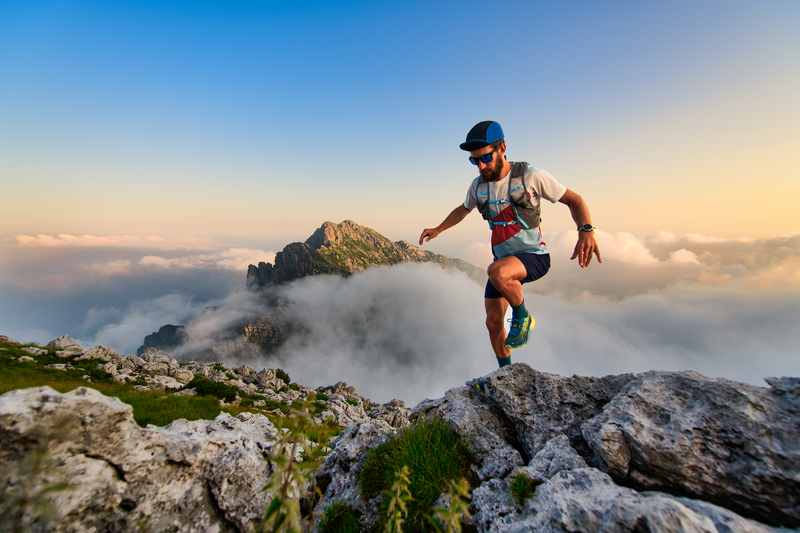
95% of researchers rate our articles as excellent or good
Learn more about the work of our research integrity team to safeguard the quality of each article we publish.
Find out more
ORIGINAL RESEARCH article
Front. Nutr. , 08 December 2020
Sec. Sport and Exercise Nutrition
Volume 7 - 2020 | https://doi.org/10.3389/fnut.2020.607299
This article is part of the Research Topic Ergogenic Aids: Physiological and Performance Responses View all 7 articles
Purpose: The purpose of this study was to evaluate the effects of a ketone monoester supplement on indices of muscle damage during recovery after eccentric exercise.
Methods: In a randomized, double-blind, independent group design, 20 moderately active healthy young adults consumed 360 mg per kg−1 bodyweight of a ketone monoester (KET) or energy-matched carbohydrate (CON) supplement twice daily following eccentric exercise (drop jumps). Maximal isometric voluntary contraction (MIVC) torque, counter-movement jump (CMJ) height, and muscle soreness were measured before (PRE), and immediately (POST), 24 h and 48 h post-exercise. Blood samples were collected for analysis of β-hydroxybutyrate (β-OHB), creatine kinase (CK), and select pro- and anti-inflammatory cytokines.
Results: Peak blood β-OHB concentration after supplement intake was greater (P < 0.001) in KET (4.4 ± 0.8 mM) vs. CON (0.4 ± 0.3 mM). Exercise increased CK concentration at 24 h and 48 h vs. PRE (time: P < 0.001) with no difference between KET and CON. Exercise reduced MIVC (KET: −19.9 ± 14.6; CON: −22.6 ± 11.1%) and CMJ (KET: −11.0 ± 7.5; CON: −13.0 ± 8.7%) at POST relative PRE; however, there was no difference between KET and CON on the recovery of MIVC at 24 h (KET: −15.4 ± 20.4; CON: −18.7 ± 20.1%) or 48 h (KET: −7.2 ± 21.2; CON: −11.8 ± 20.2%), or CMJ at 24 h (KET: −9.2 ± 11.5; CON: −13.4 ± 10.8) or 48 h (KET: −12.5 ± 12.4; CON: −9.1 ± 11.7). Muscle soreness was increased during post-exercise recovery (time: P < 0.001) with no differences between KET and CON. Monocyte chemoattractant protein-1 was greater (group: P = 0.007) in CON (236 ± 11 pg/mL) vs. KET (187 ± 11 pg/mL).
Conclusion: In conclusion, twice daily ingestion of a ketone monoester supplement that acutely elevates blood β-OHB concentration does not enhance the recovery of muscle performance or reduce muscle soreness following eccentric exercise in moderately active, healthy young adults.
Exercise-induced muscle damage (EIMD) is a non-pathological condition that occurs in response to unaccustomed exercise involving repeated eccentric (i.e., lengthening) muscle contractions. The main consequences of EIMD are a temporary reduction in muscle function (i.e., force production) and increased muscle soreness accompanied by an increase in intramuscular proteins [e.g., creatine kinase (CK)] in the blood and swelling of the involved muscle group (1). Consequently, EIMD may negatively impact the ability to perform during subsequent training/exercise sessions. The mechanisms of EIMD are complex but are thought to involve both a primary and secondary phase of damage (2, 3). The primary phase of EIMD involves ultrastructural damage to the muscle and/or impairments in excitation-contraction coupling due to the mechanical work performed during exercise (2, 3). The secondary phase of EIMD occurs in response to the primary phase and involves biochemical changes, the hallmarks of which are inflammation and oxidative stress, that may further exacerbate damage to the muscle and surrounding tissue (4). Given the negative symptoms associated with EIMD, there is significant interest among athletes, coaches, and the broader fitness community on novel therapeutic approaches that may effectively reduce indices of EIMD and accelerate recovery after eccentric exercise.
A variety of nutritional interventions have been evaluated in an attempt to alleviate muscle soreness and impairments in muscle function during recovery from eccentric exercise [for reviews see (2, 5–7)]. From an EIMD perspective, nutritional interventions that increase muscle protein synthesis and/or reduce protein breakdown may support recovery from damage by increasing the rate of tissue repair/remodeling or reducing the extent of damage (8). In addition, interventions that can reduce EIMD-induced increases in inflammation and/or oxidative stress may minimize further exacerbation of secondary muscle damage, and thereby contribute to improved short-term recovery (2, 9). Ketone bodies [i.e., D-β-hydroxybutyrate (β-OHB), acetoacetate, and acetone] are lipid-derived molecules that are normally produced in the body in response to starvation or carbohydrate restriction (i.e., a ketogenic diet). Under these conditions they serve as an important fuel source for metabolically active tissues including the brain and skeletal muscle. Recently, exogenous ketone “supplements” have been developed, including the ketone monoester (R)-3-hydroxybutyl (R)-3-hydroxybutyrate (10), that rapidly increases β-OHB to ~3–5 mM within ~30 min in healthy humans (11) without the need for dietary restriction. While mostly studied in terms of their acute effects on endurance exercise performance (12, 13), exogenous ketone supplements and increases in ketone body availability have been proposed to play a role in modulating recovery after exercise (14, 15). Ketone bodies are increasingly understood to act as important signaling molecules that can regulate protein metabolism, inflammation, and oxidative stress (16); putative factors involved in recovery following EIMD. For example, β-OHB has been shown to stimulate skeletal muscle protein synthesis in humans (17), and reduce muscle (18), and whole-body protein breakdown (18, 19). Anticatabolic effects of β-OHB have also recently been demonstrated in human skeletal muscle under inflammatory insult (18). Ketone bodies have been reported to mitigate inflammation and oxidative stress both in-vitro and in-vivo (20) and may stimulate muscle regeneration or remodeling by enhancing satellite cell activation and differentiation (21, 22). Collectively, these actions indicate a potential role for exogenous ketone supplementation and elevated β-OHB as a novel therapeutic approach to expedite the recovery of indices of EIMD after eccentric exercise.
The purpose of this study was to evaluate the effects of supplementation with a ketone monoester, that acutely increases blood β-OHB concentration, on indices of EIMD including muscle dysfunction and muscle soreness during recovery from a bout of eccentric exercise in healthy young moderately-active adults. We hypothesized that twice daily supplementation with a ketone monoester (KET) would reduce the concentration of select pro-inflammatory cytokines, expedite the recovery of muscle function, and reduce muscle soreness when compared to supplementation with an energy-matched carbohydrate supplement (CON).
Twenty healthy young men and women (n = 10 men and 10 women) 18–35 years of age, with a BMI >18.5 and <30.0 kg/m2 participated in this randomized, double-blind, parallel group study. Participants were moderately active (defined as regularly exercising 2–4 times per week) but unaccustomed to high-force plyometric exercise. Participants were excluded if they met any of the following criteria: identified metabolic or intestinal disorder, use of tobacco products, adherence to a ketogenic, vegetarian, or vegan diet, use of certain medications (i.e., corticosteroids, non-steroidal anti-inflammatories, or prescription strength acne medications), musculoskeletal ailments that prevented them from performing the required exercise, pregnancy, asthma, and use of certain dietary supplements (i.e., creatine, fish oil, beta-alanine). Female participants were studied during the early follicular phase of their menstrual cycle (within 5 days of the onset of menses). Participants' characteristics are presented in Table 1. All participants were informed about the purpose of the study, the experimental procedures, and possible risks prior to providing informed written consent to participate.
The study was approved by the Faculty of Medicine Institutional Review Board at McGill University on January 14, 2019 (IRB Study Number: A01-M01-19A). All participants provided written informed consent prior to study participation. The study was conducted in accordance with the ethical standards of the Faculty of Medicine Institutional Review Board at McGill University on human experimentation and in accordance with the Helsinki Declaration of 1975 as revised in October 2013.
Participants underwent an initial screening and familiarization visit during which height (via a wall-mounted stadiometer), weight (via a digital balance), heart rate and blood pressure (Omron 10 series, Model BP786CANN), and body composition (by dual-energy X-ray absorptiometry; GE Healthcare, Madison, WI, USA) were assessed. Participants were also thoroughly familiarized with the exercise testing equipment and procedures. Participants received a demonstration by one of the study investigators and then performed a guided familiarization trial for the performance measures. Participants were deemed healthy based on their responses to a medical questionnaire and screening results. The initial screening and familiarization visit, and onset of the experiment were separated by at least 4 days.
Study participants were asked to refrain from strenuous physical activity and alcohol consumption for 2 days immediately prior to the onset of the experiment and during all experimental test days. In addition, participants were required to complete food intake and physical activity questionnaires during this time. Average dietary intake prior to (pre-study phase) and during (EIMD recovery phase) the experiment was analyzed using commercially available software (Food Processor version 11.7; ESHA Research; Salem OR, USA). On the evening before each experimental test day, participants were instructed to stop consuming food or beverages other than water or their nutritional treatment (described below) by 20:00 h, after which they remained fasted until testing the following morning.
The present study utilized a randomized, double-blind, independent group design. One group (KET) received a ketone monoester, while the other group (CON) received an energy-matched amount of carbohydrate. Participants ingested their assigned treatment twice daily for 2 days during recovery following an acute bout of eccentric exercise (described below). During the study, participants were required to report to the laboratory on 3 sequential days for testing (not including the visit for preliminary testing and familiarization). Testing occurred at baseline (PRE), immediately post-exercise (POST), 24-h post-exercise (24 h), and 48-h post-exercise (48 h) and consisted of the following: tests of muscle function [maximal isometric voluntary contraction (MIVC) of the knee-extensors and counter movement jump height (CMJ)], muscle soreness [VAS questionnaire and pressure pain threshold (PPT)], markers of exercise induced muscle damage [serum creatine kinase (CK)], limb girth of the upper leg and calf (as a proxy for swelling/edema), flexibility of the quadriceps (via goniometer during a Modified Thomas Test), and plasma concentrations of select cytokines and chemokines. Testing also consisted of the Brief Assessment of Mood Adapted (BAM+) questionnaire to assess the participants “readiness” for performance (23). The randomization procedure to allocate treatment group was determined via a random-number generator (http://www.randomization.com/). An equal number of men and women were randomized to the KET and CON groups, respectively. An independent person was responsible for the randomization and preparation of the study beverages. The beverages were prepared in non-transparent plastic containers. To limit diurnal and intrasubject variation, all measures were carried out according to a standardized time schedule at the same time of day.
On Day #1 of the study, participants reported to the laboratory at ~08:00 in the overnight post-absorptive state. Participants underwent a venous blood draw from the antecubital vein via venipuncture followed by baseline (PRE) testing in the following order: BAM+ questionnaire, VAS of muscle soreness, limb circumference, PPT, and Modified Thomas Test (measures described below). Subsequently, participants performed a 5 min brisk walking warm-up on a treadmill at a speed of 5.0 km/h with an incline of 1.0, followed by assessment of knee extensor MIVC torque (Biodex 4 Pro, Biodex Medical Systems, Shirley, NY, USA) and CMJ height (Dual force plates model 9260AA6, Kistler group, Winterthur, Switzerland). Participants then performed an eccentric exercise protocol consisting of 100 drop jumps off a 60 cm box (5 sets of 20 repetitions with a 2 min inter-set rest interval). Following 15 min of recovery from the eccentric exercise protocol, participants completed post-exercise testing (POST) of the following: BAM+ questionnaire, VAS of muscle soreness, limb circumference, PPT, Modified Thomas Test, knee extensor MIVC, and CMJ. Participants then received and ingested their randomly assigned treatment (KET or CON). Capillary blood samples were obtained via finger prick to assess capillary blood β-OHB concentration (FreeStyle Precision Neo, Abbott Laboratories) immediately following POST testing prior to treatment intake, and at 30, 60, 120, and 180 min following treatment intake while participants rested in a recumbent position on a bed in the laboratory. An independent person obtained the capillary samples to allow the study investigators to remain blinded to the treatment intervention. Participants then received a second treatment drink that they were instructed to consume on their own in the evening ~30 min before going to sleep. The following morning (Day #2) participants reported to the laboratory in the overnight post-absorptive state and completed the 24 h post-exercise (24 h) testing in a manner identical to PRE (baseline) testing. Participants then received and ingested the third serving of their randomly assigned treatment and returned the containers from the treatment consumed the evening prior. Participants then received their fourth and final treatment drink that they were instructed to consume on their own in the evening ~30 min before going to sleep. The following morning, participants again reported to the laboratory (Day #3) in the overnight post-absorptive state, completed the 48 h post-exercise (48 h) testing protocol (identical to the 24 h testing outlined above), and returned the containers from the treatment consumed the evening prior. For an overview of the experimental protocol see Figure 1.
Figure 1. Overview of the experimental protocol. Twenty young adults (10 men; 10 women) performed an acute bout of eccentric exercise and were studied over 48 h of post-exercise recovery. During the recovery period, participants received a ketone monoester (KET) or energy-matched carbohydrate (CON) supplement two times per day (in the morning immediately after testing and in the evening 30 min prior to sleep). Testing occurred at baseline (PRE) before the bout of eccentric exercise, and then immediately (POST), 24 h (24 h), and 48 h (48 h) after exercise and included the following: venous blood sample (except at POST), BAM+, VAS muscle soreness, limb (thigh and calf) circumference, PPT, flexibility, MIVC, and CMJ. Capillary blood samples were taken before (0 min) and after (30, 60, 120, 180 min) intake of the first supplement serving. BAM+, brief assessment of mood adapted; CMJ, counter-movement jump; CON, carbohydrate control group; KET, ketone monoester group; MIVC, maximal isometric voluntary contraction; PPT, pressure-pain threshold; VAS, visual analog scale. Figure created with BioRender.com.
Venous blood samples were drawn into a 4-mL vacutainer for serum and two 3-mL vacutainers coated with Ethylenediaminetetraacetic acid (EDTA) for plasma during PRE, 24 h and 48 h time-points. Samples were centrifuged at 3,000 g for 15 min at 4°C. Plasma and serum were then aliquoted and stored at −80°C until further analysis. Analysis of total CK concentration was carried out on serum samples using a chemistry analyzer (Beckman Coulter Olympus AU5800) at the Clinical Biochemistry Laboratory of the McGill University Health Centre. According to data provided by the laboratory, the coefficient of variation (CV) for total CK is 2.5%. Analysis of circulating granulocyte colony stimulating factor (G-CSF), interleukin-1 beta (IL-1β), interleukin-1 receptor antagonist (IL-1ra), interleukin-6 (IL-6), interleukin-8 (IL-8), interleukin-10 (IL-10), interleukin-12p70 [IL-12(p70)], interleukin-15 (IL-15), monocyte chemoattractant protein 1 (MCP-1), and tumor necrosis factor alpha (TNF-α) was carried out on plasma samples using Luminex xMAP technology for multiplexed quantification of human cytokines, chemokines, and growth factors. The multiplexing analysis was performed in duplicate using the Luminex™ 200 system (Luminex, Austin, TX, USA) by Eve Technologies Corp (Calgary, Alberta). In the first multi-plex experiment, six markers were simultaneously measured in the samples using Eve Technologies' Custom Human High Sensitivity 6-Plex Discovery Assay® (MilliporeSigma, Burlington, Massachusetts, USA) according to the manufacturer's protocol. The 6-plex consisted of IL-6, IL-8, IL-10, IL-12p70, Il-1β, and TNF-α. Assay sensitivities of these markers are 0.11, 0.13, 0.56, 0.15, 0.14, and 0.16 pg/mL, respectively. In the second multiplex experiment, 4 markers were simultaneously measured in the samples using Eve Technologies' Custom Human Cytokine Panel 1 4-Plex (MilliporeSigma, Burlington, Massachusetts, USA) according to the manufacturer's protocol. The 4-plex consisted of IL-1ra, IL-15, MCP-1, and G-CSF. Assay sensitivities of these markers are 8.3, 1.2, 1.9, and 1.8 pg/mL, respectively. At least one of the two duplicate samples were below the limit of analytical sensitivity in 35% of samples for G-CSF, 42% of samples for IL-1ra, and 47% of samples for IL-15. Therefore, these analytes were removed from analysis. The CV's for the analyzed data were as follows: IL-1β: 9.3%; IL-6: 7.0%; IL-8: 9.5%; IL-10: 8.8%; IL-12p70: 8.4%; MCP-1: 5.7%, and TNF-α 7.8%.
The Brief Assessment of Mood Adapted (BAM+) questionnaire was used to qualitatively assess participants' mood, recovery status and overall performance readiness (23). The questionnaire contains six items from The Brief Assessment of Mood (BAM) and four questions relating to confidence, motivation, muscle soreness and sleep quality. The BAM+ consists of ten questions and participants are asked to mark a line on a 100 mm VAS, anchored with “not at all” and “extremely” at each end. The lines for each question were measured with a ruler, and an overall recovery score was calculated as described by Shearer et al. (23).
Muscle soreness was assessed via VAS questionnaire and determination of PPT. For assessment of muscle soreness using the VAS questionnaire, participants were asked to perform a squat (at ~90° knee flexion) and rate their level of perceived muscle soreness in their lower limbs by marking a 100 mm horizontal line scale. 0 on the VAS represented “no soreness” and 100 mm represented “unbearably painful.” The line placement was measured with a ruler and recorded. The inter-day CV for this measure in our laboratory is 8.3%.
PPT was assessed using a handheld digital algometer (Somedic SenseLAB AB, Sodala, Sweden) at three pre-marked sites: the vastus lateralis (VL), rectus femoris (RF), and gastrocnemius. The VL was assessed mid-way between the superior aspect of the greater trochanter and head of the tibia. The RF was assessed mid-way between the anterior patella and inguinal fold. The gastrocnemius was assessed on the medial aspect of the calf at relaxed maximum girth. The sites were marked with semi-permanent ink to ensure consistent measurements between days. PPT was assessed while participants lay supine on a table by a study investigator applying pressure at the sites with the algometer until the participant indicated they felt the sensation of pressure transition to the feeling of pain, at which point the pressure value in kPa was recorded. Each site was assessed twice unless recordings differed by 100 or more kPa, in which case the site was assessed a third time and the average was recorded. The reported CV for this measure using these three sites is 9.5% (24).
Circumference at the mid-thigh and calf was assessed as a proxy of limb swelling using an anthropometric tape measure. Both sites were measured with the participant in a standing position. The mid-thigh measure was determined as the mid-point between the inguinal crease and superior aspect of the patella. The calf measurement was made at the widest part of the calf. Both sites were marked with semi-permanent ink to ensure consistent measurements between days (25, 26). The inter-day CV for this measure in our laboratory is 1.0%.
The Modified Thomas test was used to assess flexibility about the thigh region (including hip and knee joint) using a goniometer. The test was performed by having the participant lay supine on the edge of a treatment table, hold his or her non-testing knee to the chest, while letting the opposite (tested) thigh and leg hang freely off the table. The goniometer was lined up to the greater trochanter, the lateral epicondyle of the femur and the lateral malleolus. Hip extension angle (X° – 90°) and knee flexion angle (180° – X°) were then determined. The inter-day CV for this measure in our laboratory is 1.0%.
MIVC of the knee extensors were assessed using a dynamometer (Biodex 4 Pro, Biodex Medical Systems, Shirley, NY, USA). Participants were seated in an upright position, securely fastened with the knee angle set at 90° and instructed to perform a maximal force knee extension for 3 s. Each participant performed five contractions separated by 1 min of rest. Force was recorded in Torque (Nm). The peak value from five maximal contractions was used for analysis. The inter-day CV for this measure in our laboratory is 5.1%.
A force plate system (Dual force plates model 9260AA6, Kistler group, Winterthur, Switzerland) was used to measure CMJ height (cm). Participants were required to descend into a squat position (to a ~90° knee angle) before jumping vertically with maximum effort. During testing, participants' hands remained on the hips. Each participant performed three maximal effort jumps separated by 30 s of rest. The average value of the three jumps was used for analysis. The inter-day CV for this measure in our laboratory is 1.8%.
β-OHB concentration in capillary blood was determined using a handheld monitor (FreeStyle Precision Neo, Abbott Laboratories, Witney, UK). Fingertip capillary samples were collected using a lancet following cleaning with alcohol and allowing to air dry. The first blood droplet sample was discarded with a cotton swab and the subsequent droplet samples were used for analysis. Capillary blood β-OHB concentration was assessed immediately following POST testing prior to treatment intake, and at 30, 60, 120, and 180 min after treatment intake.
The eccentric bout of exercise consisted of 100-drop jumps from a 60 cm high box with a non-slip rubber top (Northern Lights Fitness Products Inc. Cornwall, ON, Canada). Each repetition of the drop jump was performed by having the participant first step onto the box (alternating between legs during each step-up), then “drop” off the box onto the floor and land on two feet, while immediately descending to a ~90° knee angle and jumping vertically with maximal effort. Participants performed five sets of 20 repetitions with a 2-min inter-set rest period. The technique was first demonstrated by one of the study investigators on two occasions before participants performed the exercise bout. Each participant was given corrective feedback and strong verbal encouragement to ensure maximal efforts. Drop jumps have been utilized in several muscle damage research studies and have been demonstrated to induce significant lower limb muscle soreness and declines in muscle function (25, 27–32).
The KET group received the ketone monoester (R)-3-hydroxybutyl (R)-3-hydroxybutyrate (Pure ΔG® Ketone Ester; HVMN, CA, USA) at a dose of 360 mg per kg−1 body weight per serving. The CON group received an energy-matched amount of carbohydrate as a combination of Glacier Cherry Gatorade (G2—Gatorade Company, Inc., Chicago, IL, USA) and Dextrose powder (NOW foods, Inc., Bloomingdale IL, USA) with 10 drops of liquid Vanilla Stevia (Stevia Select Inc, USA). All treatments were volume-matched and prepared in opaque drinking containers. Participants ingested their assigned treatment twice daily (morning and evening) for 2 days (48 h) during recovery following an acute bout of eccentric exercise (described above). The morning treatments were consumed at the laboratory under direct supervision of one of the study investigators, while participants consumed the evening treatments on their own ~30 min before going to bed. Participants were asked to return the used bottles at the end of the study.
Due to technical issues, analysis of plasma cytokine/chemokine concentration was not performed in two subjects, therefore plasma cytokine/chemokine data represent n = 9 per group. Participants' physical characteristics, baseline (PRE) test results (for MIVC, CMJ, VAS, PPT, limb circumference, flexibility, and BAM+), and peak capillary blood β-OHB concentration were compared with independent sample t-tests. The peak response (i.e., peak increase or decrease), calculated as the peak change from baseline (PRE) during recovery after eccentric exercise was compared with independent sample t-tests. All other dependent variables were tested using a 2-factor (group × time) repeated-measures ANOVA. When a statistically significant main effect for time or group × time interaction was observed, Bonferroni-corrected post-hoc comparisons were performed. Assumptions of the ANOVA models were assessed using Mauchley's test and the D'Agostino–Pearson omnibus normality test at a significance of P < 0.05. If a significant Mauchley's test was determined, the Greenhouse-Geisser correction factor was used to adjust the degrees of freedom accordingly. For data that did not pass normality, values were transformed with the ln of the value. The statistical analysis was performed on transformed data, but non-transformed data are presented in graphic or tabular form for clarity. Sample size was determined by completing a power analysis (power = 0.9, α = 0.05) based on differences in isometric strength (MIVC) data from Bowtell et al. (33) during recovery from eccentric muscle damaging exercise. This determined a sample size of 6 in each group would provide statistical power at 90%, with an alpha level of 0.05. However, to preserve power and account for dropouts, we recruited n = 10 per group. Statistical analysis was performed with use of the Statistical Package for the Social Sciences (SPSS, Version 24. IBM Corp., Armonk, NY, USA) and GraphPad Prism (Version 8. GraphPad Software., San Diego, CA, USA). For all analyses, differences were considered statistically significant at P < 0.05. All data are expressed as means ± SD.
There were no differences between KET and CON groups for the participants' characteristics or baseline (PRE) test scores (Table 1).
Average total energy, fat, and protein intake, as well as protein intake relative to bodyweight were not different between the pre-study phase compared to the EIMD recovery phase (all P > 0.05) and were not different between KET and CON groups (P > 0.05). Average carbohydrate intake was lower during the EIMD recovery phase compared to the pre-study phase (time; P = 0.03) but was not different between KET and CON groups (P > 0.05). Average dietary intake of study participants is shown in Table 2.
Table 2. Average dietary intake of study participants over 2 days prior to the onset of the experiment (pre-study phase) and during the experiment (EIMD recovery phase) who ingested a ketone monoester supplement (KET) or energy-matched carbohydrate supplement (CON).
Capillary blood β-OHB concentration (Figure 2) increased (group × time interaction; P < 0.001) following ingestion of the ketone monoester and was significantly higher in KET compared to CON at 30 (KET: 4.0 ± 1.3; CON: 0.1 ± 0.03 mM), 60 (KET: 4.1 ± 0.8; CON: 0.1 ± 0.0 mM), 120 (KET: 2.7 ± 0.6; CON: 0.3 ± 0.2 mM), and 180 (KET: 1.3 ± 0.5; CON: 0.4 ± 0.3 mM) min after intake (all P < 0.001).
Figure 2. Capillary blood β-OHB concentration (mM) immediately following POST testing prior to treatment intake (t = 0 min) and 30, 60, 120, and 180 min after treatment intake. Values represent means ± SD. *Indicates difference between KET and CON. Data were analyzed with a two-factor repeated measures (within subject factor: time; between-subject factor: group) ANOVA. Bonferroni-corrected post-hoc comparisons were performed following a significant main effect for time or group × time interaction. Time effect: P < 0.001; group effect: P < 0.001; group × time interaction: P < 0.001. β-OHB, beta-hydroxybutyrate; CON, carbohydrate control group; KET, ketone monoester group.
Serum CK concentration (Figure 3) was increased (time; P < 0.001) at 24 h (319 ± 315 IU·L−1; P < 0.001) and 48 h (198 ± 155 IU·L−1; P = 0.037) after eccentric exercise when compared to PRE (136 ± 122 IU·L−1). There were no differences between KET and CON (group; P = 0.918). The peak increase in CK concentration from PRE after eccentric exercise was not different between groups (KET: 162 ± 195; CON: 235 ± 385 IU·L−1; P = 0.595).
Figure 3. Serum creatine kinase concentration (IU·L−1) at baseline (PRE) prior to exercise, and 24 h and 48 h after exercise. Values represent means ± SD. Times without a common letter differ. Data were analyzed with a two-factor repeated measures (within subject factor: time; between-subject factor: group) ANOVA. Bonferroni-corrected post-hoc comparisons were performed following a significant main effect for time or group × time interaction. Time effect: P < 0.001; group effect: P = 0.918; group × time interaction: P = 0.313. CON, carbohydrate control group; KET, ketone monoester group.
MIVC torque (Figure 4A) was decreased (time; P < 0.001) at POST (−21.2 ± 12.7%; P < 0.001) and 24 h (−17.1 ± 19.8%; P = 0.003) compared to PRE following eccentric exercise. There were no differences in MIVC between KET and CON (group; P = 0.612). The peak decline in absolute MIVC torque from PRE during recovery after eccentric exercise was not different between groups (KET: −43.7 ± 26.0; CON: −54.6 ± 28.3 Nm; P = 0.497). Counter movement jump height (Figure 4B) was decreased (time; P < 0.001) at POST (−12.0 ± 8.0%; P < 0.001), 24 h (−11.3 ± 11.1%; P = 0.003), and 48 h (−10.8 ± 11.9%; P = 0.010) compared to PRE after eccentric exercise. There were no differences in CMJ between KET and CON (group; P = 0.571). The peak decline in absolute CMJ height from PRE during recovery after eccentric exercise was not different between groups (KET: −5.2 ± 3.8; CON: −4.9 ± 2.5 cm; P = 0.875).
Figure 4. Percent change in maximal isometric voluntary contraction (A) and counter-movement jump (B) relative to baseline (PRE) prior to exercise, and immediately (POST), 24 h and 48 h after exercise. Values represent means ± SD. Times without a common letter differ. Data were analyzed with a two-factor repeated measures (within subject factor: time; between-subject factor: group) ANOVA. Bonferroni-corrected post-hoc comparisons were performed following a significant main effect for time or group × time interaction. Maximal isometric voluntary contraction; time effect: P < 0.001; group effect: P = 0.612; group × time interaction: P = 0.594. Counter-movement jump; time effect: P < 0.001; group effect: P = 0.571; group × time interaction: P = 0.378. CMJ, counter-movement jump; CON, carbohydrate control group; KET, ketone monoester group; MIVC, maximal isometric voluntary contraction.
Ratings of muscle soreness (Figure 5A) were increased (time; P < 0.001) after eccentric exercise at POST, 24 h, and 48 h compared to PRE (all P < 0.001). Ratings of muscle soreness were also greater at 24 h (P = 0.010) compared to POST. There were no differences in ratings of muscle soreness between KET and CON (group; P = 0.896). The peak increase in absolute ratings of muscle soreness from PRE during recovery after eccentric exercise was not different between groups (KET: 53 ± 18; CON: 59 ± 20 cm; P = 0.946). The PPT (Figure 5B) was reduced (time; P < 0.001) at 24 h (P = 0.002) following eccentric exercise compared to PRE. There were no differences in PPT between KET and CON (group; P = 0.113). The peak decline in absolute PPT from PRE during recovery after eccentric exercise was not different between groups (KET: −129 ± 154; CON: −62 ± 39 kPa; P = 0.276).
Figure 5. Visual analog scale derived muscle soreness (A) and percent change in pressure-pain threshold (B) at or relative to baseline (PRE) prior to exercise, and immediately (POST), 24 h and 48 h after exercise. Values represent means ± SD. Times without a common letter differ. Data were analyzed with a two-factor repeated measures (within subject factor: time; between-subject factor: group) ANOVA. Bonferroni-corrected post-hoc comparisons were performed following a significant main effect for time or group × time interaction. Visual analog scale derived muscle soreness; time effect: P < 0.001; group effect: P = 0.896; group × time interaction: P = 0.517. Pressure-pain threshold; time effect: P < 0.001; group effect: P = 0.113; group × time interaction: P = 0.492. CON, carbohydrate control group; KET, ketone monoester group; PPT, pressure-pain threshold; VAS, visual analog scale.
Thigh circumference (see Supplementary Figure 1A) was increased (time; P < 0.001) at POST (P < 0.001) and 24 h (P = 0.015) compared to PRE. There were no differences in thigh circumference between KET and CON (group; P = 0.584). The peak increase in absolute thigh circumference from PRE during recovery after eccentric exercise was not different between groups (KET: 1.4 ± 0.9; CON: 1.4 ± 0.8 cm; P = 1.000). Calf circumference (see Supplementary Figure 1B) was increased (time; P = 0.001) at POST compared to PRE (P < 0.001) following the bout of eccentric exercise. There were no differences in calf circumference between KET and CON (group; P = 0.771). The peak increase in absolute calf circumference from PRE during recovery after eccentric exercise was not different between groups (KET: 0.6 ± 0.4; CON: 0.6 ± 0.4 cm; P = 0.791). Flexibility (see Supplementary Figure 1C) was not different over time (time; P = 0.428) or between KET and CON (group; P = 0.594).
BAM+ scores (see Supplementary Figure 2) were reduced (time; P = 0.001) at POST (P = 0.011) and 24 h (P = 0.008) following the bout of eccentric exercise compared to PRE. There were no differences in BAM+ scores between KET and CON (group; P = 0.793). The peak decline in BAM+ from PRE during recovery after eccentric exercise was not different between groups (KET: −28.1 ± 25.0; CON: −33.4 ± 22.7 AU; P = 0.626).
Plasma cytokines and chemokines are shown in Table 3. Plasma IL-1β showed a group × time interaction (P = 0.027); however, Bonferroni corrected pairwise comparisons showed no differences between KET and CON at any time-point (all P > 0.05). Plasma IL-6 was not different over time (time; P = 0.650) or between KET and CON (group; P = 0.166). Plasma IL-8 was not different over time (time; P = 0.677) or between KET and CON (group; P = 0.998). Plasma IL-10 was not different over time (time; P = 0.259) or between KET and CON (group; P = 0.594). Plasma IL-12(p70) was not different over time (time; P = 0.185) or between KET and CON (group; P = 0.287). Plasma MCP-1 was greater in CON compared to KET (group; P = 0.007). Plasma TNF-α was not different over time (time; P = 0.619) or between KET and CON (group; P = 0.153).
Table 3. Plasma IL-1β, IL-6, IL-8, IL-10, IL-12(p70), MCP-1, and TNF-α concentration at baseline (PRE) prior to exercise, and 24 h and 48 h after exercise in participants who ingested a ketone monoester supplement (KET) or energy-matched carbohydrate supplement (CON).
In the current study, we evaluated the impact of twice daily (morning and evening 30 min prior to sleep) supplementation (360 mg per kg−1 bodyweight per serving) with a ketone monoester (KET) as compared to an energy-matched carbohydrate control (CON) on indices of EIMD during recovery from a single bout of eccentric exercise in healthy, moderately active, young adults. MIVC was reduced by 19.9 ± 14.6 and 22.6 ± 11.1%, while CMJ was reduced by 11.0 ± 7.5 and 13.0 ± 8.7% at POST in KET and CON groups, respectively, following eccentric exercise. Acute ingestion of the ketone monoester supplement following POST testing markedly elevated circulating blood β-OHB concentration from 30 to 180 min after intake, demonstrating acute nutritional ketosis in the KET group. However, twice daily ketone monoester supplementation did not alter the exercise-induced increase in serum CK concentration, or expedite the recovery of MIVC or CMJ at 24 h or 48 h after exercise compared to CON. Similarly, there were no differences between KET and CON on measures of muscle soreness (VAS and PPT), limb girth, flexibility, BAM+, or the concentration of select pro- and anti-inflammatory cytokines. Overall, twice daily ingestion of a ketone monoester supplement that acutely elevates blood β-OHB concentration does not expedite the recovery of muscle performance or reduce muscle soreness following eccentric exercise in moderately active, healthy young adults.
Consistent with previous work (10), acute ingestion of the ketone monoester (KET) led to a rapid (within 30 min) and pronounced (peak: 4.4 ± 0.8 mM) increase in blood β-OHB concentration that was sustained for at least 180 min (Figure 2). Changes in blood β-OHB concentration were assessed in response to initial (first) supplement intake on Day 1 following POST testing; blood β-OHB concentration following supplement intake in the evening was not assessed as participants were instructed to ingest their respective treatment at home ~30 min prior to sleep. It has previously been reported that ketone monoester intake increases the blood β-OHB: acetoacetate concentration in a 5:1 ratio (34). Therefore, peak total blood ketone body concentrations of ~5.3 mM may have been achieved post-exercise; concentrations similar to those achieved with fasting (35).
Consistent with other studies utilizing the same exercise protocol (25, 31, 32), drop-jump exercise resulted in increases in serum CK concentration when assessed at 24 h and 48 h post-exercise (Figure 3). It has been suggested that a ketogenic diet may reduce EIMD based on the observation that blood CK was reduced 24 h post-exercise in rodents fed a ketogenic vs. control diet (36). Although both exogenous ketone supplements and a ketogenic diet both increase circulating blood ketone body concentration, they exert two distinct metabolic states (37–39) and may therefore have different effects on post-exercise recovery and/or performance. For example, ketone monoester ingestion can increase circulating β-OHB 3–5 mM within ~30 min without requiring carbohydrate restriction (11), whereas achieving nutritional ketosis via a ketogenic diet may take days to weeks and requires dietary carbohydrate restriction (37, 38). The lack of difference between KET and CON on serum CK concentration in the present study suggests that ketone monoester supplementation after exercise might not be beneficial for attenuating damage to the cell membrane resulting from eccentric exercise.
The decline in muscle force production following eccentric exercise is considered one of the most reliable indirect markers of EIMD (40, 41). Although not unequivocal, a number of nutritional interventions including use of branched-chain amino acids, protein, creatine, omega-3 polyunsaturated fatty acids, vitamin D, beetroot, pomegranate, and cherries have been reported to expedite impaired force production and/or performance after muscle damaging exercise [for reviews see (2, 5–7)]. In the present study, we hypothesized that ketone monoester supplementation and elevated β-OHB may accelerate the recovery of indices of muscle damage via mechanisms related to the reported capacity of β-OHB to stimulate protein synthesis (11, 17) and suppress proteolysis (19), and/or to modulate inflammation (42) and oxidative stress (43–45). Eccentric exercise led to a similar decline in MIVC at POST, indicating a similar initial response to the exercise stimulus in both the KET and CON group (Figure 4A). However, the recovery of MIVC did not differ between KET and CON groups when assessed 24 h or 48 h after exercise. Similarly, the eccentric exercise protocol led to an early decline in CMJ height at POST (Figure 4B); however, the recovery of CMJ height was not expedited in KET compared to CON at 24 h or 48 h post-exercise. To our knowledge, this is the first study to evaluate the impact of ketone monoester supplementation as a nutritional strategy to accelerate the recovery of impaired muscle function after eccentric exercise. In terms of the effects of ketone supplements on post-exercise recovery, Vandoorne et al. (11) recently reported that co-ingestion of a ketone monoester with protein and carbohydrate after strenuous exercise enhanced the post-exercise activation of downstream targets of mTORC1 compared to protein and carbohydrate only. Furthermore, ketone bodies increased leucine-mediated protein synthesis rates in C2C12 cells. However, post-exercise recovery was evaluated for 5 h and changes in muscle performance were not assessed. Huang et al. (36) reported that a ketogenic diet accelerated post-exercise recovery of performance in rodents based on measures of locomotion time taken 24 h after a bout of exhaustive exercise. However, as indicated above, there are substantial differences between a ketogenic diet and ketone supplements (37–39) and the translation of these findings to humans is unclear. Overall, twice daily supplementation with a ketone monoester that acutely raises circulating β-OHB does not expedite the recovery of muscle function based on static (MIVC) or dynamic (CMJ) measures of performance over the initial 2 days after eccentric exercise.
Muscle soreness is a common symptom following eccentric exercise and is the most utilized marker of muscle injury in muscle damage research (40). Recent evidence indicates that intramuscular generation of molecules, such as nerve growth factor, bradykinin, and prostaglandin E2, likely play an important role in muscle soreness due to EIMD (3, 46). These substances can be synthesized by immune cells and act to excite and sensitize local muscle nociceptors, resulting in sensations of pain and soreness in the muscle belly and connective tissues (3). A number of nutritional interventions have been reported to reduce muscle soreness following eccentric exercise [for reviews see (2, 5–7)]. In the present study, muscle soreness was increased in response to eccentric exercise based on both VAS and PPT measures, but there was no difference between KET and CON groups (Figures 5A,B). Therefore, ketone monoester supplementation does not appear to be an effective nutritional strategy to reduce muscle soreness that manifests over the initial 2 days during recovery after eccentric exercise.
Nutritional solutions to expedite the recovery of indices of muscle damage are thought to interact with the secondary damage cascade characterized by inflammation and oxidative stress, to attenuate the exacerbation of damage and aid subsequent recovery (2, 9). Ketone bodies appear to modulate inflammation and immune cell function, with β-OHB exerting a predominantly anti-inflammatory response (20). β-OHB has been reported to lead to anti-inflammatory effects in TNF-α and lipopolysaccharide-induced inflammation by reducing pro-inflammatory proteins, such as inducible nitric oxide synthase and cyclooxygenase-2, or cytokines, such as TNF-α, IL-1β, IL-6, and MCP-1, partly by inhibiting NF-κB (47, 48). In a recent animal study, β-OHB was also shown to decrease inflammation via reducing inflammatory cytokines, such as IL-1β via blocking the NOD-, LRR-, and pyrin domain-containing protein 3 (NLRP3) inflammasome pathway (42). Antioxidant and oxidative stress-mitigating roles of ketone bodies have also been described (20). For example, ketone bodies have been shown to reduce cellular damage, injury, death, and apoptosis in cardiomyocytes and neurons (45, 49, 50), with β-OHB able to scavenge reactive oxygen species, such as hydroxyl anion (49). Recently, β-OHB has been shown to be a histone deacetylase inhibitor, which can increase the expression of antioxidant genes and proteins responsible for decreasing cellular oxidative stress (43). In the present study, we measured plasma concentrations of select pro- and anti-inflammatory cytokines [IL-1β, IL-6, IL-10, IL-12(p70), and TNF-α] and chemokines (IL-8 and MCP-1) at baseline (PRE), 24 h and 48 h post-exercise recovery (Table 3). We observed no difference over time or between KET and CON groups on all markers except MCP-1, which was lower in KET vs. CON (group: P = 0.007). This may suggest that (1) the eccentric exercise drop-jump protocol was not damaging enough to induce a systemic inflammatory response, (2) that these systemic markers are not representative of the inflammatory response to eccentric exercise, or (3) that we missed early circulating cytokine responses due to our chosen sampling time-points (i.e., 24 h and 48 h post-exercise). Regarding the first point, previous studies using a similar exercise protocol (51, 52) have reported a marked inflammatory response, including significant elevations in IL-6 up to 24 h post-exercise (52). Differences between these studies and the present investigation may relate to differences in the study population. Regarding the second point, a review by Peake et al. (53) indicated that plasma concentrations of IL-1β, IL-6, IL-8, IL-10, MCP-1, and TNF-α increase in response to exercise. However, plasma cytokine responses with exercise are quite variable between individuals and are dependent on the combination of mode, intensity, and duration of exercise (53). Therefore, we believe there was a strong rationale for examining the plasma concentrations of these cytokines and chemokines with exercise but acknowledge that a different type and/or intensity of exercise may have led to a more robust systemic cytokine response. Regarding the third point, Paulsen et al. (41) mapped the time course for systemic cytokine responses to various forms of eccentric exercise and reported that many systemic cytokine responses occur <24 h post-exercise. Given that the first post-exercise blood sample in the present study was taken 24 h after exercise, we may not have captured early time and or group-dependent differences in circulating cytokines. However, even if we missed any group dependent differences in circulating cytokine concentration that may have occurred early after exercise (<24 h), these were clearly insufficient to augment the recovery of muscle function (MIVC and CMJ) or muscle soreness (VAS and PPT) at 24 h and 48 h post-exercise. To better capture the exercise-induced inflammatory response, early post-exercise blood sample collection, evaluation of additional markers of inflammation, and assessment of intramuscular cytokine mRNA/protein expression and/or inflammatory cell (e.g., neutrophils, macrophages) infiltration (4) may be necessary.
Potential limitations to the present study include the parallel group nature of the study design, choice of eccentric exercise protocol to study indices of EIMD, and utilization of moderately active (regularly exercising 2–4 times per week) research participants. The inter-subject response to EIMD is quite variable (54), and therefore, the absence of a significant difference between the KET and CON group on indices of EIMD may be partly due to the high biological variability of the subjects. While numerous studies have applied box drop exercise within the context of EIMD research (24, 25, 31, 32, 55), the amount of muscle damage experienced by the majority of the research participants in the present study based on the decline in MIVC would be considered mild to moderate (41). Utilization of a more damaging form of eccentric exercise (e.g., unaccustomed isolated maximal eccentric exercise across a large range of motion) and/or utilization of exercise naïve research participants to minimize any potential impact of the repeated bout effect may have resulted in more severe EIMD. Whether ketone monoester supplementation may facilitate recovery following severe EIMD [i.e., eccentric exercise leading to a decline in force-generating capacity >50% and/or recovery of force-generating capacity that exceeds 7 days (41)] is unclear and requires further investigation. More severe EIMD that is accompanied by a greater inflammatory response may represent an environment where any potential benefit of ketone monoester supplementation on post-exercise recovery can be better unmasked.
In summary, results of the present study demonstrate that twice daily ingestion of a ketone monoester supplement that acutely elevates blood β-OHB concentration does not enhance the recovery of muscle performance or reduce muscle soreness following an acute bout of eccentric exercise in moderately active, healthy young adults.
The raw data supporting the conclusions of this article will be made available by the authors, without undue reservation.
The studies involving human participants were reviewed and approved by Faculty of Medicine Institutional Review Board of McGill University. The patients/participants provided their written informed consent to participate in this study.
PM-A and TC-V conceived and designed the research, analyzed the data, interpreted the results of the experiments, prepared the figures, drafted the manuscript, and hold the primary responsibility for the final content. PM-A, JL, and JD conducted the research. PM-A, JM, and TC-V edited and revised the manuscript. All authors read and approved the final manuscript.
Funding was provided by internal McGill University research funds to TC-V.
The authors declare that the research was conducted in the absence of any commercial or financial relationships that could be construed as a potential conflict of interest.
The authors acknowledge the enthusiastic support of the subjects who volunteered to participate in this study. We also thank Ms. Ophir Danenberg for her assistance with analysis of the dietary intake records and Ms. Chloé Fleurent-Grégoire for her assistance with analysis of the VAS data. We also thank Prof. Ross Andersen and Dr. Julie Côté for providing access to some of the research equipment used in this study.
The Supplementary Material for this article can be found online at: https://www.frontiersin.org/articles/10.3389/fnut.2020.607299/full#supplementary-material
1. Howatson G, van Someren KA. The prevention and treatment of exercise-induced muscle damage. Sports Med. (2008) 38:483–503. doi: 10.2165/00007256-200838060-00004
2. Owens DJ, Twist C, Cobley JN, Howatson G, Close GL. Exercise-induced muscle damage: what is it, what causes it and what are the nutritional solutions? Eur J Sport Sci. (2019) 19:71–85. doi: 10.1080/17461391.2018.1505957
3. Hyldahl RD, Hubal MJ. Lengthening our perspective: morphological, cellular, and molecular responses to eccentric exercise. Muscle Nerve. (2014) 49:155–70. doi: 10.1002/mus.24077
4. Peake JM, Neubauer O, Della Gatta PA, Nosaka K. Muscle damage and inflammation during recovery from exercise. J Appl Physiol (1985). (2017) 122:559–70. doi: 10.1152/japplphysiol.00971.2016
5. Harty PS, Cottet ML, Malloy JK, Kerksick CM. Nutritional and supplementation strategies to prevent and attenuate exercise-induced muscle damage: a brief review. Sports Med Open. (2019) 5:1. doi: 10.1186/s40798-018-0176-6
6. Sousa M, Teixeira VH, Soares J. Dietary strategies to recover from exercise-induced muscle damage. Int J Food Sci Nutr. (2014) 65:151–63. doi: 10.3109/09637486.2013.849662
7. Bongiovanni T, Genovesi F, Nemmer M, Carling C, Alberti G, Howatson G. Nutritional interventions for reducing the signs and symptoms of exercise-induced muscle damage and accelerate recovery in athletes: current knowledge, practical application and future perspectives. Eur J Appl Physiol. (2020) 120:1965–96. doi: 10.1007/s00421-020-04432-3
8. Pasiakos SM, Lieberman HR, McLellan TM. Effects of protein supplements on muscle damage, soreness and recovery of muscle function and physical performance: a systematic review. Sports Med. (2014) 44:655–70. doi: 10.1007/s40279-013-0137-7
9. Bloomer RJ. The role of nutritional supplements in the prevention and treatment of resistance exercise-induced skeletal muscle injury. Sports Med. (2007) 37:519–32. doi: 10.2165/00007256-200737060-00005
10. Clarke K, Tchabanenko K, Pawlosky R, Carter E, Todd King M, Musa-Veloso K, et al. Kinetics, safety and tolerability of (R)-3-hydroxybutyl (R)-3-hydroxybutyrate in healthy adult subjects. Regul Toxicol Pharmacol. (2012) 63:401–8. doi: 10.1016/j.yrtph.2012.04.008
11. Vandoorne T, De Smet S, Ramaekers M, Van Thienen R, De Bock K, Clarke K, et al. Intake of a ketone ester drink during recovery from exercise promotes mTORC1 signaling but not glycogen resynthesis in human muscle. Front Physiol. (2017) 8:310. doi: 10.3389/fphys.2017.00310
12. Margolis LM, O'Fallon KS. Utility of ketone supplementation to enhance physical performance: a systematic review. Adv Nutr. (2020) 11:412–9. doi: 10.1093/advances/nmz104
13. Valenzuela PL, Morales JS, Castillo-Garcia A, Lucia A. Acute ketone supplementation and exercise performance: a systematic review and meta-analysis of randomized controlled trials. Int J Sports Physiol Perform. (2020) 15:298–308. doi: 10.1123/ijspp.2019-0918
14. Pinckaers PJ, Churchward-Venne TA, Bailey D, van Loon LJ. Ketone bodies and exercise performance: the next magic bullet or merely hype? Sports Med. (2017) 47:383–91. doi: 10.1007/s40279-016-0577-y
15. Evans M, Cogan KE, Egan B. Metabolism of ketone bodies during exercise and training: physiological basis for exogenous supplementation. J Physiol. (2017) 595:2857–71. doi: 10.1113/JP273185
16. Stubbs BJ, Koutnik AP, Volek JS, Newman JC. From bedside to battlefield: intersection of ketone body mechanisms in geroscience with military resilience. Geroscience. (2020). doi: 10.1007/s11357-020-00277-y. [Epub ahead of print].
17. Nair KS, Welle SL, Halliday D, Campbell RG. Effect of beta-hydroxybutyrate on whole-body leucine kinetics and fractional mixed skeletal muscle protein synthesis in humans. J Clin Invest. (1988) 82:198–205. doi: 10.1172/JCI113570
18. Thomsen HH, Rittig N, Johannsen M, Moller AB, Jorgensen JO, Jessen N, et al. Effects of 3-hydroxybutyrate and free fatty acids on muscle protein kinetics and signaling during LPS-induced inflammation in humans: anticatabolic impact of ketone bodies. Am J Clin Nutr. (2018) 108:857–67. doi: 10.1093/ajcn/nqy170
19. Sherwin RS, Hendler RG, Felig P. Effect of ketone infusions on amino acid and nitrogen metabolism in man. J Clin Invest. (1975) 55:1382–90. doi: 10.1172/JCI108057
20. Puchalska P, Crawford PA. Multi-dimensional roles of ketone bodies in fuel metabolism, signaling, and therapeutics. Cell Metab. (2017) 25:262–84. doi: 10.1016/j.cmet.2016.12.022
21. Zou X, Meng J, Li L, Han W, Li C, Zhong R, et al. Acetoacetate accelerates muscle regeneration and ameliorates muscular dystrophy in mice. J Biol Chem. (2016) 291:2181–95. doi: 10.1074/jbc.M115.676510
22. Goossens C, Weckx R, Derde S, Dufour T, Vander Perre S, Pauwels L, et al. Adipose tissue protects against sepsis-induced muscle weakness in mice: from lipolysis to ketones. Crit Care. (2019) 23:236. doi: 10.1186/s13054-019-2506-6
23. Shearer DA, Sparkes W, Northeast J, Cunningham DJ, Cook CJ, Kilduff LP. Measuring recovery: an adapted brief assessment of mood (BAM+) compared to biochemical and power output alterations. J Sci Med Sport. (2017) 20:512–7. doi: 10.1016/j.jsams.2016.09.012
24. Clifford T, Ventress M, Allerton DM, Stansfield S, Tang JCY, Fraser WD, et al. The effects of collagen peptides on muscle damage, inflammation and bone turnover following exercise: a randomized, controlled trial. Amino Acids. (2019) 51:691–704. doi: 10.1007/s00726-019-02706-5
25. Howatson G, Hoad M, Goodall S, Tallent J, Bell PG, French DN. Exercise-induced muscle damage is reduced in resistance-trained males by branched chain amino acids: a randomized, double-blind, placebo controlled study. J Int Soc Sports Nutr. (2012) 9:20. doi: 10.1186/1550-2783-9-20
26. Keane KM, Salicki R, Goodall S, Thomas K, Howatson G. Muscle damage response in female collegiate athletes after repeated sprint activity. J Strength Cond Res. (2015) 29:2802–7. doi: 10.1519/JSC.0000000000000961
27. Howatson G, Goodall S, van Someren KA. The influence of cold water immersions on adaptation following a single bout of damaging exercise. Eur J Appl Physiol. (2009) 105:615–21. doi: 10.1007/s00421-008-0941-1
28. Bridgeman LA, Gill ND, Dulson DK, McGuigan MR. The effect of exercise-induced muscle damage after a bout of accentuated eccentric load drop jumps and the repeated bout effect. J Strength Cond Res. (2017) 31:386–94. doi: 10.1519/JSC.0000000000001725
29. Nosaka K, Kuramata T. Muscle soreness and serum enzyme activity following consecutive drop jumps. J Sports Sci. (1991) 9:213–20. doi: 10.1080/02640419108729882
30. Jakeman JR, Byrne C, Eston RG. Lower limb compression garment improves recovery from exercise-induced muscle damage in young, active females. Eur J Appl Physiol. (2010) 109:1137–44. doi: 10.1007/s00421-010-1464-0
31. Clifford T, Bell O, West DJ, Howatson G, Stevenson EJ. The effects of beetroot juice supplementation on indices of muscle damage following eccentric exercise. Eur J Appl Physiol. (2016) 116:353–62. doi: 10.1007/s00421-015-3290-x
32. Clifford T, Howatson G, West DJ, Stevenson EJ. Beetroot juice is more beneficial than sodium nitrate for attenuating muscle pain after strenuous eccentric-bias exercise. Appl Physiol Nutr Metab. (2017) 42:1185–91. doi: 10.1139/apnm-2017-0238
33. Bowtell JL, Sumners DP, Dyer A, Fox P, Mileva KN. Montmorency cherry juice reduces muscle damage caused by intensive strength exercise. Med Sci Sports Exerc. (2011) 43:1544–51. doi: 10.1249/MSS.0b013e31820e5adc
34. Soto-Mota A, Norwitz NG, Clarke K. Why a d-beta-hydroxybutyrate monoester? Biochem Soc Trans. (2020) 48:51–9. doi: 10.1042/BST20190240
35. Cahill GF Jr. Fuel metabolism in starvation. Annu Rev Nutr. (2006) 26:1–22. doi: 10.1146/annurev.nutr.26.061505.111258
36. Huang Q, Ma S, Tominaga T, Suzuki K, Liu C. An 8-week, low carbohydrate, high fat, ketogenic diet enhanced exhaustive exercise capacity in mice part 2: effect on fatigue recovery, post-exercise biomarkers and anti-oxidation capacity. Nutrients. (2018) 10:1339. doi: 10.3390/nu10101339
37. Shaw DM, Merien F, Braakhuis A, Maunder E, Dulson DK. Exogenous ketone supplementation and keto-adaptation for endurance performance: disentangling the effects of two distinct metabolic states. Sports Med. (2020) 50:641–56. doi: 10.1007/s40279-019-01246-y
38. Poff AM, Koutnik AP, Egan B. Nutritional ketosis with ketogenic diets or exogenous ketones: features, convergence, and divergence. Curr Sports Med Rep. (2020) 19:251–9. doi: 10.1249/JSR.0000000000000732
39. Ma S, Suzuki K. Keto-adaptation and endurance exercise capacity, fatigue recovery, and exercise-induced muscle and organ damage prevention: a narrative review. Sports (Basel). (2019) 7:40. doi: 10.3390/sports7020040
40. Warren GL, Lowe DA, Armstrong RB. Measurement tools used in the study of eccentric contraction-induced injury. Sports Med. (1999) 27:43–59. doi: 10.2165/00007256-199927010-00004
41. Paulsen G, Mikkelsen UR, Raastad T, Peake JM. Leucocytes, cytokines and satellite cells: what role do they play in muscle damage and regeneration following eccentric exercise? Exerc Immunol Rev. (2012) 18:42–97.
42. Youm YH, Nguyen KY, Grant RW, Goldberg EL, Bodogai M, Kim D, et al. The ketone metabolite beta-hydroxybutyrate blocks NLRP3 inflammasome-mediated inflammatory disease. Nat Med. (2015) 21:263–9. doi: 10.1038/nm.3804
43. Shimazu T HM, Newman J, He W, Shirakawa K, Le, Moan N GC, et al. Suppression of oxidative stress by β-hydroxybutyrate, an endogenous histone deacetylase inhibitor. Science. (2013) 339:211–4. doi: 10.1126/science.1227166
44. Newman JC, Verdin E. Ketone bodies as signaling metabolites. Trends Endocrinol Metab. (2014) 25:42–52. doi: 10.1016/j.tem.2013.09.002
45. Maalouf M SP, Davis L, Kim DY, Rho JM. Ketones inhibit mitochondrial production of reactive oxygen species production following glutamate excitotoxicity by increasing NADH oxidation. Neuroscience. (2007) 145:256–64. doi: 10.1016/j.neuroscience.2006.11.065
46. Murase S, Terazawa E, Queme F, Ota H, Matsuda T, Hirate K, et al. Bradykinin and nerve growth factor play pivotal roles in muscular mechanical hyperalgesia after exercise (delayed-onset muscle soreness). J Neurosci. (2010) 30:3752–61. doi: 10.1523/JNEUROSCI.3803-09.2010
47. Fu SP, Li SN, Wang JF, Li Y, Xie SS, Xue WJ, et al. BHBA suppresses LPS-induced inflammation in BV-2 cells by inhibiting NF-kappaB activation. Mediators Inflamm. (2014) 2014:983401. doi: 10.1155/2014/983401
48. Gambhir D, Ananth S, Veeranan-Karmegam R, Elangovan S, Hester S, Jennings E, et al. GPR109A as an anti-inflammatory receptor in retinal pigment epithelial cells and its relevance to diabetic retinopathy. Invest Ophthalmol Vis Sci. (2012) 53:2208–17. doi: 10.1167/iovs.11-8447
49. Haces ML, Hernandez-Fonseca K, Medina-Campos ON, Montiel T, Pedraza-Chaverri J, Massieu L. Antioxidant capacity contributes to protection of ketone bodies against oxidative damage induced during hypoglycemic conditions. Exp Neurol. (2008) 211:85–96. doi: 10.1016/j.expneurol.2007.12.029
50. Nagao M, Toh R, Irino Y, Mori T, Nakajima H, Hara T, et al. beta-Hydroxybutyrate elevation as a compensatory response against oxidative stress in cardiomyocytes. Biochem Biophys Res Commun. (2016) 475:322–8. doi: 10.1016/j.bbrc.2016.05.097
51. Dousset E, Avela J, Ishikawa M, Kallio J, Kuitunen S, Kyrolainen H, et al. Bimodal recovery pattern in human skeletal muscle induced by exhaustive stretch-shortening cycle exercise. Med Sci Sports Exerc. (2007) 39:453–60. doi: 10.1249/mss.0b013e31802dd74e
52. Chatzinikolaou A, Fatouros IG, Gourgoulis V, Avloniti A, Jamurtas AZ, Nikolaidis MG, et al. Time course of changes in performance and inflammatory responses after acute plyometric exercise. J Strength Cond Res. (2010) 24:1389–98. doi: 10.1519/JSC.0b013e3181d1d318
53. Peake JM, Gatta PD, Suzuki K, Nieman DC. Cytokine expression and secretion by skeletal muscle cells: regulatory mechanisms and exercise effects. Exerc Immunol Rev. (2015) 21:8–25.
54. Beaton LJ, Tarnopolsky MA, Phillips SM. Variability in estimating eccentric contraction-induced muscle damage and inflammation in humans. Can J Appl Physiol. (2002) 27:516–26. doi: 10.1139/h02-028
Keywords: ketone supplements, ketosis, post-exercise recovery, muscle damage, eccentric exercise, exercise performance
Citation: Martin-Arrowsmith PW, Lov J, Dai J, Morais JA and Churchward-Venne TA (2020) Ketone Monoester Supplementation Does Not Expedite the Recovery of Indices of Muscle Damage After Eccentric Exercise. Front. Nutr. 7:607299. doi: 10.3389/fnut.2020.607299
Received: 16 September 2020; Accepted: 11 November 2020;
Published: 08 December 2020.
Edited by:
Michael D. Roberts, Auburn University, United StatesReviewed by:
Cleiton Augusto Libardi, Federal University of São Carlos, BrazilCopyright © 2020 Martin-Arrowsmith, Lov, Dai, Morais and Churchward-Venne. This is an open-access article distributed under the terms of the Creative Commons Attribution License (CC BY). The use, distribution or reproduction in other forums is permitted, provided the original author(s) and the copyright owner(s) are credited and that the original publication in this journal is cited, in accordance with accepted academic practice. No use, distribution or reproduction is permitted which does not comply with these terms.
*Correspondence: Tyler A. Churchward-Venne, dHlsZXIuY2h1cmNod2FyZC12ZW5uZUBtY2dpbGwuY2E=
Disclaimer: All claims expressed in this article are solely those of the authors and do not necessarily represent those of their affiliated organizations, or those of the publisher, the editors and the reviewers. Any product that may be evaluated in this article or claim that may be made by its manufacturer is not guaranteed or endorsed by the publisher.
Research integrity at Frontiers
Learn more about the work of our research integrity team to safeguard the quality of each article we publish.