- State Key Laboratory of Fire Science, Department of Safety Science and Engineering, University of Science and Technology of China, Hefei, China
In this work, anodization was utilized to synthesize highly ordered TiO2 nanotube arrays, and ZnO was successfully decorated onto the surface of as-prepared TiO2 nanotubes via a facile impregnation method. The gas sensing performance of a TiO2@ZnO composite was systematically studied. At a working temperature of 300°C, the response of TiO2@ZnO to 100 ppm H2 was ~340, 2.7 times larger than that of pristine TiO2. A power–law relationship between the response and H2 concentration was observed. In addition, the response time was significantly reduced by four times, and improved selectivity to H2 was achieved. The highly improved sensing performance of TiO2 nanotubes by ZnO decoration may be attributed to the enhanced oxygen adsorption and formation of n-n heterojunctions.
Introduction
Hydrogen is widely used as an important energy carrier and chemical material. Rapid and accurate detection of hydrogen is highly important due to its risky properties such as low minimum ignition energy (0.017 mJ) and high heat of combustion (142 kJ/g H2) (Silva et al., 2012). Among the various types of hydrogen sensing technology (Hübert et al., 2011), the resistance-based semiconductor gas sensor has attracted considerable attention due to its high sensitivity, short response time, and long-term stability. On the one hand, using various nanostructures of large surface area, such as nanofibers, nanowires, and nanotubes, has been well demonstrated to favor high gas sensing performance (Li et al., 2015a; Zhang et al., 2016; Chen and Yi, 2018). On the other hand, modification of the materials' surface with noble metals and/or oxides is another simple method to improve the gas sensor performance (Miller et al., 2014).
Among various n-type semiconductors, TiO2 has been widely studied for gas sensing (Zhao et al., 2015; Wang et al., 2017). A TiO2 ordered nanotube was proved to have exceptionally high response to hydrogen owing to its unique hierarchical morphology (Varghese et al., 2003). Further improvement of its gas sensing performance was mainly achieved through (1) optimizing the microstructural parameters of the nanotubes such as pore diameter and tube length (Mor et al., 2006) and (2) decorating the surface with noble metals such as Pt and Pd as a catalyst (Joo et al., 2010; Şennik et al., 2010; Xiong et al., 2016). Recently, it was found that by decorating the TiO2 ordered nanotubes with nanoparticles of non-noble metal, i.e., SnO2, both the sensitivity and response/recovery speed can be significantly enhanced (Xun et al., 2018), which was ascribed to formation of heterojunctions. Similar heterojunction formation and its promoting effect may also occur when other gas sensing semiconductor oxides are employed as a modifier for TiO2, which is yet to be studied.
This work synthesized highly aligned TiO2 nanotubes by anodic oxidation and decorated the nanotubes with ZnO nanoparticles via an immersion–calcination method. The sensing performance of the nanotubes was systemically studied, and possible sensing mechanism was discussed.
Experimental Section
Synthesis
Chemical reagents were purchased from Sinopharm Chemical Reagent Co., Ltd, China. Ti foils were purchased from Tianjin Ida. Pretreatment of high-purity titanium foils (25-mm thickness, 10 × 20 mm) including cleaning and chemical polishing is similar to that of previous research. The anodization process of the Ti foil was conducted in a two-electrode configuration connected to the DC power supply (Querli, Shanghai) with a voltage of 30 V for 3 h, using electrolyte of ethylene glycol containing 0.3 wt% NF4F and 5 vol% DI water. The anodized titanium foil was cleaned with ethylene glycol and deionized water in sequence before it was annealed in ambient air at 450°C for 3 h to induce crystallization. For the preparation of TiO2@ZnO, 0.0652 g of zinc acetate [Zn(CH3COO)2·2H2O] was dissolved in 20 mL of ethanol as precursor. TiO2 nanotubes were immersed into the solution for 12 h before they were calcined at 450°C. Two similar samples were prepared for either composition, denoted as TiO2 and TiO2-2 for pristine TiO2 and as TiO2@ZnO and TiO2@ZnO-2 for TiO2@ZnO.
Sensor Test
The typical gas sensor fabrication process and measurement setup parameters can be found in other work (Xun et al., 2018). In brief, the sensor was attached to two electrodes using conductive paste (DAD-87, Shanghai Research Institute of Synthetic Resins) and placed in a testing apparatus with a ceramic heating plate. The temperature and gas-flow rate were controlled by a DC power supply and mass flow controller (CS200, Sevenstar Electronics, Beijing), respectively. Resistance variation was recorded by Keithley 6482. Sensor response was defined as S = Ra/Rg (or Rg/Ra in the case of NO2 as target gas), where Ra and Rg are the resistance of the sensor in air and test gas, respectively. The response (recovery) time was the time that the resistance variation reached 90% of the total value after introduction (removal) of the target gas.
Characterization
Crystal structure was analyzed by powder X-ray diffraction (XRD, TTR III) using Cu Kα radiation. The morphologies and microstructure of the nanotubes were investigated by scanning electron microscopy (FE-SEM, SU8220) equipped with an energy-dispersive spectrometer (EDS) and transmission electron microscopy (TEM, JEM-2011). X-ray photoelectron spectroscopy (XPS) was performed on an ESCLAB 250 spectrometer using Al Kα as an excitation source and C1s binding energy at 284.8 eV as energy reference.
Results and Discussions
Material Analysis
Figure 1 shows the XRD patterns of the as-prepared samples. For the pristine TiO2 sample, all the peaks can be indexed to the anatase structure of titanium dioxide (JCPDS 01-089-4921) and Ti (JCPDS 044-1294). The XRD pattern of the as-synthesized TiO2@ZnO sample was similar to that of the pristine sample. The diffraction peak of zinc oxide was not observed, which may be due to the low loading amount of zinc oxide.
To illustrate the composition and chemical states of the elements in the samples, XPS studies were carried out, and results were presented in Figure 2. Ti and O were present in all the samples, and Zn was detected for the impregnated one (Figure 2A). No impurity element other than carbon contamination was observed. As shown in Figure 2B, two peaks at a binding energy of ~458.8 and ~464.6 eV could be attributed to Ti 2p1/2 and Ti 2p3/2, respectively, indicating that the Ti element was present in the form of Ti4+. The binding energy for both Ti 2p1/2 and Ti 2p3/2 remained almost invariant after ZnO decoration. Figure 2C demonstrates two peaks at a binding energy of ~1,045.3 and ~1,022.2 eV, corresponding to Zn 2p1/2 and Zn 2p3/2, respectively, implying the +2 oxidation state of Zn in the composite. Besides, the Zn/(Ti + Zn) ratio at the surface of the TiO2 nanotube was confirmed to be 25.2 at% by XPS analysis. The O 1s peak for both samples can be deconvoluted into two peaks at a binding energy of ~529.9 and ~531.4 eV, which corresponded to lattice oxygen (Olat) and absorbed oxygen (Oabs), respectively. The Oabs/(Oabs + Olat) ratio of TiO2@ZnO composites (22.0%) was distinctly larger than that of pristine TiO2 (19.5%), suggesting that ZnO decoration generated more surface adsorption sites.
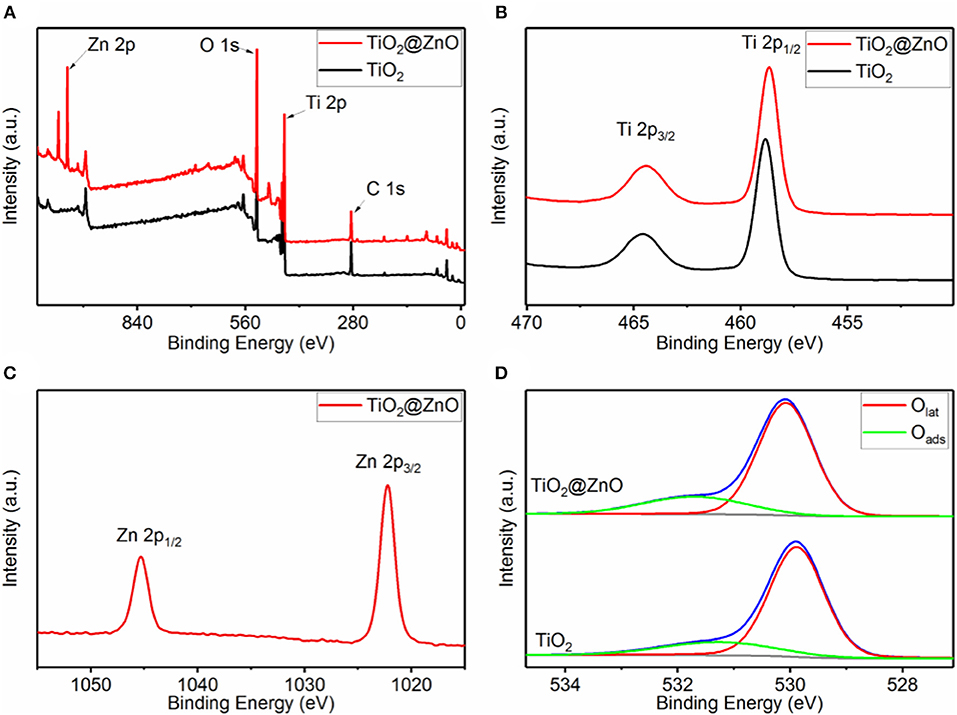
Figure 2. X-ray photoelectron spectroscopy (XPS) spectra of pristine TiO2 and TiO2@ZnO nanotubes. (A) Survey spectrum, (B) Ti 2p, (C) Zn 2p, and (D) O 1s.
Figures 3A–D show the SEM micrographs of the as-prepared ordered arrays of pristine TiO2 and TiO2@ZnO samples. The pore diameter of the pristine TiO2 nanotubes is ~80 nm with a wall thickness of ~10 nm, and the length is ~2.4 μm. There was no evident difference between the TiO2@ZnO sample and the pristine one, indicating that the impregnation treatment did not affect the morphology of the nanotubes. EDS elemental mapping analysis was performed on TiO2@ZnO as showed in Figures 3E–G, which revealed a homogeneous distribution of the Zn elements throughout the surface (Figure 3G). It can also be noticed that the Ti signal in the hollow part of the nanotube is more intense than that around the tube wall (Figure 3F), which can be ascribed to the unreacted Ti foil at the bottom. EDX analysis confirmed the coexistence of Ti, Zn, and O elements for the composite with a Zn/(Ti + Zn) ratio of 3.5 at%.
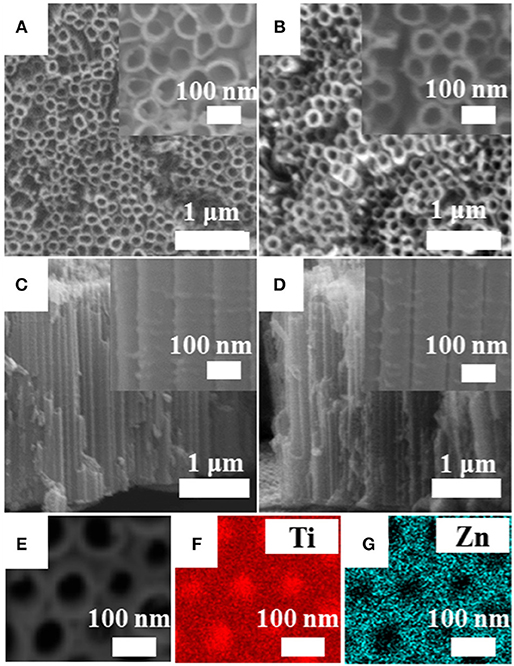
Figure 3. Scanning electron microscopy (SEM) images of (A,B) surface and (C,D) cross-sectional view of (A,C) pristine TiO2 and (B,D) TiO2@ZnO nanotubes. Energy-dispersive spectrometer (EDS) elemental images of TiO2@ZnO with (E) SEM images, (F) Ti, and (G) Zn.
More in-depth microstructural analysis was performed on both samples with TEM (Figure 4). The pristine TiO2 nanotubes presented a clear view (Figure 4A), while some extra nanoparticles in the size of 5–10 nm were observed with an even distribution throughout the ZnO-decorated nanotubes (Figure 4B). Selected area electron diffraction (SAED) revealed some extra diffraction pots in addition to the main diffraction rings of TiO2 (Figure 4C), corresponding to the (100) and (002) planes of ZnO (JCPDS 36-4521). These results indicated that TiO2 nanotubes modified with ZnO nanoparticles were successfully obtained.
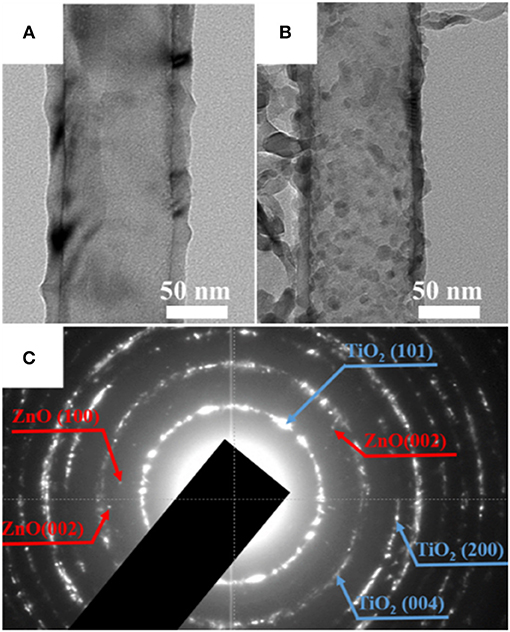
Figure 4. Transmission electron microscopy (TEM) images of (A) pristine TiO2 nanotubes and (B) TiO2@ZnO composites. (C) SAED pattern of TiO2@ZnO.
Gas Sensing Properties
ZnO decoration was found to significantly increase the resistance in air of the TiO2 nanotubes, corresponding to an increase in Ra from ~40 kΩ to ~6 MΩ at 300°C. Figure 5A compares the response of the sensors to 100 ppm H2 at different temperatures. The pristine TiO2 sensor exhibited typical volcano-shape temperature dependence of response. The response at the working temperature of 300°C is 126, which is 3.2 times that of samples with a tube length of 3–4 μm and a diameter of ~100 nm under the same conditions (Xun et al., 2018). This indicates that the response can be increased by changing the morphology of nanotubes, i.e., reducing the diameter and length. A similar behavior of temperature dependence of response was observed, and the response was improved after the ZnO impregnation. At the optimum temperature of 350°C for both sensors, the response of the TiO2@ZnO composite to 100 ppm H2 was ~520, about 1.8 times higher than that of pristine TiO2.
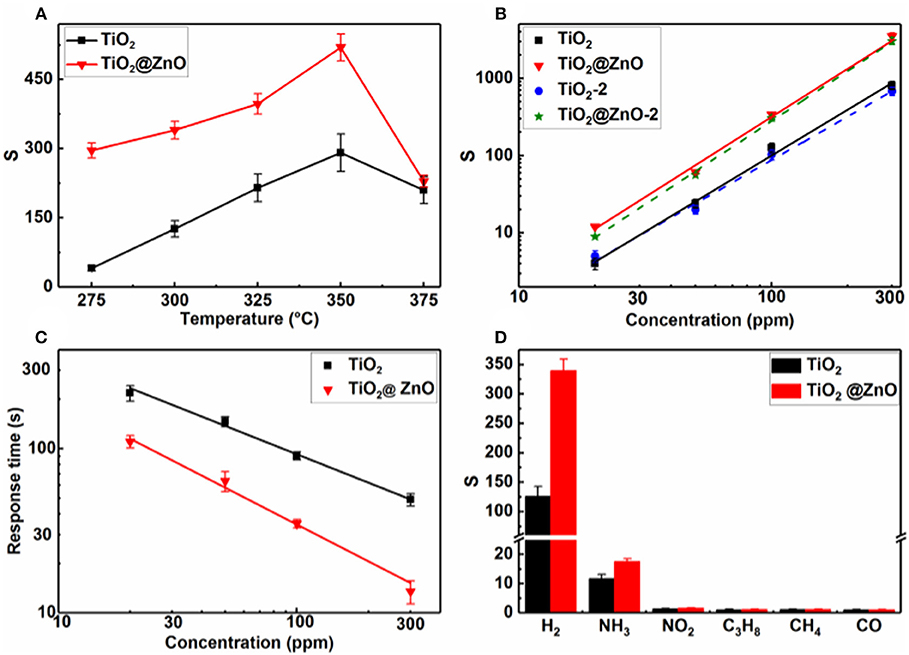
Figure 5. Response of pristine TiO2 and TiO2@ZnO composite sensors as a function of (A) temperature to 100-ppm H2 and (B) H2 concentration at 300°C; (C) response time of pristine TiO2 and TiO2@ZnO composite sensors vs. H2 concentration at 300°C; (D) response of pristine TiO2 and TiO2@ZnO composite sensors to 100-ppm various gases at 300°C.
Figure 5B shows that response increased with the increase in H2 concentration for both kinds of sensors. A linear relationship between the response and H2 concentration on the log–log scale was observed for all samples, which accords with the power–law theory proposed by Yamazoe and Shimanoe (2008). The response of the TiO2@ZnO composite was higher than that of the pristine one at all tested concentrations, and the enhancement was more pronounced at higher concentrations. Taking S = 1.2 as the detection threshold (Liu et al., 2017; Gao et al., 2019), the detection limit calculated for the TiO2@ZnO composite sensor was 7.5 ppm, which was smaller than that of the pristine TiO2 sensor (10.3 ppm). It can also be seen that the response of two different TiO2@ZnO sensors was very close to each other, indicating good consistency of the sensors.
Figure 5C shows that for both pristine TiO2 and TiO2@ZnO, the response time decreased as the concentration increased. A much faster response speed was observed for the latter sample. The response time of TiO2@ZnO is 22 s for 100 ppm H2 at 300°C, which is 4.2 times faster than that of the pristine sample. A power–law relationship was observed between the response time and the concentration, which may be accounted for by a nonlinear diffusion-reaction model (Xun et al., 2018). In contrast to the fast response speed, the recovery process was sluggish, typically with a recovery time over 2,000 s. Moreover, the recovery became slower while the concentration increased.
To illustrate the selectivity of the sensors, the response to 100-ppm various gases at 300°C was compared (Figure 5D). Because hydrogen is highly combustive and explosive, NH3, C3H8, NO2, CO, and CH4 were selected to examine the cross-sensitivity, which are very common interfering gases for fire detection. It can be seen that the pristine TiO2 showed some minor response to NH3 and insignificant response to the other interfering gases. The response to both H2 and NH3 increased while that to other gases remained after ZnO decoration. Nevertheless, the H2 response increased much more pronouncedly than the NH3 one. As a result, the selectivity, i.e., the response ratio of H2 over the most interfering gas, NH3, increased from 19.6 to 82.3 after ZnO loading. It should be noted that humidity is also a very common interference for semiconductor gas sensors. Although the humidity effect was not tested for the present samples, a significant humidity effect has been observed for other similar gas sensors based on TiO2 nanotubes in our group's research. Some strategies have been adopted to eliminate the humidity impact, e.g., by using a reference element for compensation (Zhan et al., 2007) or by decorating hydrophobic materials (Yao et al., 2016).
Gas Sensing Mechanism
The relatively high sensitivity of pristine TiO2 toward H2 can be attributed to the unique hierarchical morphology of the nanotubes. The small half-tube-wall thickness is comparable to that of the space charge layer, which favors hydrogen adsorption and its reaction with surface oxygen (Paulose et al., 2005; Hazra et al., 2015). The improved H2 response of TiO2@ZnO may be a result of the synergistic contribution from two effects. Firstly, the enhanced O2 adsorption due to ZnO decoration revealed by XPS in Figure 2 suggested generation of more active sites on the material surface, which is beneficial to the gas surface reactions. Secondly, n-n heterojunctions would be formed between the ZnO nanoparticles and TiO2 nanotubes. Previous research has reported that ZnO (3.37 eV) has a slightly larger band gap than that of TiO2 (3.2 eV); (Lin et al., 2013), and both conduction band energy and valance band energy of ZnO are higher than those of TiO2, leading to transfer of electrons from the conduction band of ZnO to that of TiO2 (Yang et al., 2009; Sarkar et al., 2014; Ng et al., 2018). Heterojunctions formed on the materials surface have been suggested to benefit the gas sensing performance via several routes, such as promoting gas reactions and regulating the conduction channel inside the materials (Lou et al., 2013; Li et al., 2015b; Gu et al., 2017), which can be applied to explain the enhancement of the composite sensor in this work.
Conclusions
Highly ordered TiO2 nanotube arrays were synthesized by anodic oxidation. Decoration of ZnO nanoparticles on the nanotubes was achieved by immersion and calcination. Gas sensing test results indicate that significantly larger response and better selectivity to H2 as well as shorter response time were achieved by ZnO loading. The TiO2@ZnO sensor exhibited a response as high as 520 toward 100-ppm H2 at 350°C. The excellent gas sensing performance of the composite may be attributed mainly to the highly ordered nanotube morphology and heterojunction formation at the interface of ZnO and TiO2.
Author Contributions
JY designed the experiments. AY and HX conducted the experiments and analyzed the results. JY and AY wrote the manuscript.
Funding
This work was supported by the National Natural Science Foundation of China (grant nos. 61871359 and U1432108).
Conflict of Interest Statement
The authors declare that the research was conducted in the absence of any commercial or financial relationships that could be construed as a potential conflict of interest.
References
Chen, D., and Yi, J. (2018). One-pot electrospinning and gas-sensing properties of LaMnO3 perovskite/SnO2 heterojunction nanofibers. J. Nanopart. Res. 20:65. doi: 10.1007/s11051-018-4158-x
Gao, H., Yu, Q., Chen, K., Sun, P., Liu, F., Yan, X., et al. (2019). Ultrasensitive gas sensor based on hollow tungsten trioxide–nickel oxide (WO3-NiO) nanoflowers for fast and selective xylene detection. J. Colloid Interface Sci. 535, 458–468. doi: 10.1016/j.jcis.2018.10.010
Gu, D., Li, X., Zhao, Y., and Wang, J. (2017). Enhanced NO2 sensing of SnO2/SnS2 heterojunction based sensor. Sens. Actuators B 244, 67–76. doi: 10.1016/j.snb.2016.12.125
Hazra, A., Bhowmik, B., Dutta, K., Chattopadhyay, P., and Bhattacharyya, P. (2015). Stoichiometry, length, and wall thickness optimization of TiO2 nanotube array for efficient alcohol sensing. ACS Appl. Mater. Interfaces 7, 9336–9348. doi: 10.1021/acsami.5b01785
Hübert, T., Boon-Brett, L., Black, G., and Banach, U. (2011). Hydrogen sensors—a review. Sens. Actuators B 157, 329–352. doi: 10.1016/j.snb.2011.04.070
Joo, S., Muto, I., and Hara, N. (2010). Hydrogen gas sensor using Pt-and Pd-added anodic TiO2 nanotube films. J. Electrochem. Soc. 157, J221–J226. doi: 10.1149/1.3374643
Li, T., Zeng, W., and Wang, Z. (2015a). Quasi-one-dimensional metal-oxide-based heterostructural gas-sensing materials: a review. Sens. Actuators B 221, 1570–1585. doi: 10.1016/j.snb.2015.08.003
Li, W., Ma, S., Li, Y., Yang, G., Mao, Y., Luo, J., et al. (2015b). Enhanced ethanol sensing performance of hollow ZnO–SnO2 core–shell nanofibers. Sens. Actuators B 211, 392–402. doi: 10.1016/j.snb.2015.01.090
Lin, L., Yang, Y., Men, L., Wang, X., He, D., Chai, Y., et al. (2013). A highly efficient TiO2@ ZnO n–p–n heterojunction nanorod photocatalyst. Nanoscale 5, 588–593. doi: 10.1039/c2nr33109h
Liu, J., Wang, T., Wang, B., Sun, P., Yang, Q., Liang, X., et al. (2017). Highly sensitive and low detection limit of ethanol gas sensor based on hollow ZnO/SnO2 spheres composite material. Sens. Actuators B 245, 551–559. doi: 10.1016/j.snb.2017.01.148
Lou, Z., Li, F., Deng, J., Wang, L., and Zhang, T. (2013). Branch-like hierarchical heterostructure (α-Fe2O3/TiO2): a novel sensing material for trimethylamine gas sensor. ACS Appl. Mater. Interfaces 5, 12310–12316. doi: 10.1021/am402532v
Miller, D. R., Akbar, S. A., and Morris, P. A. (2014). Nanoscale metal oxide-based heterojunctions for gas sensing: a review. Sens. Actuators B 204, 250–272. doi: 10.1016/j.snb.2014.07.074
Mor, G. K., Varghese, O. K., Paulose, M., Shankar, K., and Grimes, C. A. (2006). A review on highly ordered, vertically oriented TiO2 nanotube arrays: Fabrication, material properties, and solar energy applications. Sol. Energy Mater. Sol. Cells 90, 2011–2075. doi: 10.1016/j.solmat.2006.04.007
Ng, S., Kuberský, P., Krbal, M., Prikryl, J., Gärtnerová, V., Moravcová, D., et al. (2018). ZnO coated anodic 1D TiO2 nanotube layers: efficient photo-electrochemical and gas sensing heterojunction. Adv. Eng. Mater. 20:1700589. doi: 10.1002/adem.201700589
Paulose, M., Varghese, O. K., Mor, G. K., Grimes, C. A., and Ong, K. G. (2005). Unprecedented ultra-high hydrogen gas sensitivity in undoped titania nanotubes. Nanotechnology 17:398. doi: 10.1088/0957-4484/17/2/009
Sarkar, A., Singh, A. K., Khan, G. G., Sarkar, D., and Mandal, K. (2014). TiO2/ZnO core/shell nano-heterostructure arrays as photo-electrodes with enhanced visible light photoelectrochemical performance. RSC Adv. 4, 55629–55634. doi: 10.1039/c4ra09456e
Şennik, E., Colak, Z., Kılınç, N., and Ozturk, Z. Z. (2010). Synthesis of highly-ordered TiO2 nanotubes for a hydrogen sensor. Int. J. Hydrogen Energy 35, 4420–4427. doi: 10.1016/j.ijhydene.2010.01.100
Silva, S. F., Coelho, L., Frazão, O., Santos, J. L., and Malcata, F. X. (2012). A review of palladium-based fiber-optic sensors for molecular hydrogen detection. IEEE Sens. J. 12, 93–102. doi: 10.1109/jsen.2011.2138130
Varghese, O. K., Gong, D., Paulose, M., Ong, K. G., and Grimes, C. A. (2003). Hydrogen sensing using titania nanotubes. Sens. Actuators B 93, 338–344. doi: 10.1016/s0925-4005(03)00222-3
Wang, Y., Wu, T., Zhou, Y., Meng, C., Zhu, W., and Liu, L. (2017). TiO2-based nanoheterostructures for promoting gas sensitivity performance: designs, developments, and prospects. Sensors 17:1971. doi: 10.3390/s17091971
Xiong, Y., Chen, W., Li, Y., Cui, P., Guo, S., Chen, W., et al. (2016). Contrasting room-temperature hydrogen sensing capabilities of Pt-SnO2 and Pt-TiO2 composite nanoceramics. Nano Res. 9, 3528–3535. doi: 10.1007/s12274-016-1229-0
Xun, H., Zhang, Z., Yu, A., and Yi, J. (2018). Remarkably enhanced hydrogen sensing of highly-ordered SnO2-decorated TiO2 nanotubes. Sens. Actuators B 273, 983–990. doi: 10.1016/j.snb.2018.06.120
Yamazoe, N., and Shimanoe, K. (2008). Theory of power laws for semiconductor gas sensors. Sens. Actuators B 128, 566–573. doi: 10.1016/j.snb.2007.07.036
Yang, H. Y., Yu, S. F., Lau, S. P., Zhang, X., Sun, D. D., and Jun, G. (2009). Direct growth of ZnO nanocrystals onto the surface of porous TiO2 nanotube arrays for highly efficient and recyclable photocatalysts. Small 5, 2260–2264. doi: 10.1002/smll.200900724
Yao, M. S., Tang, W. X., Wang, G. E., Nath, B., and Xu, G. (2016). MOF thin film-coated metal oxide nanowire array: significantly improved chemiresistor sensor performance. Adv. Mater. 28, 5229–5234. doi: 10.1002/adma.201506457
Zhan, Z., Lu, J., Song, W., Jiang, D., and Xu, J. (2007). Highly selective ethanol In2O3-based gas sensor. Mater. Res. Bull. 42, 228–235. doi: 10.1016/j.materresbull.2006.06.006
Zhang, J., Liu, X., Neri, G., and Pinna, N. (2016). Nanostructured materials for room-temperature gas sensors. Adv. Mater. 28, 795–831. doi: 10.1002/adma.201503825
Keywords: hydrogen sensor, semiconductor, TiO2 nanotubes, heterojunction, anodic oxidation
Citation: Yu A, Xun H and Yi J (2019) Improving Hydrogen Sensing Performance of TiO2 Nanotube Arrays by ZnO Modification. Front. Mater. 6:70. doi: 10.3389/fmats.2019.00070
Received: 28 January 2019; Accepted: 01 April 2019;
Published: 30 April 2019.
Edited by:
Xiaogan Li, Dalian University of Technology (DUT), ChinaReviewed by:
Han Jin, Ningbo University, ChinaAlexander Gaskov, Lomonosov Moscow State University, Russia
Copyright © 2019 Yu, Xun and Yi. This is an open-access article distributed under the terms of the Creative Commons Attribution License (CC BY). The use, distribution or reproduction in other forums is permitted, provided the original author(s) and the copyright owner(s) are credited and that the original publication in this journal is cited, in accordance with accepted academic practice. No use, distribution or reproduction is permitted which does not comply with these terms.
*Correspondence: Jianxin Yi, eWp4QHVzdGMuZWR1LmNu