- School of Health Science and Engineering, University of Shanghai for Science and Technology, Shanghai, China
In this study, a two-dimensional Ti3C2Tx MXene compounded with carbon nanohorn (CNH) by an electrostatic self-assembly method was proposed and then fabricated as room temperature ammonia (NH3) gas sensors. The successful preparation of the Ti3C2Tx/CNH nanocomposite has been characterized in detail. The NH3 sensing performance based on Ti3C2Tx/CNH also has been tested at room temperature. The optimal Ti3C2Tx/CNH sensor has a response value of 21.6% to 100 ppm NH3 at room temperature, which is 10 times higher than that of the pure Ti3C2Tx sensor. Furthermore, this sensor is endowed with excellent selectivity, reliable long-term stability, and reproducibility. The enhanced sensing performance is associated with the interconnected structure and the synergistic effect of Ti3C2Tx and CNH. This work provides an effective way to prepare MXene-based sensitive materials for NH3 sensors, which shows excellent NH3 detection potential at room temperature.
1 Introduction
Ammonia (NH3) detection is important in the industrial environment and for early diagnosis of medical diseases. Excess NH3 is harmful to human health as it increases the incidence of respiratory and cardiovascular diseases (Li et al., 2016; Achary et al., 2018; Wang et al., 2018; Wu et al., 2019). Long-term exposure may cause blindness or ammonia poisoning in human beings. NH3 exposure limitations have been set as 35 ppm for 10 min and 25 ppm for 8 h (Fedoruk et al., 2005; Gangopadhyay et al., 2008). In addition, it can be directly life-threatening when the concentrations of NH3 are up to 300 ppm (Raj et al., 2010). NH3 is also a natural body metabolite, and high levels of NH3 in exhaled gas may reflect several liver and lung-related diseases (Narasimhan et al., 2001; Pagonas et al., 2012; Obermeier et al., 2017), which is potentially applied for noninvasive disease diagnostics. The concentration of exhaled NH3 for healthy people is in the range of 0.4–1.8 ppm, but that in the breath of end-stage renal disease patients is normally beyond 4.9 ppm. Thus, NH3 sensors can potentially be applied for noninvasive diagnosis of renal disease (Ji et al., 2019).
MXene is a group of transition metal carbides, nitrides, and carbonitrides with a layered structure similar to that of two-dimensional graphene (Su et al., 2022). Due to their unique surficial and electrical properties, multiple MXenes with a rich chemical composition have a wide range of promising applications in energy storage, hydrogen storage, chemical biosensors, and purification (Zhu et al., 2017; Zhang et al., 2018). Ti3C2Tx MXenes with high electrical conductivity, numerous active sites, and abundant terminal functional groups have been widely used as an excellent sensitive material for gas detection at room temperature. The type and number of oxygen-containing functional groups can change the conductivity of Ti3C2Tx MXenes and also effectively act as functional sites for gas adsorption. Ti3C2Tx MXene is a potential NH3-sensitive material (Anasori et al., 2017), which displays more sensitivity to NH3 than CO2, O2, and H2 because the etched Ti3C2Tx surface is enriched with oxygen functional groups (Wu et al., 2019). However, pristine Ti3C2Tx MXenes with relatively lower capability have rarely been used as sensing materials directly.
The methods to improve the sensor performance mainly include modification of second-phase materials to enhance the activity of the sensitive material or the design of new structures to further promote the practical production applications of gas sensors. The construction of heterostructures effectively improves the gas sensing performance of Ti3C2Tx MXene. The electronic properties regulated by the interface of heterostructures may result in enhanced charge transport. Researchers have fabricated the gas sensing materials based on the MXene heterostructures with carbon nanostructures of reduced graphene oxide and carbon nanotubes (Lee et al., 2020; Hassan et al., 2021; Seekaew et al., 2023). These heterostructures combine the high conductivity and structural stability of carbon nanostructures with the merit of Ti3C2Tx MXene (Keshtkara et al., 2017). Carbon nanohorn (CNH) is a carbon nanomaterial with an angular appearance and aggregated subspherical shape and has abundant active sites and good electrical conductivity (Zhu et al., 2009; Zhu et al., 2019). It has also been widely used for immunosensing, storage, and electrochemical detection of gases such as methane and hydrogen (Ojeda et al., 2014; Chen et al., 2017; Zheng et al., 2020). Zhou et al. (2022) prepared In2O3/CNH nanocomposites and fabricated ideal low-power H2S gas sensors. Therefore, combining Ti3C2Tx MXene with CNH may be promising for improving the sensing properties.
Herein, Ti3C2Tx/CNH nanocomposites have been fabricated by an electrostatic self-assembly method. The CNH surface is positively charged by adding the surfactant and then combined with the negatively charged Ti3C2Tx via an electrostatic self-assembly method. CNH has a larger specific surface area, providing more reaction sites and gas adsorption capacity, and the gas sensor made of Ti3C2Tx/CNH nanocomposite displays good response, excellent selectivity, and reliable stability for NH3 detection at room temperature.
2 Experimental section
2.1 Preparation of sensing materials
2.1.1 Preparation of Ti3C2Tx MXene
For selective etching of Al from Ti3AlC2 MAX, we used a mixed etching solution containing hydrochloric acid and sodium fluoride as follows: 2 g Ti3AlC2 powder was slowly added to an etching solution composed of 40 mL 6 M hydrochloric acid and 2 g sodium fluoride (Wu et al., 2019). The mixed dispersion was continuously stirred at 60°C for 48 h in a water bath. The dispersion was then washed with ethanol and deionized water several times to remove the acidic residue until the pH value was 6. The precipitate was dried in a vacuum oven at 60°C for approximately 8 h to obtain the multilayer Ti3C2Tx powder. Then, an appropriate amount of Ti3C2Tx powder was added to deionized water and dispersed by ultrasonication to obtain 2 mg/mL of Ti3C2Tx dispersion.
2.1.2 Synthesis of Ti3C2Tx/CNH nanocomposites
Figure 1 shows the synthesis process of Ti3C2Tx/CNH nanocomposites, where Ti3C2Tx/CNH nanocomposites were successfully synthesized by an electrostatic self-organization method. CNH was obtained from Henan Yingneng Novel Material Technology Co., Ltd. CNH (50 mg) was dispersed in 10 mL of polydimethyldiallylammonium chloride (PDDA) and stirred for 6 h at 25°C to obtain positively charged CNH. Then, different amounts of Ti3C2Tx were added (CNH accounted for 0.1, 0.5, 1.0, and 5.0 wt%, respectively), and the dispersions were continued to be stirred for 24 h at 25°C. The obtained dispersion was centrifuged and washed with deionized water and ethanol in turn and dried in a vacuum oven to obtain Ti3C2Tx/CNH nanocomposites.
2.2 Fabrication and characterization of the gas-sensing devices
The component of the sensor mainly includes a ceramic tube with a gold electrode coated with a sensitive material and a sensor base. The process of fabrication is as follows: the sensitive material is mixed with ethanol and sonicated for 15 min to form a stable dispersion. Then, 5 μL of the prepared dispersion was uniformly coated on the ceramic tube by using a pipette, and the gold electrode was completely covered to form a sensitive layer. The gas-sensing performance was analyzed at room temperature and 40%–45% relative humidity (RH) by using a CGS-8 analysis system from Elite Technology Co., Ltd. A static gas-blending method was used to obtain the target gases with different concentrations, and the detailed processes have been listed in the previous work (Han et al., 2023). The gas-sensing response is defined as S = |Rg-Ra|/Ra × 100%, where Rg and Ra represent the sensors’ resistance in the target gas and air, respectively (Han et al., 2023; Yao et al., 2023).
2.3 Characterizations
The crystal phases and chemical bonding of the as-prepared materials were analyzed by X-ray powder diffraction (XRD, Rigaku Ultima IV) and X-ray photoelectron spectroscopy (XPS, Shimadzu/Kratos AXIS SUPRA+), respectively. Field-emission scanning electron microscopy (FESEM, JSM-7001F) and transmission electron microscopy (TEM, JEM-F200) were performed to characterize the morphology and microstructure of the sensitive materials, respectively. The Fourier-transform infrared (FT-IR) spectrum was performed on PerkinElmer Spectrum 3.
3 Results and discussion
3.1 Characterizations of sensitive materials
Figure 2A displays the XRD patterns of Ti3AlC2 and Ti3C2Tx MXene. The typical diffraction peaks of Ti3AlC2-MAX at 9.5, 19.2, 34.1, 36.9, 38.9, 41.8, 48.4, 42.4, 56.5, 60.2, 65.4, 70.2, and 74.0° correspond to (002), (004), (101), (103), (104), (105), (107), (108), (109), (110), (1011), (119), and (1013) crystal planes, respectively (Tian et al., 2022). Compared with Ti3AlC2, the characteristic peaks of the (002) and (004) facets of Ti3C2Tx were reduced from 2θ = 9.5° to 5.7° and 2θ = 19.2° to 17.2°, respectively. Furthermore, the appearance of new characteristic peaks at 28.8° for (006) and 34.7° for (0010) also implies successful formation of Ti3C2Tx. Figure 2B presents the XRD patterns of CNH, Ti3C2Tx, and Ti3C2Tx/CNH composites. For the XRD patterns of Ti3C2Tx/CNH, the characteristic peaks of Ti3C2Tx can be observed in all Ti3C2Tx/CNH composites with different amounts of CNH, but no pronounced CNH peaks can be found for the low CNH content (Zhou et al., 2022).
The morphologies of Ti3AlC2, Ti3C2Tx, CNH, and Ti3C2Tx/CNH nanocomposites were characterized by FESEM. As shown in Figure 3A, Ti3AlC2 has a dense multilayer structure. SEM images of multilayer Ti3C2Tx are displayed in Figure 3B, and there is an obvious spacing between the lamellae, a typical accordion-like structure, and a smooth surface. Figure 3C presents the SEM image of CNH nanospheres with an average diameter of 70–110 nm. The morphology of Ti3C2Tx/CNH (0.5 wt%) composite nanomaterials is exhibited in Figure 3D, indicating the successful combination between CNH and Ti3C2Tx.
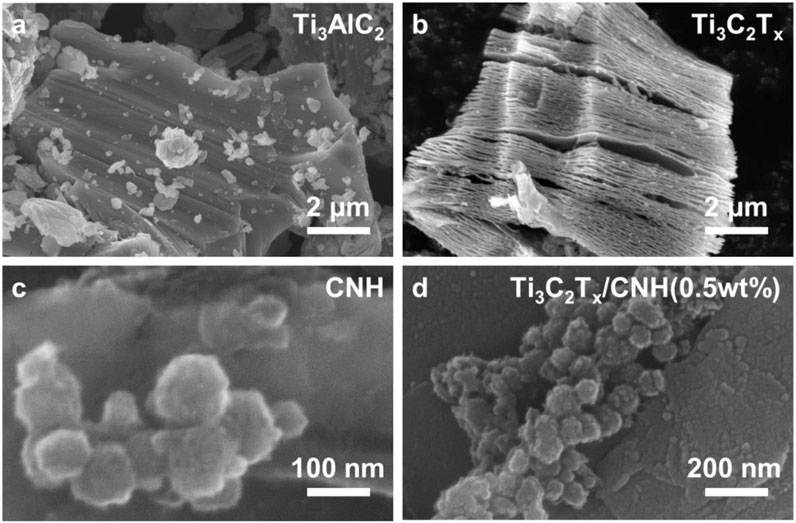
Figure 3. FESEM images of the (A) Ti3AlC2, (B) Ti3C2Tx, (C) CNH, and (D) Ti3C2Tx/CNH (0.5 wt%) nanocomposites.
To further investigate the microstructure of the sensitive material, the Ti3C2Tx/CNH sample was characterized by TEM and high-resolution transmission electron microscopy (HRTEM). The TEM images of Ti3C2Tx/CNH (0.5 wt%) in Figure 4A also confirm the successful combination between Ti3C2Tx and CNH nanospheres. For Ti3C2Tx/CNH, it can be seen that Ti3C2Tx is composed of thin sheets with a two-dimensional lamellar structure, and the presence of CNH nanospheres is confirmed. The HRTEM images of the Ti3C2Tx/CNH composites are shown in Figure 4B. The spacing of the lattice surfaces in Ti3C2Tx is 0.237 nm, which can be attributed to the (110) crystal plane of Ti3C2Tx (Zhang et al., 2018). The lattice spacing stripes are not obvious due to the poor crystallinity of CNH. The CNH microspheres and Ti3C2Tx nanosheets are displayed in Figure 4, which are involved in the successful preparation of Ti3C2Tx/CNH nanocomposites.
The chemical bonding of Ti3C2Tx/CNH samples was further analyzed by XPS, and the corresponding results are exhibited in Figure 5. Figure 5A shows the C 1s spectrum of Ti3C2Tx/CNH with peaks at 293.3, 286.4, 284.8, and 281.4 eV, which are attributed to C=O, C–O, C–C, and C–Ti functional groups, respectively (Zhou et al., 2022). For the O spectrum in Figure 5B, the peak at 531.1 eV is typical of surface lattice oxygen, while the peak located at 532.9 eV is characteristic of surface-adsorbed oxygen species (O2– and O−) (Andreeva et al., 1998; Hu et al., 2018; Lee et al., 2018). The surface-adsorbed oxygen reacts with the target gas molecules through redox reactions, thus effectively enhancing the gas-sensitive properties (Wang et al., 2015). The high-resolution Ti 2p spectrum is displayed in Figure 5C, which corresponds the peaks at 460.7, 459.3, 456.6, and 453.6 eV to Ti–X (Ti+), T–C (Ti2+), TixOy (Ti3+), and Ti–O (Ti2+), respectively, where Ti–X corresponds to the sub-stoichiometric ratios of titanium carbide or titanium oxide nitride (Halim et al., 2014). The F 1s spectrum is presented in Figure 5D, and the peaks appearing at 688.9 and 687.5 eV can correspond to F–Ti and F–C, respectively (He et al., 2021). Therefore, the XPS results indicate the successful preparation of Ti3C2Tx/CNH composites, which is consistent with the XRD, SEM, and TEM results. Supplementary Figure S1 shows the FT-IR spectra of Ti3C2Tx, CNH, and Ti3C2Tx/CNH. The bands lower than 1,000 cm−1 correspond to the vibrations of Ti–O and C–O. The bands at 1,350 and 1,630 cm−1 may be related to the vibrations of O–H stretching and C=O bonding, respectively (Zhou et al., 2022).
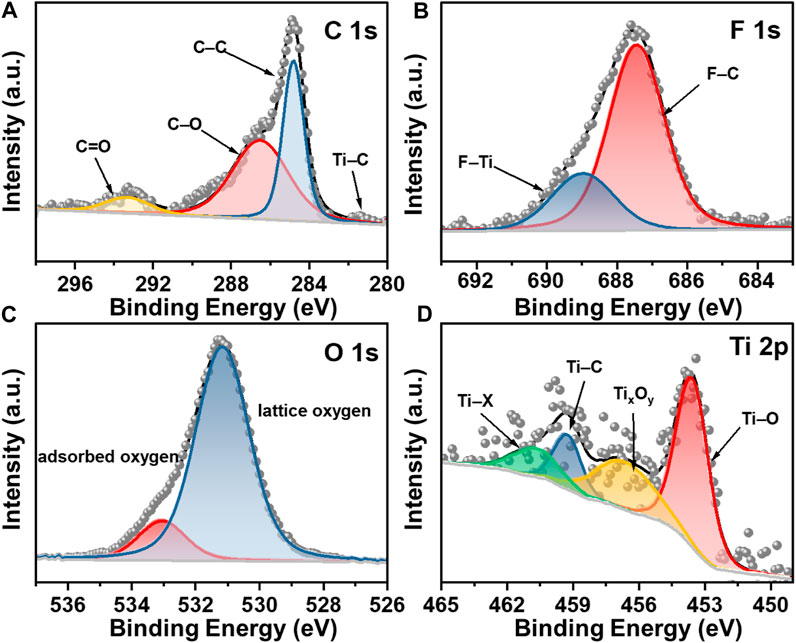
Figure 5. (A) C 1s, (B) F 1s, (C) O 1s, and (D) Ti 2p fine spectrum of Ti3C2Tx/CNH (0.5 wt%) composites, respectively.
3.2 Gas-sensing performance
To investigate the effect of CNH content on the NH3-sensing performance of Ti3C2Tx/CNH sensors, the sensing characteristics of Ti3C2Tx/CNH composite sensors with different CNH contents have been tested at room temperature. Figure 6A shows I–V curves of the Ti3C2Tx, CNH, and Ti3C2Tx/CNH (0.5 wt%) sensors, which display the typical Ohmic contacts, and thus, the resistances of the gas sensors are decided by the sensitive materials rather than the contact characteristics (Yuan et al., 2018; Han et al., 2021). As shown in Figure 6B, Supplementary Figure S2, the responses of pure Ti3C2Tx, CNH, Ti3C2Tx/CNH (0.1 wt%), Ti3C2Tx/CNH (0.5 wt%), Ti3C2Tx/CNH (1.0 wt%), and Ti3C2Tx/CNH (5.0 wt%) sensors to 100 ppm NH3 are 2.0, 8.7, 21.6, 10.8, and 8.7%, respectively. The Ti3C2Tx/CNH (0.5 wt%) sensor is endowed with the highest response, which is approximately 10 times the response value of previously reported Ti3C2Tx-based sensors, indicating that CNH nanospheres significantly enhance the response to NH3 (Wu et al., 2019). As the CNH content increases, the response to NH3 increases accordingly, and the best peak-response (∼21.6%) is obtained at a CNH content of 0.5 wt%, indicating that the cooperation between Ti3C2Tx and CNH significantly enhances the NH3-response. However, as the CNH content in the composite continues to increase, the response of the composite to NH3 decreases instead. It may be because CNH has a large specific surface area, which can provide more reactive sites and help in improving the gas-sensitive response, while excess CNH covered with Ti3C2Tx decreases the number of reactive sites on the Ti3C2Tx surface, which affects the adsorption of NH3 by Ti3C2Tx. Selectivity is considered to be an important key metric for evaluating gas sensors. The response and recovery time of the gas sensor are important parameters to be examined when the sensor is used in practice. As shown in Figure 6C, when the gas sensor is exposed to 100 ppm NH3, the resistance value of the gas-sensing element reaches 90% of the equilibrium value in about 48 s, and the sensor recovery time is calculated as 196 s (Chen et al., 2022). The response of the Ti3C2Tx/CNH (0.5 wt%) sensor at room temperature to various interfering gases at 100 ppm is displayed in Figure 6D. The sensor has responses of 2, 1, 2, 2, 1, 21.6, and 3% to 100 ppm hydrogen sulfide, isopropyl alcohol, ethylene glycol, acetone, ethanol, ammonia, and formaldehyde, respectively. The Ti3C2Tx/CNH-based sensor shows excellent selectivity for NH3 gas. As listed in Table 1, the response of the Ti3C2Tx/CNH-based sensor is competitive with that of the reported Ti3C2Tx-based NH3 sensors.
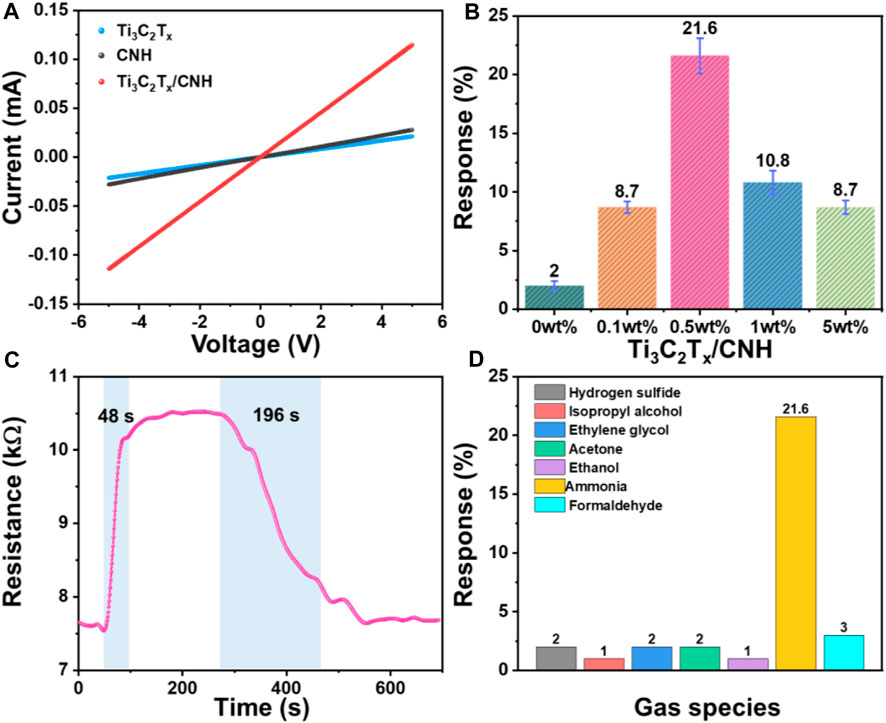
Figure 6. (A) I–V curves of comparison of Ti3C2Tx, CNH, and Ti3C2Tx/CNH (0.5 wt%). (B) Response of different ratios of Ti3C2Tx/CNH samples to 100 ppm NH3 at room temperature. (C) Dynamic response/recovery characteristic of the Ti3C2Tx/CNH (0.5 wt%) sensor to 100 ppm NH3 at room temperature. (D) Response of the Ti3C2Tx/CNH (0.5 wt%) sensor to different gases at 100 ppm.
The dynamic response of the optimal Ti3C2Tx/CNH (0.5 wt%) sensor to different concentrations (1–50 ppm) of NH3 gas is displayed in Figure 7A. Figure 7B shows that the corrected curve is almost linear, with a correlation coefficient R2 of 0.9877. The detection limit of this Ti3C2Tx/CNH sensor is calculated to be about 250 ppb (Han et al., 2023). The response of the Ti3C2Tx/CNH (0.5 wt%) gas sensor increases linearly with increasing NH3 concentration, showing a good linearity. Reproducibility and long-term stability are also important indexes in the practical application of the sensor. Exposure of the sensor to 50 ppm NH3 has been performed for six consecutive cycles at room temperature (Figure 7C). The results show that the composite sensor has good cycling reproducibility with no significant change in NH3 detection during the continuous adsorption–desorption process. Moreover, as shown in Figure 7D, the stable response of the sensor to 100 ppm NH3 over 30 days indicates that the device also exhibits relatively good long-term stability. Furthermore, Supplementary Figure S3 shows the response/recovery characteristics of the Ti3C2Tx/CNH sensor to 50 ppm NH3 at 20, 40, and 55% RH conditions. The results show that the response values of the Ti3C2Tx/CNH sensor to 50 ppm NH3 remain stable at about 10% within the RH range of 20%–55%.
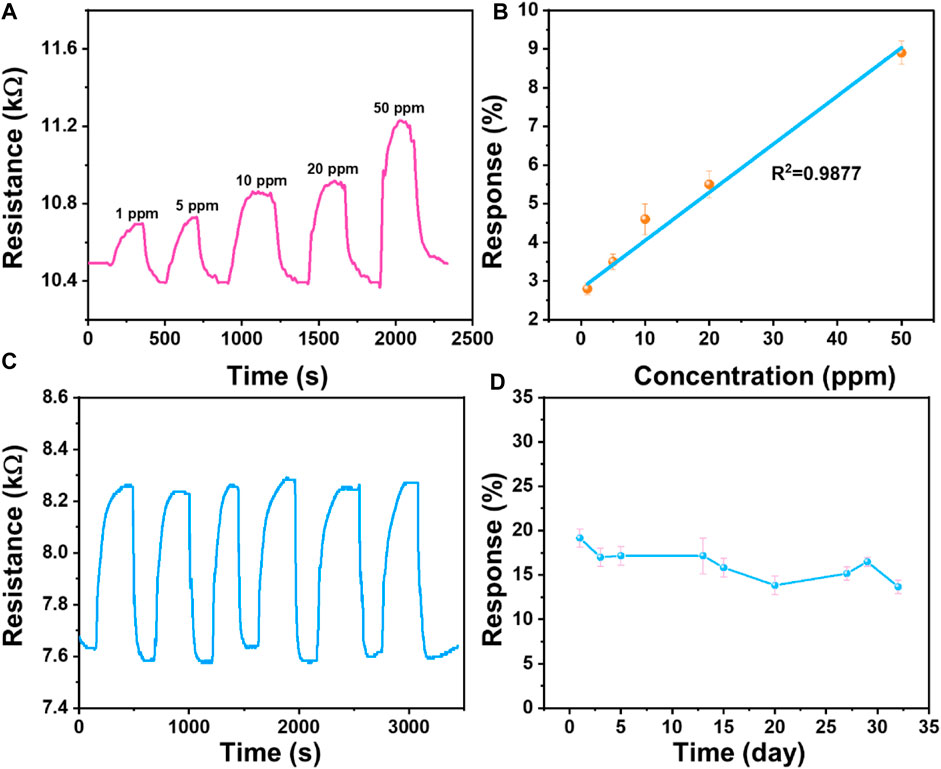
Figure 7. (A) Dynamic response/recovery curve of the Ti3C2Tx/CNH (0.5 wt%) sensor at room temperature for a concentration range of 1–50 ppm NH3. (B) Fitting curves between gas concentrations and response values of the Ti3C2Tx/CNH (0.5 wt%) sensor. (C) Repeatability of the Ti3C2Tx/CNH (0.5 wt%) sensor to 50 ppm NH3 for six continuous cycles. (D) Long-term stability of the Ti3C2Tx/CNH (0.5 wt%) sensor to 100 ppm NH3.
3.3 Gas-sensing mechanisms
The improved gas-sensing performance of the Ti3C2Tx/CNH (0.5 wt%)-based sensor for NH3 can be explained by the following aspects:
Ti3C2Tx has abundant and active surface termination groups, which is beneficial for the sensing performance. The decorated CNH can provide more adsorption sites for the target gas, and the loading of CNH particles on the surface and interlayers of Ti3C2Tx nanosheets slows down the accumulation of layered Ti3C2Tx nanosheets and increases the Ti3C2Tx nanosheet layer spacing. Compared with pure Ti3C2Tx, the loaded CNH increases the specific surface area of Ti3C2Tx/CNH composites, improves the adsorption and diffusion of NH3, and contributes to the improved NH3-response.
A schematic diagram of the gas-sensing mechanism of the Ti3C2Tx/CNH sensor is displayed in Figures 8A, B. Ti3C2Tx exhibits p-type sensing behavior, and the relevant literature shows that its bandgap is 1.45 eV. CNH is a p-type semiconductor with a bandgap of 0.4 eV. The work functions of Ti3C2Tx and CNH are 3.9 and 5.0 eV, respectively (Lee et al., 2017; Schultz et al., 2019; Zhou et al., 2022). As shown in Figure 8C, in the Ti3C2Tx/CNH nanocomposite, nanoscale p–p heterojunctions are formed between Ti3C2Tx and CNH. Since the work function of CNH is higher than that of Ti3C2Tx, electrons will flow from the latter to the former, resulting in the formation of a hole depletion layer (HDL) in CNH and a hole accumulation layer (HAL) in Ti3C2Tx (Yao et al., 2023).
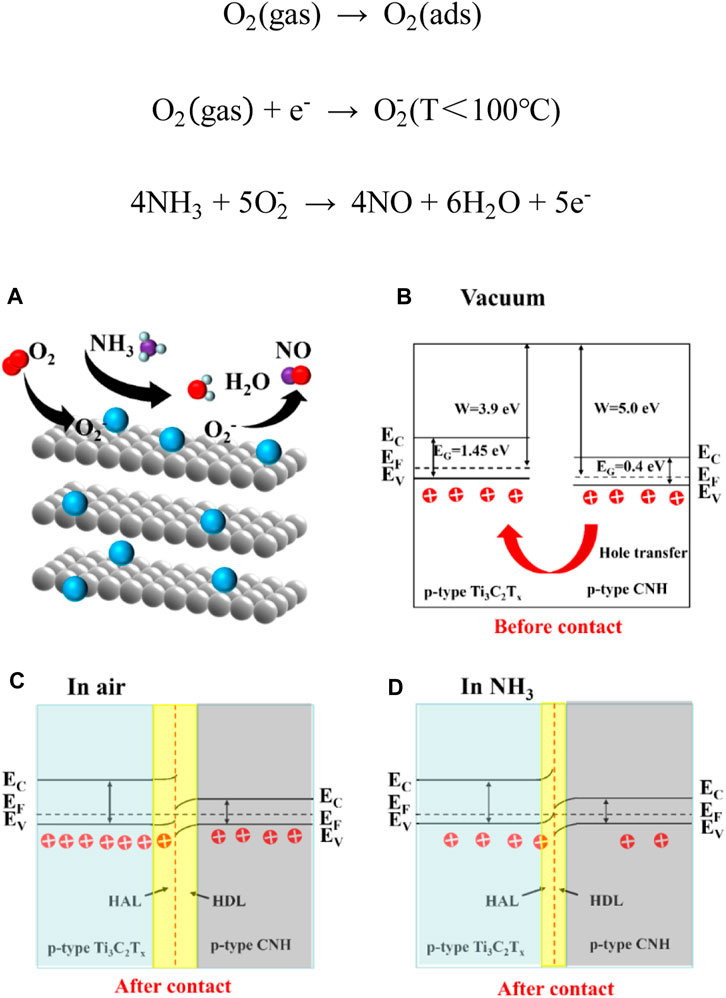
Figure 8. Schematic diagram of (A) the gas-sensing mechanism of the Ti3C2Tx/CNH sensor, (B) energy band diagram of Ti3C2Tx and CNH before forming a complex, and energy band structure of Ti3C2Tx/CNH composite in (C) air and (D) NH3.
In air, oxygen molecules are adsorbed on the surface of the composite (Eqs 1 and 2). The oxygen molecules adsorb on the CNH surface, and the negative charge formed on the surface will increase its work function, thus increasing the work function difference between CNH and Ti3C2Tx. This will require transfer of more electrons to compensate. As a result, the HAL thickness in Ti3C2Tx increases, and the resistance of the sensor in air is relatively low. When the composite is exposed to NH3, the NH3 molecules react with
4 Conclusion
Ti3C2Tx/CNH nanocomposites have been prepared by an electrostatic self–assembly method, and the characterization and gas–sensitive sensing properties of the material are investigated. The experimental results show the Ti3C2Tx/CNH (0.5 wt%) nanocomposite sensor has good gas–sensing performance for NH3 gas at room temperature, indicating that CNH can effectively optimize the sensor and make Ti3C2Tx MXene more suitable for detecting NH3 at room temperature.
Data availability statement
The original contributions presented in the study are included in the article/Supplementary Material; further inquiries can be directed to the corresponding author.
Author contributions
YH: writing–review and editing, conceptualization, funding acquisition, investigation, and writing–original draft. YD: writing–original draft. YY: writing–review and editing. ZL: writing–review and editing. ZZ: writing–review and editing.
Funding
The author(s) declare that financial support was received for the research, authorship, and/or publication of this article. The authors gratefully acknowledge financial supports by the National Natural Science Foundation of China (62104149) and the Shanghai Sailing Program (21YF1431400).
Conflict of interest
The authors declare that the research was conducted in the absence of any commercial or financial relationships that could be construed as a potential conflict of interest.
Publisher’s note
All claims expressed in this article are solely those of the authors and do not necessarily represent those of their affiliated organizations, or those of the publisher, the editors, and the reviewers. Any product that may be evaluated in this article, or claim that may be made by its manufacturer, is not guaranteed or endorsed by the publisher.
Supplementary material
The Supplementary Material for this article can be found online at: https://www.frontiersin.org/articles/10.3389/fmats.2024.1383538/full#supplementary-material
References
Achary, L. S. K., Kumar, A., Barik, B., Nayak, P. S., Tripathy, N., Kar, J. P., et al. (2018). Reduced graphene oxide-CuFe2O4 nanocomposite: a highly sensitive room temperature NH3 gas sensor. Sens. Actuators B 272, 100–109. doi:10.1016/j.snb.2018.05.093
Anasori, B., Lukatskaya, M. R., and Gogotsi, Y. (2017). 2D metal carbides and nitrides (MXenes) for energy storage. Nat. Rev. Mat. 2 (2), 16098. doi:10.1038/natrevmats.2016.98
Andreeva, D., Tabakova, T., Idakiev, V., Christov, P., and Giovanoli, R. (1998). Au/α-Fe2O3 catalyst for water–gas shift reaction prepared by deposition–precipitation. Appl. Catal. A 169 (1), 9–14. doi:10.1016/s0926-860x(97)00302-5
Chen, C., Jiang, M., Luo, X., Tai, H., Jiang, Y., Yang, M., et al. (2022). Ni-Co-P hollow nanobricks enabled humidity sensor for respiratory analysis and human-machine interfacing. Sens. Actuators, B 370, 132441. doi:10.1016/j.snb.2022.132441
Chen, J., He, P., Bai, H., Lei, H., Liu, K., Dong, F., et al. (2017). Novel phosphomolybdic acid/single-walled carbon nanohorn-based modified electrode for non-enzyme glucose sensing. J. Electroanal. Chem. 784, 41–46. doi:10.1016/j.jelechem.2016.12.003
Fedoruk, M. J., Bronstein, R., and Kerger, B. D. (2005). Ammonia exposure and hazard assessment for selected household cleaning product uses. J. Expo. Anal. Environ. Epidemiol. 15 (6), 534–544. doi:10.1038/sj.jea.7500431
Gangopadhyay, R. K., and Das, S. K. (2008). Ammonia leakage from refrigeration plant and the management practice. Process Saf. Prog. 27 (1), 15–20. doi:10.1002/prs.10208
Halim, J., Lukatskaya, M. R., Lu, J., Cook, K. M., Smith, C. R., Näslund, L. Å., et al. (2014). Transparent conductive two-dimensional titanium carbide epitaxial thin films. Chem. Mat. 26 (7), 2374–2381. doi:10.1021/cm500641a
Han, Y., Ding, Y., Zhang, W., Zhuang, H., Wang, Z., Li, Z., et al. (2023). SnS2/Ti3C2Tx hybrids for conductometric triethylamine detection at room temperature. Sens. Actuators, B 381, 133360. doi:10.1016/j.snb.2023.133360
Han, Y., Liu, Y., Su, C., Chen, X., Li, B., Jiang, W., et al. (2021). Hierarchical WS2−WO3 nanohybrids with P−N heterojunctions for NO2 detection. ACS Appl. Nano Mat. 4, 1626–1634. doi:10.1021/acsanm.0c03094
Hassan, K., Stanley, N., Tung, T., Yap, P. L., Rastin, H., Yu, L., et al. (2021). Extrusion-printed CNT–graphene sensor array with embedded MXene/PEDOT:PSS heater for enhanced NO2 sensing at low temperature. Adv. Mat. Interfaces 8, 2101175. doi:10.1002/admi.202101175
He, T., Liu, W., Lv, T., Ma, M., Liu, Z., Vasiliev, A., et al. (2021). MXene/SnO2 heterojunction based chemical gas sensors. Sens. Actuators, B 329, 129275. doi:10.1016/j.snb.2020.129275
Hu, X., Zhu, Z., Li, Z., Xie, L., Wu, Y., and Zheng, L. (2018). Heterostructure of CuO microspheres modified with CuFe2O4 nanoparticles for highly sensitive H2S gas sensor. Sens. Actuators, B 264, 139–149. doi:10.1016/j.snb.2018.02.110
Ji, H., Zeng, W., and Li, Y. (2019). Gas sensing mechanisms of metal oxide semiconductors: a focus review. Nanoscale 11 (47), 22664–22684. doi:10.1039/c9nr07699a
Keshtkara, S., Rashidib, A., and Kootia, M. (2017). Development of tin dioxide quantum dots/multi-walled carbon nanotubes and tin dioxide quantum dots/carbon nanohorns nanohybrids as low temperatures natural gas sensors. Ceram. Int. 41, 4314326–4314333. doi:10.1016/j.ceramint.2017.07.188
Kim, S., Lee, J., Doo, S., Kang, Y. C., and Koo, C. M. (2021). Metal-ion-intercalated MXene nanosheet films for NH3 gas detection. ACS Appl. Nano Mat. 4, 14249–14257. doi:10.1021/acsanm.1c03814
Kim, S. J., Koh, H.-J., Ren, C. E., Kwon, O., Maleski, K., Cho, S. Y., et al. (2018). Metallic Ti3C2Tx MXene gas sensors with ultrahigh signal-to-noise ratio. ACS Nano 12, 986–993. doi:10.1021/acsnano.7b07460
Lee, E., VahidMohammadi, A., Prorok, B. C., Yoon, Y. S., Beidaghi, M., and Kim, D. J. (2017). Room temperature gas sensing of two-dimensional titanium carbide (MXene). ACS Appl. Mat. Interfaces 9 (42), 37184–37190. doi:10.1021/acsami.7b11055
Lee, J., Kim, S. Y., Yoo, H. S., and Lee, W. (2022). Pd-WO3 chemiresistive sensor with reinforced self-assembly for hydrogen detection at room temperature. Sens. Actuators, B 368, 132236. doi:10.1016/j.snb.2022.132236
Lee, S., Eom, W., Shin, H., Ambade, R. B., Bang, J. H., Kim, H. W., et al. (2020). Room-temperature, highly durable Ti3C2Tx MXene/graphene hybrid fibers for NH3 gas sensing. ACS Appl. Mat. Interfaces 12, 10434–10442. doi:10.1021/acsami.9b21765
Li, Y., Ban, H., and Yang, M. (2016). Highly sensitive NH3 gas sensors based on novel polypyrrole-coated SnO2 nanosheet nanocomposites. Sens. Actuators B 224, 449–457. doi:10.1016/j.snb.2015.10.078
Narasimhan, L. R., Goodman, W., and Patel, C. K. N. (2001). Correlation of breath ammonia with blood urea nitrogen and creatinine during hemodialysis. Proc. Natl. Acad. Sci. 98 (8), 4617–4621. doi:10.1073/pnas.071057598
Obermeier, J., Trefz, P., Happ, J., Schubert, J. K., Staude, H., Fischer, D. C., et al. (2017). Exhaled volatile substances mirror clinical conditions in pediatric chronic kidney disease. PLoS One 12 (6), e0178745. doi:10.1371/journal.pone.0178745
Ojeda, I., Garcinuño, B., Moreno-Guzmán, M., González-Cortés, A., Yudasaka, M., Iijima, S., et al. (2014). Carbon nanohorns as a scaffold for the construction of disposable electrochemical immunosensing platforms application to the determination of fibrinogen in human plasma and urine. Anal. Chem. 86 (15), 7749–7756. doi:10.1021/ac501681n
Pagonas, N., Vautz, W., Seifert, L., Slodzinski, R., Jankowski, J., Zidek, W., et al. (2012). Volatile organic compounds in uremia. PLoS One 7 (9), e46258. doi:10.1371/journal.pone.0046258
Raj, V. B., Nimal, A. T., Parmar, Y., Sharma, M., Sreenivas, K., and Gupta, V. (2010). Cross-sensitivity and selectivity studies on ZnO surface acoustic wave ammonia sensor. Sens. Actuators, B 147 (2), 517–524. doi:10.1016/j.snb.2010.03.079
Schultz, T., Frey, N. C., Hantanasirisakul, K., Park, S., May, S. J., Shenoy, V. B., et al. (2019). Surface termination dependent work function and electronic properties of Ti3C2Tx MXene. Chem. Mat. 31 (17), 6590–6597. doi:10.1021/acs.chemmater.9b00414
Seekaew, Y., Kamlue, S., and Wongchoosuk, C. (2023). Room-temperature ammonia gas sensor based on Ti3C2Tx MXene/graphene oxide/CuO/ZnO nanocomposite. ACS Appl. Nano Mat. 6, 9008–9020. doi:10.1021/acsanm.3c01637
Su, Y., Li, W., Cheng, X., Zhou, Y., Yang, S., Zhang, X., et al. (2022). High-performance piezoelectric composites via β phase programming. Nat. Commun. 13 (4867), 1–12. doi:10.1038/s41467-022-32518-3
Suh, J. M., Sohn, W., Shim, Y.-S., Choi, J. S., Song, Y. G., Kim, T. L., et al. (2018). P–p heterojunction of nickel oxide-decorated cobalt oxide nanorods for enhanced sensitivity and selectivity toward volatile organic compounds. ACS Appl. Mat. Interfaces 10 (1), 1050–1058. doi:10.1021/acsami.7b14545
Tian, X., Yao, L., Cui, X., Zhao, R., Chen, T., Xiao, X., et al. (2022). A two-dimensional Ti3C2TX MXene@TiO2/MoS2 heterostructure with excellent selectivity for the room temperature detection of ammonia. J. Mat. Chem. A 10 (10), 5505–5519. doi:10.1039/d1ta10773a
Wang, J., Li, Z., Zhang, S., Yan, S., Cao, B., Wang, Z., et al. (2018). Enhanced NH3 gas-sensing performance of silica modified CeO2 nanostructure based sensors. Sens. Actuators, B 255, 862–870. doi:10.1016/j.snb.2017.08.149
Wang, S., Zhang, J., Yang, J., Gao, X., Zhang, H., Wang, Y., et al. (2015). Spinel ZnFe2O4 nanoparticle-decorated rod-like ZnO nanoheterostructures for enhanced gas sensing performances. RSC Adv. 5 (13), 10048–10057. doi:10.1039/c4ra14033h
Wu, M., He, M., Hu, Q., Wu, Q., Sun, G., Xie, L., et al. (2019). Ti3C2 MXene-based sensors with high selectivity for NH3 detection at room temperature. ACS Sens. 4 (10), 2763–2770. doi:10.1021/acssensors.9b01308
Yang, Z., Liu, A., Wang, C., Liu, F., He, J., Li, S., et al. (2019). Improvement of gas and humidity sensing properties of organ-like MXene by alkaline treatment. ACS Sens. 4, 1261–1269. doi:10.1021/acssensors.9b00127
Yao, Y., Li, Z., Han, Y., Xie, L., Zhao, X., and Zhu, Z. (2023). Fabrication and characterization of a MnO2/Ti3C2Tx based gas sensor for highly sensitive and selective detection of lung cancer marker hexanal. Chem. Eng. J. 451, 139029. doi:10.1016/j.cej.2022.139029
Yuan, W., Yang, K., Peng, H., Li, F., and Yin, F. (2018). A flexible VOCs sensor based on a 3D Mxene framework with a high sensing performance. J. Mat. Chem. A 6, 18116–18124. doi:10.1039/c8ta06928j
Zhang, Y., Guo, B., Hu, L., Xu, Q., Li, Y., Liu, D., et al. (2018). Synthesis of SnS nanoparticle-modified MXene (Ti3C2Tx) composites for enhanced sodium storage. J. Alloys Compd. 732, 448–453. doi:10.1016/j.jallcom.2017.10.223
Zhang, Y., Wang, L., Zhang, N., and Zhou, Z. (2018). Adsorptive environmental applications of MXene nanomaterials: a review. RSC Adv. 8 (36), 19895–19905. doi:10.1039/c8ra03077d
Zheng, W., Liu, Y., Yang, P., Chen, Y., Tao, J., Hu, J., et al. (2020). Carbon nanohorns enhanced electrochemical properties of Cu-based metal organic framework for ultrasensitive serum glucose sensing. J. Electroanal. Chem. 862, 114018. doi:10.1016/j.jelechem.2020.114018
Zhou, M., Yao, Y., Han, Y., Xie, L., Zhao, X., Barsan, N., et al. (2022). Ultrasensitive gas sensor based on nanocube In2O3-CNH composite at low operating temperature. Sens. Actuators, B 354, 131224. doi:10.1016/j.snb.2021.131224
Zhu, G., Fiston, M. N., Qian, J., and Kingsford, O. J. (2019). Highly sensitive electrochemical sensing of para-chloronitrobenzene using a carbon nanohorn–nanotube hybrid modified electrode. Anal. Methods 11 (8), 1125–1130. doi:10.1039/c8ay02680g
Zhu, J., Ha, E., Zhao, G., Zhou, Y., Huang, D., Yue, G., et al. (2017). Recent advance in MXenes: a promising 2D material for catalysis, sensor and chemical adsorption. Coord. Chem. Rev. 352, 306–327. doi:10.1016/j.ccr.2017.09.012
Keywords: gas sensors, Ti3C2Tx, ammonia, MXene, room temperature
Citation: Han Y, Ding Y, Yao Y, Li Z and Zhu Z (2024) Heterostructured Ti3C2Tx/carbon nanohorn-based gas sensor for NH3 detection at room temperature. Front. Mater. 11:1383538. doi: 10.3389/fmats.2024.1383538
Received: 07 February 2024; Accepted: 15 April 2024;
Published: 24 May 2024.
Edited by:
Qinghong Yuan, East China Normal University, ChinaReviewed by:
Kedhareswara Sairam Pasupuleti, Chungnam National University, Republic of KoreaZheng Guo, Anhui University, China
Copyright © 2024 Han, Ding, Yao, Li and Zhu. This is an open-access article distributed under the terms of the Creative Commons Attribution License (CC BY). The use, distribution or reproduction in other forums is permitted, provided the original author(s) and the copyright owner(s) are credited and that the original publication in this journal is cited, in accordance with accepted academic practice. No use, distribution or reproduction is permitted which does not comply with these terms.
*Correspondence: Zhigang Zhu, emhpZ2FuZ196aHUyNTlAMTYzLmNvbQ==
†These authors have contributed equally to this work