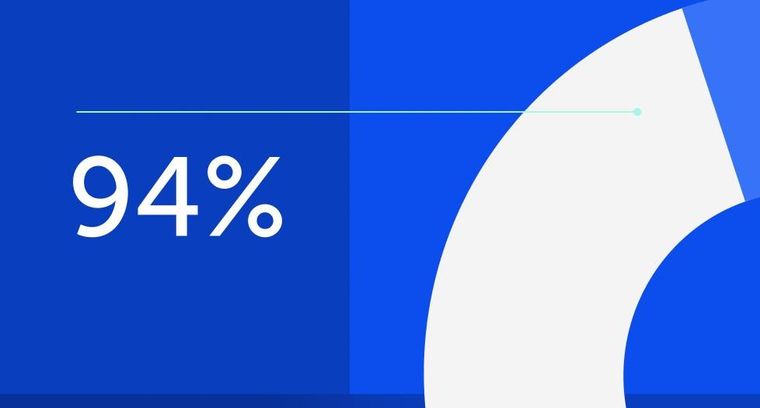
94% of researchers rate our articles as excellent or good
Learn more about the work of our research integrity team to safeguard the quality of each article we publish.
Find out more
ORIGINAL RESEARCH article
Front. Mater., 15 March 2022
Sec. Structural Materials
Volume 9 - 2022 | https://doi.org/10.3389/fmats.2022.864264
This article is part of the Research TopicAdvances in Design and Implementation of Cementitious Backfills (ADICB)View all 10 articles
Cemented paste backfill (CPB) has been increasingly utilized in mines for efficient mineral obtaining and regional ground support. To guarantee the work performance, the mechanical properties of CPB have long been a topic of study among researchers. But the research progress on the tensile strength of CPB is limited, mainly because of the lack of an appropriate test method due to the low tensile strength of CPB. Therefore, instead of the conventional splitting indirect tensile strength test method, a new direct tension test method, which utilizes the specifically designed compression to tension load converter (CTLC) and dog-bone-shaped specimen, has been applied to study the direct tensile properties of CPB. In this study, the direct tensile strength (DTS) of 47 CPB mix designs were measured using CTLC, and the unconfined compressive strength (UCS) of the corresponding mix design was also tested. The experimental results showed that the increase in the binder content, solid mass content, and curing period led to higher CPB direct tensile strength, and the DTS of CPB was most sensitive to the binder content. Furthermore, the influence of the slurry mass solid content on the tensile strength of CPB was not linear. The influence of the binder content became increasingly notable with the increase in the solid content, especially if the binder content exceeded 75%. The effect of the curing period was found to be rather marginal due to the decreasing amount of un-hydrated cementitious materials left with the increase of the curing period. Overall, the DTS generated using dog-bone specimens and the CTLC apparatus are valid for better mine backfill designs. Finally, a linear correlative between UCS and DTS with a formula in the form of σDT (DTS) = 0.171 σc (UCS) was obtained, and the correlation was sufficient for further calculation of DTS using measured UCS.
Mining with backfill, which utilizes solid mine wastes to manage and backfill mined-out stopes, has become a well-accepted method for efficient mineral obtaining and regional ground support (Grice, 1998; Belem and Benzaazoua, 2004; Jahanbakhshzadeh et al., 2017). The cemented paste backfill (CPB), a kind of the backfill method, has been increasingly utilized in mines across the world (Benzaazoua et al., 2008; Thompson et al., 2012). CPB is a composite backfill material usually consisting of mine tailings, water, and a hydraulic binder (Belem et al., 2000); ingredients will be first homogeneously mixed in a backfill plant; and the prepared slurries/paste will then be transported by pipelines and placed in underground stopes to backfill the voids. After days of binder hydration, the cemented backfill body can acquire adequate strength and provide a stable platform for mining the next stage (le Roux, et al., 2005; Rankine and Sivakugan, 2007). To guarantee the work performance, the mechanical properties of CPB have long been a study focus and application concerns by researchers and engineers, and many significant achievements have been worked out to better understand its properties, including the strength acquisition mechanism (Nasir and Fall, 2010) and failure features under uniaxial (Yilmaz et al., 2014) or triaxial compression (Klein and Simon, 2006; Fall et al., 2007). However, in terms of the tensile strength, due to the lack of an appropriate test method, the research progress has been limited. Although the tensile strength may only account for 20–30% of compressive strength (Komurlu et al., 2007; Fall et al., 2010), in many cases, such as in cut and fill mining or the large exposure of primary CPB during the ore mining of a secondary stope, the tensile strength is the key factor resulting in the failure of a cemented body (Yu, 1992). Indeed, it is necessary to precisely measure and analyze the tensile strength of CPB.
At present, the tensile strength test of CPB commonly takes the standards and methods of concrete or rocks as references (Huang et al., 2011; Chen et al., 2019; Zhou et al., 2021). Currently, the methods can be divided into three categories, namely, the direct tension test, splitting tensile test, and bending/flexural test (Raphael, 1984; Zheng et al., 2001; Erzar and Forquin, 2010). First, the direct tension test will have both ends of the specimen clamped firmly, and then axial tension will be produced by the loading instrument to damage it. The highest recorded stress value is the direct tensile strength (DTS). Hoek et al. (Hoek, 1964) proposed a dog-bone-shaped sample for the DTS test of rocks, as the extended parts of a sample ends can be conveniently clamped by designed wedge gadgets so that direct tension can be effectively applied. Another well-accepted way to clamp the sample is to glue the ends with the pressure arm of a press machine, which has been recommended as a standard DTS test method of a rock by ISRM in 1978 (Bieniawski and Hawkes, 1978), and many studies have been conducted by using this test (Rots and De Borst, 1989; Bolzan and Huber, 1993; Kim and Reda Taha, 2014). There are other direct test methods by changing the shapes of a specimen (Van Mier and Van Vliet, 2002), such as the dumbbell shape (Klanphumeesri., 2002) or “8” shape (Tamrakar et al., 2005). But the common problem of them is that the assembly of samples has to be properly centered; otherwise, the load on the specimen will be higher than the allowed tension value and results in a larger DTS (Cattaneo and Rosati, 1999; Nguyen et al., 2017; Alhussainy et al., 2019).
By contrast, the splitting and the bending tests are both indirect methods. For the splitting test, the “Brazilian test” is recommended and widely used as the standard method for calculating the indirect tensile strength (ITS) of concrete and rock (Carneiro, 1943; Fairhurst, 1964; Rocco et al., 1999). This method was developed separately by Caneiro (Cattaneo and Rosati, 1999) and Akazawa (Akazawa, 1943) in 1943. During the test, a cylindrical sample was placed horizontally in the press chamber, and the load was applied on the sample gradually until a vertical crack formed at the center of its cross section and finally penetrates the sample (Fairhurst, 1964). Then, based on the method theory, the applied force on the sample can be converted into ITS. However, some studies pointed out that the contact surfaces of the sample and press machine in this test are usually very narrow, making the tensile crack eccentric and leading to the overestimation of the real tensile strength (Lin and Wood, 2003; Fahimifar and Malekpour, 2012; Yong., 2005). For the bending test, a cubic sample was used, and after fixing the top and bottom, a concentrated load will be applied on the center part until the test piece bends and finally breaks. Then the highest applied force can be converted to the ITS (British Standard Institution, 1983; ASTM, 2012). This is also a convenient test method, but the conversion theory regarding the bending strength to ITS is based on many restricted assumptions, for example, the cross-sectional area of the test piece is assumed to be unchanged during the test, or the mechanical parameters of the sample such as Young’s modulus are all assumed to be unchanged (Wright and Garwood, 1952; Rüsch, 1960; Zweben et al., 1979). Therefore, studies have argued that the ITS obtained by this method would be bigger than the real tensile strength (Rüsch, 1960; Arezoumandi et al., 2015).
In terms of the tensile strength test of CPB, the splitting test is the most commonly used method (Yilmaz et al., 2009; Deng et al., 2017; Zhang and Zhang, 2020; Libos and Cui, 2021; Li et al., 2022). But many research studies assume that the crack failure begins when the tensile stress reaches a maximum point, and the splitting test is more suitable for brittle materials because they will not turn into plastic deformation before the tensile fracture occurs (Yu et al., 1997; Coviello et al., 2005; Lu et al., 2007; Li and Wong, 2013). Thus, as CPB is a kind of a relatively soft material (softer than rocks), the tensile strength of it may not be appropriately tested by using the splitting test. Moreover, for the bending test, as CPB usually has a larger porosity and smaller Young’s modulus than rocks and concrete, the cross-sectional area of the specimen will change sharply during the test, which, as explained above, cannot properly match the theoretically assumption.
Therefore, in the present study, the dog-bone-shaped CPB specimens were prepared so that direct tensile tests could be carried out. To optimize the sample clamping by avoiding stress concentration (Sedlacek and Halden, 1962), a compression to tension load converter (CTLC) was utilized (Pan and Grabinsky, 2021). The aim of this article was to verify and further use a new direct tensile strength test method to measure and empirically analyze the direct tensile properties of CPB.
To obtain dog-bone-shaped CPB specimens, a special mold has been designed for casting and curing of fresh CPB, which is shown in Figure 1A. It can be found that the mold consists of a baseplate, two enclosure plates, and two-sided baffles. The components are all made from polylactic acid (PLA), which is a kind of waterproof and bio-degradable material. Also, all components were separate and could be assembled by bolts. After assembling, some waterproof agents, such as silicone grease, need to be applied on the baseplate to increase the sealability of the mold, and then the fresh CPB can be cast into it. Thereafter, we used plastic films to cover the surface of the mold to prevent the evaporation of fresh CPB and put the mold into a curing room with steady temperature and humidity for designed days. After curing, only the baseplate and two-sided baffles need to be removed, and the left parts of the mold will be put with the cured CPB test piece together into the CTLC for next testing (see Figure 1B).
FIGURE 1. Design and dimensions of the curing mold: (A) dimensions of different parts of the mold and (B) the usage of the mold.
As aforementioned, during the DTS test of the dog-bone sample, the extended head and foot parts will be clamped firmly before testing. As the tensile strength of CPB is much lower than concrete or a rock, the pre-stress caused by clamping may lead to extra pressure on the sample, which will affect the test results. Therefore, a CTLC needs to be used (see Figure 2).
FIGURE 2. Compression to tension load converter: (A) different parts of the apparatus and (B) the assembly of the apparatus.
As shown in Figure 2A, this apparatus was assembled by using two brackets with the same dimensions, of which the length, width, and height are 158, 116, and 176.7 mm, respectively. Also, each bracket consists of a fixing plate, a loading plate, and four screw rods (diameter 10 mm). There was a clamping groove on a fixing plate, which can carry the extended parts of the specimen. The cured CPB samples need to be firstly installed into the two fixing plates. Thereafter, the screw rods are used to connect the loading plate. As the two brackets were separated in order not to be hinged, the specimen will hang down and be clamped by weight and then centered. The assembly process is shown in Figure 2B. In the test, this apparatus could be placed into any uniaxial loading press, and by applying pressure on the loading plate, the relative movement of the brackets could convert the compressive stress to direct tensile stress on the testing sample. Also, as the apparatus was made from an aluminum alloy, its weight was limited and would not apply much prestress on the sample, affecting the accuracy of the test.
The processed tailings used in this study were sourced from the Jinchanghe gold mine, Yunnan province, China. About 500 kg of wet tailings was obtained at the processing plant of the Jinchanghe gold mine and dried by the oven in the lab for cemented paste backfill (CPB) sample preparation.
Ordinary Portland cement (OPC 42.5) sourced from a local manufacturer was used as the cementitious binder for backfill sample preparation. Tap water was used for sample preparations.
Following sampling, the physical and chemical characterizations of the tailings were determined. Figure 3 illustrates the particle size distribution (PSD) of the used tailings determined by using a Malvern Mastersizer 2000 (Malvern Instruments Ltd., Malvern, United Kingdom). As shown in Figure 3, the tailings consisted of 75.51% fine particles less than 74 μm and 57.75% of fine fractions less than 38 μm which are suitable for cement paste backfill slurry preparation (Yılmaz et al., 2014; Zhao et al., 2020).
The chemical characterizations of the tailing material are shown in Table 1. The chemical composition of tailings mainly consisted of silicon dioxide (SiO2), ferric oxide (Fe2O3), and calcium oxide (CaO), with mass fractions of 34.96%, 25.28%, and 21.81%, respectively.
In our study, a total of 48 mix designs were examined, as shown in Table 2. The following naming system was used to represent specific mix designs:
where
The solid mass content and binder content is defined as follows:
where
A bakery mixer was used for CPB slurry preparation in this study. An appropriate number of tailings and binder were initially weighed and mixed in the dry form for 5 min according to the mix design shown in Table 2. Tap water was then added to achieve the designed solid mass content of CPB slurry and wet mixed for at least 5 min to obtain homogenized paste slurry. The resultant slurries were poured into I-shaped rectangular molds (as discussed in Section 2.1) and cylindrical molds (50 mm diameter, 100 mm length) to form rectangular dog-bone specimens and cylindrical specimens for the direct tensile (DT) and unconfined compression (UC) tests. During the pouring of paste-like slurries, the molds were filled with one-third length increments each time. After filling each layer, a small metal rod was used to tamp the mold approximately 25 times to remove entrapped air. The prepared CPB samples were then sealed and cured at a constant temperature in a humidity chamber maintained at 90% relative humidity and a temperature of 20 ± 0.5°C to premeasured curing periods.
Unconfined compression (UC) tests were conducted using cylindrical specimens at designed curing periods (7, 14, and 28 days) with a computer-controlled loading machine (HM-5030, Humboldt Mfg. Co., Elgin, IL, United States), as shown in Figure 4, in accordance with ASTM C39–18 (ASTM C39/C39M-18, 2018). The axial loading rate was fixed at 1mm/min, and the strains and corresponding stresses were recorded during the unconfined compressive test until the failure of the CPB sample. For the accuracy of test results, five cylindrical samples were prepared, and tests were conducted in triplicate for each mix at various curing periods. The average value was considered for further analyses.
Direct tensile (DT) tests were conducted using rectangular dog-bone specimens at designed curing periods (7, 14, and 28 days) with the same computer-controlled loading machine (HM-5030, Humboldt Mfg. Co., Elgin, IL, United States) as the UC test. A compression to tension load converter (CTLC), as shown in Figure 5 and illustrated in Section 2.2, was used to convert the axial load provided by the loading machine to a tensile load. The loading rate of the machine was fixed at 0.2 mm/s, and the strains and corresponding stresses were recorded during the unconfined compressive test until the failure of dog-bone-shaped specimens. Considering the weight of CTLC and dog-bone-shaped specimens, the direct tensile strength of each sample was calculated as follows:
where
For the accuracy of test results, five dog-bone-shaped specimens were prepared, and tests were conducted in triplicates for each mix at various curing periods. The average value was considered for further analyses.
In this study, the authors successfully measured the direct tensile strength of CPB rectangular dog-bone specimens of 47 mix designs. For the sample S68B9.1T7, the tensile strength was too low, and the sample could easily break under the gravity of the CTLC apparatus during installation; hence, the tensile strength could not be obtained.
Figure 6 illustrates the failure pattern of the five samples for the mix S70B20T28; the observed fracture surface is a flat and typically straight fracture line perpendicular to the direction of the tensile force. Among the five specimens of S70B20T28, samples 3, 4, and 5 failed at the center of dog-bone specimens, as Pan et al. (Pan, A. N., & Grabinsky, M. W., 2021) reported, where the smallest cross-section is at or near the midsection of the failure zone of all the specimens. However, samples 1 and 2 failed at the grip of the dog-bone sample. It might have been caused by the partial separation of the head plate and sample, which led to a reposition of both ends of the dog-bone sample and brought non-uniform tensile stress across the specimen. During our empirical study, all samples that failed such as this were not included for further analyses. The average value of three successful tests will be used as the direct tensile strength of each mix design.
Figure 7 illustrates the variations of the direct tensile strength (DTS, σDT) against the binder content with different solid mass contents at various curing periods. Overall, the increase in the binder content, solid mass content, and curing period led to a higher cemented paste backfill tensile strength.
FIGURE 7. Variations of direct tensile strength σDT against the binder content with different solid mass contents for samples cured for (A) 7 days, (B) 14 days, and (C) 28 days.
For instance, the sample S70B9.1T7, exhibited a direct tensile strength (σDT) of 115.45 kPa, while the inclusion of Bc = 11.1%, 14.3%, and 20%, at the same 7-day curing condition, resulted in σDT of 159.94, 219.57 and 408.66 kPa, respectively. For the same binder content of 11.1% and curing period of seven days, the tensile strength was 146.32, 159.94, 174.64, and 229.65 kPa for samples S68B11.1T7, S70B11.1T7, S72B11.1T7, and S75B11.1T7, respectively. Similarly, for any given binder content and solid mass content, the increase of the curing period promoted an increase of CPB tensile strength at a smaller step. For instance, the tensile strength for the sample S68B20T7 resulted in 408.66 kPa, and the tensile strength increased with values of 448.69 and 470.44 kPa for the same mix proportion curried at 14 and 28 days.
With the analysis of tensile strength results, even the increase in the binder content, solid mass content, and curing period promoted the resultant sample tensile strength. The tensile strength sensitivity to these three influencing factors was different. The CPB tensile strength was most sensitive for the binder content, as the tensile strength increased from 115.45 to 408.66 kPa, with a change of 9.1% binder content to 20% binder content, at the same 7-day curing period and 70% solid mass content. As shown in Figure 6, the increment rate of tensile strength dramatically increased while the solid mass content increased to 75%. Hence, the influence of the solid mass content of the slurry was not linear. The influence of Bc became increasingly notable with the increase in the solid content, especially for Bc after 75%. In a similar but adverse manner, with the same solid content and binder content, the increment of tensile strength was more pronounced for the 7–14-day curing period than the 14–28-day curing period. Moreover, the effect of the curing period was found to be rather marginal due to the decreasing amount of un-hydrated cementitious materials left with the increase in the curing period.
This study measured the unconfined compressive strength (UCS) of all 48 different mix designs using prepared CPB cylindrical samples. Uniaxial compressive strength (UCS) is one of the significant and common characteristics used to define the mechanical performance of CPB. For cement paste backfill, the tensile strength is difficult and expensive to measure; hence, tensile strength is often calculated based on UCS results (Nazir, et al., 2013). Figure 8 illustrates the direct tensile strength, σDT, variations against un-confined compressive strength, σc, for all CPB mix designs. Figure 8 is generated based on the successfully measured direct tensile strength of CPB rectangular dog-bone specimens of 47 different mix designs and the corresponding UCS value measures. As the direct tensile strength of the sample, S68B9.1T7 was too low, and the sample easily broke under the gravity of the CTLC apparatus during installation. Hence, the experimental data of mix design S68B9.1T7 was not included in Figure 8. A linear correlation was found between σc and σDT. The variations of σDT lied within the 0.149 σc < σDT < 0.208 σc domains (σDT and σc in kPa). In this case, a linear correlative formula in the form of σDT = 0.171 σc (R2 = 0.9304) was obtained. Deng et al. (2017) measured the UCS and splitting tensile strength of CPB samples by varying solid contents, binder content, and curing period. Their results indicated that the splitting tensile strength was about 10%–20% of UCS. Pan and Grabinsky et al. (2021) measured the direct tensile strength and UCS of CPB samples with different binder contents, and the direct tensile strength was about 16%–25% of CPB UCS. Hence, the direct tensile strength measured using dog-bone specimens and CTLC apparatus is correct, and the correlation is sufficient for further calculation of direct tensile strength using measured UCS. This linear correlation between σDT and σc is based on a large amount of experimental data, which can be used as an experience formula to guide engineers on the relationship between the direct tensile and unconfined compressive strength. However, the σDT and σc experience conversion formula still got its limitations. It is not applicable when the strength is deficient.
FIGURE 8. Variations of direct tensile strength σDT against unconfined compressive strength σc for all CPB mix designs.
This study presents an experimental study on the direct tensile strength measurement of the CPB dog-bone sample. A compression to tension load converter (CTLC) was used to transmit compression to tensile load on dog-bone samples to achieve direct tensile measurement using a standard loading machine. In this study, the direct tensile strength and unconfined compressive strength were successfully measured for a total of 47 CPB mix designs, and the following conclusions can be drawn:
First, by using dog-bone specimens and CTLC apparatus, the direct tensile strength of CPB could be measured effectively. This method is the first proven scientifically valid measurement method for tensile strength of CPB, which can provide a reliable means for obtaining the tensile mechanical parameters of mine backfill.
Second, the increase in the binder content, solid mass content, and curing period leads to higher cemented paste backfill tensile strength. The tensile strength sensitivity to these three influencing factors differs, and the CPB tensile strength is most sensitive to the binder content.
Third, the influence of slurries’ mass solid content on CPB tensile strength is not linear. The influence of binder content becomes increasingly notable with the increase of solid content, especially for binder content after 75%.
Fourth, the effect of the curing period was found to be rather marginal due to the decreasing amount of un-hydrated cementitious materials left with the increase of the curing period.
Fifth, a linear correlative experience conversion formula between UCS and DTS in the form of σDT = 0.171 σc (R2 = 0.9304) is first obtained. The correlation is sufficient for further calculation of DTS using measured UCS.
Finally, the σDT and σc experience conversion formulas still have their limitations; it is not applicable when the strength is deficient. The applicability of σDT and σc experience conversion formula on CPB samples prepared by various binders and aggregates should also be further developed.
The original contributions presented in the study are included in the article/Supplementary Material, further inquiries can be directed to the corresponding author.
LG and GT contributed significantly to the design of the study. LG, XP, and YZ developed the math analysis model and wrote the first draft of the manuscript. LG and GT provided constructive discussions and contributed to manuscript revision. GL and AP made important revisions to the manuscript. All authors agree to be accountable for the content of this work.
This research was supported by the National Key R&D Program of China (No. 2021YFC2900600).
The authors declare that the research was conducted in the absence of any commercial or financial relationships that could be construed as a potential conflict of interest.
All claims expressed in this article are solely those of the authors and do not necessarily represent those of their affiliated organizations, or those of the publisher, the editors, and the reviewers. Any product that may be evaluated in this article, or claim that may be made by its manufacturer, is not guaranteed or endorsed by the publisher.
Akazawa, T. (1943). New Test Method for Evaluating Internal Stress Due to Compression of concrete (The Splitting Tension Test)(part 1). J. Jpn. Soc. Civ Eng. 29, 777
Alhussainy, F., Hasan, H. A., Sheikh, M. N., and Hadi, M. N. (2019). A New Method for Direct Tensile Testing of concrete. J. Test. Eval. 47 (2), 704–718. doi:10.1520/jte20170067
American Society for Testing and Materials (Astm), (2012). Standard Test Method for Flexural Strength of Soil-Cement Using Simple Beam with Third-point Loading’ West Conshohocken. West Conshohocken, Pennsylvania: ASTM International.
Arezoumandi, M., Smith, A., Volz, J. S., and Khayat, K. H. (2015). An Experimental Study on Flexural Strength of Reinforced concrete Beams with 100% Recycled concrete Aggregate. Eng. Structures 88, 154. doi:10.1016/j.engstruct.2015.01.043
ASTM C39 C39M-18 (2018). Standard Test Method for Compressive Strength of Cylindrical Concrete Specimens. West Conshohocken, PA: ASTM International.
Belem, T., and Benzaazoua, M. (2004). An Overview on the Use of Paste Backfill Technology as a Ground Support Method in Cut-And-Fill Mines. In Proceedings of the 5th Int. Symp. On Ground Support in Mining and Underground Construction. Villaescusa & Potvin (eds.), pp. 637
Belem, T., Benzaazoua, M., and Bussière, B. (2000). “Mechanical Behaviour of Cemented Paste Backfill,” in Proc. Of 53rd Canadian Geotechnical Conference (Montreal, 373–380.
Benzaazoua, M., Bussière, B., Demers, I., Aubertin, M., Fried, É., and Blier, A. (2008). Integrated Mine Tailings Management by Combining Environmental Desulphurization and Cemented Paste Backfill: Application to Mine Doyon, Quebec, Canada. Minerals Eng. 21 (4), 330–340. doi:10.1016/j.mineng.2007.11.012
Bieniawski, Z. T., and Hawkes, I. (1978). Suggested Methods for Determining Tensile Strength of Rock Materials. Int. J. Rock Mech. Mining Sci. 15 (3), 99–103. doi:10.1016/0148-9062(78)90956-7
Bolzan, P. E., and Huber, G. A. (1993). Direct Tension Test Experiments (No. SHRP-A-641). Washington, DC: Strategic Highway Research ProgramNational Research Council.
British Standard Institution(Bs), (1983). -118 Method for Determination of Flexural Strength’. London: BSI
Carneiro, F. L. L. B. (1943). A New Method to Determine the Tensile Strength of concrete. Proc. 5th Meet. Braz. Assoc. Tech. Rules 3 (16), 126
Cattaneo, S., and Rosati, G. (1999). Effect of Different Boundary Conditions in Direct Tensile Tests: Experimental Results. Mag. Concrete Res. 51 (5), 365–374. doi:10.1680/macr.1999.51.5.365
Chen, X., Shi, X., Zhou, J., and Yu, Z. (2019). Influence of Polypropylene Fiber Reinforcement on Tensile Behavior and Failure Mode of Tailings Cemented Paste Backfill. IEEE Access 7, 69015–69026. doi:10.1109/access.2019.2919480
Coviello, A., Lagioia, R., and Nova, R. (2005). On the Measurement of the Tensile Strength of Soft Rocks. Rock Mech. Rock Engng. 38 (4), 251–273. doi:10.1007/s00603-005-0054-7
Deng, X., Zhang, J., Klein, B., Zhou, N., and Dewit, B. (2017). Experimental Characterization of the Influence of Solid Components on the Rheological and Mechanical Properties of Cemented Paste Backfill. Int. J. Mineral Process. 168, 116–125. doi:10.1016/j.minpro.2017.09.019
Erzar, B., and Forquin, P. (2010). An Experimental Method to Determine the Tensile Strength of concrete at High Rates of Strain. Exp. Mech. 50 (7), 941–955. doi:10.1007/s11340-009-9284-z
Fahimifar, A., and Malekpour, M. (2012). Experimental and Numerical Analysis of Indirect and Direct Tensile Strength Using Fracture Mechanics Concepts. Bull. Eng. Geol. Environ. 71 (2), 269–283. doi:10.1007/s10064-011-0402-7
Fairhurst, C. (1964). On the Validity of the 'Brazilian' Test for Brittle Materials. Int. J. Rock Mech. Mining Sci. Geomechanics Abstr. 1 (4), 535–546. doi:10.1016/0148-9062(64)90060-9
Fall, M., Belem, T., Samb, S., and Benzaazoua, M. (2007). Experimental Characterization of the Stress-Strain Behaviour of Cemented Paste Backfill in Compression. J. Mater. Sci. 42 (11), 3914–3922. doi:10.1007/s10853-006-0403-2
Fall, M., Célestin, J. C., Pokharel, M., and Touré, M. (2010). A Contribution to Understanding the Effects of Curing Temperature on the Mechanical Properties of Mine Cemented Tailings Backfill. Eng. Geology. 114 (3-4), 397–413. doi:10.1016/j.enggeo.2010.05.016
Grice, T. (1998). Underground Mining with Backfill. Brisbane, Nov: 2nd Annual Summit on Mine Tailings Disposal Systems, 24–25.
Huang, S., Xia, K., and Qiao, L. (2011). Dynamic Tests of Cemented Paste Backfill: Effects of Strain Rate, Curing Time, and Cement Content on Compressive Strength. J. Mater. Sci. 46 (15), 5165–5170. doi:10.1007/s10853-011-5449-0
Jahanbakhshzadeh, A., Aubertin, M., and Li, L. (2017). A New Analytical Solution for the Stress State in Inclined Backfilled Mine Stopes. Geotech Geol. Eng. 35 (3), 1151–1167. doi:10.1007/s10706-017-0171-6
Kim, J. J., and Reda Taha, M. (2014). Experimental and Numerical Evaluation of Direct Tension Test for Cylindrical concrete Specimens. Adv. Civil Eng. doi:10.1155/2014/156926
Klanphumeesri, S. (2002). Direct Tension Testing of Rock Specimens, Master’s Thesis. Nakhon Ratchasima, Thailand: Suranaree University of Technology.
Klein, K., and Simon, D. (2006). Effect of Specimen Composition on the Strength Development in Cemented Paste Backfill. Can. Geotech. J. 43 (3), 310–324. doi:10.1139/t06-005
Komurlu, E., Kesimal, A., and Demir, S. (2016). Experimental and Numerical Analyses on Determination of Indirect (Splitting) Tensile Strength of Cemented Paste Backfill Materials under Different Loading Apparatus. Geomech. Eng. 10 (6), 775–791. doi:10.12989/gae.2016.10.6.775
le Roux, K., Bawden, W. F., and Grabinsky, M. F. (2005). Field Properties of Cemented Paste Backfill at the Golden Giant Mine. Mining Techn. 114 (2), 65–80. doi:10.1179/037178405x44557
Li, D., and Wong, L. N. Y. (2013). The Brazilian Disc Test for Rock Mechanics Applications: Review and New Insights. Rock Mech. Rock Eng. 46 (2), 269–287. doi:10.1007/s00603-012-0257-7
Li, Z., Shi, X., and Chen, X. (2022). Effect of Rice Straw on Tensile Properties of Tailings Cemented Paste Backfill. Appl. Sci. 12 (1), 526. doi:10.3390/app12010526
Libos, I. L. S., and Cui, L. (2021). Time- and Temperature-Dependence of Compressive and Tensile Behaviors of Polypropylene Fiber-Reinforced Cemented Paste Backfill. Front. Struct. Civ. Eng. 15 (4), 1025–1037. doi:10.1007/s11709-021-0741-9
Lin, Z., and Wood, L. (2003). Concrete Uniaxial Tensile Strength and cylinder Splitting Test. J. Struct. Eng. 129 (5), 692–698. doi:10.1061/(asce)0733-9445(2003)129:5(692)
Lu, N., Wu, B., and Tan, C. P. (2007). Tensile Strength Characteristics of Unsaturated Sands. J. Geotech. Geoenviron. Eng. 133 (2), 144–154. doi:10.1061/(asce)1090-0241(2007)133:2(144)
Nasir, O., and Fall, M. (2010). Coupling Binder Hydration, Temperature and Compressive Strength Development of Underground Cemented Paste Backfill at Early Ages. Tunnelling Underground Space Techn. 25 (1), 9–20. doi:10.1016/j.tust.2009.07.008
Nazir, R., Momeni, E., Armaghani, D. J., and Amin, M. M. (2013). Correlation between Unconfined Compressive Strength and Indirect Tensile Strength of limestone Rock Samples. Electron. J. Geotech Eng. 18 (1), 1737
Nguyen, D. H., Dao, V. T. N., and Lura, P. (2017). Tensile Properties of concrete at Very Early Ages. Construction Building Mater. 134, 563–573. doi:10.1016/j.conbuildmat.2016.12.169
Pan, A. N., and Grabinsky, M. W. (2021). Tensile Strength of Cemented Paste Backfill. Geotechnical Test. J. 44 (6), 1–13. doi:10.1520/gtj20200206
Rankine, R. M., and Sivakugan, N. (2007). Geotechnical Properties of Cemented Paste Backfill from Cannington Mine, Australia. Geotech Geol. Eng. 25 (4), 383–393. doi:10.1007/s10706-006-9104-5
Rocco, C., Guinea, G. V., Planas, J., and Elices, M. (1999). Size Effect and Boundary Conditions in the Brazilian Test: Experimental Verification. Mat. Struct. 32 (3), 210–217. doi:10.1007/bf02481517
Rots, J. G., and De Borst, R. (1989). Analysis of concrete Fracture in “Direct” Tension. Int. J. Sol. Structures 25 (12), 1381–1394. doi:10.1016/0020-7683(89)90107-8
Rüsch, H. (1960). Researches toward a General Flexural Theory for Structural concrete. J. Am. Concrete Inst. 57 (1), 1–28.
Sedlacek, R., and Halden, F. A. (1962). Method for Tensile Testing of Brittle Materials. Rev. Scientific Instr. 33 (3), 298–300. doi:10.1063/1.1717827
Tamrakar, S. B., Toyosawa, Y., and Itoh, K. (20052004). Tensile Strength of Soil Measured Using Newly Developed Tensile Strength Apparatus. Kiyose, Japan: Research Reports of National Institute of Industrial Safety
Thompson, B. D., Bawden, W. F., and Grabinsky, M. W. (2012). In Situ measurements of Cemented Paste Backfill at the Cayeli Mine. Can. Geotech. J. 49 (7), 755–772. doi:10.1139/t2012-040
Van Mier, J. G. M., and Van Vliet, M. R. A. (2002). Uniaxial Tension Test for the Determination of Fracture Parameters of concrete: State of the Art. Eng. Fracture Mech. 69 (2), 235–247. doi:10.1016/s0013-7944(01)00087-x
Wright, P. J. F., and Garwood, F. (1952). The Effect of the Method of Test on the Flexural Strength of concrete. Mag. Concrete Res. 4 (11), 67–76. doi:10.1680/macr.1952.4.11.67
Yilmaz, E., Benzaazoua, M., Belem, T., and Bussière, B. (2009). Effect of Curing under Pressure on Compressive Strength Development of Cemented Paste Backfill. Minerals Eng. 22 (9-10), 772–785. doi:10.1016/j.mineng.2009.02.002
Yılmaz, T., Ercikdi, B., Karaman, K., and Külekçi, G. (2014). Assessment of Strength Properties of Cemented Paste Backfill by Ultrasonic Pulse Velocity Test. Ultrasonics 54 (5), 1386–1394. doi:10.1016/j.ultras.2014.02.012
Yong, Y. U. (2005). Questioning the Validity of the Brazilian Test for Determining Tensile Strength of Rocks. Chin. J. Rock Mech. Eng. 24 (7), 1150
Yu, T. R. (1992). “Mechanisms of Fill Failure and Fill Strength Requirements,” in Proceedings of 6th Canadian Rock Mechanics Symposium, 1–6.
Yu, Y., Jianxun, Z., and Jichun, Z. (1997). A Modified Brazilian Disk Tension Test. Int. J. Rock Mech. Mining Sci. 46 (2), 421
Zhang, X., and Zhang, S. (2020). Experimental Investigation on Mechanical Properties of In Situ Cemented Paste Backfill Containing Coal Gangue and Fly Ash. Adv. Civil Eng. 2020, 1–12. doi:10.1155/2020/7964267
Zhao, Y., Taheri, A., Karakus, M., Chen, Z., and Deng, A. (2020). Effects of Water Content, Water Type and Temperature on the Rheological Behaviour of Slag-Cement and Fly Ash-Cement Paste Backfill. Int. J. Mining Sci. Techn. 30 (3), 271–278. doi:10.1016/j.ijmst.2020.03.003
Zheng, W., Kwan, A. K. H., and Lee, P. K. K. (2001). Direct Tension Test of concrete. Mater. J. 98 (1), 63–71.
Zhou, Y., Yan, Y., Zhao, K., Yu, X., Song, Y., Wang, J., et al. (2021). Study of the Effect of Loading Modes on the Acoustic Emission Fractal and Damage Characteristics of Cemented Paste Backfill. Construction Building Mater. 277, 122311. doi:10.1016/j.conbuildmat.2021.122311
Keywords: tensile strength, direct tension, cemented backfill, tailings, dog-bone specimen
Citation: Guo L, Peng X, Zhao Y, Liu G, Tang G and Pan A (2022) Experimental Study on Direct Tensile Properties of Cemented Paste Backfill. Front. Mater. 9:864264. doi: 10.3389/fmats.2022.864264
Received: 28 January 2022; Accepted: 11 February 2022;
Published: 15 March 2022.
Edited by:
Erol Yilmaz, Recep Tayyip Erdoğan University, TurkeyReviewed by:
Eren Komurlu, Giresun University, TurkeyCopyright © 2022 Guo, Peng, Zhao, Liu, Tang and Pan. This is an open-access article distributed under the terms of the Creative Commons Attribution License (CC BY). The use, distribution or reproduction in other forums is permitted, provided the original author(s) and the copyright owner(s) are credited and that the original publication in this journal is cited, in accordance with accepted academic practice. No use, distribution or reproduction is permitted which does not comply with these terms.
*Correspondence: Xiaopeng Peng, pengxiaopeng@bgrimm.com
Disclaimer: All claims expressed in this article are solely those of the authors and do not necessarily represent those of their affiliated organizations, or those of the publisher, the editors and the reviewers. Any product that may be evaluated in this article or claim that may be made by its manufacturer is not guaranteed or endorsed by the publisher.
Research integrity at Frontiers
Learn more about the work of our research integrity team to safeguard the quality of each article we publish.