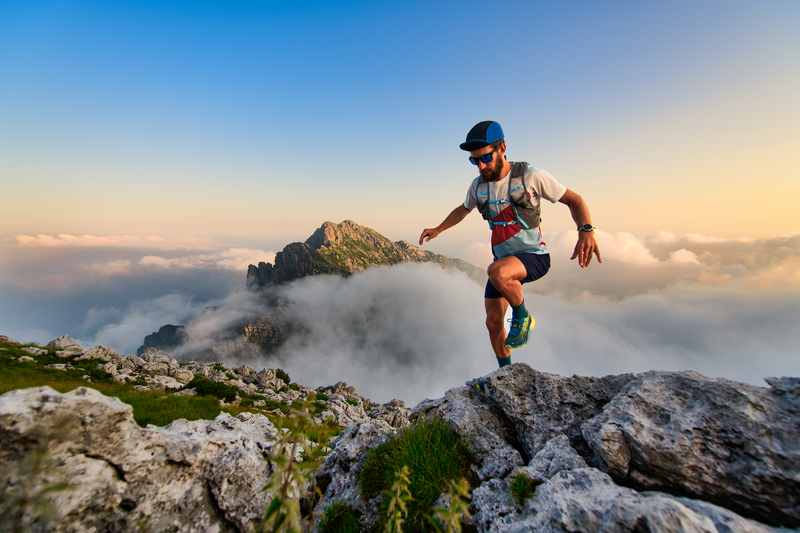
95% of researchers rate our articles as excellent or good
Learn more about the work of our research integrity team to safeguard the quality of each article we publish.
Find out more
ORIGINAL RESEARCH article
Front. Mater. , 21 May 2024
Sec. Smart Materials
Volume 11 - 2024 | https://doi.org/10.3389/fmats.2024.1400258
With the energy crisis and environmental pollution becoming a growing concern worldwide, the development of clean and renewable energy from the environment has become an imperative for human survival and development. However, the equipment used to harvest clean renewable energy is large, subject to environmental impacts and regional differences (such as wind, solar and tidal energy). In this study, a biodegradable eggshell membrane triboelectric nanogenerator (EM-TENG) is introduced for the purpose of harvesting low-frequency mechanical energy. A Wireless Intelligent Motion Monitoring System (WIMMS) has been created using EM-TENG. It includes a Bluetooth sensor terminal and an intelligent processing terminal for digital signal reception on a host computer. The EM-TENG can be attached to knee and ankle joints to monitor posture. Therefore, for real-time monitoring of joint and kinetic chain changes during land training of ice dance athletes, the intelligent ice dance land training aid system is important. As a wearable motion monitoring sensor, EM-TENGs application in intelligent motion monitoring, intelligent wearable devices and big data analytics is being promoted.
Many problems such as the global energy crisis and ecological changes have been caused by the continuous consumption of fossil fuels and the massive emission of carbon dioxide (Coccia, 2020; Gurieff et al., 2020; Liu et al., 2020; Feng et al., 2021; Wang et al., 2022; Qu et al., 2023). The search for carbon emission reduction and renewable energy technologies is an imperative for the green economy and sustainable development of human civilization in response to global energy shortages and current environmental pollution problems (Zhu et al., 2020; Li Y. H. et al., 2021). Photovoltaic, thermoelectric, piezoelectric, electrostatic and electromagnetic induction-based systems are flourishing by offering a new energy harvesting strategy (Zhao J. et al., 2022). However, these system architecture applications are too com-plex and severely limited by light intensity, temperature variations, etc. Furthermore, the increasing prevalence of diverse intelligent machines and electronic devices has presented a significant challenge in ensuring an adequate supply of energy for all electronic devices and sensors. Consequently, there is a pressing necessity to harness available energy from the surrounding environment. (Li Y. H. et al., 2023; Mi et al., 2023). The tribo-electric nanogenerator (TENG), invented by Zhonglin Wang and his team in 2012, has been widely recognized as a novel, practical and effective device for harvesting energy from the environment, as evidenced by numerous studies conducted by researchers (Fan et al., 2021; Li Y. M. et al., 2023; Du et al., 2023; Li et al., 2024; Shao et al., 2024). The TENG as an innovative technology based on the coupling effects of contact electrification and electrostatic induction converts low-frequency mechanical energy into electrical energy (Yin et al., 2019; Guo and Liu, 2020; Sun et al., 2020; Pradel and Fukata, 2021; Yang et al., 2021; Hao et al., 2022; Wen et al., 2023; Zhu et al., 2023). Because of its unique advantages of self-powered, low-cost, simple structure, easy manufacturing and various material options, it has been used in various fields, such as environmental energy harvesting, medical monitoring, intelligent security systems, etc (Liang et al., 2017; Wen et al., 2018; Xie et al., 2020; Luo J. et al., 2021; Shen et al., 2022; Zeng et al., 2022; Zhu et al., 2022; Bagchi et al., 2023). Except energy harvesting, TENG can be designed as an adaptable and low-cost wearable signal transmission device, which is widely noticed in the field of motion information monitoring (Yi et al., 2019; Li R. et al., 2021; Su et al., 2021; Dudem et al., 2022; Linnamo, 2023).
Ice dance is famous for its “ballet on ice”. It combines the beauty of music, gestures and power, which is one of the most ornamental winter sports. Ice dance has a strong focus on steps and strict limitations on technical movements, emphasizing the use of movement to express music. Land-based training is an important training approach and method for the whole ice sport to perfect and improve the competitive ability (Kovacs et al., 2004). Ice dance land technique training is a special training conducted on land, which has deepening and integrating facilitating effects on the comprehensive ability of ice dance athletes and can improve their technical and performance ability. However, excessive physical load and experience inculcation in the training process are still the main ways to improve ice dance performance. Its scientific and systematic data monitoring and analysis are lacking. At present, in the field of monitoring technology, systematizing movement and improving sports training performance, artificial intelligence has a wide range of applications (Haug et al., 2019; Gang et al., 2021; Lu et al., 2021; Tomita et al., 2021). Such as wearable technology to promote real-time sports data collection, intelligent training venues and digital technology to promote functional monitoring systematization, AI and intelligent robotics to promote efficient sports training, etc (Chung et al., 2019; Xie et al., 2019; Luo Z. B. et al., 2021; Cheng et al., 2021; Zhao T. M. et al., 2022). Ice dance will also benefit from the application of artificial intelligence to further improve the ability to monitor various sport performance related parameters (Panfili et al., 2022; Cao et al., 2023). Conventional electronic devices usually require external power sources to operate, with problems such as limited battery and supercapacitor life, heavy weight, high replacement cost and potential environmental pollution. The development of an EM-TENG based Wireless Intelligent Movement Monitoring System (Wireless Intelligent Motion Monitoring System) to monitor ice dance land training is essential (Bruening et al., 2018; Kim et al., 2021; Lu et al., 2022).
This study introduces a versatile and eco-friendly EM-TENG with eggshell membrane and polytetrafluoroethylene friction layers. EM-TENG is small, light, stable, sensitive and biodegradable. To avoid sweat erosion of EM-TENG, it was encapsulated with PDMS. Subsequently, it was utilized to monitor the land technique training of ice dancers, with the collected data being analyzed to develop personalized training programs for the athletes. This information serves as a valuable resource for addressing challenges encountered during ice dance training. The EM-TENG can quickly and accurately transfer data to the terminal for visual display due to its sensitive response time (53 ms) and excellent performance. It is based on EM-TENG, the Bluetooth sensor terminal and the terminal for receiving the digital signal in the intelligent processing of the host computer. A system combines requirements and indicators of land training with ice training to im-prove the technical and performance abilities of ice dancers has been designed which can also avoids the damage caused by overtraining. Therefore, this study not only opens a broad prospect of sports monitoring applications in the field of ice and snow sports but also provides important references for achieving sustainable development of smart sports.
The transparent PTFE has been purchased from the Taizhou Huafu Plastics Industry Co., LTD. (Taizhou, China). PDMS solution and hardener were purchased from Tianjin Youheng Electronic Technology Co., LTD. (Tianjin, China). The magnet wire was sourced from Wuhu Erite Electromechanical Equipment Co. (Wuhu, Anhui, China). The double-conductive aluminum foil tape was a purchase from Taobao.
To make the PDMS support layer: Firstly, mix the PDMS solution and hardener at a ratio of 10:1 by weight and stir until well combined. Secondly, pour the mixed solution into the mold and heat it in an oven at 85 °C for 15 min. Finally, remove the cured PDMS from the mold and trim it to obtain the PDMS support layer.
EM Preparation: buy ostrich eggs from Taobao and make a small hole in the top. After handled the liquid, remove the eggshell with tweezers and extract membrane. After cleaed with DI water, cut the eggshell membrane and dry it in an oven at 85 °C.
Preparation of EM-TENG sensors: Initially, the PDMS support layer was cut to size, followed by the cut of the eggshell membrane, PTFE membrane and double conductive aluminum foil to match. Subsequently, the eggshell membrane and PTFE membrane were affixed to the double conductive aluminum foil to create two friction layers for the TENG. Furthermore, in the construction of the device, PTFE was employed as the negative layer, flexible PDMS served as an intermediate support layer for facilitated contact and separation between the two friction layers and eggshell was utilized as the positive layer. The three layers were arranged in a stacked configuration and affixed with tape at both extremities. Subsequently, the entire assembly was encased in PDMS to create a fully integrated device measured 7.0 cm in length, 4.0 cm in width and 0.3 cm in height.
To simulate joint movement, the EM-TENG has been attached to a stepper motor. The stepper motor’s asynchronous pitch and frequency were adjusted to test EM-TENG performance. Waveform signals were generated by EM-TENG and recorded by oscilloscope (Sto1102c, Shenzhen, China).
A personalized data monitoring and analysis platform has been built to monitor ice dance land training. The EM-TENG can record the movement data of the ice dancers in real time and to transmit it wirelessly to the computer at the top. Coaches can use EM-TENG data to improve athletes’ technique, create personalized training plans and address training issues. Figure 1A demonstrates that the EM-TENG can be attached to the knee and ankle joints to track changes in joint motion of ice dance athletes during land and ice training, with data transmitted wirelessly via Bluetooth for real-time monitoring. Figure 1B illustrates the process in which the biodegradable eggshell membrane was washed with DI water, dried in an oven at 85 °C for 15 min and then cut to the required dimensions to produce the positive friction material for the TENG. The eggshell membrane’s substantial specific surface area and porous structure, coupled with its inert nature that prevents interference with other substances, render it an effective carrier for the development of biosensors. Eggshell membranes are not only thin (100 µm) and rich in micro fibrous structure, but also have unique properties such as elasticity, thermal stability, chemical stability and hydrophilic surface that make them highly desirable triboelectrical materials. Figures 1C,D show the SEM images of eggshell membrane and ostrich eggshell membrane, respectively. Compared with the eggshell membrane, ostrich eggshell membrane has a richer surface fiber structure and higher surface roughness. Supplementary Figure S1 shows the voltage output of four eggshell membranes (egg, duck egg, goose egg, ostrich egg) as positive friction layers, and it is obvious that the ostrich eggshell membrane outputs the largest voltage. Additionally, this technology has the capability to provide power to portable electronic devices and facilitate self-powered data collection during ice-dancing routines. The collected data can then be analyzed through wireless transmission and utilized to generate visual images, as depicted in Figure 1E. The EM-TENG can be easily formed on demand, demonstrating its advantages in cost-effective and industrial friendly manufacturing. In addition, using EM to make sensors instead of synthetic polymers can reduce chemical waste and develop high-value products using waste agricultural by-products. Our work proposes a biodegradable EM-TENG that provides a holistic environmental design concept for self-powered wearable electronics and offers the prospect of a wide range of applications for sustainable energy systems.
Figure 1. EM-TENG application scenarios and preparation process. (A) The use of EM-TENG in ice dance training on land and on ice. (B) The preparation process of the EM-TENG friction layer. (C) SEM images of eggshell membrane. (D) SEM images of ostrich eggshell membrane. (E) Intelligent motion monitoring application of EM-TENG.
Figure 2A illustrates the manufacturing process of the EM-TENG, which begins with cleaned the eggshell membrane using DI water and dried it in an 85 °C oven. Subsequently, a PDMS solution and curing agent are combined in a 10:1 ratio by weight, allowed to stand to eliminate air bubbles and then cast. A support layer was then obtained by dried in an oven at 85 °C. Finally, a sandwich structure was created by layering the upper eggshell membrane, intermediate PDMS support layer, lower polytetrafluoroethylene (PTFE) membrane and conductive aluminum foil adhesive on top and bottom. The final step involves pouring PDMS over the entire Sensor to prevent sweating. Figure 2B shows the working mechanism of EM-TENG. The diagram (State I) depicts the initial state in which the upper and lower dielectric friction layers are adhered without the presence of external forces, suggesting the absence of charge and electrical neutrality in the electrical output. Upon application to the surface of the EM-TENG, the eggshell membrane (EM) and polytetrafluoroethylene (PTFE) undergo a gradual separation process, resulting in the generation of equal and opposite induced charges on the surface of the friction layer. The polytetrafluoroethylene (PTFE) layer exhibits a tendency to lose electrons, whereas the eggshell membrane (EM) layer demonstrates a propensity to gain electrons, resulting in the transfer of electrons from the PTFE surface to the eggshell membrane (EM) layer (state II). Upon complete removal of the external force, the process of charge transfer and electron flow ceases (state III). When the EM-TENG undergoes compression once more, the proximity of the upper and lower friction layers results in the generation of a reversed internal electric field, facilitating the transfer of electrons from the electrodes of the eggshell membrane (EM) layer to those of the polytetrafluoroethylene (PTFE) layer (state IV). When the upper and lower friction layers are in full contact, the cycle is complete. A new cycle is started by releasing and compressing the EM-TENG. The corresponding simulation of the potential distribution by COMSOL for four different states is shown in Figure 2C. The EM-TENG is well suited to various motion monitoring applications due to its unique operating principle and excellent output voltage performance.
Figure 2. Preparation and operational mechanisms of EM-TENG. (A) Fabrication of the EM-TENG. (B) The principle of operation of the EM-TENG is a schematic diagram. (C) The operating principle of the EM-TENG has been simulated by means of COMSOL software.
In its role as a motion monitoring sensor, EM-TENG must ensure its capability to cater to diverse movement requirements. Therefore, preliminary electrical performance testing has been conducted on EM-TENG before it is put into practical use. Figure 3A displays the output voltage of EM-TENG under identical bending angles and forces, but at varying frequencies. At low frequencies (1 Hz, 2 Hz, 3 Hz and 4 Hz), the outputting voltage of EM-TENG remains consistent at approximately 36 V. The sustained output voltage is since at lower frequencies, there is no accumulation of triboelectricity. This finding also serves to illustrate the stability of the EM-TENG in monitoring low-frequency motion. Ice dancing requires a motion monitor with stable performance and fast joint angle changes and EM-TENG can meet ice dancing monitoring requirements. Figure 3B shows the EM-TENG response at different frequencies calculated using the following equation:
Figure 3. EM-TENG electrical output performance. (A) Output voltage of EM-TENG at different frequencies. (B) The voltage response of the EM-TENG has been measured at different frequencies. (C) The relation between the angle of the EM-TENG at the same frequency and output voltage. (D) A linear relationship exists between the output voltage of the EM-TENG and the bending angle at the same frequency. (E) Output voltage of the EM-TENG under different temperature conditions. (F) The reaction time of EM-TENG is 53 ms. (G) The voltage and power of the EM-TENG were measured at different loads. (H) EM-TENG charges 1 μF, 3.3 μF, 4.7 μF and 10 μF capacitors. (I) Stability tested of EM-TENG.
In the formula, V0 and Vi are output voltages at 1 Hz and another frequency. When the frequency is 1 Hz, 2 Hz, 3 Hz and 4 Hz, the frequency response of EM-TENG is 0%, 0.05%, 0.01% and 0.02%, respectively. The data suggest that EM-TENG is a stable method for monitoring human motion. It is capable of sensitively and accurately monitoring rapid changes in joint movement and angles during ice dance competitions. A stepper motor was used to simulate changes in joint angle to measure the voltage output of the EM-TENG at different bending angles, as shown in Supplementary Figure S2 This means that it is possible to set an absolute movement value for each step, which is then used to calculate its height. The bending angle of the sensor can be calculated using the cosine function (2cosβ). The voltage output of the EM-TENG at the same frequency but with different bending angles is shown in Figure 3C. When the bending angle is 62°, 94°, 128° and 148°, the triboelectric outputting voltage is 8.1 V, 7 V, 4.2 V and 3.6 V, respectively. The EM-TENG effectively measures changes in angles by monitoring output voltage, enabling coaches to monitor angle changes at individual joints in ice dancers’ bodies and devise tailored training regimens to enhance performance. Figure 3D shows the relationship between the bending angle of the EM-TENG and the output voltage, calculated using an equation.:
The study found significant correlation between EM-TENG angle and voltage, with a Pearson correlation coefficient of −0.98362. Figure 3E shows the outputting voltage of EM-TENG at different temperatures. It can be seen from the figure that the maximum outputting voltage at 25.8°C, 32°C, 36.1°C and 40.8 °C is 10.2 V, 11.5 V, 11.3 V and 11.3 V, respectively. Compared with the normal human temperature of 37°C, EM-TENG has no significant changes in its outputting voltage at different temperatures, indicating that this device has good stability in the face of temperature changes. Figure 3F illustrates the charge and discharge reaction time of the EM-TENG device, with a recorded reaction time of 53 milliseconds, suggesting a notable high-pressure sensing sensitivity of the EM-TENG. The comparison of outputting voltage, outputting power and outputting current of the EM-TENG is shown in Figure 3G. From Figure 3G, the output voltage will increase as the resistance of the load increases, while the output current will decrease as the resistance of the load increases. Additionally, it is noted that EM-TENG demonstrates a peak power output of 17.92 mW when subjected to a load resistance of 7 MΩ, suggesting that the most efficient resistance for EM-TENG operation is 7 MΩ. Figure 3H shows the charging conditions of the different capacitors of the EM-TENG. At a frequency of 4 Hz, the outputting voltages of the EM-TENG at 1 μF, 3.3 μF, 4.7 μF and 10 µF capacitors charged to 28 s are 4.88 V, 4.24 V, 2.96 V and 1.39 V, respectively. The EM-TENG has the capability to function as both a sensor and an energy harvester by transforming the mechanical energy generated by human movement into electrical energy. Figure 3I shows the results of the stability test conducted on the EM-TENG. Even after 2,800 test cycles, the EM-TENG remained stable, demonstrating its excellent fatigue resistance and ability to monitor ice dancers continuously.
To assess the degradability of the eggshell films, they were buried in soil at a depth of 10 cm and maintained at room temperature for a period of 40 days, with the soil being kept adequately moist. Observations of decomposition were conducted on the first, 10th, 30th and 40th days of the experiment. After 40 days, most of the eggshell films had degraded, as shown in Supplementary Figure S3.
EM-TENG has the capability to capture the mechanical energy generated by the movement of the human body and transform it into an electrical signal for providing feedback. To investigate the functionality of EM-TENG as a power source, we tested its practical application by using the harvested energy to charge various commercial electronic products. The pulse current output from the TENG is typically rectified and stored in energy storage devices such as batteries or capacitors, which then supply power to electronic devices. Figure 4A shows the equivalent circuit diagram of EM-TENG, which includes capacitors, rectifier bridges and loads. The rectifier bridge converts the alternating current generated by the EM-TENG as it moves into direct current, which is then stored in the capacitor. The electrical energy produced by the act of touched and pressed the EM-TENG has the capability to immediately illuminate 63 commercial green LEDs, as demonstrated in Figure 4B and Supplementary Video S1. This serves as evidence of the exceptional electrical performance of the EM-TENG and its ability to consistently supply power to the device. The actual charged and discharged conditions of the electronic watch and calculator (Supplementary Video S2) are shown in Figures 4C, D, respectively. Initially, the EM-TENG was tapped manually to charge the capacitor for a specified duration, followed by the activation of the power switch to provide power to both the calculator and electronic watch. Subsequently, successful illumination of the electronic calculator and watch was observed during the experimental trial, as depicted in Figures 4C, D. These practices have demonstrated that EM-TENG exhibits significant potential for use in sustainable clean energy applications.
Figure 4. EM-TENG performance test. (A) Equivalent circuit diagram for charged and discharged in EM-TENG. (B) EM-TENG has the capability to illuminate 63 green LEDs and is utilized as a power source. (C) EM-TENG charges the electric watch. (D) Charged the calculator.
Ice Dance is a performance of dancing steps and sliding movements on the ice with the rhythm of music. The imitation of the movement on land is an essential basic method of practice for the ice dance. A solid foundation for competitive performance and improved fitness is achieved through proper and effective land training. In the training process, the land training movements designed based on the technical characteristics of ice dance can deepen and integrate the comprehensive ability of ice dancer athletes. For example, conducting basic step, Mohawk step and twist step imitation exercises on land. In this work, our focus is to monitor the training of these ice dance step exercises on land. The voltage output of the knee and ankle joints of two athletes during basic step exercises is depicted in Figure 5A. The smoothness of the curve correlates with improved stability in the knee and ankle joints. Athlete 1 exhibits a smoother voltage output curve compared to Athlete 2, suggesting superior knee and ankle stability in Athlete 1. Under high-speed ice dance movements, the stability of the knee and ankle joints ensures that the body is in a low center of gravity state, which helps to reduce wind resistance during movement. Figure 5B shows knee joint angle variation for two athletes in mohawk stride training. By comparing the two, the outputting voltage of Athlete 1 is more stable, while the outputting voltage of Athlete 2 is more fluctuating and unstable. The reason for this is that before and after the mohawk, the knee and ankle of the gliding foot must be bent and pushed downwards, requiring high stability of the knee and ankle. EM-TENG’s simulation training is a crucial component in sports training, particularly in assisting ice skaters with the development of their technical skills. By monitoring movement signals, athletes can receive guidance in practicing the mohawk and progress from generalization to automatization in their skill execution. Ensure that they have better stability in competitions to achieve higher athletic results. Twist step is a continuous twisting movement that requires sufficient speed to complete and sufficient sliding speed will make it easier for athletes to perform the twist step. The fluctuations in output voltage of the knee joint during the twist step of the athletes are illustrated in Figure 5C. Athlete 1 exhibits a notably more consistent output voltage compared to Athlete 2, as depicted in Figure 5C. This is because the twist step is completed through very precise lifting and rapid turning of the foot, with very small and delicate movements. The unstable outputting voltage of Athlete 2 may be due to the inability to control their own speed, resulting in unstable twisting. It is important to watch the center of gravity and avoid swaying back and forth. Therefore, using EM-TENG can help athletes find a suitable speed and rhythm to complete ice dance movements better. The operation of the wireless intelligent land training system based on EM-TENG is shown in Figure 5D. The EM-TENG is used to monitor ice dancers during trained on the ice by attached to their ankle and knee joints. As the athlete performs exercises on land, the angle of the ankle and knee changes with the athlete’s range of motion and the EM-TENG device monitors and collects movement data during the exercise. The system then transmits the collected motion data via radio to a receiving device for processed in real time. Finally, the ice dancer’s movement performance can be scored in the form of a visualization interface. It also effectively helps athletes and coaches to understand sports performance in real-time, so that they can plan appropriate training plans.
Figure 5. Application of EM-TENG in ice dance Land training. (A) Outputting voltage diagram for basic steps trained. (B) Outputting voltage diagram of Mohawk steps training. (C) Outputting voltage diagram of twisting steps training. (D) The operating diagram of the ice dance land training assistance system.
In summary, a biodegradable eggshell membrane triboelectric nanogenerator was designed and fabricated for energy harvest and ice dance land training monitoring. The system uses eggshell diaphragms, PTFE film, PDMS support layer and aluminum foil electrodes to create an EM-TENG with a 300 V open circuit voltage. It also has a sensitive response time (53 ms). The flexibility, light weight and pliability of the material facilitate easy attachment to the ankles and knees of athletes. During the dancer’s movement, the EM-TENG undergoes deformation, leading to the generation of electrical power through the interaction of friction and electrostatic forces. Serving as a data transmitter, the EM-TENG wirelessly sends real-time data from ice dance training to a host computer for processing, enabling accurate recording and evaluation of technical movements, which are then displayed on a terminal device. This research will provide an effective method for the next-generation of portable and wearable applications that are environmentally friendly. Furthermore, digitalizing sports training is important for the development of sports.
The original contributions presented in the study are included in the article/Supplementary Material, further inquiries can be directed to the corresponding authors.
The studies involving humans were approved by Biology and Medical Ethics Committee of Northeastern University. The studies were conducted in accordance with the local legislation and institutional requirements. The participants provided their written informed consent to participate in this study.
BL: Conceptualization, Data curation, Validation, Writing–original draft, Writing–review and editing. ZX: Data curation, Validation, Writing–original draft. QF: Data curation, Writing–original draft. YW: Investigation, Writing–original draft. MZ: Investigation, Writing–original draft. ZL: Funding acquisition, Project administration, Supervision, Writing–original draft, Writing–review and editing. YM: Conceptualization, Data curation, Supervision, Validation, Writing–original draft, Writing–review and editing. SZ: Conceptualization, Data curation, Funding acquisition, Project administration, Supervision, Writing–original draft, Writing–review and editing.
The author(s) declare that financial support was received for the research, authorship, and/or publication of this article. Winter Sports Training monitoring technical services, 2020021300002.
The authors declare that the research was conducted in the absence of any commercial or financial relationships that could be construed as a potential conflict of interest.
All claims expressed in this article are solely those of the authors and do not necessarily represent those of their affiliated organizations, or those of the publisher, the editors and the reviewers. Any product that may be evaluated in this article, or claim that may be made by its manufacturer, is not guaranteed or endorsed by the publisher.
The Supplementary Material for this article can be found online at: https://www.frontiersin.org/articles/10.3389/fmats.2024.1400258/full#supplementary-material
Bagchi, B., Datta, P., Fernandez, C. S., Gupta, P., Jaufuraully, S., David, A. L. L., et al. (2023). Flexible triboelectric nanogenerators using transparent copper nanowire electrodes: energy harvesting, sensing human activities and material recognition. Mater. Horizons 10 (8), 3124–3134. doi:10.1039/d3mh00404j
Bruening, D. A., Reynolds, R. E., Adair, C. W., Zapalo, P., and Ridge, S. T. (2018). A sport-specific wearable jump monitor for figure skating. Plos One 13 (11), e0206162. doi:10.1371/journal.pone.0206162
Cao, X. L., Xiong, Y., Sun, J., Xie, X. Y., Sun, Q. J., and Wang, Z. L. (2023). Multidiscipline applications of triboelectric nanogenerators for the intelligent era of internet of things. Nano-Micro Lett. 15 (1), 14. doi:10.1007/s40820-022-00981-8
Cheng, Y. M., Wang, K., Xu, H., Li, T. A., Jin, Q. H., and Cui, D. X. (2021). Recent developments in sensors for wearable device applications. Anal. Bioanal. Chem. 413 (24), 6037–6057. doi:10.1007/s00216-021-03602-2
Chung, S., Lim, J., Noh, K. J., Kim, G., and Jeong, H. (2019). Sensor data acquisition and multimodal sensor fusion for human activity recognition using deep learning. Sensors 19 (7), 1716. doi:10.3390/s19071716
Coccia, M. (2020). How (Un)sustainable environments are related to the diffusion of COVID-19: the relation between coronavirus disease 2019, air pollution, wind resource and energy. Sustainability 12 (22), 9709. doi:10.3390/su12229709
Du, G. L., Wang, J. L., Liu, Y. H., Yuan, J. X., Liu, T., Cai, C. C., et al. (2023). Fabrication of advanced cellulosic triboelectric materials via dielectric modulation. Adv. Sci. 10 (15), e2206243. doi:10.1002/advs.202206243
Dudem, B., Dharmasena, R., Riaz, R., Vivekananthan, V., Wijayantha, K. G. U., Lugli, P., et al. (2022). Wearable triboelectric nanogenerator from waste materials for autonomous information transmission via morse code. Acs Appl. Mater. Interfaces 14 (4), 5328–5337. doi:10.1021/acsami.1c20984
Fan, F.-R., Tian, Z.-Q., and Wang, Z. L. (2021). Flexible triboelectric generator. Nano Energy 1, 328–334. doi:10.1016/j.nanoen.2012.01.004
Feng, X., Li, Q., and Wang, K. (2021). Waste plastic triboelectric nanogenerators using recycled plastic bags for power generation. Acs Appl. Mater Inter 13 (1), 400–410. doi:10.1021/acsami.0c16489
Gang, X. C., Guo, Z. H., Cong, Z. F., Wang, J., Chang, C. Y., Pan, C. X., et al. (2021). Textile triboelectric nanogenerators simultaneously harvesting multiple “High-Entropy” kinetic energies. Acs Appl. Mater. Interfaces 13 (17), 20145–20152. doi:10.1021/acsami.1c03250
Guo, Q.-Z., and Liu, C.-P. (2020). Derivation of analytical equations with experimental verification for working mechanism of triboelectric nanogenerators in contact-separation mode. Nano Energy 76, 104969. doi:10.1016/j.nanoen.2020.104969
Gurieff, N., Green, D., Koskinen, I., Lipson, M., Baldry, M., Maddocks, A., et al. (2020). Healthy power: reimagining hospitals as sustainable energy hubs. Sustainability 12 (20), 8554. doi:10.3390/su12208554
Hao, Y. T., Li, X. M., Chen, B. D., and Zhu, Z. Y. (2022). Marine monitoring based on triboelectric nanogenerator: ocean energy harvesting and sensing. Front. Mar. Sci. 9. doi:10.3389/fmars.2022.1038035
Haug, W. B., Drinkwater, E. J., Cicero, N. J., Barthell, J. A., and Chapman, D. W. (2019). The impact of dry-land sprint start training on the short track speed skating start. J. Strength Cond. Res. 33 (2), 544–548. doi:10.1519/jsc.0000000000001892
Kim, W. G., Kim, D. W., Tcho, I. W., Kim, J. K., Kim, M. S., and Choi, Y. K. (2021). Triboelectric nanogenerator: structure, mechanism, and applications. Acs Nano 15 (1), 258–287. doi:10.1021/acsnano.0c09803
Kovacs, E. J., Birmingham, T. B., Forwell, L., and Litchfield, R. B. (2004). Effect of training on postural control in figure skaters - a randomized controlled trial of neuromuscular versus basic off-ice training programs. Clin. J. Sport Med. 14 (4), 215–224. doi:10.1097/00042752-200407000-00004
Li, R., Wei, X., Xu, J., Chen, J., Li, B., Wu, Z., et al. (2021b). Smart wearable sensors based on triboelectric nanogenerator for personal healthcare monitoring. Micromachines 12 (4), 352. doi:10.3390/mi12040352
Li, Y. H., Yu, J. R., Wei, Y. C., Wang, Y. F., Feng, Z. Y., Cheng, L. Q., et al. (2023a). Recent progress in self-powered wireless sensors and systems based on TENG. Sensors-Basel 23 (3), 1329. doi:10.3390/s23031329
Li, Y. H., Zhao, Z. H., Gao, Y. K., Li, S. X., Zhou, L. L., Wang, J., et al. (2021a). Low-cost, environmentally friendly, and high-performance triboelectric nanogenerator based on a common waste material. Acs Appl. Mater Inter 13 (26), 30776–30784. doi:10.1021/acsami.1c09192
Li, Y. M., Chen, S. E., Yan, H., Jiang, H. W., Luo, J. J., Zhang, C., et al. (2023b). Biodegradable, transparent, and antibacterial alginate-based triboelectric nanogenerator for energy harvesting and tactile sensing. Chem. Eng. J. 468, 143572. doi:10.1016/j.cej.2023.143572
Li, Y. M., Liu, X., Ren, Z. W., Luo, J. J., Zhang, C., Cao, C. Y., et al. (2024). Marine biomaterial-based triboelectric nanogenerators: insights and applications. Nano Energy 119, 109046. doi:10.1016/j.nanoen.2023.109046
Liang, Q., Zhang, Q., Yan, X., Liao, X., Han, L., Yi, F., et al. (2017). Recyclable and green triboelectric nanogenerator. Adv. Mater. 29 (5). doi:10.1002/adma.201604961
Linnamo, V. (2023). Sensor technology for sports monitoring. Sensors 23 (2), 572. doi:10.3390/s23020572
Liu, R., Dong, X., Zhang, P., Zhang, Y., Wang, X., and Gao, Y. (2020). Study on the sustainable development of an arid basin based on the coupling process of ecosystem health and human wellbeing under land use change-A case study in the manas river basin, xinjiang, China. Sustainability 12 (3), 1201. doi:10.3390/su12031201
Lu, Z., Jia, C. J., Yang, X., Zhu, Y. S., Sun, F. X., Zhao, T. M., et al. (2022). A flexible TENG based on micro-structure film for speed skating techniques monitoring and biomechanical energy harvesting. Nanomaterials 12 (9), 1576. doi:10.3390/nano12091576
Lu, Z., Zhu, Y. S., Jia, C. J., Zhao, T. M., Bian, M. Y., Jia, C. F., et al. (2021). A self-powered portable flexible sensor of monitoring speed skating techniques. Biosensors-Basel. 11 (4), 108. doi:10.3390/BIOS11040108
Luo, J., Gao, W., and Wang, Z. L. (2021a). The triboelectric nanogenerator as an innovative technology toward intelligent sports. Adv. Mater. 33 (17), e2004178. doi:10.1002/adma.202004178
Luo, Z. B., Duan, J. P., Xu, H. C., Wang, Y. Y., Liu, J. W., Yao, X., et al. (2021b). Flexible capacitive pressure sensor based on an embedded rib fabric with a bionic sloping petal structure. Ieee Sensors J. 21 (18), 20119–20128. doi:10.1109/JSEN.2021.3097349
Mi, Y. J., Lu, Y., Shi, Y. L., Zhao, Z. Q., Wang, X. Q., Meng, J. J., et al. (2023). Biodegradable polymers in triboelectric nanogenerators. Polymers-Basel 15 (1), 222. doi:10.3390/polym15010222
Panfili, A., Spanò, A., and Cortesi, A. (2022). A wearable system for jump detection in inline figure skating. Sensors 22 (4), 1650. doi:10.3390/s22041650
Pradel, K. C., and Fukata, N. (2021). Systematic optimization of triboelectric nanogenerator performance through surface micropatterning. Nano Energy 83, 105856. doi:10.1016/j.nanoen.2021.105856
Qu, Z. G., Wang, X. P., Huang, M. K., Chen, C. X., An, Y., Yin, W. L., et al. (2023). An eccentric-structured hybrid triboelectric-electromagnetic nanogenerator for low-frequency mechanical energy harvesting. Nano Energy 107, 108094. doi:10.1016/j.nanoen.2022.108094
Shao, Y. Z., Du, G. L., Luo, B., Liu, T., Zhao, J. M., Zhang, S., et al. (2024). A tough monolithic-integrated triboelectric bioplastic enabled by dynamic covalent chemistry. Adv. Mater 36, e2311993. doi:10.1002/adma.202311993
Shen, S., Xiao, X., Yin, J., and Chen, J. (2022). Self-powered smart gloves based on triboelectric nanogenerators. Small Methods 6 (10), e2200830. doi:10.1002/smtd.202200830
Su, Y. J., Chen, G. R., Chen, C. X., Gong, Q. C., Xie, G. Z., Yao, M. L., et al. (2021). Self-powered respiration monitoring enabled by a triboelectric nanogenerator. Adv. Mater. 33 (35), e2101262. doi:10.1002/adma.202101262
Sun, L. L., Lin, S. Q., Tang, W., Chen, X., and Wang, Z. L. (2020). Effect of redox atmosphere on contact electrification of polymers. Acs Nano 14, 17354–17364. doi:10.1021/acsnano.0c07480
Tomita, Y., Iizuka, T., Irisawa, K., and Imura, S. (2021). Detection of movement events of long-track speed skating using wearable inertial sensors. Sensors 21 (11), 3649. doi:10.3390/s21113649
Wang, C. F., Lu, L., Li, W., Shao, D. Y., Zhang, C. L., Lu, J., et al. (2022). Green-in-green biohybrids as transient biotriboelectric nanogenerators. iScience 25 (12), 105494. doi:10.1016/j.isci.2022.105494
Wen, H., Yang, X., Huang, R., Zheng, D., Yuan, J., Hong, H., et al. (2023). Universal energy solution for triboelectric sensors toward the 5G era and internet of things. Adv. Sci. 10 (22), e2302009. doi:10.1002/advs.202302009
Wen, Z., Yang, Y., Sun, N., Li, G., Liu, Y., Chen, C., et al. (2018). A wrinkled PEDOT:PSS film based stretchable and transparent triboelectric nanogenerator for wearable energy harvesters and active motion sensors. Adv. Funct. Mater. 28 (37). doi:10.1002/adfm.201803684
Xie, L. P., Chen, P., Chen, S., Yu, K., and Sun, H. B. (2019). Low-cost and highly sensitive wearable sensor based on napkin for health monitoring. Sensors 19 (15), 3427. doi:10.3390/s19153427
Xie, Z., Zeng, Z., Wang, Y., Yang, W., Xu, Y., Lu, X., et al. (2020). Novel sweep-type triboelectric nanogenerator utilizing single freewheel for random triggering motion energy harvesting and driver habits monitoring. Nano Energy 68, 104360. doi:10.1016/j.nanoen.2019.104360
Yang, D., Ni, Y. F., Kong, X. X., Li, S. Y., Chen, X. Y., Zhang, L. Q., et al. (2021). Self-healing and elastic triboelectric nanogenerators for muscle motion monitoring and photothermal treatment. Acs Nano 15 (9), 14653–14661. doi:10.1021/acsnano.1c04384
Yi, F., Zhang, Z., Kang, Z., Liao, Q. L., and Zhang, Y. (2019). Recent advances in triboelectric nanogenerator-based health monitoring. Adv. Funct. Mater. 29 (41). doi:10.1002/adfm.201808849
Yin, X., Liu, D., Zhou, L. L., Li, X. Y., Zhang, C. L., Cheng, P., et al. (2019). Structure and dimension effects on the performance of layered triboelectric nanogenerators in contact-separation mode. Acs Nano 13 (1), 698–705. doi:10.1021/acsnano.8b07935
Zeng, Y., Xiang, H., Zheng, N., Cao, X., Wang, N., and Wang, Z. L. (2022). Flexible triboelectric nanogenerator for human motion tracking and gesture recognition. Nano Energy. 91, 106601. doi:10.1016/j.nanoen.2021.106601
Zhao, J., Wang, D., Zhang, F., Pan, J. S., Claesson, P., Larsson, R., et al. (2022a). Self-powered, long-durable, and highly selective oil-solid triboelectric nanogenerator for energy harvesting and intelligent monitoring. Nano-Micro Lett. 14 (1), 160. doi:10.1007/S40820-022-00903-8
Zhao, T. M., Fu, Y. M., Sun, C. X., Zhao, X. S., Jiao, C. X., Du, A., et al. (2022b). Wearable biosensors for real-time sweat analysis and body motion capture based on stretchable fiber-based triboelectric nanogenerators. Biosens. Bioelectron. 205, 114115. doi:10.1016/j.bios.2022.114115
Zhu, J. X., Zhu, M. L., Shi, Q. F., Wen, F., Liu, L., Dong, B. W., et al. (2020). Progress in TENG technology-A journey from energy harvesting to nanoenergy and nanosystem. Ecomat 2 (4). doi:10.1002/EOM2.12058
Zhu, Y., Sun, F., Jia, C., Zhao, T., and Mao, Y. (2022). A stretchable and self-healing hybrid nano-generator for human motion monitoring. Nanomaterials 12 (1), 104. doi:10.3390/nano12010104
Keywords: biodegradable, flexible, wearable devices, triboelectric nanogenerator, sports monitoring
Citation: Liu B, Xie Z, Feng Q, Wang Y, Zhang M, Lu Z, Mao Y and Zhang S (2024) Biodegradable flexible triboelectric nanogenerator for winter sports monitoring. Front. Mater. 11:1400258. doi: 10.3389/fmats.2024.1400258
Received: 13 March 2024; Accepted: 01 May 2024;
Published: 21 May 2024.
Edited by:
Vineet Kumar, Yeungnam University, Republic of KoreaReviewed by:
Yaokun Pang, Qingdao University, ChinaCopyright © 2024 Liu, Xie, Feng, Wang, Zhang, Lu, Mao and Zhang. This is an open-access article distributed under the terms of the Creative Commons Attribution License (CC BY). The use, distribution or reproduction in other forums is permitted, provided the original author(s) and the copyright owner(s) are credited and that the original publication in this journal is cited, in accordance with accepted academic practice. No use, distribution or reproduction is permitted which does not comply with these terms.
*Correspondence: Zhuo Lu, bHV6NTYwQG5lbnUuZWR1LmNu; Yupeng Mao, bWFveXVwZW5nQHBlLm5ldS5lZHUuY24=; Shouwei Zhang, emhhbmdzdzE3OEBuZW51LmVkdS5jbg==
Disclaimer: All claims expressed in this article are solely those of the authors and do not necessarily represent those of their affiliated organizations, or those of the publisher, the editors and the reviewers. Any product that may be evaluated in this article or claim that may be made by its manufacturer is not guaranteed or endorsed by the publisher.
Research integrity at Frontiers
Learn more about the work of our research integrity team to safeguard the quality of each article we publish.