the Mini-Mental State Examination (MMSE) for the assessment of cognitive function in 870 people aged 90 years or older, and cardinality testing of the collected data revealed that the mild cognitive index was significantly different from normal in those who regularly consumed animal oils and legumes (94). In contrast, no significant differences were found for the other 10 foods, including tea, in both the unadjusted and adjusted models (94). Numerous studies have demonstrated the potential of tea consumption to mitigate cognitive decline in older adults; however, experimental evidence supporting its efficacy in AD is lacking (95). Controlled studies examining AD cases have not yielded significant findings regarding tea consumption, thus limiting the inference of beneficial effects of green tea catechins solely based on AD pathogenesis and in vitro studies (96). Despite this, the observed efficacy of green tea in AD surpasses initial expectations, warranting further investigation into the specific role of catechins in AD patients.
Given that Aβ aggregation is recognized as a pivotal factor in the pathogenesis of AD and its impact on the human nervous system, Mahsa Amirpour et al. investigated the neuroprotective potential of green tea in a streptozotocin (STZ)-induced AD model. Their study examined the effects of green tea on cognitive decline, inflammation, and oxidative stress (97). The findings demonstrated that the active compounds present in green tea could mitigate cognitive impairment and ameliorate learning and memory deficits associated with STZ injection (81). Furthermore, green tea may reduce the risk of AD through antioxidative and anti-inflammatory pathways, thus positioning it as a potential preventive intervention (90) (Table 1).
Tingting Chen et al. used mice as a model to demonstrate that the polyphenolic compounds EGC and ECG effectively alleviated Aβ40 aggregation and protofibrillar toxicity by chelating Cu2+ and Zn2+ and reduced ROS production, thereby mitigating Cu2+-Aβ40 and Zn2+-Aβ40induced neuronal toxicity (111). The results showed that tea polyphenols had significant beneficial effects on different aspects of AD pathology (112). Among them, catechin ECG had the most significant effect due to the therapeutic effect of ECG through the BBB, reducing Aβ plaques in the brains of APP/PS1 mice and thus protecting neurons from damage (111). Therefore, the potential of catechins to prevent or improve AD symptoms was laterally demonstrated (111). Lee JW et al. found that EGCG reduced Aβ1-42-induced memory dysfunction by altering the secretion of α-secretase, in addition to EGCG inhibiting Aβ1-42-induced apoptosis (113). These findings imply that EGCG may be a useful tool for delaying the start or progression of AD (Figure 3).
Figure 3. The multifactorial pathophysiology of Alzheimer’s disease is depicted in an illustration. Furthermore, epigallocatechin-3-gallate is highlighted as a potential therapeutic intervention for AD, attributed to its ability to counteract oxidative stress and chronic neuroinflammation.
Neuroinflammation as a pathogenesis of AD has been confirmed by numerous studies. It has been found that cerebrospinal fluid levels of pro-inflammatory factors such as IL-1β, IL-6, and TNF-α are high in AD patients and increase with disease progression (114, 115). In addition, microglia, which play an important role in chronic neuroinflammation, are also involved in this process. Microglia resist the onset and progression of AD by degrading Aβ and tau. However, Aβ in turn activates microglia through TLRs to release pro-neuroinflammatory mediators. In the early stages of AD development, neuroprotective phenotypic microglia appear around Aβ plaques (116, 117). However, in late AD pathogenesis, elevated expression of proinflammatory factors will result in the emergence of microglia with a proinflammatory phenotype and a decrease in their phagocytic activity (118, 119). Pro-inflammatory microglia drive tau proliferation and toxicity by promoting neuroinflammation, such as activation of NLRP3 inflammasomes or induction of NF-kB signaling (23). Defective microglial autophagy leads to dysregulation of lipid metabolism, which increases the pathology of tau within neurons further exacerbating AD (23).
Numerous studies have shown that EGCG treatment of AD is associated with chronic neuroinflammation induced by microglia of anti-inflammatory phenotype (105). Wei et al. conducted in vitro experiments demonstrating that EGCG effectively suppressed the expression of TNFα, IL-1β, IL-6, and iNOS while concurrently restoring intracellular antioxidant levels, including Nrf2 and HO-1. These actions counteracted the pro-inflammatory effects of microglia (120). Furthermore, EGCG inhibited the secretion of pro-inflammatory factors from Aβ-induced pro-inflammatory microglia phenotypes and attenuated microglial neurotoxicity (121). Importantly, EGCG also mitigated Aβ-induced cytotoxicity by attenuating ROS-mediated NF-κB activation and MAPK signaling pathways, including JNK and p38 signaling (121). In vitro investigations have demonstrated that Aβ deposition significantly diminishes following intraperitoneal injection of EGCG at a dose of 20 mg/kg or oral administration of EGCG at 50 mg/kg in drinking water (109, 122). Similarly, Li et al. observed a substantial reduction in Aβ deposition in the frontal cortex (60%) and hippocampus (52%) following oral administration of EGCG at a dose of 20 mg/kg/day for 3 months in an AD mouse model (123). Furthermore, recent findings by Lee et al. revealed that EGCG attenuated LPS-induced memory impairment and neuronal apoptosis, concomitant with a reduction in the expression of inflammatory cytokines TNF-α, IL-1β, and IL-6 (105). These results align with in vitro observations, suggesting that EGCG holds promise as a therapeutic agent for neuroinflammation-associated AD.
The brain is particularly vulnerable to oxidative damage due to its high content of easily oxidizable lipids, elevated oxygen consumption rates, and limited antioxidant defense mechanisms. Age-related increases in brain oxidation contribute to the recognized risk of AD (124). Under normal physiological conditions, SOD catalyzes the conversion of superoxide anions to hydrogen peroxide, thereby safeguarding cells against free radical assault. However, in the presence of elevated levels of certain metal ions such as Fe and Cu, SOD can convert hydrogen peroxide to the more hazardous hydroxyl radical (125). Notably, AD patients exhibit heightened SOD activity, diminished glutamine synthetase activity, and elevated lipid peroxidation, collectively resulting in heightened oxidative stress and accumulation of free radicals. Free radicals inflict damage upon biofilms, disrupting the intracellular milieu and precipitating cellular senescence and demise (126). Peroxidation of impaired lipids results in ribonucleic acid inactivation, prompting DNA and RNA cross-linking and instigating DNA mutations (127). Decomposition of peroxidized lipids yields aldehydes, such as acrolein, which react with phosphoric acid and proteins to generate lipofuscin (128). Accumulation of lipofuscin in the brain contributes to cognitive impairment (129). Furthermore, mitochondrial dysfunction and oxidative stress in AD patients are intricately intertwined, with evidence indicating mutual exacerbation, culminating in AD pathogenesis (130).
Numerous studies have delineated the involvement of increased oxidative stress in AD pathogenesis, and highlighted the potential of EGCG’s antioxidant properties in mitigating this process (131, 132). Abdul M. Haque et al. observed that long-term administration of green tea catechins to AD model mice significantly ameliorated cognitive impairment, accompanied by reduced ROS levels and enhanced antioxidant capacity in the hippocampus and cortex (133). Similarly, Regina Biasibetti et al. investigated the effects of oral EGCG administration (10 mg/kg/day) for 1 month in a rat model of dementia, revealing cognitive deficits reversal and notable reductions in ROS levels and NO production (110). Catechins exert their antioxidative effects by scavenging free radicals and chelating metal ions such as Fe and Cu, thereby reducing ROS production. This dual action mitigates oxidative stress in both peripheral and brain tissues, thereby inhibiting further deterioration of cognitive deficits-associated behaviors (134). Mitochondrial dysfunction enhances ROS generation via the NADPH oxidase pathway (135). EGCG reinstates mitochondrial respiration rate, ATP levels, ROS levels, and membrane potential (102). Its antioxidant properties scavenge ROS production and safeguard against mitochondrial damage (136). Furthermore, EGCG treatment mitigates neuronal apoptosis triggered by endoplasmic reticulum stress subsequent to Aβ exposure. The inflammatory response to neuronal injury induced by various stimuli culminates in the release of pro-inflammatory cytokines and cytotoxins, further exacerbating oxidative stress (137). Numerous studies have demonstrated EGCG’s protective effects against lipopolysaccharide-induced memory impairment and inflammatory responses (105, 138). Through mechanisms associated with protein kinase C (PKC), which facilitates the generation of nontoxic soluble peptide APPβ (sAPPβ) and cell survival, catechins may exert an influence on AD (139, 140). Levites et al. reported that EGCG (1–5 μM) enhances sAPPβ production from PC12 and human neuroblastoma cells (141).
PD follows AD as the second most prevalent neurodegenerative disorder affecting middle-aged and elderly individuals. While PD is uncommon before the age of 50, its incidence escalates markedly with advancing age, peaking between 70 and 85 years, afflicting 7 to 10 million individuals worldwide (142, 143). Pathologically, PD is characterized by the degeneration and loss of dopaminergic neurons within the substantia nigra pars compacta, accompanied by the formation of eosinophilic inclusion bodies known as Lewy bodies within the residual neurons. These alterations disrupt the balance between dopamine and cholinergic neurotransmitters, culminating in aberrant motor function within the basal ganglia. The resultant motor and non-motor symptoms include postural reflex deficits, bradykinesia, muscular rigidity, gait disturbances, and resting tremor (144, 145). The episodic nature of PD in most cases suggests a multifactorial etiology involving genetic susceptibility and environmental influences. While the precise pathogenesis remains elusive, current hypotheses implicate abnormal aggregation of α-synuclein, mitochondrial dysfunction, calcium dyshomeostasis, oxidative stress, and neuroinflammation (146).
In order to determine the relationship between PD incidence and tea consumption, Quintana et al. examined a total of 12 studies from 1981 to 2003, comprising 2,215 cases and 145,578 controls. Their analysis revealed that tea consumption can prevent PD and that this protective effect is more pronounced in the Chinese population (147). In order to study the non-hereditary factors associated with PD, Hosseini Tabatabaei N. et al. used a sample of 150 people, including 75 PD patients and 75 people as controls, and showed that tea intake was protective against PD and that adherence to daily tea consumption reduced the risk of PD by 80% (148). A case–control study was conducted by Harvey Checkoway et al. By studying and counting PD cases (n = 210) and controls (n = 347), it was found that people who drank two or more cups of green tea per day had a reduced incidence of PD compared to those who did not drink green tea (149). According to research by E-K Tan and colleagues, drinking one unit of tea (3 cups per day for 10 years) would result in a 28% decrease in the incidence of PD (150). The effects of tea consumption on 60 patients with idiopathic PD were examined by Chahra CD et al. According to the study’s findings, PD patients who drank tea in addition to traditional medication experienced improvements in their non-motor symptoms and depression (143). Boris Kandinov et al. also demonstrated that drinking tea and smoking delayed the age of PD attacks, while drinking coffee may have the opposite effect (151). Observational epidemiological studies in PD have more experimental data demonstrating a protective effect of green tea compared to AD, and even though epidemiological findings support the beneficial effects of tea consumption, some have not yet provided clear evidence. Therefore, more research is required to determine the connection between drinking tea and the risk of PD.
Pathological accumulation of metal ions or a rapid increase in monoamine oxidase B (MAO-B) activity can induce endogenous dopamine (DA) oxidation, leading to α-synuclein aggregation, mitochondrial dysfunction, and other factors contributing to the heightened incidence of PD. Consequently, mitigation strategies involve the use of ROS scavengers, DA oxidation inhibitors, MAO-B inhibitors, and DA quenchers (152). Zhou et al. demonstrated that catechins can impede DA oxidation by inhibiting enzymes and metal ions. Furthermore, they inhibit MAO-B activity, detoxify ROS, DA quenchers, and harmful DA oxidation byproducts, while regulating the Nrf2-Keap1 and PGC-1 pathways. These findings underscore the inhibitory effects of tea polyphenols on DA-related toxicity (153). In a study by Shyh-Mirnin Ph.D. et al., the influence of EGCG on MAO-B enzyme activity in the adult rat brain was investigated, revealing a decrease in MAO-B enzyme activity (154).
PD primarily affects dopaminergic neurons in the substantia nigra pars compacta (SNpc) region of the brain (155). The neurotoxins 1-methyl-4-phenyl-1,2,3,6-tetrahydropyridine (MPTP) or 6-hydroxydopamine (6-OHDA) specifically damage this brain region, resulting in the loss of dopaminergic neurons (156). This neuronal loss leads to disrupted neural firing patterns and impaired motor control (157). Weinreb et al. investigated the impact of pretreatment with tea extract (0.51 mg/kg) and the tea polyphenol EGCG (2.10 mg/kg) on dopamine neurogenesis loss in the substantia nigra of MPTP-induced PD mouse models (158). Their study revealed a considerable mitigation of neurogenesis loss (158). Siddique Y. H. et al. examined the effects of EGCG in an α-synuclein (h-αS) transgenic Drosophila model of PD, analyzing statistical data and markers of changes in climbing capacity, lipid peroxidation, and apoptosis (159). Their findings demonstrated that various concentrations of EGCG (0.25, 0.50, and 1.0 g/mL) substantially delayed the loss of climbing ability in Drosophila, while reducing oxidative stress and apoptosis (159).
In a study by Tingting Zhou et al., a PD mice model induced by MPTP was utilized to investigate the potential therapeutic effects of EGCG for PD. The results demonstrated that EGCG administration ameliorated impaired locomotion behavior in MPTP-treated mice and protected tyrosine hydroxylase-positive cells in the substantia nigra pars compacta from MPTP-induced toxicity (160). Additionally, following EGCG treatment, flow cytometric analysis revealed an increase in the CD3 + CD4+ to CD3 + CD8+ T cell ratio in peripheral blood of MPTP-treated mice. Furthermore, EGCG appeared to downregulate the expression of inflammatory mediators such as TNF and IL-6 in serum (160). These findings suggest that EGCG may confer neuroprotective effects in MPTP-induced PD mice models, potentially by modulating peripheral immune responses.
Current understanding of PD pathogenesis implicates neurofilaments, synaptic vesicle proteins, and ubiquitinated α-synuclein as primary contributors to the disease pathology (161). Additionally, Lewy bodies may exacerbate the release of free radicals, excessive nitric oxide synthesis, microglia-mediated inflammation, and disruption of protein degradation pathways, further exacerbating the pathophysiology (162). Specific beneficial effects and mechanisms of action of EGCG in PD are summarized in Table 2. In conclusion, EGCG exhibit diverse pharmacological activities in PD by modulating gene expression and interfering with signaling pathways (172). Despite substantial experimental evidence supporting this notion, challenges such as low solubility, limited bioavailability, and BBB impermeability hinder efficient delivery of EGCG to the brain and impede clinical translation (173). Overcoming these obstacles necessitates cross-sectional studies aimed at elucidating chemical modification strategies and optimizing drug delivery mechanisms to enhance their therapeutic efficacy.
Numerous studies have established neuroinflammation as a significant etiological factor in PD, playing a pivotal role in its early pathogenesis. Notably, activated microglia make substantial contributions to this process. Evidence supporting the involvement of activated microglia-mediated chronic neuroinflammation in PD includes: (1) Pro-inflammatory effects are often observed in activated microglia surrounding dopaminergic neurons, with the degree of microglial activation correlating with dopaminergic endings loss in PD (174, 175). (2) Injured neurons release excessive α-synuclein, activating proinflammatory factors like TNF-α, NO, and IL-1β produced by microglia, thereby modulating chronic neuroinflammation in PD (176, 177). (3) Jmjd3, critical for microglial cell phenotype expression, when inhibited, leads to overactivation of pro-inflammatory microglial responses, exacerbating neuroinflammation and neuronal cell death (178). Additionally, it has been proposed that α-synuclein aggregates exert toxicity on neurons only in the presence of microglia (179, 180). In PD patients, misfolded α-synuclein is released from injured neurons into the extracellular fluid, where it binds to Toll-like receptors (TLRs), Fcγ receptors (FcγR), or nucleotide-binding oligomerization domain-like receptors (NLRPs), further activating microglia (181–184). The proinflammatory cytokines released by activated microglia subsequently activate protein kinase R (PKR), leading to phosphorylation of α-synuclein at Ser129, a process considered of significant pathological importance, particularly in Lewy bodies of PD patients. Moreover, microglia are involved in the clearance of protein deposits, including α-synuclein and Aβ, from astrocytes (185–187). Activation of microglia upregulates MHC I expression on neurons, promoting neuronal presentation of α-synuclein antigen. Subsequently, these neurons are targeted and eliminated by α-synuclein-reactive T cells (187). The emergence of α-synuclein pathology follows microglial activation, suggesting α-synuclein’s pivotal role in PD progression, albeit not as an initiator. Similarly, mounting evidence suggests that the immune response contributes to neuronal death as a cause rather than a consequence (22).
A growing body of evidence suggests that EGCG may impede or postpone the progression of PD by targeting chronic neuroinflammation. EGCG exhibits potent anti-inflammatory activity both in vitro and in vivo, primarily attributed to its ability to inhibit microglia-induced cytotoxicity (120). In vitro studies have demonstrated that EGCG suppresses the secretion of pro-inflammatory factors from LPS-activated microglia by downregulating the expression of iNOS and TNF-α (188). Furthermore, EGCG has been shown to inhibit microglial activation and reduce neuronal damage in SH-SY5Y and rat mesencephalic cultures (188). Gülşen Özduran et al. reported that EGCG restored viability in PD model cells, inhibited apoptosis, and enhanced survival by attenuating 6-OHDA-induced expression of TNF-α and IL-1β in SK-N-AS cells (189). The findings from the in vivo study corroborate those observed in vitro, further substantiating the potential of EGCG to mitigate the inflammatory response associated with microglia-mediated damage to dopaminergic neurons. Al-Amri et al. demonstrated that EGCG significantly increased the number of TH-immunoreactive neurons in the midbrain of PD model rats by reducing the production of TNF-α and NO (170). Similarly, EGCG liposomes alleviated symptoms in a PD rat model by suppressing the expression of NO and TNF-α in microglia exhibiting an LPS-induced inflammatory phenotype (165). In summary, EGCG shows promise as a therapeutic and prophylactic agent for PD, exerting neuroprotective effects both in vivo and in vitro through the inhibition of neuroinflammation (Figure 4).
Figure 4. A schematic representation illustrates the neuroprotective effects of EGCG in Parkinson’s disease. EGCG exerts neuroprotection by inhibiting oxidative stress, neuronal apoptosis, and neuroinflammatory responses via diverse molecular mechanisms.
PD patients commonly exhibit reduced mitochondrial complex I activity and increased ROS production (190). This diminished function of proton pumps on mitochondria, coupled with decreased membrane voltage and the opening of permeability channels, initiates the apoptotic process. Deficiency in mitochondrial complex I can result in oxidative stress, heightening neuronal susceptibility to excitotoxic injury. The densely packed substantia nigra is particularly vulnerable to elevated oxidative stress compared to other brain regions. Under normal conditions, H2O2 generated by dopamine toxicity is neutralized by reduced glutathione, mitigating potential harm. However, in the remaining dopamine neurons of PD patients, ineffective scavenging of H2O2 may occur due to compensatory mechanisms, including accelerated toxicity production in dopamine metabolism, heightened monoamine oxidase (MAO)-B activity, and reduced glutathione levels (191). Excessive H2O2 reacts with Fe2+ via Fenton chemistry, yielding highly toxic hydroxyl radicals, culminating in lipid peroxidation and apoptosis of nigral neurons. This oxidative stress and mitochondrial dysfunction form a reciprocal relationship, perpetuating a vicious cycle.
The neuroprotective effects of EGCG, attributed to its antioxidant properties, have been observed in PD (Figure 4). Typically, α-synuclein localizes to the mitochondria-associated membrane, and its presence may disrupt mitochondrial function by promoting the formation of the mitochondrial permeability transition pore (mPTP), leading to mitochondrial membrane potential (MMP) loss, subsequent mitochondrial degradation, and ultimately cell death (192). Compounds capable of preserving mitochondrial activity are therefore deemed invaluable in combating PD. EGCG has been shown to safeguard mitochondrial function by preventing Ca2+ influx through voltage-gated calcium channels and mitochondrial Ca2+ uptake via the mitochondrial Ca2+ uniporter (159, 193). Furthermore, in vivo studies have demonstrated EGCG’s ability to reduce oxidative stress by decreasing serum protein carbonyls and mitigating neurotoxicity in the MPTP-induced mouse model of PD (166). Similarly, Pinto et al. reported that EGCG improved cognitive dysfunction induced by 6-OHDA in male Wistar rats. 6-OHDA is known to induce ROS generation. EGCG treatment reversed striatal oxidative stress and attenuated immunohistochemical alterations (194). In conclusion, EGCG has been shown to alleviate PD by inhibiting neurotoxin-induced oxidative stress injury both in vitro and in vivo.
When evaluating EGCG for clinical therapeutic applications, significant concerns arise regarding its safety, toxicity, and optimal dosage post-treatment. While numerous studies have highlighted EGCG’s beneficial impact on neurodegenerative diseases due to its antioxidant and anti-neurotoxic properties, others have reported adverse effects such as heightened oxidative stress and the generation of toxic EGCG metabolites (195–197). Hence, there is a critical need for systematic investigations into EGCG’s bioavailability, toxicity profile, and appropriate dosing regimens.
EGCG has been extensively investigated for its notable health-promoting effects, with particular focus on its neuroprotective properties. Despite these benefits, the bioavailability of EGCG is limited, posing a challenge for its clinical application in treating neurodegenerative diseases. Following oral administration, EGCG exhibits a mean peak plasma concentration between 1.3 and 2.2 h, a half-life ranging from 1.9 to 4.6 h, and is almost completely metabolized within 24 h (198). Pharmacokinetic studies reveal that merely 0.1% of the ingested EGCG dose reaches detectable levels in the bloodstream at its peak concentration time (Tmax) in healthy individuals (39, 199). This minimal absorption occurs primarily through passive diffusion (paracellular and transcellular diffusion) in the small intestine, while the remaining EGCG reaches the colon to be degraded by intestinal microbial enzymes (198, 200, 201). EGCG undergoes phase II metabolism in enterocytes and hepatocytes following ingestion (199, 202). The polyphenolic hydroxyl structure of EGCG facilitates binding reactions such as methylation, glucuronidation, sulfation, and cysteine binding, contributing to its limited bioavailability (203). Upon entering the colon, EGCG encounters rapid hydrolysis of conjugate groups like glucuronides and sulfates by colonic microbiota. Subsequently, glycosides are released and further catabolized into ring cleavage products and low molecular weight phenolic acids (198, 204). While absorption studies traditionally focus on the small intestine, phenolic acid metabolites degraded by colonic microorganisms constitute approximately 40% of the ingested EGCG, underscoring the significant role of colonic metabolism in EGCG bioavailability.
Keiko Unno et al. demonstrated that EGCG can penetrate the BBB to access the brain parenchyma, influencing neuronal cell proliferation and neurogenesis, thus potentially mitigating neurodegenerative diseases (34). There are two common views on the impact of EGCG bioavailability on neuroprotection. We know that only a small fraction of oral EGCG is absorbed into the circulation. In addition, Shimizu et al. found that oral EGCG accumulates primarily in the gut (50%), with less than 0.01% distributed in the liver, blood, and brain (205). Upon comparison of the distribution of EGCG in mice following oral and intravenous administration, it was observed that the majority of orally administered EGCG entered the bloodstream in its glucuronidated form. Additionally, a significant portion of EGCG accumulated in the small intestine and colon (206). Intravenously injected EGCG was rapidly distributed in an uncoupled state in other tissues such as brain, liver, and lung (207, 208). These findings underscore the notion that while intravenous EGCG achieves rapid tissue penetration, oral administration necessitates absorption through the intestine followed by redistribution to tissues and organs. Thus, intestinal absorption emerges as a critical factor limiting EGCG bioavailability and its potential neuroprotective effects across the BBB.
An alternative perspective posits that the gut harbors a substantial population of immune cells and neural networks, and EGCG has the potential to modulate signaling and functional disruptions in intestinal neuroimmune communication via the brain-gut axis (13, 209–211). This theory underscores the gut-brain axis as pivotal in brain injury, neuroinflammation, and related diseases, with microbiota signaling pathways playing a crucial role in neuroprotection (212, 213). It is known that gut microbes can metabolize EGCG into fission products that are more bioavailable and easier to pass through the BBB to exert neuroprotective effects (214). Moreover, evidence supporting EGCG’s neuroprotective effects via BBB-mediated anti-neuroinflammation and reduction of oxidative stress includes: (i) EGCG enhances dopamine neuron activity in the gut, decreases serotonin levels in the colon, and increases hippocampal 5-hydroxytryptamine levels by enhancing intestinal permeability (215–217). (ii) EGCG alleviates intestinal inflammation and repairs the intestinal barrier by altering the gut microbiome. The alteration of the gut microbiome ultimately results in the alleviation of neuroinflammation and neurodegenerative diseases by affecting physiological processes such as immune cell development and migration, amyloid deposition, BDNF and NMDA signaling (218–220). (iii) EGCG also impacts the metabolome of gut microbes, influencing short-chain fatty acids, secondary bile acids, and tryptophan-related metabolites (221, 222). These metabolites traverse the BBB and modulate the host’s nervous system.
Despite its limited oral bioavailability, EGCG can induce toxicity, particularly when administered in fasted states or at high doses. Numerous studies have questioned whether EGCG has a clinical therapeutic role, as well as concerns about EGCG toxicity during treatment of various neurodegenerative diseases. Multiple system atrophy (MSA) is a rare neurodegenerative disorder characterized by neuronal loss and gliosis in various regions of the CNS, including the striatum, olivocerebellum, and central autonomic structures (223). A histopathological hallmark of MSA is the presence of oligodendrocyte cytoplasmic inclusions containing misfolded and aggregated α-synuclein (223, 224). EGCG has been shown to inhibit α-synuclein aggregation and mitigate associated toxicity. Johannes Levin et al. conducted a randomized, double-blind, parallel-group, placebo-controlled clinical trial, which demonstrated that 48 weeks of EGCG treatment did not alter disease progression or provide clinical benefit in MSA (225). Two patients discontinued EGCG therapy due to severe hepatotoxicity during the trial (225). The study concluded that elevated transaminase concentrations at therapeutic doses greater than 1,200 mg would cause hepatotoxicity (225). However, the study affirms that EGCG is generally well tolerated in humans and supports the idea that EGCG therapy acting on the α-synuclein oligomer formation may be an effective target for the treatment of neurodegenerative diseases (225). Additionally, numerous animal studies have highlighted adverse effects of EGCG, particularly affecting the liver and kidneys (226–228). We focus on the hepatotoxicity and nephrotoxicity of EGCG and briefly summarize the other adverse effects of EGCG (gastrointestinal toxicity).
The liver is known to be the major drug metabolizing organ in the human body. Initially, K Nakagawa et al. examined the distribution of EGCG (500 mg/kg body weight) in the body after 1 h of oral administration in rats (227). They observed that EGCG concentrations were highest in the intestine, followed by the liver, with plasma levels approximately one-fourth of those in the liver and notably lower concentrations in the brain (227). Autopsy findings further confirmed EGCG induced hepatotoxicity, correlating the extent of liver damage with dosage, route, and duration of EGCG administration (228). Studies on oral EGCG toxicity have documented varying degrees of hepatotoxicity, ranging from mild elevation in liver enzymes (alanine aminotransferase (ALT) and aspartate aminotransferase (AST)) to severe hepatocellular necrosis and bile duct hyperplasia as therapeutic doses increased (229). Thus, it is evident that the liver is a significant target organ for EGCG toxicity.
Animal studies have shown that the severity of liver injury produced by EGCG treatment is related to dose, administration route, and treatment duration. Balaji Ramachandran et al. investigated the relationship between the adverse effects of EGCG treatment with dose and administration route by giving EGCG (108, 67.8, 21.1, and 6.6 mg/kg/d) orally or intraperitoneally to mice (230). Subcutaneous injection of 108 mg/kg EGCG resulted in severe hepatic parenchymal congestion, hepatocellular balloon-like degeneration, kupffer cell hyperplasia, and calcification (acute hepatitis); serum levels of bilirubin, AST, ALT, and ALP were markedly elevated, leading to mortality by the 8th day of treatment (230). Mice injected with 67.8 mg/kg EGCG subcutaneously exhibited moderate hepatic peritoneal and mild lobular inflammation; elevated serum AST and ALT levels were observed, with mortality occurring by day 16 of the experiment (230). In comparison to subcutaneous injection, oral administration of EGCG resulted in lower hepatotoxicity, with significant liver damage observed only in mice receiving 108 mg/kg EGCG orally. Notably, increasing EGCG doses correlated exclusively with hepatic toxicity, ranging from mild periportal inflammation to severe hepatitis (230). Similarly, Dongxu Wang et al. investigated the dose-dependent hepatotoxic effects of subcutaneously injected EGCG (55, 70, and 125 mg/kg/day) in mice (229). Their findings revealed that all mice injected with 125 mg/kg or 70 mg/kg EGCG succumbed within 2 days, showing severe hepatotoxicity characterized by elevated serum levels of ALT, AST, and 4-HNE, along with increased expression of Nrf2 target genes in the liver. Mice injected with 55 mg/kg EGCG exhibited hepatotoxic effects but survived the duration of the study (229). It has also been demonstrated that subcutaneous injection of 45 mg/kg/day of EGCG represents the maximum tolerated dose in mice, with long-term administration at this dose showing no impact on the body’s oxidative defense mechanisms (231). However, injections of 55 or 75 mg/kg/day of EGCG induced hepatotoxicity in mice, accompanied by inhibition of hepatic antioxidant enzymes and increased nuclear distribution of Nrf2 (231). Furthermore, repeated injections of 75 mg/kg/day of EGCG altered the oxidative defense mechanism, significantly reducing levels of SOD, catalase, and GPX (231). Subcutaneous injection of EGCG in mice at doses exceeding 100 mg/kg/day induces severe hepatotoxicity and dose-dependent mortality, with higher concentrations leading to accelerated death. This treatment also inhibits Nrf2 target gene expression and diminishes antioxidant defense capacity (231). Similarly, gavage administration of EGCG yielded comparable results: mice exhibited hepatic congestion and a slight elevation in ALT levels after receiving 750 mg/kg/day of EGCG for 5 consecutive days (228). Following gavage of 750 mg/kg/day of EGCG for 7 consecutive days, mice exhibited a significant increase in ALT, MDA, MT, and γH2AX levels in the liver, along with hepatocyte degeneration, resulting in a mortality rate of 75% (232). single gavage of 1,500 mg/kg of EGCG led to a 108-fold increase in ALT levels and an 85% mortality rate among mice (232). Metabolites EGCG-2′-cysteine and EGCG-2″-cysteine were detected in urine following high-dose gavage of EGCG (233). Notably, EGCG administered via diet was well tolerated and demonstrated reduced hepatotoxicity compared to gavage administration in animals (233). Studies administering EGCG to Beagles indicated that fasting increased the likelihood of hepatotoxicity compared to animals that were fed prior to treatment (234). These findings underscore the influence of dose, route of administration, treatment duration, and nutritional status on EGCG-induced hepatotoxicity.
Animal experiments have shown that EGCG induced hepatotoxicity correlates with changes in several oxidative stress markers in the body, including MDA, 4-HNE, MT, γH2AX, and Nrf2 (228, 229, 231, 235). MDA and 4-HNE are products of lipid peroxidation and serve as biochemical indicators of oxidative stress (228). MT and γH2AX are molecular markers associated with oxidative stress. All these biomarkers suggest that hepatotoxicity induced by EGCG treatment is largely induced by oxidative stress (201). Nrf2 functions as a crucial transcription factor in antioxidant defense. Under normal physiological conditions, Nrf2 is sequestered by Keap1; however, during oxidative stress, Nrf2 dissociates from Keap1 and translocates to the nucleus where it binds to antioxidant response elements. This activation of the Nrf2-ARE signaling pathway upregulates the expression of various antioxidant genes such as HO-1, GST, and NADP (H): NQO1 (231). The Nrf2-ARE signaling pathway activates and enhances the expression of downstream antioxidant enzymes, serving as a critical cellular defense mechanism against oxidative stress (236). This pathway, particularly in the liver, is pivotal in mitigating EGCG-induced hepatotoxicity (236). Animal studies have shown that subcutaneous injection of EGCG at 45 mg/kg/day in mice does not impair major hepatic antioxidant defenses but modestly increases hepatic expression of Nrf2 target genes (231). Conversely, injection of 75 mg/kg/day of EGCG inhibits major hepatic antioxidant enzymes while significantly elevating Nrf2 expression and its target genes (231). Injection of 100 mg/kg/day of EGCG notably suppresses the hepatic Nrf2 pathway (231). These findings indicate a biphasic response of Nrf2 to different EGCG doses. In summary, EGCG-induced hepatotoxicity involves the inhibition of major antioxidant enzymes, with the Nrf2 salvage pathway playing a crucial role in mitigating toxicity. However, this pathway becomes inhibited at higher concentrations of EGCG.
Nora O. Abdel Rasheed et al. investigated potential nephrotoxic effects of EGCG treatment in diabetic mice, a crucial concern due to the kidney’s vulnerability in diabetes (237). Diabetic mice injected with 100 mg/kg EGCG daily for 4 days exhibited decreased resistance to oxidative stress, as indicated by elevated NADPH oxidase levels and reduced expression of Nrf2, HO-1, and HSP90 (237). Serum levels of CYS-C and NGAL were significantly elevated, and histopathological analysis confirmed EGCG-induced renal injury in diabetic mice (237). Similarly, another study demonstrated nephrotoxicity in colitis mice treated with green tea extract containing 35% EGCG, evidenced by increased serum creatinine levels (a nephropathy biomarker), and elevated expression of antioxidant enzymes (HO-1 and NQO1) and HSP 90 (238). These findings collectively underscore the potential nephrotoxic effects of EGCG treatment, exacerbated by oxidative stress implicated in diabetes and its complications (239). Thus, caution is advised when considering EGCG supplements for diabetic patients, particularly at high doses.
In addition to nephrotoxicity and hepatotoxicity, numerous studies have documented gastrointestinal toxicity associated with EGCG administration, whether by gavage or in diet, in animal models (240–242). The severity of gastrointestinal effects varied with dosage, ranging from mild gastric erosion and vomiting to severe ulceration, hemorrhage, and epithelial necrosis. Notably, gastrointestinal toxicity was more pronounced in animals administered EGCG via gavage or when fasted, whereas administration via diet, water, or capsule resulted in milder effects (234, 243). In conclusion, treatment with EGCG at high doses or for prolonged duration may have adverse effects, and the above data suggest that the boundary between protective and toxic doses of EGCG may be narrow.
Another critical issue is establishing safe dosage levels of EGCG to optimize therapeutic efficacy while minimizing adverse effects. Current clinical studies on EGCG dosages vary widely, and extrapolation from animal dosages to humans is virtually impossible (244–255). Safety data from human studies indicate distinct toxicity thresholds for EGCG consumed as a beverage compared to capsules or tablets, necessitating separate consideration of safe intake levels. Studies have shown that ingestion of up to 676 mg of EGCG in capsules or tablets did not result in significant adverse effects in healthy adults or patients with various conditions (256). In addition, liver toxicity has been documented with the intake of 800 mg or 1,200 mg of EGCG (225, 253). However, considering that the pro-health benefits of EGCG are similar to those of nutrients. Jiang Hu et al. used an approach similar to the Institute of Medicine (IOM) nutrient risk assessment to determine the safe intake of EGCG (257). The results indicated that the safe intake of EGCG in capsules or tablets for adults is 338 mg/day (257). This safe dose is consistent with the dose derived from animal data (322 mg/day) and is consistent with recent doses proposed by Yates et al. (258) and Dekant et al. (195). Regarding the toxicity threshold for EGCG intake in the form of beverages, the highest reported intake level of EGCG was 704 mg/day with no apparent adverse effects (245). For the current study, it is still uncertain what the standardized safe intake level of EGCG is, as the data currently available from human clinical studies may vary in terms of design, duration, and subject populations. However, the results of the current analyses suggest that diluting and/or slowing the rate of systemic administration of EGCG often appears to be better tolerated by the body. Even so, careful calculation of daily EGCG intake is important when EGCG is used as a dietary supplement. When other EGCG sources are available, EGCG intake may require health-based guidance. The use of EGCG as a clinical agent for neurodegenerative diseases still requires further evaluation of toxicity and dosage.
In conclusion, this review highlights the significant potential of EGCG, a prominent catechin abundant in green tea, as a therapeutic agent for neurodegenerative diseases. By targeting chronic neuroinflammation and oxidative stress, EGCG demonstrates promising neuroprotective effects in conditions such as AD and PD. Through its antioxidant properties and anti-inflammatory activities, EGCG shows efficacy in mitigating key pathological mechanisms associated with neurodegeneration. The comprehensive exploration of EGCG’s molecular mechanisms, including its modulation of autoimmune responses, nervous-immune system interactions, and inflammatory pathways, underscores its therapeutic relevance in AD and PD. Observational epidemiological studies and experimental investigations provide compelling evidence for EGCG’s neuroprotective effects, supporting its potential as a therapeutic intervention. Furthermore, EGCG’s ability to scavenge free radicals, chelate iron, and attenuate neuroinflammatory processes highlights its multifaceted mechanisms of action. Overall, EGCG emerges as a promising natural compound with the capacity to combat chronic neuroinflammation and oxidative stress, offering novel avenues for the development of neuroprotective strategies in the treatment of neurodegenerative disorders. Further research into EGCG’s therapeutic potential, including clinical trials and mechanistic studies, is warranted to fully elucidate its efficacy and safety profile in neurodegenerative diseases.
SL: Writing – original draft, Writing – review & editing. ZW: Writing – review & editing. GL: Supervision, Writing – review & editing. MC: Funding acquisition, Supervision, Writing – review & editing.
The author(s) declare financial support was received for the research, authorship, and/or publication of this article. This manuscript was supported by Hunan Provincial Science and Technology Department (2019TP2004).
We gratefully acknowledge the AI-powered technology used to enhance the article’s clarity, grammar, and overall quality.
The authors declare that the research was conducted in the absence of any commercial or financial relationships that could be construed as a potential conflict of interest.
All claims expressed in this article are solely those of the authors and do not necessarily represent those of their affiliated organizations, or those of the publisher, the editors and the reviewers. Any product that may be evaluated in this article, or claim that may be made by its manufacturer, is not guaranteed or endorsed by the publisher.
1. Kim, JM, and Heo, HJ. The roles of catechins in regulation of systemic inflammation. Food Sci Biotechnol. (2022) 31:957–70. doi: 10.1007/s10068-022-01069-0
2. Yang, CS, and Wang, X. Green tea and cancer prevention. Nutr Cancer. (2010) 62:931–7. doi: 10.1080/01635581.2010.509536
3. Huang, F, Zheng, X, Ma, X, Jiang, R, Zhou, W, Zhou, S, et al. Theabrownin from Pu-erh tea attenuates hypercholesterolemia via modulation of gut microbiota and bile acid metabolism. Nat Commun. (2019) 10:4971. doi: 10.1038/s41467-019-12896-x
4. Huang, HT, Cheng, TL, Lin, SY, Ho, CJ, Chyu, JY, Yang, RS, et al. Osteoprotective roles of Green tea catechins. Antioxidants (Basel). (2020) 9:1136. doi: 10.3390/antiox9111136
5. Ferenczyová, K, Kindernay, L, Vlkovičová, J, Kaločayová, B, Rajtík, T, and Barteková, M. Pharmacology of catechins in ischemia-reperfusion injury of the heart. Antioxidants (Basel). (2021) 10:1390. doi: 10.3390/antiox10091390
6. Filippini, T, Malavolti, M, Borrelli, F, Izzo, AA, Fairweather-Tait, SJ, Horneber, M, et al. Green tea (Camellia sinensis) for the prevention of cancer. Cochrane Database Syst Rev. (2020) 3:Cd005004. doi: 10.1002/14651858.CD005004.pub3
7. Kamal, DAM, Salamt, N, Zaid, SSM, and Mokhtar, MH. Beneficial effects of Green tea catechins on female reproductive disorders: a review. Molecules. (2021) 26:2675. doi: 10.3390/molecules26092675
8. Hayakawa, S, Ohishi, T, Miyoshi, N, Oishi, Y, Nakamura, Y, and Isemura, M. Anti-cancer effects of Green tea Epigallocatchin-3-gallate and coffee chlorogenic acid. Molecules. (2020) 25:4553. doi: 10.3390/molecules25194553
9. Almatroodi, SA, Almatroudi, A, Khan, AA, Alhumaydhi, FA, Alsahli, MA, and Rahmani, AH. Potential therapeutic targets of epigallocatechin gallate (EGCG), the Most abundant catechin in Green tea, and its role in the therapy of various types of cancer. Molecules. (2020) 25:3146. doi: 10.3390/molecules25143146
10. Braicu, C, Ladomery, MR, Chedea, VS, Irimie, A, and Berindan-Neagoe, I. The relationship between the structure and biological actions of green tea catechins. Food Chem. (2013) 141:3282–9. doi: 10.1016/j.foodchem.2013.05.122
11. Sidhu, D, Vasundhara, M, and Dey, P. The intestinal-level metabolic benefits of green tea catechins: mechanistic insights from pre-clinical and clinical studies. Phytomedicine. (2024) 123:155207. doi: 10.1016/j.phymed.2023.155207
12. Ntamo, Y, Jack, B, Ziqubu, K, Mazibuko-Mbeje, SE, Nkambule, BB, Nyambuya, TM, et al. Epigallocatechin gallate as a nutraceutical to potentially target the metabolic syndrome: novel insights into therapeutic effects beyond its antioxidant and anti-inflammatory properties. Crit Rev Food Sci Nutr. (2024) 64:87–109. doi: 10.1080/10408398.2022.2104805
13. Chen, Y, Liu, Z, and Gong, Y. Neuron-immunity communication: mechanism of neuroprotective effects in EGCG. Crit Rev Food Sci Nutr. (2023) 22:1–20. doi: 10.1080/10408398.2023.2212069
14. Yang, JZ, Zhang, KK, Liu, Y, Li, XW, Chen, LJ, Liu, JL, et al. Epigallocatechin-3-gallate ameliorates polystyrene microplastics-induced anxiety-like behavior in mice by modulating gut microbe homeostasis. Sci Total Environ. (2023) 892:164619. doi: 10.1016/j.scitotenv.2023.164619
15. Chiu, YH, Wu, YW, Hung, JI, and Chen, MC. Epigallocatechin gallate/L-ascorbic acid-loaded poly-γ-glutamate microneedles with antioxidant, anti-inflammatory, and immunomodulatory effects for the treatment of atopic dermatitis. Acta Biomater. (2021) 130:223–33. doi: 10.1016/j.actbio.2021.05.032
16. Spagnuolo, C, Moccia, S, and Russo, GL. Anti-inflammatory effects of flavonoids in neurodegenerative disorders. Eur J Med Chem. (2018) 153:105–15. doi: 10.1016/j.ejmech.2017.09.001
17. Kumari, S, Dhapola, R, and Reddy, DH. Apoptosis in Alzheimer's disease: insight into the signaling pathways and therapeutic avenues. Apoptosis. (2023) 28:943–57. doi: 10.1007/s10495-023-01848-y
18. Liu, G, Yang, C, Wang, X, Chen, X, Wang, Y, and Le, W. Oxygen metabolism abnormality and Alzheimer's disease: An update. Redox Biol. (2023) 68:102955. doi: 10.1016/j.redox.2023.102955
19. Li, Y, Xia, Y, Yin, S, Wan, F, Hu, J, Kou, L, et al. Targeting microglial α-synuclein/TLRs/NF-kappaB/NLRP3 inflammasome Axis in Parkinson's disease. Front Immunol. (2021) 12:719807. doi: 10.3389/fimmu.2021.719807
20. Langworth-Green, C, Patel, S, Jaunmuktane, Z, Jabbari, E, Morris, H, Thom, M, et al. Chronic effects of inflammation on tauopathies. Lancet Neurol. (2023) 22:430–42. doi: 10.1016/S1474-4422(23)00038-8
21. Sies, H. Oxidative eustress: the physiological role of oxidants. Sci China Life Sci. (2023) 66:1947–8. doi: 10.1007/s11427-023-2336-1
22. Zhang, W, Xiao, D, Mao, Q, and Xia, H. Role of neuroinflammation in neurodegeneration development. Signal Transduct Target Ther. (2023) 8:267. doi: 10.1038/s41392-023-01486-5
23. Gao, C, Jiang, J, Tan, Y, and Chen, S. Microglia in neurodegenerative diseases: mechanism and potential therapeutic targets. Signal Transduct Target Ther. (2023) 8:359. doi: 10.1038/s41392-023-01588-0
24. Chu, E, Mychasiuk, R, Hibbs, ML, and Semple, BD. Dysregulated phosphoinositide 3-kinase signaling in microglia: shaping chronic neuroinflammation. J Neuroinflammation. (2021) 18:276. doi: 10.1186/s12974-021-02325-6
25. Singh, D. Astrocytic and microglial cells as the modulators of neuroinflammation in Alzheimer's disease. J Neuroinflammation. (2022) 19:206. doi: 10.1186/s12974-022-02565-0
26. Patani, R, Hardingham, GE, and Liddelow, SA. Functional roles of reactive astrocytes in neuroinflammation and neurodegeneration. Nat Rev Neurol. (2023) 19:395–409. doi: 10.1038/s41582-023-00822-1
27. Yu, H, Chang, Q, Sun, T, He, X, Wen, L, An, J, et al. Metabolic reprogramming and polarization of microglia in Parkinson's disease: role of inflammasome and iron. Ageing Res Rev. (2023) 90:102032. doi: 10.1016/j.arr.2023.102032
28. Bao, H, Cao, J, Chen, M, Chen, M, Chen, W, Chen, X, et al. Biomarkers of aging. Sci China Life Sci. (2023) 66:893–1066. doi: 10.1007/s11427-023-2305-0
29. Chu, C, Deng, J, Man, Y, and Qu, Y. Green tea extracts Epigallocatechin-3-gallate for different treatments. Biomed Res Int. (2017) 2017:5615647. doi: 10.1155/2017/5615647
30. Yao, L, Yang, Y, Yang, X, and Rezaei, MJ. The interaction between nutraceuticals and gut microbiota: a novel therapeutic approach to prevent and treatment Parkinson's disease. Mol Neurobiol. (2024). doi: 10.1007/s12035-024-04151-2
31. Mandel, SA, Avramovich-Tirosh, Y, Reznichenko, L, Zheng, H, Weinreb, O, Amit, T, et al. Multifunctional activities of green tea catechins in neuroprotection. Modulation of cell survival genes, iron-dependent oxidative stress and PKC signaling pathway. Neurosignals. (2005) 14:46–60. doi: 10.1159/000085385
32. Kuriyama, S, Hozawa, A, Ohmori, K, Shimazu, T, Matsui, T, Ebihara, S, et al. Green tea consumption and cognitive function: a cross-sectional study from the Tsurugaya project. Am J Clin Nutr. (2006) 83:355–61. doi: 10.1093/ajcn/83.2.355
33. Hu, G, Bidel, S, Jousilahti, P, Antikainen, R, and Tuomilehto, J. Coffee and tea consumption and the risk of Parkinson's disease. Mov Disord. (2007) 22:2242–8. doi: 10.1002/mds.21706
34. Unno, K, and Nakamura, Y. Green tea suppresses brain aging. Molecules. (2021) 26:4897. doi: 10.3390/molecules26164897
35. Shirakami, Y, and Shimizu, M. Possible mechanisms of Green tea and its constituents against cancer. Molecules. (2018) 23:2284. doi: 10.3390/molecules23092284
36. Naware, NS, Ambatkar, SS, Kamble, TS, Bangar, S, Uppar, KB, Shirke, K, et al. A review focusing on the benefits of green tea catechins as nutraceuticals. Sci Phytochem. (2023) 2:1–12. doi: 10.58920/sciphy02020001
37. Della Via, FI, Alvarez, MC, Basting, RT, and Saad, STO. The effects of Green tea catechins in Hematological malignancies. Pharmaceuticals. (2023) 16:1021. doi: 10.3390/ph16071021
38. Mokra, D, Joskova, M, and Mokry, J. Therapeutic effects of green tea polyphenol (−)-Epigallocatechin-3-gallate (EGCG) in relation to molecular pathways controlling inflammation, oxidative stress, and apoptosis. Int J Mol Sci. (2022) 24:340. doi: 10.3390/ijms24010340
39. Zhang, S, Mao, B, Cui, S, Zhang, Q, Zhao, J, Tang, X, et al. Absorption, metabolism, bioactivity, and biotransformation of epigallocatechin gallate. Crit Rev Food Sci Nutr. (2023) 64:6546–66. doi: 10.1080/10408398.2023.2170972
40. Haddad, F, Mohammed, N, Gopalan, R, Ayoub, YA, Nasim, MT, and Assi, K. Development and optimisation of inhalable EGCG nano-liposomes as a potential treatment for pulmonary arterial hypertension by implementation of the design of experiments approach. Pharmaceutics. (2023) 15:539. doi: 10.3390/pharmaceutics15020539
41. Alam, M, Ali, S, Ashraf, GM, Bilgrami, AL, Yadav, DK, and Hassan, MI. Epigallocatechin 3-gallate: from green tea to cancer therapeutics. Food Chem. (2022) 379:132135. doi: 10.1016/j.foodchem.2022.132135
42. Shabab, T, Khanabdali, R, Moghadamtousi, SZ, Kadir, HA, and Mohan, G. Neuroinflammation pathways: a general review. Int J Neurosci. (2017) 127:624–33. doi: 10.1080/00207454.2016.1212854
43. Teleanu, RI, Chircov, C, Grumezescu, AM, Volceanov, A, and Teleanu, DM. Antioxidant therapies for neuroprotection—a review. J Clin Med. (2019) 8:1659. doi: 10.3390/jcm8101659
44. Wendimu, MY, and Hooks, SB. Microglia phenotypes in aging and neurodegenerative diseases. Cells. (2022) 11:2091. doi: 10.3390/cells11132091
45. Farkhondeh, T, Pourbagher-Shahri, AM, Ashrafizadeh, M, Folgado, SL, Rajabpour-Sanati, A, Khazdair, MR, et al. Green tea catechins inhibit microglial activation which prevents the development of neurological disorders. Neural Regen Res. (2020) 15:1792–8. doi: 10.4103/1673-5374.280300
46. Han, S, Yuan, X, Zhao, F, Manyande, A, Gao, F, Wang, J, et al. Activation of LXRs alleviates neuropathic pain-induced cognitive dysfunction by modulation of microglia polarization and synaptic plasticity via PI3K/AKT pathway. Inflamm Res. (2024) 73:157–74. doi: 10.1007/s00011-023-01826-9
47. Xin, Y, Tian, M, Deng, S, Li, J, Yang, M, Gao, J, et al. The key drivers of brain injury by systemic inflammatory responses after sepsis: microglia and neuroinflammation. Mol Neurobiol. (2023) 60:1369–90. doi: 10.1007/s12035-022-03148-z
48. Kwon, HS, and Koh, S-H. Neuroinflammation in neurodegenerative disorders: the roles of microglia and astrocytes. Transl Neurodegener. (2020) 9:42. doi: 10.1186/s40035-020-00221-2
49. Wang, Z, and Weaver, DF. Microglia and microglial-based receptors in the pathogenesis and treatment of Alzheimer’s disease. Int Immunopharmacol. (2022) 110:109070. doi: 10.1016/j.intimp.2022.109070
50. Franco, R, Lillo, A, Rivas-Santisteban, R, Reyes-Resina, I, and Navarro, G. Microglial adenosine receptors: from preconditioning to modulating the M1/M2 balance in activated cells. Cells. (2021) 10:1124. doi: 10.3390/cells10051124
51. Guo, S, Wang, H, and Yin, Y. Microglia polarization from M1 to M2 in neurodegenerative diseases. Front Aging Neurosci. (2022) 14:815347. doi: 10.3389/fnagi.2022.815347
52. Neal, ML, Beier, EE, Hossain, MM, Boyle, A, Zheng, J, Kim, C, et al. Voltage-gated proton channel Hv1 regulates neuroinflammation and dopaminergic neurodegeneration in Parkinson’s disease models. Antioxidants. (2023) 12:582. doi: 10.3390/antiox12030582
53. Jin, S, Park, M, and Song, JH. (−)-Epigallocatechin-3-gallate inhibits voltage-gated proton currents in BV2 microglial cells. Eur J Pharmacol. (2013) 698:154–60. doi: 10.1016/j.ejphar.2012.11.036
54. Qu, Z, Meng, F, Zhou, H, Li, J, Wang, Q, Wei, F, et al. NitroDIGE analysis reveals inhibition of protein S-nitrosylation by epigallocatechin gallates in lipopolysaccharide-stimulated microglial cells. J Neuroinflammation. (2014) 11:17. doi: 10.1186/1742-2094-11-17
55. Zwolak, I. Epigallocatechin gallate for management of heavy metal-induced oxidative stress: mechanisms of action, efficacy, and concerns. Int J Mol Sci. (2021) 22:4027. doi: 10.3390/ijms22084027
56. Rana, A, Samtiya, M, Dhewa, T, Mishra, V, and Aluko, RE. Health benefits of polyphenols: a concise review. J Food Biochem. (2022) 46:e14264. doi: 10.1111/jfbc.14264
57. Nikoo, M, Regenstein, JM, and Ahmadi Gavlighi, H. Antioxidant and antimicrobial activities of (−)-epigallocatechin-3-gallate (EGCG) and its potential to preserve the quality and safety of foods. Compr Rev Food Sci Food Saf. (2018) 17:732–53. doi: 10.1111/1541-4337.12346
58. Sies, H, Belousov, VV, Chandel, NS, Davies, MJ, Jones, DP, Mann, GE, et al. Defining roles of specific reactive oxygen species (ROS) in cell biology and physiology. Nat Rev Mol Cell Biol. (2022) 23:499–515. doi: 10.1038/s41580-022-00456-z
59. Thiruvengadam, M, Venkidasamy, B, Subramanian, U, Samynathan, R, Ali Shariati, M, Rebezov, M, et al. Bioactive compounds in oxidative stress-mediated diseases: targeting the NRF2/ARE signaling pathway and epigenetic regulation. Antioxidants. (2021) 10:1859. doi: 10.3390/antiox10121859
60. Wu, S, Liao, X, Zhu, Z, Huang, R, Chen, M, Huang, A, et al. Antioxidant and anti-inflammation effects of dietary phytochemicals: the Nrf2/NF-κB signalling pathway and upstream factors of Nrf2. Phytochemistry. (2022) 204:113429. doi: 10.1016/j.phytochem.2022.113429
61. Mandel, S, Amit, T, Reznichenko, L, Weinreb, O, and Youdim, MB. Green tea catechins as brain-permeable, natural iron chelators-antioxidants for the treatment of neurodegenerative disorders. Mol Nutr Food Res. (2006) 50:229–34. doi: 10.1002/mnfr.200500156
62. Olson, KR, Briggs, A, Devireddy, M, Iovino, NA, Skora, NC, Whelan, J, et al. Green tea polyphenolic antioxidants oxidize hydrogen sulfide to thiosulfate and polysulfides: a possible new mechanism underpinning their biological action. Redox Biol. (2020) 37:101731. doi: 10.1016/j.redox.2020.101731
63. Bawono, LC, Khairinisa, MA, Jiranusornkul, S, and Levita, J. The role of catechins of Camellia sinensis leaves in modulating antioxidant enzymes: a review and case study. J Appl Pharm Sci. (2023) 13:052–65.
64. Talebi, M, Talebi, M, Farkhondeh, T, Mishra, G, Ilgün, S, and Samarghandian, S. New insights into the role of the Nrf2 signaling pathway in green tea catechin applications. Phytother Res. (2021) 35:3078–112. doi: 10.1002/ptr.7033
65. Nagai, K, Jiang, MH, Hada, J, Nagata, T, Yajima, Y, Yamamoto, S, et al. (−)-epigallocatechin gallate protects against NO stress-induced neuronal damage after ischemia by acting as an anti-oxidant. Brain Res. (2002) 956:319–22. doi: 10.1016/S0006-8993(02)03564-3
66. Kurutas, EB. The importance of antioxidants which play the role in cellular response against oxidative/nitrosative stress: current state. Nutr J. (2015) 15:1–22. doi: 10.1186/s12937-016-0186-5
67. Hossen, I, Kaiqi, Z, Hua, W, Junsong, X, Mingquan, H, and Yanping, C. Epigallocatechin gallate (EGCG) inhibits lipopolysaccharide-induced inflammation in RAW 264.7 macrophage cells via modulating nuclear factor kappa-light-chain enhancer of activated B cells (NF-κ B) signaling pathway. Food Sci Nutr. (2023) 11:4634–50. doi: 10.1002/fsn3.3427
68. James, A, Wang, K, and Wang, Y. Therapeutic activity of green tea epigallocatechin-3-gallate on metabolic diseases and non-alcoholic fatty liver diseases: the current updates. Nutrients. (2023) 15:3022. doi: 10.3390/nu15133022
69. Weng, C-L, Chen, C-C, Tsou, H-H, Liu, T-Y, and Wang, H-T. Areca nut procyanidins prevent ultraviolet light B-induced photoaging via suppression of cyclooxygenase-2 and matrix metalloproteinases in mouse skin. Drug Chem Toxicol. (2022) 45:353–9. doi: 10.1080/01480545.2019.1696813
70. Erkkinen, MG, Kim, MO, and Geschwind, MD. Clinical neurology and epidemiology of the major neurodegenerative diseases. Cold Spring Harb Perspect Biol. (2018) 10:a033118. doi: 10.1101/cshperspect.a033118
71. Dugger, BN, and Dickson, DW. Pathology of neurodegenerative diseases. Cold Spring Harb Perspect Biol. (2017) 9:a028035. doi: 10.1101/cshperspect.a028035
72. Kovacs, GG. Molecular pathology of neurodegenerative diseases: principles and practice. J Clin Pathol. (2019) 72:725–35. doi: 10.1136/jclinpath-2019-205952
73. Radi, E, Formichi, P, Battisti, C, and Federico, A. Apoptosis and oxidative stress in neurodegenerative diseases. J Alzheimers Dis. (2014) 42:S125–52. doi: 10.3233/JAD-132738
74. Matthews, FE, Stephan, BC, Robinson, L, Jagger, C, Barnes, LE, Arthur, A, et al. A two decade dementia incidence comparison from the cognitive function and ageing studies I and II. Nat Commun. (2016) 7:11398. doi: 10.1038/ncomms11398
75. Chen, X-Y, Liu, C, Xue, Y, and Chen, L. Changed firing activity of nigra dopaminergic neurons in Parkinson's disease. Neurochem Int. (2023) 162:105465. doi: 10.1016/j.neuint.2022.105465
76. Sveinbjornsdottir, S. The clinical symptoms of Parkinson's disease. J Neurochem. (2016) 139:318–24. doi: 10.1111/jnc.13691
77. Maragakis, N.J., and Galvez-Jimenez, N., Epidemiology and pathogenesis of amyotrophic lateral sclerosis. Uptodate. Eichler AF (ed.) (2018).
78. Vildan, C, Sule, D, Turker, B, Hilmi, U, and Sibel, KB. Genetic alterations of C9orf72, SOD1, TARDBP, FUS, and UBQLN2 genes in patients with amyotrophic lateral sclerosis. Cogent Med. (2019) 6:1582400. doi: 10.1080/2331205X.2019.1582400
79. Zuccato, C, Valenza, M, and Cattaneo, E. Molecular mechanisms and potential therapeutical targets in Huntington's disease. Physiol Rev. (2010) 90:905–81. doi: 10.1152/physrev.00041.2009
80. Jimenez-Sanchez, M, Licitra, F, Underwood, BR, and Rubinsztein, DC. Huntington's disease: mechanisms of pathogenesis and therapeutic strategies. Cold Spring Harb Perspect Med. (2017) 7:a024240. doi: 10.1101/cshperspect.a024240
81. Farkhondeh, T, Yazdi, HS, and Samarghandian, S. The protective effects of Green tea catechins in the Management of Neurodegenerative Diseases: a review. Curr Drug Discov Technol. (2019) 16:57–65. doi: 10.2174/1570163815666180219115453
82. Fahn, S, and Cohen, G. The oxidant stress hypothesis in Parkinson's disease: evidence supporting it. Ann Neurol. (1992) 32:804–12. doi: 10.1002/ana.410320616
83. Berg, D, Gerlach, M, Youdim, MB, Double, KL, Zecca, L, Riederer, P, et al. Brain iron pathways and their relevance to Parkinson's disease. J Neurochem. (2001) 79:225–36. doi: 10.1046/j.1471-4159.2001.00608.x
84. Taylor, JM, Main, BS, and Crack, PJ. Neuroinflammation and oxidative stress: co-conspirators in the pathology of Parkinson’s disease. Neurochem Int. (2013) 62:803–19. doi: 10.1016/j.neuint.2012.12.016
85. Manoharan, RR, Prasad, A, Pospíšil, P, and Kzhyshkowska, J. ROS signaling in innate immunity via oxidative protein modifications. Front Immunol. (2024) 15:1359600. doi: 10.3389/fimmu.2024.1359600
86. Ballard, C, Gauthier, S, Corbett, A, Brayne, C, Aarsland, D, and Jones, E. Alzheimer's disease. Lancet. (2011) 377:1019–31. doi: 10.1016/S0140-6736(10)61349-9
87. Reiman, EM. Alzheimer's disease and other dementias: advances in 2013. Lancet Neurol. (2014) 13:3–5. doi: 10.1016/S1474-4422(13)70257-6
88. Daulatzai, MA. Cerebral hypoperfusion and glucose hypometabolism: key pathophysiological modulators promote neurodegeneration, cognitive impairment, and Alzheimer's disease. J Neurosci Res. (2017) 95:943–72. doi: 10.1002/jnr.23777
89. Sharifzadeh, M, Ranjbar, A, Hosseini, A, and Khanavi, M. The effect of Green tea extract on oxidative stress and spatial learning in streptozotocin-diabetic rats. Iran J Pharm Res. (2017) 16:201–9.
90. Amirpour, M, Mirshekar, MA, Sedaghat, G, Montazerifar, F, Shourestani, S, Arabmoazzen, S, et al. The effects of green tea on cognitive impairments in the rat model of Alzheimer's disease: protection against inflammatory and oxidative damage. Nutr Neurosci. (2021) 25:1–9. doi: 10.1080/1028415X.2021.2003946
91. Noguchi-Shinohara, M, Yuki, S, Dohmoto, C, Ikeda, Y, Samuraki, M, Iwasa, K, et al. Consumption of green tea, but not black tea or coffee, is associated with reduced risk of cognitive decline. PLoS One. (2014) 9:e96013. doi: 10.1371/journal.pone.0096013
92. Fernando, W, Somaratne, G, Goozee, KG, Williams, S, Singh, H, and Martins, RN. Diabetes and Alzheimer’s disease: can tea phytochemicals play a role in prevention? J Alzheimers Dis. (2017) 59:481–501. doi: 10.3233/JAD-161200
93. Yang, Q, Xiang, Y, Ma, G, Cao, M, Fang, Y, Xu, W, et al. A nomogram prediction model for mild cognitive impairment in non-dialysis outpatient patients with chronic kidney disease. Ren Fail. (2024) 46:2317450. doi: 10.1080/0886022X.2024.2317450
94. Wang, Z, Dong, B, Zeng, G, Li, J, Wang, W, Wang, B, et al. Is there an association between mild cognitive impairment and dietary pattern in Chinese elderly? Results from a cross-sectional population study. BMC Public Health. (2010) 10:1–7. doi: 10.1186/1471-2458-10-595
95. Lange, KW, Lange, KM, and Nakamura, Y. Green tea, epigallocatechin gallate and the prevention of Alzheimer’s disease: clinical evidence. Food Sci Human Wellness. (2022) 11:765–70. doi: 10.1016/j.fshw.2022.03.002
96. Payne, A, Nahashon, S, Taka, E, Adinew, GM, and Soliman, KF. Epigallocatechin-3-gallate (EGCG): New therapeutic perspectives for neuroprotection, aging, and neuroinflammation for the modern age. Biomolecules. (2022) 12:371. doi: 10.3390/biom12030371
97. Amirpour, M, Mirshekar, MA, Sedaghat, G, Montazerifar, F, Shourestani, S, Arabmoazzen, S, et al. The effects of green tea on cognitive impairments in the rat model of Alzheimer’s disease: protection against inflammatory and oxidative damage. Nutr Neurosci. (2022) 25:2659–67.
98. Nan, S, Wang, P, Zhang, Y, and Fan, J. Epigallocatechin-3-gallate provides protection against Alzheimer's disease-induced learning and memory impairments in rats. Drug Des Devel Ther. (2021) 15:2013–24. doi: 10.2147/DDDT.S289473
99. Bao, J, Liu, W, Zhou, HY, Gui, YR, Yang, YH, Wu, MJ, et al. Epigallocatechin-3-gallate alleviates cognitive deficits in APP/PS1 mice. Curr Med Sci. (2020) 40:18–27. doi: 10.1007/s11596-020-2142-z
100. Chang, X, Rong, C, Chen, Y, Yang, C, Hu, Q, Mo, Y, et al. (−)-Epigallocatechin-3-gallate attenuates cognitive deterioration in Alzheimer′s disease model mice by upregulating neprilysin expression. Exp Cell Res. (2015) 334:136–45. doi: 10.1016/j.yexcr.2015.04.004
101. Cano, A, Ettcheto, M, Chang, JH, Barroso, E, Espina, M, Kühne, BA, et al. Dual-drug loaded nanoparticles of Epigallocatechin-3-gallate (EGCG)/ascorbic acid enhance therapeutic efficacy of EGCG in a APPswe/PS1dE9 Alzheimer's disease mice model. J Control Release. (2019) 301:62–75. doi: 10.1016/j.jconrel.2019.03.010
102. Dragicevic, N, Smith, A, Lin, X, Yuan, F, Copes, N, Delic, V, et al. Green tea epigallocatechin-3-gallate (EGCG) and other flavonoids reduce Alzheimer's amyloid-induced mitochondrial dysfunction. J Alzheimers Dis. (2011) 26:507–21. doi: 10.3233/JAD-2011-101629
103. Mori, T, Koyama, N, Tan, J, Segawa, T, Maeda, M, and Town, T. Combined treatment with the phenolics (−)-epigallocatechin-3-gallate and ferulic acid improves cognition and reduces Alzheimer-like pathology in mice. J Biol Chem. (2019) 294:2714–31. doi: 10.1074/jbc.RA118.004280
104. Rasoolijazi, H, Joghataie, MT, Roghani, M, and Nobakht, M. The beneficial effect of (−)-epigallocatechin-3-gallate in an experimental model of Alzheimer's disease in rat: a behavioral analysis. Iran Biomed J. (2007) 11:237–43.
105. Lee, YJ, Choi, DY, Yun, YP, Han, SB, Oh, KW, and Hong, JT. Epigallocatechin-3-gallate prevents systemic inflammation-induced memory deficiency and amyloidogenesis via its anti-neuroinflammatory properties. J Nutr Biochem. (2013) 24:298–310. doi: 10.1016/j.jnutbio.2012.06.011
106. Guo, Y, Zhao, Y, Nan, Y, Wang, X, Chen, Y, and Wang, S. (−)-Epigallocatechin-3-gallate ameliorates memory impairment and rescues the abnormal synaptic protein levels in the frontal cortex and hippocampus in a mouse model of Alzheimer's disease. Neuroreport. (2017) 28:590–7. doi: 10.1097/WNR.0000000000000803
107. Liu, M, Chen, F, Sha, L, Wang, S, Tao, L, Yao, L, et al. (−)-Epigallocatechin-3-gallate ameliorates learning and memory deficits by adjusting the balance of TrkA/p75NTR signaling in APP/PS1 transgenic mice. Mol Neurobiol. (2014) 49:1350–63. doi: 10.1007/s12035-013-8608-2
108. Jia, N, Han, K, Kong, JJ, Zhang, XM, Sha, S, Ren, GR, et al. (−)-Epigallocatechin-3-gallate alleviates spatial memory impairment in APP/PS1 mice by restoring IRS-1 signaling defects in the hippocampus. Mol Cell Biochem. (2013) 380:211–8. doi: 10.1007/s11010-013-1675-x
109. Rezai-Zadeh, K, Shytle, D, Sun, N, Mori, T, Hou, H, Jeanniton, D, et al. Green tea epigallocatechin-3-gallate (EGCG) modulates amyloid precursor protein cleavage and reduces cerebral amyloidosis in Alzheimer transgenic mice. J Neurosci. (2005) 25:8807–14. doi: 10.1523/JNEUROSCI.1521-05.2005
110. Biasibetti, R, Tramontina, AC, Costa, AP, Dutra, MF, Quincozes-Santos, A, Nardin, P, et al. Green tea (−)epigallocatechin-3-gallate reverses oxidative stress and reduces acetylcholinesterase activity in a streptozotocin-induced model of dementia. Behav Brain Res. (2013) 236:186–93. doi: 10.1016/j.bbr.2012.08.039
111. Chen, T, Yang, Y, Zhu, S, Lu, Y, Zhu, L, Wang, Y, et al. Inhibition of Aβ aggregates in Alzheimer’s disease by epigallocatechin and epicatechin-3-gallate from green tea. Bioorg Chem. (2020) 105:104382. doi: 10.1016/j.bioorg.2020.104382
112. Luo, M, Gan, R-Y, Li, B-Y, Mao, Q-Q, Shang, A, Xu, X-Y, et al. Effects and mechanisms of tea on Parkinson’s disease, Alzheimer’s disease and depression. Food Rev Int. (2023) 39:278–306.
113. Lee, JW, Lee, YK, Ban, JO, Ha, TY, Yun, YP, Han, SB, et al. Green tea (−)-epigallocatechin-3-gallate inhibits beta-amyloid-induced cognitive dysfunction through modification of secretase activity via inhibition of ERK and NF-kappaB pathways in mice. J Nutr. (2009) 139:1987–93. doi: 10.3945/jn.109.109785
114. Tarkowski, E, Andreasen, N, Tarkowski, A, and Blennow, K. Intrathecal inflammation precedes development of Alzheimer's disease. J Neurol Neurosurg Psychiatry. (2003) 74:1200–5. doi: 10.1136/jnnp.74.9.1200
115. Brosseron, F, Krauthausen, M, Kummer, M, and Heneka, MT. Body fluid cytokine levels in mild cognitive impairment and Alzheimer's disease: a comparative overview. Mol Neurobiol. (2014) 50:534–44. doi: 10.1007/s12035-014-8657-1
116. Yan, LJ, Xiao, M, Chen, R, and Cai, Z. Metabolic dysfunction of astrocyte: An initiating factor in Beta-amyloid pathology? Aging Neurodegener. (2013) 1:7–14.
117. Hickman, S, Izzy, S, Sen, P, Morsett, L, and El Khoury, J. Microglia in neurodegeneration. Nat Neurosci. (2018) 21:1359–69. doi: 10.1038/s41593-018-0242-x
118. Kempuraj, D, Thangavel, R, Natteru, PA, Selvakumar, GP, Saeed, D, Zahoor, H, et al. Neuroinflammation induces neurodegeneration. J Neurol Neurosurg Spine. (2016) 1:1.
119. Glass, CK, Saijo, K, Winner, B, Marchetto, MC, and Gage, FH. Mechanisms underlying inflammation in neurodegeneration. Cell. (2010) 140:918–34. doi: 10.1016/j.cell.2010.02.016
120. Cheng-Chung Wei, J., and Huang, H.C., W.J. Chen, C., Huang, N., Peng, C.H., and Lin, C.L., Epigallocatechin gallate attenuates amyloid β-induced inflammation and neurotoxicity in EOC 13.31 microglia. Eur J Pharmacol 770 (2016) 16–24. doi: 10.1016/j.ejphar.2015.11.048
121. Cascella, M, Bimonte, S, Muzio, MR, Schiavone, V, and Cuomo, A. The efficacy of Epigallocatechin-3-gallate (green tea) in the treatment of Alzheimer's disease: an overview of pre-clinical studies and translational perspectives in clinical practice. Infect Agent Cancer. (2017) 12:36. doi: 10.1186/s13027-017-0145-6
122. Rezai-Zadeh, K, Arendash, GW, Hou, H, Fernandez, F, Jensen, M, Runfeldt, M, et al. Green tea epigallocatechin-3-gallate (EGCG) reduces beta-amyloid mediated cognitive impairment and modulates tau pathology in Alzheimer transgenic mice. Brain Res. (2008) 1214:177–87. doi: 10.1016/j.brainres.2008.02.107
123. Li, Q, Gordon, M, Tan, J, and Morgan, D. Oral administration of green tea epigallocatechin-3-gallate (EGCG) reduces amyloid beta deposition in transgenic mouse model of Alzheimer's disease. Exp Neurol. (2006) 198:576. doi: 10.1016/j.expneurol.2006.02.062
124. Tchekalarova, J, and Tzoneva, R. Oxidative stress and aging as risk factors for Alzheimer’s disease and Parkinson’s disease: the role of the antioxidant melatonin. Int J Mol Sci. (2023) 24:3022. doi: 10.3390/ijms24033022
125. Jomova, K, Alomar, SY, Alwasel, SH, Nepovimova, E, Kuca, K, and Valko, M. Several lines of antioxidant defense against oxidative stress: antioxidant enzymes, nanomaterials with multiple enzyme-mimicking activities, and low-molecular-weight antioxidants. Arch Toxicol. (2024) 98:1323–67. doi: 10.1007/s00204-024-03696-4
126. Panigrahi, LL, Shekhar, S, Sahoo, B, and Arakha, M. Adsorption of antimicrobial peptide onto chitosan-coated iron oxide nanoparticles fosters oxidative stress triggering bacterial cell death. RSC Adv. (2023) 13:25497–507. doi: 10.1039/D3RA04070D
127. Li, Y, and Wang, X. The role of DNA and RNA guanosine oxidation in cardiovascular diseases. Pharmacol Res. (2024) 204:107187. doi: 10.1016/j.phrs.2024.107187
128. Fu, Y, He, Y, Phan, K, Bhatia, S, Pickford, R, Wu, P, et al. Increased unsaturated lipids underlie lipid peroxidation in synucleinopathy brain. Acta Neuropathol Commun. (2022) 10:165. doi: 10.1186/s40478-022-01469-7
129. Siddiqui, N, Sharma, A, Kesharwani, A, and Parihar, VK. Exploring role of natural compounds in molecular alterations associated with brain ageing: a perspective towards nutrition for ageing brain. Ageing Res Rev. (2024) 97:102282. doi: 10.1016/j.arr.2024.102282
130. Lim, EY, Lee, S-Y, Shin, HS, and Kim, G-D. Reactive oxygen species and strategies for antioxidant intervention in acute respiratory distress syndrome. Antioxidants. (2023) 12:2016. doi: 10.3390/antiox12112016
131. Gandhi, S, and Abramov, AY. Mechanism of oxidative stress in neurodegeneration. Oxidative Med Cell Longev. (2012) 2012:428010. doi: 10.1155/2012/428010
132. Praticò, D. Evidence of oxidative stress in Alzheimer's disease brain and antioxidant therapy: lights and shadows. Ann N Y Acad Sci. (2008) 1147:70–8. doi: 10.1196/annals.1427.010
133. Haque, AM, Hashimoto, M, Katakura, M, Hara, Y, and Shido, O. Green tea catechins prevent cognitive deficits caused by Abeta1-40 in rats. J Nutr Biochem. (2008) 19:619–26. doi: 10.1016/j.jnutbio.2007.08.008
134. Weinreb, O, Amit, T, Mandel, S, and Youdim, MB. Neuroprotective molecular mechanisms of (−)-epigallocatechin-3-gallate: a reflective outcome of its antioxidant, iron chelating and neuritogenic properties. Genes Nutr. (2009) 4:283–96. doi: 10.1007/s12263-009-0143-4
135. Du, K, Liu, M, Zhong, X, Yao, W, Xiao, Q, Wen, Q, et al. Epigallocatechin gallate reduces amyloid β-induced neurotoxicity via inhibiting endoplasmic reticulum stress-mediated apoptosis. Mol Nutr Food Res. (2018) 62:e1700890. doi: 10.1002/mnfr.201700890
136. He, Y, Cui, J, Lee, JC, Ding, S, Chalimoniuk, M, Simonyi, A, et al. Prolonged exposure of cortical neurons to oligomeric amyloid-β impairs NMDA receptor function via NADPH oxidase-mediated ROS production: protective effect of green tea (−)-epigallocatechin-3-gallate. ASN Neuro. (2011) 3:e00050. doi: 10.1042/AN20100025
137. Aggarwal, BB, and Harikumar, KB. Potential therapeutic effects of curcumin, the anti-inflammatory agent, against neurodegenerative, cardiovascular, pulmonary, metabolic, autoimmune and neoplastic diseases. Int J Biochem Cell Biol. (2009) 41:40–59. doi: 10.1016/j.biocel.2008.06.010
138. Wu, KJ, Hsieh, MT, Wu, CR, Wood, WG, and Chen, YF. Green tea extract ameliorates learning and memory deficits in ischemic rats via its active component polyphenol Epigallocatechin-3-gallate by modulation of oxidative stress and neuroinflammation. Evid Based Complement Alternat Med. (2012) 2012:163106. doi: 10.1155/2012/163106
139. Berra, E, Municio, MM, Sanz, L, Frutos, S, Diaz-Meco, MT, and Moscat, J. Positioning atypical protein kinase C isoforms in the UV-induced apoptotic signaling cascade. Mol Cell Biol. (1997) 17:4346–54. doi: 10.1128/MCB.17.8.4346
140. Pervin, M, Unno, K, Ohishi, T, Tanabe, H, Miyoshi, N, and Nakamura, Y. Beneficial effects of Green tea catechins on neurodegenerative diseases. Molecules. (2018) 23:1297. doi: 10.3390/molecules23061297
141. Levites, Y, Amit, T, Mandel, S, and Youdim, MB. Neuroprotection and neurorescue against Abeta toxicity and PKC-dependent release of nonamyloidogenic soluble precursor protein by green tea polyphenol (−)-epigallocatechin-3-gallate. FASEB J. (2003) 17:952–4. doi: 10.1096/fj.02-0881fje
142. Tanner, CM, and Ben-Shlomo, Y. Epidemiology of Parkinson's disease. Adv Neurol. (1999) 80:153–9.
143. Chahra, C, Anis, H, Bissene, D, Mejda, S, Jihène, M, Salma, N, et al. The effect of Origanum majorana tea on motor and non-motor symptoms in patients with idiopathic Parkinson's disease: a randomized controlled pilot study. Parkinsonism Relat Disord. (2021) 91:23–7. doi: 10.1016/j.parkreldis.2021.08.013
144. Agid, Y. Parkinson's disease: pathophysiology. Lancet. (1991) 337:1321–4. doi: 10.1016/0140-6736(91)92989-F
145. Lang, AE, and Lozano, AM. Parkinson's disease. First of two parts. N Engl J Med. (1998) 339:1044–53. doi: 10.1056/NEJM199810083391506
146. Hattoria, N, Wanga, M, Taka, H, Fujimura, T, Yoritaka, A, Kubo, S, et al. Toxic effects of dopamine metabolism in Parkinson's disease. Parkinsonism Relat Disord. (2009) 15:Suppl 1, S35–S38. doi: 10.1016/S1353-8020(09)70010-0
147. Barranco Quintana, JL, Allam, MF, Del Castillo, AS, and Navajas, RF. Parkinson's disease and tea: a quantitative review. J Am Coll Nutr. (2009) 28:1–6. doi: 10.1080/07315724.2009.10719754
148. Tabatabaei, NH, Babakhani, B, Tabatabaei, AH, Vahabi, Z, and Soltanzadeh, A. Non-genetic factors associated with the risk of Parkinson's disease in Iranian patients. Funct Neurol. (2013) 28:107–13. doi: 10.11138/FNeur/2013.28.2.107
149. Checkoway, H, Powers, K, Smith-Weller, T, Franklin, GM, Longstreth, WT Jr, and Swanson, PD. Parkinson's disease risks associated with cigarette smoking, alcohol consumption, and caffeine intake. Am J Epidemiol. (2002) 155:732–8. doi: 10.1093/aje/155.8.732
150. Tan, EK, Tan, C, Fook-Chong, SM, Lum, SY, Chai, A, Chung, H, et al. Dose-dependent protective effect of coffee, tea, and smoking in Parkinson's disease: a study in ethnic Chinese. J Neurol Sci. (2003) 216:163–7. doi: 10.1016/j.jns.2003.07.006
151. Kandinov, B, Giladi, N, and Korczyn, AD. Smoking and tea consumption delay onset of Parkinson's disease. Parkinsonism Relat Disord. (2009) 15:41–6. doi: 10.1016/j.parkreldis.2008.02.011
152. Biosa, A, Arduini, I, Soriano, ME, Giorgio, V, Bernardi, P, Bisaglia, M, et al. Dopamine oxidation products as mitochondrial endotoxins, a potential molecular mechanism for preferential neurodegeneration in Parkinson's disease. ACS Chem Neurosci. (2018) 9:2849–58. doi: 10.1021/acschemneuro.8b00276
153. Zhou, ZD, Xie, SP, Saw, WT, Ho, PGH, Wang, H, Lei, Z, et al. The therapeutic implications of tea polyphenols against dopamine (DA) neuron degeneration in Parkinson's disease (PD). Cells. (2019) 8:911. doi: 10.3390/cells8080911
154. Lin, SM, Wang, SW, Ho, SC, and Tang, YL. Protective effect of green tea (−)-epigallocatechin-3-gallate against the monoamine oxidase B enzyme activity increase in adult rat brains. Nutrition. (2010) 26:1195–200. doi: 10.1016/j.nut.2009.11.022
155. Malar, DS, Prasanth, MI, Brimson, JM, Sharika, R, Sivamaruthi, BS, Chaiyasut, C, et al. Neuroprotective properties of Green tea (Camellia sinensis) in Parkinson's disease: a review. Molecules. (2020) 25:3926. doi: 10.3390/molecules25173926
156. Salari, S, and Bagheri, M. In vivo, in vitro and pharmacologic models of Parkinson's disease. Physiol Res. (2019) 68:17–24. doi: 10.33549/physiolres.933895
157. Magrinelli, F, Picelli, A, Tocco, P, Federico, A, Roncari, L, Smania, N, et al. Pathophysiology of motor dysfunction in Parkinson's disease as the rationale for drug treatment and rehabilitation. Parkinsons Dis. (2016) 2016:9832839. doi: 10.1155/2016/9832839
158. Levites, Y, Weinreb, O, Maor, G, Youdim, MB, and Mandel, S. Green tea polyphenol (−)-epigallocatechin-3-gallate prevents N-methyl-4-phenyl-1,2,3,6-tetrahydropyridine-induced dopaminergic neurodegeneration. J Neurochem. (2001) 78:1073–82. doi: 10.1046/j.1471-4159.2001.00490.x
159. Siddique, YH, Jyoti, S, and Naz, F. Effect of epicatechin gallate dietary supplementation on transgenic drosophila model of Parkinson's disease. J Diet Suppl. (2014) 11:121–30. doi: 10.3109/19390211.2013.859207
160. Zhou, T, Zhu, M, and Liang, Z. (−)-Epigallocatechin-3-gallate modulates peripheral immunity in the MPTP-induced mouse model of Parkinson's disease. Mol Med Rep. (2018) 17:4883–8. doi: 10.3892/mmr.2018.8470
161. Picca, A, Guerra, F, Calvani, R, Romano, R, Coelho-Júnior, HJ, Bucci, C, et al. Mitochondrial dysfunction, protein misfolding and neuroinflammation in Parkinson’s disease: roads to biomarker discovery. Biomol Ther. (2021) 11:1508. doi: 10.3390/biom11101508
162. Simpson, DS, and Oliver, PL. ROS generation in microglia: understanding oxidative stress and inflammation in neurodegenerative disease. Antioxidants. (2020) 9:743. doi: 10.3390/antiox9080743
163. Cheng, CY, Barro, L, Tsai, ST, Feng, TW, Wu, XY, Chao, CW, et al. Epigallocatechin-3-gallate-loaded liposomes favor anti-inflammation of microglia cells and promote neuroprotection. Int J Mol Sci. (2021) 22:3037. doi: 10.3390/ijms22063037
164. Martinez-Perez, DA, Jimenez-Del-Rio, M, and Velez-Pardo, C. Epigallocatechin-3-gallate protects and prevents paraquat-induced oxidative stress and neurodegeneration in knockdown dj-1-β Drosophila melanogaster. Neurotox Res. (2018) 34:401–16. doi: 10.1007/s12640-018-9899-x
165. Tseng, HC, Wang, MH, Chang, KC, Soung, HS, Fang, CH, Lin, YW, et al. Protective effect of (−)Epigallocatechin-3-gallate on rotenone-induced parkinsonism-like symptoms in rats. Neurotox Res. (2020) 37:669–82. doi: 10.1007/s12640-019-00143-6
166. Xu, Q, Langley, M, Kanthasamy, AG, and Reddy, MB. Epigallocatechin gallate has a Neurorescue effect in a mouse model of Parkinson disease. J Nutr. (2017) 147:1926–31. doi: 10.3945/jn.117.255034
167. Shen, J, Xie, J, Ye, L, Mao, J, Sun, S, Chen, W, et al. Neuroprotective effect of green tea extract (−)-epigallocatechin-3-gallate in a preformed fibril-induced mouse model of Parkinson's disease. Neuroreport. (2024) 35:421–30. doi: 10.1097/WNR.0000000000002027
168. Choi, JY, Park, CS, Kim, DJ, Cho, MH, Jin, BK, Pie, JE, et al. Prevention of nitric oxide-mediated 1-methyl-4-phenyl-1,2,3,6-tetrahydropyridine-induced Parkinson's disease in mice by tea phenolic epigallocatechin 3-gallate. Neurotoxicology. (2002) 23:367–74. doi: 10.1016/S0161-813X(02)00079-7
169. Kim, JS, Kim, JM, Jeong-Ja, O, and Jeon, BS. Inhibition of inducible nitric oxide synthase expression and cell death by (−)-epigallocatechin-3-gallate, a green tea catechin, in the 1-methyl-4-phenyl-1,2,3,6-tetrahydropyridine mouse model of Parkinson's disease. J Clin Neurosci. (2010) 17:1165–8. doi: 10.1016/j.jocn.2010.01.042
170. Al-Amri, JS, Hagras, MM, and Mohamed, IM. Effect of epigallocatechin-3-gallate on inflammatory mediators release in LPS-induced Parkinson's disease in rats. Indian J Exp Biol. (2013) 51:357–62.
171. Kang, KS, Wen, Y, Yamabe, N, Fukui, M, Bishop, SC, and Zhu, BT. Dual beneficial effects of (−)-epigallocatechin-3-gallate on levodopa methylation and hippocampal neurodegeneration: in vitro and in vivo studies. PLoS One. (2010) 5:e11951. doi: 10.1371/journal.pone.0011951
172. Sergi, CM. Epigallocatechin gallate for Parkinson's disease. Clin Exp Pharmacol Physiol. (2022) 49:1029–41. doi: 10.1111/1440-1681.13691
173. Singh, A, Kukreti, R, Saso, L, and Kukreti, S. Oxidative stress: a key modulator in neurodegenerative diseases. Molecules. (2019) 24:1583. doi: 10.3390/molecules24081583
174. Tang, Y, and Le, W. Differential roles of M1 and M2 microglia in neurodegenerative diseases. Mol Neurobiol. (2016) 53:1181–94. doi: 10.1007/s12035-014-9070-5
175. Zhang, W, Wang, T, Pei, Z, Miller, DS, Wu, X, Block, ML, et al. Aggregated alpha-synuclein activates microglia: a process leading to disease progression in Parkinson's disease. FASEB J. (2005) 19:533–42. doi: 10.1096/fj.04-2751com
176. Hirsch, EC, and Hunot, S. Neuroinflammation in Parkinson's disease: a target for neuroprotection? Lancet Neurol. (2009) 8:382–97. doi: 10.1016/S1474-4422(09)70062-6
177. Rojanathammanee, L, Murphy, EJ, and Combs, CK. Expression of mutant alpha-synuclein modulates microglial phenotype in vitro. J Neuroinflammation. (2011) 8:44. doi: 10.1186/1742-2094-8-44
178. Tang, Y, Li, T, Li, J, Yang, J, Liu, H, Zhang, XJ, et al. Jmjd3 is essential for the epigenetic modulation of microglia phenotypes in the immune pathogenesis of Parkinson's disease. Cell Death Differ. (2014) 21:369–80. doi: 10.1038/cdd.2013.159
179. Luk, KC, Kehm, V, Carroll, J, Zhang, B, O'Brien, P, Trojanowski, JQ, et al. Pathological α-synuclein transmission initiates Parkinson-like neurodegeneration in nontransgenic mice. Science. (2012) 338:949–53. doi: 10.1126/science.1227157
180. Acuña, L, Hamadat, S, Corbalán, NS, González-Lizárraga, F, Dos-Santos-Pereira, M, Rocca, J, et al. Rifampicin and its derivative rifampicin quinone reduce microglial inflammatory responses and neurodegeneration induced in vitro by α-synuclein fibrillary aggregates. Cells. (2019) 8:776. doi: 10.3390/cells8080776
181. Duffy, MF, Collier, TJ, Patterson, JR, Kemp, CJ, Luk, KC, Tansey, MG, et al. Lewy body-like alpha-synuclein inclusions trigger reactive microgliosis prior to nigral degeneration. J Neuroinflammation. (2018) 15:129. doi: 10.1186/s12974-018-1171-z
182. Krashia, P, Cordella, A, Nobili, A, La Barbera, L, Federici, M, Leuti, A, et al. Blunting neuroinflammation with resolvin D1 prevents early pathology in a rat model of Parkinson's disease. Nat Commun. (2019) 10:3945. doi: 10.1038/s41467-019-11928-w
183. Cardinale, A, and Calabrese, V. The intricate debate on neurodegeneration and neuroinflammation in Parkinson's disease: which came first? Neural Regen Res. (2023) 18:125–6. doi: 10.4103/1673-5374.343895
184. Choi, I, Zhang, Y, Seegobin, SP, Pruvost, M, Wang, Q, Purtell, K, et al. Microglia clear neuron-released α-synuclein via selective autophagy and prevent neurodegeneration. Nat Commun. (2020) 11:1386. doi: 10.1038/s41467-020-15119-w
185. Kawahata, I, Finkelstein, DI, and Fukunaga, K. Pathogenic impact of α-synuclein phosphorylation and its kinases in α-Synucleinopathies. Int J Mol Sci. (2022) 23:6216. doi: 10.3390/ijms23116216
186. Ghanem, SS, Majbour, NK, Vaikath, NN, Ardah, MT, Erskine, D, Jensen, NM, et al. α-Synuclein phosphorylation at serine 129 occurs after initial protein deposition and inhibits seeded fibril formation and toxicity. Proc Natl Acad Sci USA. (2022) 119:e2109617119. doi: 10.1073/pnas.2109617119
187. Xia, Y, Zhang, G, Kou, L, Yin, S, Han, C, Hu, J, et al. Reactive microglia enhance the transmission of exosomal α-synuclein via toll-like receptor 2. Brain. (2021) 144:2024–37. doi: 10.1093/brain/awab122
188. Li, R, Huang, YG, Fang, D, and Le, WD. (−)-epigallocatechin gallate inhibits lipopolysaccharide-induced microglial activation and protects against inflammation-mediated dopaminergic neuronal injury. J Neurosci Res. (2004) 78:723–31. doi: 10.1002/jnr.20315
189. Özduran, G, Becer, E, Vatansever, HS, and Yücecan, S. Neuroprotective effects of catechins in an experimental Parkinson's disease model and SK-N-AS cells: evaluation of cell viability, anti-inflammatory and anti-apoptotic effects. Neurol Res. (2022) 44:511–23. doi: 10.1080/01616412.2021.2024715
190. Tryphena, KP, Nikhil, US, Pinjala, P, Srivastava, S, Singh, SB, and Khatri, DK. Mitochondrial complex I as a pathologic and therapeutic target for Parkinson’s disease. ACS Chem Neurosci. (2023) 14:1356–68. doi: 10.1021/acschemneuro.2c00819
191. Luk, KC. Oxidative stress and α-synuclein conspire in vulnerable neurons to promote Parkinson’s disease progression. J Clin Invest. (2019). 129:3530–3531. doi: 10.1172/JCI130351
192. Feng, ST, Wang, ZZ, Yuan, YH, Sun, HM, Chen, NH, and Zhang, Y. Update on the association between alpha-synuclein and tau with mitochondrial dysfunction: implications for Parkinson's disease. Eur J Neurosci. (2021) 53:2946–59. doi: 10.1111/ejn.14699
193. Ludtmann, MHR, Angelova, PR, Horrocks, MH, Choi, ML, Rodrigues, M, Baev, AY, et al. α-Synuclein oligomers interact with ATP synthase and open the permeability transition pore in Parkinson's disease. Nat Commun. (2018) 9:2293. doi: 10.1038/s41467-018-04422-2
194. Bitu Pinto, N, da Silva Alexandre, B, Neves, KR, Silva, AH, Leal, LK, and Viana, GS. Neuroprotective properties of the standardized extract from Camellia sinensis (Green tea) and its Main bioactive components, epicatechin and epigallocatechin gallate, in the 6-OHDA model of Parkinson's disease. Evid Based Complement Alternat Med. (2015) 2015:161092. doi: 10.1155/2015/161092
195. Dekant, W, Fujii, K, Shibata, E, Morita, O, and Shimotoyodome, A. Safety assessment of green tea based beverages and dried green tea extracts as nutritional supplements. Toxicol Lett. (2017) 277:104–8. doi: 10.1016/j.toxlet.2017.06.008
196. Siblini, H, Al-Hendy, A, Segars, J, González, F, Taylor, HS, Singh, B, et al. Assessing the hepatic safety of epigallocatechin gallate (EGCG) in reproductive-aged women. Nutrients. (2023) 15:320. doi: 10.3390/nu15020320
197. Chen, IJ, Liu, C-Y, Chiu, J-P, and Hsu, C-H. Therapeutic effect of high-dose green tea extract on weight reduction: a randomized, double-blind, placebo-controlled clinical trial. Clin Nutr. (2016) 35:592–9. doi: 10.1016/j.clnu.2015.05.003
198. Mehmood, S, Maqsood, M, Mahtab, N, Khan, MI, Sahar, A, Zaib, S, et al. Epigallocatechin gallate: phytochemistry, bioavailability, utilization challenges, and strategies. J Food Biochem. (2022) 46:e14189. doi: 10.1111/jfbc.14189
199. Gan, RY, Li, HB, Sui, ZQ, and Corke, H. Absorption, metabolism, anti-cancer effect and molecular targets of epigallocatechin gallate (EGCG): An updated review. Crit Rev Food Sci Nutr. (2018) 58:924–41. doi: 10.1080/10408398.2016.1231168
200. Bakun, P, Mlynarczyk, DT, Koczorowski, T, Cerbin-Koczorowska, M, Piwowarczyk, L, Kolasiński, E, et al. Tea-break with epigallocatechin gallate derivatives – powerful polyphenols of great potential for medicine. Eur J Med Chem. (2023) 261:115820. doi: 10.1016/j.ejmech.2023.115820
201. Ouyang, J, Zhu, K, Liu, Z, and Huang, J. Prooxidant effects of Epigallocatechin-3-gallate in health benefits and potential adverse effect. Oxidative Med Cell Longev. (2020) 2020:9723686. doi: 10.1155/2020/9723686
202. Na, HK, and Surh, YJ. Modulation of Nrf2-mediated antioxidant and detoxifying enzyme induction by the green tea polyphenol EGCG. Food Chem Toxicol. (2008) 46:1271–8. doi: 10.1016/j.fct.2007.10.006
203. Lambert, JD, and Yang, CS. Mechanisms of cancer prevention by tea constituents. J Nutr. (2003) 133:3262s–7s. doi: 10.1093/jn/133.10.3262S
204. Miyazawa, T. Absorption, metabolism and antioxidative effects of tea catechin in humans. Biofactors. (2000) 13:55–9. doi: 10.1002/biof.5520130110
205. Shimizu, K, Asakawa, T, Harada, N, Fukumoto, D, Tsukada, H, Asai, T, et al. Use of positron emission tomography for real-time imaging of biodistribution of green tea catechin. PLoS One. (2014) 9:e85520. doi: 10.1371/journal.pone.0085520
206. Lambert, JD, Lee, MJ, Lu, H, Meng, X, Hong, JJ, Seril, DN, et al. Epigallocatechin-3-gallate is absorbed but extensively glucuronidated following oral administration to mice. J Nutr. (2003) 133:4172–7. doi: 10.1093/jn/133.12.4172
207. Zeng, W, Lao, S, Guo, Y, Wu, Y, Huang, M, Tomlinson, B, et al. The influence of EGCG on the pharmacokinetics and pharmacodynamics of bisoprolol and a new method for simultaneous determination of EGCG and bisoprolol in rat plasma. Front Nutr. (2022) 9:907986. doi: 10.3389/fnut.2022.907986
208. Chen, L, Lee, MJ, Li, H, and Yang, CS. Absorption, distribution, elimination of tea polyphenols in rats. Drug Metab Dispos. (1997) 25:1045–50.
209. Luo, YP, Tang, XF, Zhang, YC, Chen, SM, Wu, Q, and Li, WJ. Epigallocatechin-3-gallate alleviates galactose-induced aging impairment via gut-brain communication. Food Funct. (2022) 13:11200–9. doi: 10.1039/D2FO00994C
210. Wang, X, Ding, C, and Li, HB. The crosstalk between enteric nervous system and immune system in intestinal development, homeostasis and diseases. Sci China Life Sci. (2024) 67:41–50. doi: 10.1007/s11427-023-2376-0
211. Xiao, L, Tang, R, Wang, J, Wan, D, Yin, Y, and Xie, L. Gut microbiota bridges the iron homeostasis and host health. Sci China Life Sci. (2023) 66:1952–75. doi: 10.1007/s11427-022-2302-5
212. Chiu, HF, Venkatakrishnan, K, and Wang, CK. The role of nutraceuticals as a complementary therapy against various neurodegenerative diseases: a mini-review. J Tradit Complement Med. (2020) 10:434–9. doi: 10.1016/j.jtcme.2020.03.008
213. Jiang, C. Progress in gut microbiota-host interaction. Sci China Life Sci. (2024) 67:851–3. doi: 10.1007/s11427-024-2577-0
214. Pervin, M, Unno, K, Takagaki, A, Isemura, M, and Nakamura, Y. Function of Green tea catechins in the brain: epigallocatechin gallate and its metabolites. Int J Mol Sci. (2019) 20:3630. doi: 10.3390/ijms20153630
215. Ng, HLH, Premilovac, D, Rattigan, S, Richards, SM, Muniyappa, R, Quon, MJ, et al. Acute vascular and metabolic actions of the green tea polyphenol epigallocatechin 3-gallate in rat skeletal muscle. J Nutr Biochem. (2017) 40:23–31. doi: 10.1016/j.jnutbio.2016.10.005
216. Li, G, Yang, J, Wang, X, Zhou, C, Zheng, X, and Lin, W. Effects of EGCG on depression-related behavior and serotonin concentration in a rat model of chronic unpredictable mild stress. Food Funct. (2020) 11:8780–7. doi: 10.1039/D0FO00524J
217. El-Missiry, MA, Othman, AI, El-Sawy, MR, and Lebede, MF. Neuroprotective effect of epigallocatechin-3-gallate (EGCG) on radiation-induced damage and apoptosis in the rat hippocampus. Int J Radiat Biol. (2018) 94:798–808. doi: 10.1080/09553002.2018.1492755
218. He, Y, Yang, Z, Pi, J, Cai, T, Xia, Y, Cao, X, et al. EGCG attenuates the neurotoxicity of methylglyoxal via regulating MAPK and the downstream signaling pathways and inhibiting advanced glycation end products formation. Food Chem. (2022) 384:132358. doi: 10.1016/j.foodchem.2022.132358
219. Bergstrom, HC, Darvesh, AS, and Berger, SP. Inducible nitric oxide inhibitors Block NMDA antagonist-stimulated motoric Behaviors and medial prefrontal cortical glutamate efflux. Front Pharmacol. (2015) 6:292. doi: 10.3389/fphar.2015.00292
220. Wang, JH, Cheng, J, Li, CR, Ye, M, Ma, Z, and Cai, F. Modulation of Ca2+ signals by epigallocatechin-3-gallate(EGCG) in cultured rat hippocampal neurons. Int J Mol Sci. (2011) 12:742–54. doi: 10.3390/ijms12010742
221. Zuo, G, Chen, M, Zuo, Y, Liu, F, Yang, Y, Li, J, et al. Tea polyphenol epigallocatechin gallate protects against nonalcoholic fatty liver disease and associated endotoxemia in rats via modulating gut microbiota dysbiosis and alleviating intestinal barrier dysfunction and related inflammation. J Agric Food Chem. (2024) 72:9067–86. doi: 10.1021/acs.jafc.3c04832
222. Naito, Y, Ushiroda, C, Mizushima, K, Inoue, R, Yasukawa, Z, Abe, A, et al. Epigallocatechin-3-gallate (EGCG) attenuates non-alcoholic fatty liver disease via modulating the interaction between gut microbiota and bile acids. J Clin Biochem Nutr. (2020) 67:2–9. doi: 10.3164/jcbn.20-39
223. Poewe, W, Stankovic, I, Halliday, G, Meissner, WG, Wenning, GK, Pellecchia, MT, et al. Multiple system atrophy. Nat Rev Dis Primers. (2022) 8:56. doi: 10.1038/s41572-022-00382-6
224. Stefanova, N, and Wenning, GK. Multiple system atrophy: at the crossroads of cellular, molecular and genetic mechanisms. Nat Rev Neurosci. (2023) 24:334–46. doi: 10.1038/s41583-023-00697-7
225. Levin, J, Maaß, S, Schuberth, M, Giese, A, Oertel, WH, Poewe, W, et al. Safety and efficacy of epigallocatechin gallate in multiple system atrophy (PROMESA): a randomised, double-blind, placebo-controlled trial. Lancet Neurol. (2019) 18:724–35. doi: 10.1016/S1474-4422(19)30141-3
226. Molinari, M, Watt, KD, Kruszyna, T, Nelson, R, Walsh, M, Huang, WY, et al. Acute liver failure induced by green tea extracts: case report and review of the literature. Liver Transpl. (2006) 12:1892–5. doi: 10.1002/lt.21021
227. Nakagawa, K, and Miyazawa, T. Absorption and distribution of tea catechin, (−)-epigallocatechin-3-gallate, in the rat. J Nutr Sci Vitaminol (Tokyo). (1997) 43:679–84. doi: 10.3177/jnsv.43.679
228. Lambert, JD, Kennett, MJ, Sang, S, Reuhl, KR, Ju, J, and Yang, CS. Hepatotoxicity of high oral dose (−)-epigallocatechin-3-gallate in mice. Food Chem Toxicol. (2010) 48:409–16. doi: 10.1016/j.fct.2009.10.030
229. Wang, D, Wei, Y, Wang, T, Wan, X, Yang, CS, Reiter, RJ, et al. Melatonin attenuates (−)-epigallocatehin-3-gallate-triggered hepatotoxicity without compromising its downregulation of hepatic gluconeogenic and lipogenic genes in mice. J Pineal Res. (2015) 59:497–507. doi: 10.1111/jpi.12281
230. Ramachandran, B, Jayavelu, S, Murhekar, K, and Rajkumar, T. Repeated dose studies with pure Epigallocatechin-3-gallate demonstrated dose and route dependant hepatotoxicity with associated dyslipidemia. Toxicol Rep. (2016) 3:336–45. doi: 10.1016/j.toxrep.2016.03.001
231. Wang, D, Wang, Y, Wan, X, Yang, CS, and Zhang, J. Green tea polyphenol (−)-epigallocatechin-3-gallate triggered hepatotoxicity in mice: responses of major antioxidant enzymes and the Nrf2 rescue pathway. Toxicol Appl Pharmacol. (2015) 283:65–74. doi: 10.1016/j.taap.2014.12.018
232. Saleh, IG, Ali, Z, Abe, N, Wilson, FD, Hamada, FM, Abd-Ellah, MF, et al. Effect of green tea and its polyphenols on mouse liver. Fitoterapia. (2013) 90:151–9. doi: 10.1016/j.fitote.2013.07.014
233. Sang, S, Lambert, JD, Hong, J, Tian, S, Lee, MJ, Stark, RE, et al. Synthesis and structure identification of thiol conjugates of (−)-epigallocatechin gallate and their urinary levels in mice. Chem Res Toxicol. (2005) 18:1762–9. doi: 10.1021/tx050151l
234. Isbrucker, RA, Edwards, JA, Wolz, E, Davidovich, A, and Bausch, J. Safety studies on epigallocatechin gallate (EGCG) preparations. Part 2: dermal, acute and short-term toxicity studies. Food Chem Toxicol. (2006) 44:636–50. doi: 10.1016/j.fct.2005.11.003
235. Galati, G, Lin, A, Sultan, AM, and O'Brien, PJ. Cellular and in vivo hepatotoxicity caused by green tea phenolic acids and catechins. Free Radic Biol Med. (2006) 40:570–80. doi: 10.1016/j.freeradbiomed.2005.09.014
236. Kweon, MH, Adhami, VM, Lee, JS, and Mukhtar, H. Constitutive overexpression of Nrf2-dependent heme oxygenase-1 in A549 cells contributes to resistance to apoptosis induced by epigallocatechin 3-gallate. J Biol Chem. (2006) 281:33761–72. doi: 10.1074/jbc.M604748200
237. Rasheed, NO, Ahmed, LA, Abdallah, DM, and El-Sayeh, BM. Nephro-toxic effects of intraperitoneally injected EGCG in diabetic mice: involvement of oxidative stress, inflammation and apoptosis. Sci Rep. (2017) 7:40617. doi: 10.1038/srep40617
238. Brückner, M, Westphal, S, Domschke, W, Kucharzik, T, and Lügering, A. Green tea polyphenol epigallocatechin-3-gallate shows therapeutic antioxidative effects in a murine model of colitis. J Crohns Colitis. (2012) 6:226–35. doi: 10.1016/j.crohns.2011.08.012
239. Roghani, M, and Baluchnejadmojarad, T. Hypoglycemic and hypolipidemic effect and antioxidant activity of chronic epigallocatechin-gallate in streptozotocin-diabetic rats. Pathophysiology. (2010) 17:55–9. doi: 10.1016/j.pathophys.2009.07.004
240. Chengelis, CP, Kirkpatrick, JB, Regan, KS, Radovsky, AE, Beck, MJ, Morita, O, et al. 28-day oral (gavage) toxicity studies of green tea catechins prepared for beverages in rats. Food Chem Toxicol. (2008) 46:978–89. doi: 10.1016/j.fct.2007.10.027
241. Hsu, YW, Tsai, CF, Chen, WK, Huang, CF, and Yen, CC. A subacute toxicity evaluation of green tea (Camellia sinensis) extract in mice. Food Chem Toxicol. (2011) 49:2624–30. doi: 10.1016/j.fct.2011.07.007
242. Wang, D, Xiao, R, Hu, X, Xu, K, Hou, Y, Zhong, Y, et al. Comparative safety evaluation of Chinese Pu-erh green tea extract and Pu-erh black tea extract in Wistar rats. J Agric Food Chem. (2010) 58:1350–8. doi: 10.1021/jf902171h
243. Kapetanovic, IM, Crowell, JA, Krishnaraj, R, Zakharov, A, Lindeblad, M, and Lyubimov, A. Exposure and toxicity of green tea polyphenols in fasted and non-fasted dogs. Toxicology. (2009) 260:28–36. doi: 10.1016/j.tox.2009.03.007
244. Kim, W, Jeong, MH, Cho, SH, Yun, JH, Chae, HJ, Ahn, YK, et al. Effect of green tea consumption on endothelial function and circulating endothelial progenitor cells in chronic smokers. Circ J. (2006) 70:1052–7. doi: 10.1253/circj.70.1052
245. Toolsee, NA, Aruoma, OI, Gunness, TK, Kowlessur, S, Dambala, V, Murad, F, et al. Effectiveness of green tea in a randomized human cohort: relevance to diabetes and its complications. Biomed Res Int. (2013) 2013:412379. doi: 10.1155/2013/412379
246. Henning, SM, Wang, P, Said, JW, Huang, M, Grogan, T, Elashoff, D, et al. Randomized clinical trial of brewed green and black tea in men with prostate cancer prior to prostatectomy. Prostate. (2015) 75:550–9. doi: 10.1002/pros.22943
247. Basu, A, Sanchez, K, Leyva, MJ, Wu, M, Betts, NM, Aston, CE, et al. Green tea supplementation affects body weight, lipids, and lipid peroxidation in obese subjects with metabolic syndrome. J Am Coll Nutr. (2010) 29:31–40. doi: 10.1080/07315724.2010.10719814
248. Maki, KC, Reeves, MS, Farmer, M, Yasunaga, K, Matsuo, N, Katsuragi, Y, et al. Green tea catechin consumption enhances exercise-induced abdominal fat loss in overweight and obese adults. J Nutr. (2009) 139:264–70. doi: 10.3945/jn.108.098293
249. Nagao, T, Komine, Y, Soga, S, Meguro, S, Hase, T, Tanaka, Y, et al. Ingestion of a tea rich in catechins leads to a reduction in body fat and malondialdehyde-modified LDL in men. Am J Clin Nutr. (2005) 81:122–9. doi: 10.1093/ajcn/81.1.122
250. Nguyen, MM, Ahmann, FR, Nagle, RB, Hsu, CH, Tangrea, JA, Parnes, HL, et al. Randomized, double-blind, placebo-controlled trial of polyphenon E in prostate cancer patients before prostatectomy: evaluation of potential chemopreventive activities. Cancer Prev Res (Phila). (2012) 5:290–8. doi: 10.1158/1940-6207.CAPR-11-0306
251. McLarty, J, Bigelow, RL, Smith, M, Elmajian, D, Ankem, M, and Cardelli, JA. Tea polyphenols decrease serum levels of prostate-specific antigen, hepatocyte growth factor, and vascular endothelial growth factor in prostate cancer patients and inhibit production of hepatocyte growth factor and vascular endothelial growth factor in vitro. Cancer Prev Res (Phila). (2009) 2:673–82. doi: 10.1158/1940-6207.CAPR-08-0167
252. Garcia, FA, Cornelison, T, Nuño, T, Greenspan, DL, Byron, JW, Hsu, CH, et al. Results of a phase II randomized, double-blind, placebo-controlled trial of Polyphenon E in women with persistent high-risk HPV infection and low-grade cervical intraepithelial neoplasia. Gynecol Oncol. (2014) 132:377–82. doi: 10.1016/j.ygyno.2013.12.034
253. Ullmann, U, Haller, J, Decourt, JD, Girault, J, Spitzer, V, and Weber, P. Plasma-kinetic characteristics of purified and isolated green tea catechin epigallocatechin gallate (EGCG) after 10 days repeated dosing in healthy volunteers. Int J Vitam Nutr Res. (2004) 74:269–78. doi: 10.1024/0300-9831.74.4.269
254. Mielgo-Ayuso, J, Barrenechea, L, Alcorta, P, Larrarte, E, Margareto, J, and Labayen, I. Effects of dietary supplementation with epigallocatechin-3-gallate on weight loss, energy homeostasis, cardiometabolic risk factors and liver function in obese women: randomised, double-blind, placebo-controlled clinical trial. Br J Nutr. (2014) 111:1263–71. doi: 10.1017/S0007114513003784
255. De la Torre, R, De Sola, S, Pons, M, Duchon, A, de Lagran, MM, Farré, M, et al. Epigallocatechin-3-gallate, a DYRK1A inhibitor, rescues cognitive deficits in down syndrome mouse models and in humans. Mol Nutr Food Res. (2014) 58:278–88. doi: 10.1002/mnfr.201300325
256. Laurie, SA, Miller, VA, Grant, SC, Kris, MG, and Ng, KK. Phase I study of green tea extract in patients with advanced lung cancer. Cancer Chemother Pharmacol. (2005) 55:33–8. doi: 10.1007/s00280-004-0859-1
257. Hu, J, Webster, D, Cao, J, and Shao, A. The safety of green tea and green tea extract consumption in adults – results of a systematic review. Regul Toxicol Pharmacol. (2018) 95:412–33. doi: 10.1016/j.yrtph.2018.03.019
Keywords: catechins, (−)-epigallocatechin-3-gallate, neurodegenerative diseases, Alzheimer’s disease, Parkinson’s disease
Citation: Li S, Wang Z, Liu G and Chen M (2024) Neurodegenerative diseases and catechins: (−)-epigallocatechin-3-gallate is a modulator of chronic neuroinflammation and oxidative stress. Front. Nutr. 11:1425839. doi: 10.3389/fnut.2024.1425839
Received: 23 May 2024; Accepted: 11 July 2024;
Published: 01 August 2024.
Edited by:
Lei Zhang, University of Waterloo, CanadaReviewed by:
Vanessa Porrini, University of Brescia, ItalyCopyright © 2024 Li, Wang, Liu and Chen. This is an open-access article distributed under the terms of the Creative Commons Attribution License (CC BY). The use, distribution or reproduction in other forums is permitted, provided the original author(s) and the copyright owner(s) are credited and that the original publication in this journal is cited, in accordance with accepted academic practice. No use, distribution or reproduction is permitted which does not comply with these terms.
*Correspondence: Gang Liu, Z2FuZ2xlLmxpdUBnbWFpbC5jb20=
Disclaimer: All claims expressed in this article are solely those of the authors and do not necessarily represent those of their affiliated organizations, or those of the publisher, the editors and the reviewers. Any product that may be evaluated in this article or claim that may be made by its manufacturer is not guaranteed or endorsed by the publisher.
Research integrity at Frontiers
Learn more about the work of our research integrity team to safeguard the quality of each article we publish.