- 1Integrative Science Center of Germplasm Creation in Western China (CHONGQING) Science City, Key Laboratory of Freshwater Fish Reproduction and Development (Ministry of Education), Southwest University, Chongqing, China
- 2Secció de Bioquímica i Biologia Molecular, Departament de Bioquímica i Fisiologia, Facultat de Farmàcia i Ciències de l’Alimentació, Universitat de Barcelona, Barcelona, Spain
Introduction: Body color is a prominent phenotypic trait and a significant economic characteristic in fish. While current research mainly examines the effects of genetic factors, less emphasis has been placed on the role of endogenous hormones in fish body color.
Methods: In this study, we investigated the changes in phenotype and pigment content in juvenile Sinibrama taeniatus after a 30-day treatment with gradient concentrations of L-thyroxine. To further understanding of the regulatory roles of thyroid hormone on pigment synthesis, we investigated the expression changes in genes involved in melanin-based and non-melanin-based pigmentation under the different hormonal regimes.
Results: Compared to the control group, the body color of the low-concentration L-thyroxine treatment group (20 nM) was lighter, while that of the high-concentration group (40 nM and 80 nM) was darker. Thyroid hormone treatment simultaneously affected melanophores, xanthophores, and iridophores in different ways, leading to an increase of melanin and guanine, and a reduction of pteridine and β-carotene. These findings suggest that thyroid hormone regulates fish body color pigment synthesis through both melanin-based and non-melanin-based mechanisms. Additionally, expression of genes involved in melanin-based (e.g. ctnnb1, wnt10b, and adcy3) and non-melanin-based pigmentation (e.g. xdh, ltk, pnp4a, and mpv17) were modified by thyroid hormone treatment.
Discussion: Our results enhanced the understanding of the role of thyroid hormone in fish pigmentation and provide useful information for cultivating fish with desirable body colors in aquaculture.
1 Introduction
Body color is a striking feature of fish and one of the most important quality criteria dictating the market value of fish for both human consumption and ornamental use (Vissio et al., 2021). Fish exhibit a rich diversity of body colors, attributed to the various types of chromophores. While mammals have only one type of chromophore (melanocyte), at least six types of chromophores have been identified in fish (Schartl et al., 2016). The types of chromatophores differ among fish species, with most possessing three or four types. Fish chromophores can be categorized into two main groups: pigment-containing cells (such as melanophores, xanthophores, erythrophores, and cyanophores) and light-reflecting cells (such as iridophores and leucophores) (Sköld et al., 2016). Chromophores form specific pigment patterns through processes such as cell proliferation, differentiation, migration, and pigment synthesis (Rawls et al., 2001). These processes are regulated by a series of genes known as pigmentation genes (Baxter et al., 2019). Melanophores are the most common type of chromophore, responsible for the synthesis of melanin in fish (Logan et al., 2006). The mechanism of melanin-based pigmentation has been intensively studied in many species, such as zebrafish (Danio rerio), medaka (Oryzias Latipes), and Nile tilapia (Oreochromis niloticus) (Logan et al., 2006; Miyadai et al., 2023; Wang et al., 2022). Researches have shown that the cAMP/CREB-mitf and tyrosine metabolic pathway play an important role in the melanin-based pigmentation (Cal et al., 2017; Inagaki et al., 1998; Tachibana, 2000). In contrast, the non-melanin-based pigmentation, involving other chromophores, has been less thoroughly studied. Xanthophores and erythrophores contribute to yellow and red color through the production of pteridines and carotenoids, respectively. Genes such as csf1ra, gch2, and xdh are specifically linked to the specification and differentiation of xanthophores, as well as pteridines biosynthesis, as described in several fish mutants (Caetano-Lopes et al., 2020; Lu et al., 2022; Wang et al., 2021a). In fish, carotenoids are not synthesized endogenously but are obtained from the diet. However, the accumulation, transport, and metabolism of carotenoids are regulated by specific genes, such as scarb1, bcol, and spra (Caetano-Lopes et al., 2020; Lu et al., 2022; Tian et al., 2024). Iridophores contain purine platelets that reflect lights to generate iridescent colors. Previous studies showed that ltk, pnp4a, mpv17, and hps5 play crucial roles in iridophores differentiation and purine biosynthesis (Kimura et al., 2017; Krauss et al., 2013; Subkhankulova et al., 2023; Wang et al., 2024).
In addition to genetic factors, hormonal factors also regulate the body color of fish (Liu et al., 2023). Hormones can induce dispersion, aggregation and differentiation of chromatophores, influencing pigments synthesis (Vissio et al., 2021). Thyroid hormone (TH) is a key regulator that plays a crucial role in development, growth, metabolism, and pigmentation in fish (Guillot et al., 2016; Power et al., 2001). Recent studies have shown that TH controls the timing of adult color pattern formation during metamorphosis. In Poecilia wingei, alterations in TH levels affected the development of color patterns, leading to a variety of color patterns (Prazdnikov, 2021). TH regulates fish pigmentation by acting on chromatophores. In zebrafish, TH drives melanophores into a terminal differentiation state and limits their final number, while promoting the accumulation of orange carotenoids in xanthophores (McMenamin et al., 2014; Saunders et al., 2019). In clownfishes (Amphiprion occellaris and Amphiprion percula), TH deficiency reduced the number of iridophores and delayed the development of white stripes composed of iridophores (Salis et al., 2021). These studies suggest that TH exerts cell type-specific effects on color pattern formation across various fish species, warranting further investigation in additional fish taxa. It is worth mentioning that TH levels can be affected by environmental factors (Deal and Volkoff, 2020; Nugegoda and Kibria, 2017), linking phenotype variation to environmental change (Lema, 2020). In fish farming, the environment is inevitably affected by various factors. Therefore, it is valuable to investigate the effects of shifts in TH levels on color pattern in cultured fish, as coloration is closely related to fish market value.
Sinibrama taeniatus, an endemic fish species with considerable edible and ornamental value, is primarily distributed in the upper reaches of the Yangtze River (Liu et al., 2022). Under specific breeding conditions, S. taeniatus typically reaches sexual maturity in 4-5 months and spawns approximately about every two weeks, making a potential model fish (Zhao et al., 2023). In our previous study, we found that S. taeniatus takes a long time (~ 12 months) to form an adult pigment pattern, and the TH synthesis pathway is closely associated with pigmentation (Yuan et al., 2023). However, it is unclear whether TH plays a role in the pigmentation of S. taeniatus. In this study, we exposed juvenile S. taeniatus to water with various concentrations of TH and observed changes in body coloration. S. taeniatus possesses three types of chromatophores: melanophores, xanthophores, and iridophores (Yuan et al., 2023). We assessed both the number of chromatophores and pigments content produced by these cells after TH treatment. Additionally, we analyzed the expression patterns of key genes involved in both melanin-based and non-melanin-based pigmentation. Our study aims to elucidate how TH influences pigmentation in S. taeniatus and to provide a substantial basis for understanding the regulatory mechanisms of endocrine hormones on fish pigmentation.
2 Methods and materials
2.1 Ethics statement
All animal experiments conducted in this study were carried out in strict accordance with the guidelines and regulations set forth by the Institutional Animal Care and Use Committee of Southwest University. The research protocols were thoroughly reviewed and approved to ensure the ethical treatment and welfare of the animals involved.
2.2 Hormonal treatment
L-thyroxine (Solarbio Biotechnology, China), with a purity exceeding 98%, was dissolved in dimethyl sulfoxide to prepare a stock solution with a concentration of 10 mM. Store The stock solution was stocked in brown glass bottles at 4°C. Then was gradually diluted with double-distilled water to obtain working solutions with concentrations of 80 nM, 40 nM, 20 nM, 10 nM, and 1 nM, respectively.
2.3 Experimental fish
S. taeniatus were captured from their natural habitat in the Minjiang River (29.935426 N, 103.821574 E.) and transferred to indoor tanks in the laboratory. Two healthy adult fish were selected as broodstock, to produce offspring through artificial fertilization. Three days post-fertilization (dpf), the larvae were transferred from the egg incubator to 3-liter glass tanks. Each tank was equipped with an oxygen pump, a constant temperature system (maintaining a temperature of 26 ± 2°C), and a lighting system with a 13L:11D light cycle. The larvae were fed brine shrimp three times daily. The larvae were randomly divided into six groups (n = 100 per group), with five groups used for experimental treatments and one group serving as a control without L-thyroxine. The experimental groups were exposed to water with varying concentrations of L-thyroxine as indicated in section 2.2 for 30 days. The water in the tanks was replaced every three days with fresh water containing the corresponding concentrations of L-thyroxine. Prior to handling, the fish were anesthetized with MS-222 (Sigma-Aldrich, USA).
2.4 Chromatophore count
Melanophores on the body skin of larval S. taeniatus (n = 9) from each group were imaged using a Nikon SMZ25 stereomicroscope and quantified using ImageJ software. Our previous study indicated that xanthophores in juvenile S. taeniatus exhibit a diffuse form and appear covered by melanophores, while iridophores are small and aggregate in large numbers to reflect light (Yuan et al., 2023). Therefore, it was not feasible to identify individual xanthophores or iridophores for quantification.
2.5 Quantitative analysis of pigments
Dorsal skin samples fromS. taeniatus (n = 3) was collected for pigment detection. Skin melanin content was detected using a fish melanin ELISA kit (Ruixin, China) as described in our previous study (Yuan et al., 2023). The contents of pteridine, β-carotene, and guanine were determined using a high-performance liquid chromatography (HPLC) system (Agilent Technologies, USA). Dorsal skin samples were ground into a powder for HPLC analysis, with strict light control measures implemented during detection.
For detect guanine detection, 0.2 g of sample powder was placed in a brown centrifuge tube. Trifluoroacetic acid (5 mL) and formic acid (5 mL) were added, and the mixture was vortex-mixed for 12 minutes at 90°C. The cooled supernatant (1 mL) was filtered through a 0.45 μm membrane and passed through a chromatographic column (250 x 4.6 mm; particle size: 0.5 μm) for detection at a wavelength of 210 nm using a variable wavelength detector (VWD).
For detect pteridine detection, 0.1 g of sample powder was placed in a brown centrifuge tube with 5 mL of 0.5% potassium hydroxide solution. The pH was adjusted to 7.5 using a 0.1% dilute phosphoric acid solution. After ultrasonic agitation for 30 minutes at 4°C, the mixture was diluted to 8 mL with distilled water. The solution was filtered using a 0.45 μm membrane. Detection was carried out using a chromatographic column (Agilent EC-250 x 4.6 mm; particle size: 5 μm) equipped with a 1200-VWD detector.
For detect β-carotene detection, 0.1 g of sample powder was placed in a brown centrifuge tube with 5 mL of 0.1% BHT-ethanol solution and incubated for four hours. The sample powder underwent two extractions, and the resulting products were separated. The supernatant was collected and diluted to a final volume of 10 mL. For the assay, 0.2 mL of the test solution was filtered through a 0.22 μm membrane and passed through a chromatography column (250 x 4.6 mm; particle size: 0.5 μm) at an absorbance wavelength of 445 nm.
2.6 RNA extraction and cDNA synthesis
The dorsal skin samples (n = 3 per group) were subjected to total RNA extraction using the Animal Total RNA Isolation Kit (Foregene, China). The quality and quantity of RNA were assessed using a Nanodrop ND-1000 spectrophotometer (LabTech, USA). Reverse transcription of RNA (1μL/sample) into cDNA was performed using the PrimeScript™ Reagent Kit (Takara, Japan) for real-time PCR analysis.
2.7 Genes expression analysis using real-time PCR
The expression of genes was analyzed using the TB Green Premix Ex Taq™ II Kit (Takara, Japan) with a QuantStudio 1 system (Thermo Fisher, USA). A total of 24 pigmentation-related genes were selected to detect changes in their expression after treatment with L-thyroxine. The primer sequences for these genes were designed based on the genomic data of S. taeniatus using Primer Premier 5 (Supplementary Table A.1). Gene expression levels were determined using the 2-ΔΔCt method (Schmittgen and Livak, 2008), with β-actin serving as the reference gene.
2.8 Statistical analysis
The statistical analysis was conducted using SPSS version 25.0 (SPSS Inc., Chicago, IL, USA). Mean values were compared using a one-way analysis of variance (ANOVA), followed by the LSD post-hoc test. Statistical significance was set at P < 0.05.
3 Results
3.1 High levels of thyroid hormone reduced melanophore density
After 30 days of treatment with TH, no larvae in any of the groups perished. The larvae’s skin color lightened with increasing TH concentrations, particularly in the 20 nM group. However, larvae in the 40 nM and 80 nM groups exhibited darker skin, with the 80 nM group showing larger melanophores (Figure 1). The density of melanophores on the skin significantly decreased after TH treatment, except in the 1 nM group. In the 40 nM and 80 nM groups, melanophore density was higher than in the 10 nM and 20 nM groups but lower than in the control group (Figure 2).
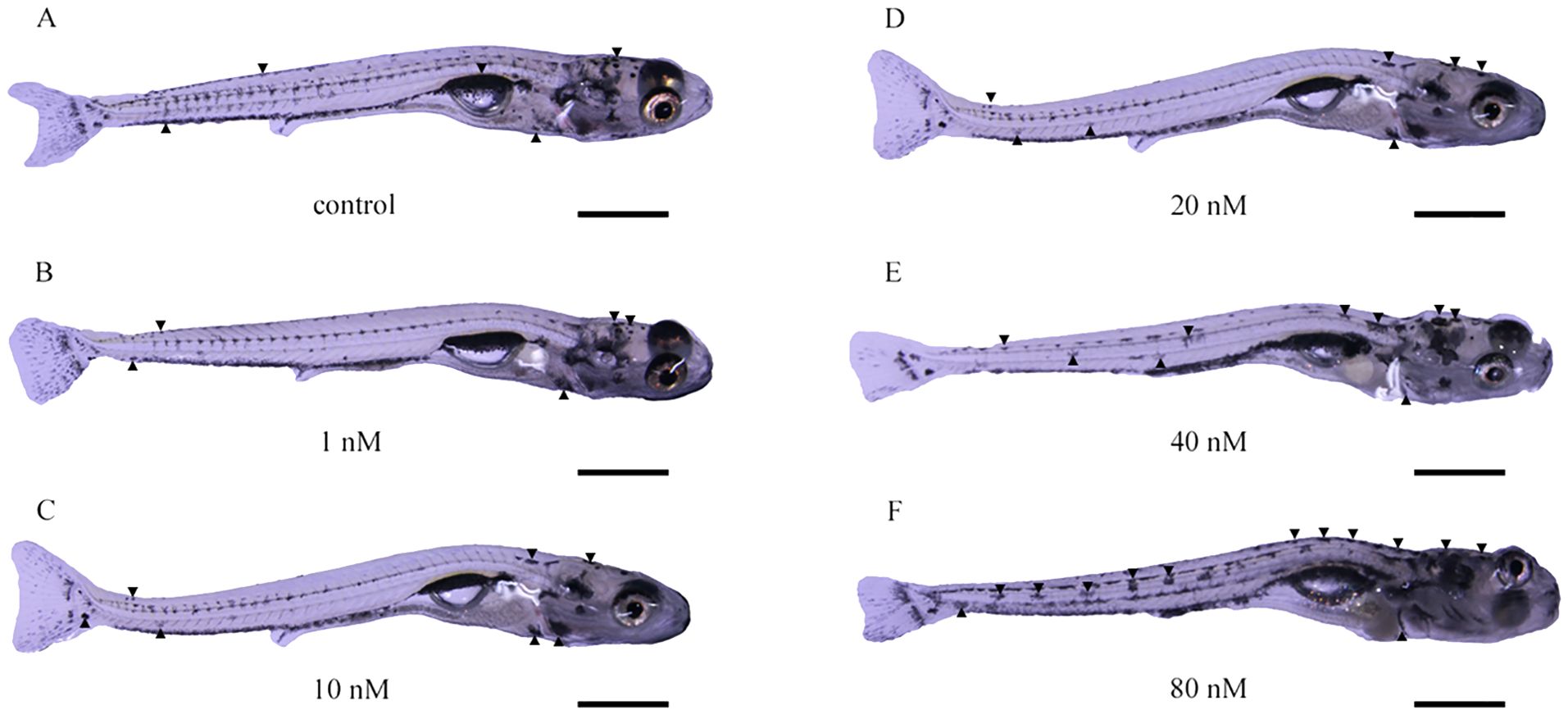
Figure 1. Effect of thyroid hormone on body color of Sinibrama taeniatus larvae. (A) control group; (B) 1 nM group; (C) 10 nM group; (D) 20 nM group; (E) 40 nM group; (F) 80 nM group. The black arrow indicates melanophore. The scale in the figure represents 0.5 mm.
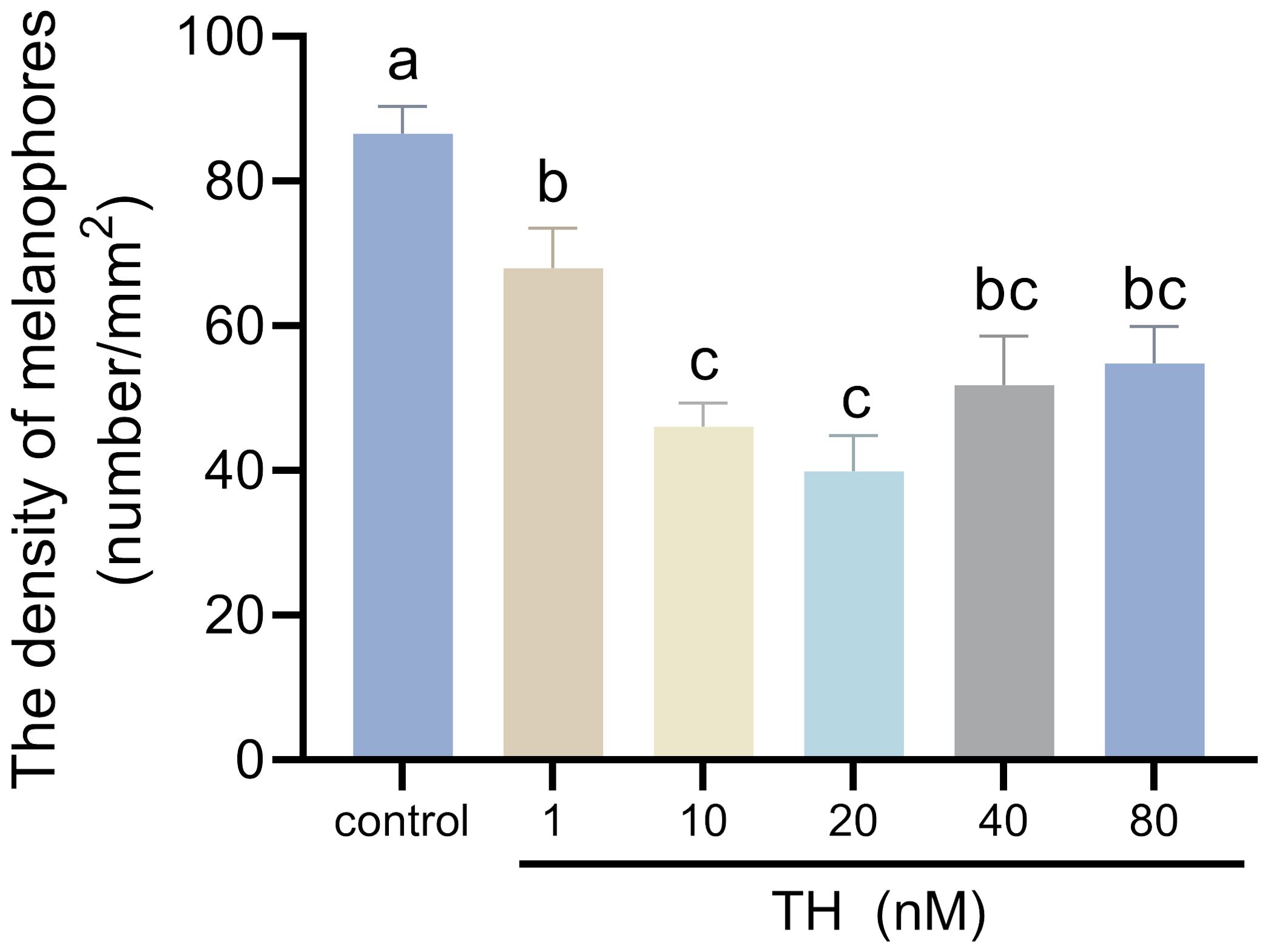
Figure 2. Effects of thyroid hormone on melanophores of Sinibrama taeniatus larvae. Significant differences among developmental stages are indicated by different letters above the error bars.
3.2 Thyroid hormone affected pigment synthesis of three types of chromatophores
The levels of melanin, pteridine, β-carotenoid, and guanine in the dorsal skin of larvae were measured after TH treatment. Melanin content produced by melanophores significantly increased with higher TH concentrations (P < 0.01), peaking in the 40 nM group (Figure 3A). Pteridine content slightly decreased in the 20 nM and 40 nM groups (P < 0.05) (Figure 3B). β-carotenoid content significantly decreased in all treatment groups compared to the control group (P < 0.01) (Figure 3C). Guanine content produced by iridophores significantly increased compared to the control group (P < 0.01), with the highest level observed in the 40 nM group (Figure 3D).
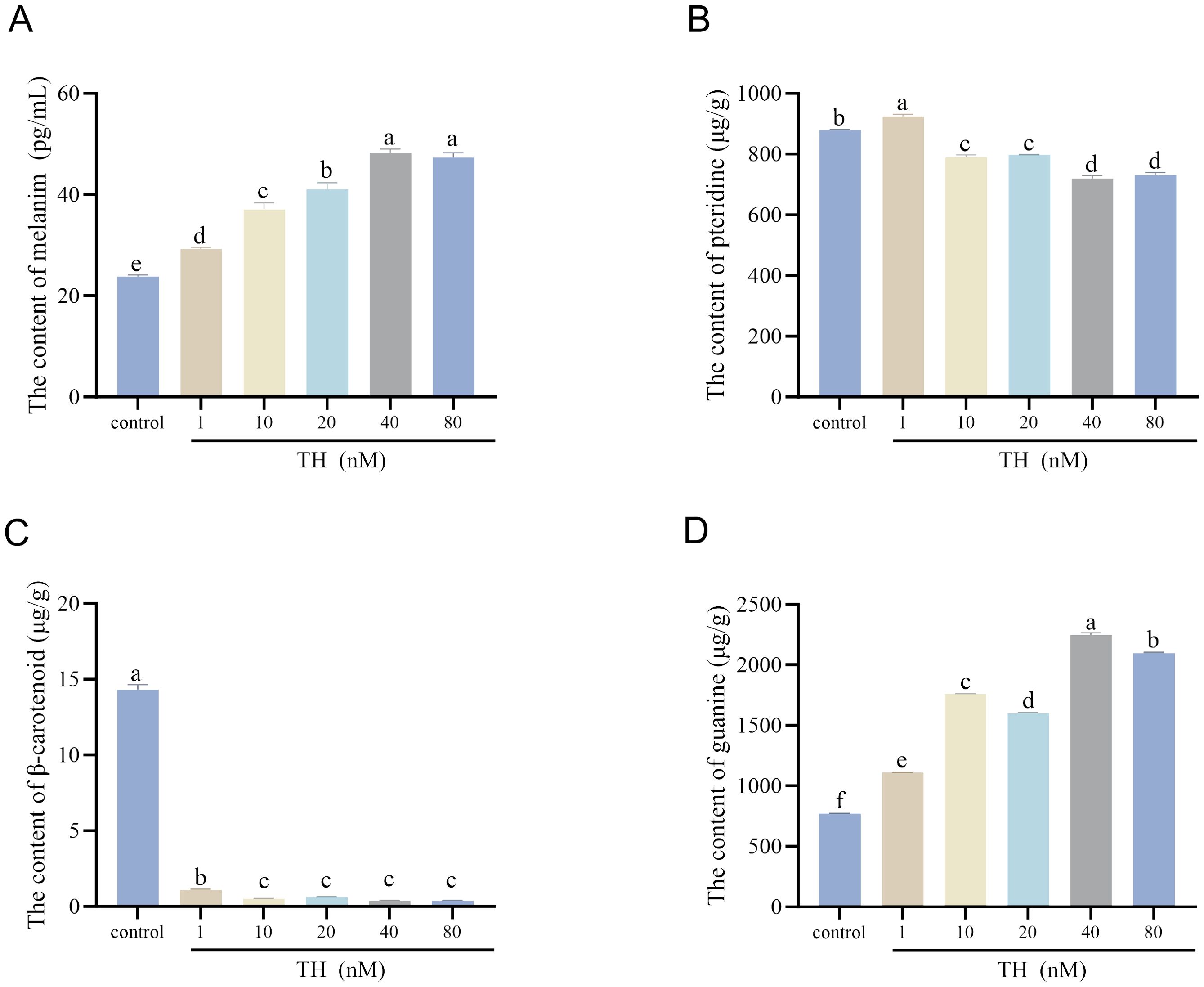
Figure 3. Effects of thyroid hormone on pigment content of Sinibrama taeniatus larvae. (A) the content of melanin after treatment with thyroid hormone; (B) the content of pteridine after treatment with thyroid hormone; (C) the content of β-carotene after treatment with thyroid hormone; (D) the content of guanine after treatment with thyroid hormone. Significant differences among developmental stages are indicated by different letters above the error bars.
3.3 Thyroid hormone-induced expression changes of genes involved in melanin-based pigmentation
For Wnt/β-Catenin signaling pathway, the expression of wnt5b, wnt10b, ctnnb1, plcb4, nfatc2, and gsk3b was detected. Ctnnb1 exhibited the lowest expression in the 20 nM group and highest in the 80 nM group, while wnt10b showed the opposite trend wnt10b. The expression of plcb4 significantly decreased with increasing concentration of TH (P < 0.01). Nfatc2, gsk3b, and wnt5b exhibited dynamic expression changes, while prkaca was significantly downregulated with increasing concentration of TH (P < 0.01). For thyroid hormone signaling pathway, the expression levels of myc and gnaq increased along with increasing concentration of TH. For PI3K−Akt signaling pathway, the expression level of akt2 decreased in the 1 nM group, then increased along with increasing concentration of TH, especially in the 80 nM group. Adcy3 which is enriched in the cAMP−PKA pathway, had the highest expression in the 80 nM group, while lower expression levels were observed in the 1 nM and 20 nM group (Figure 4).
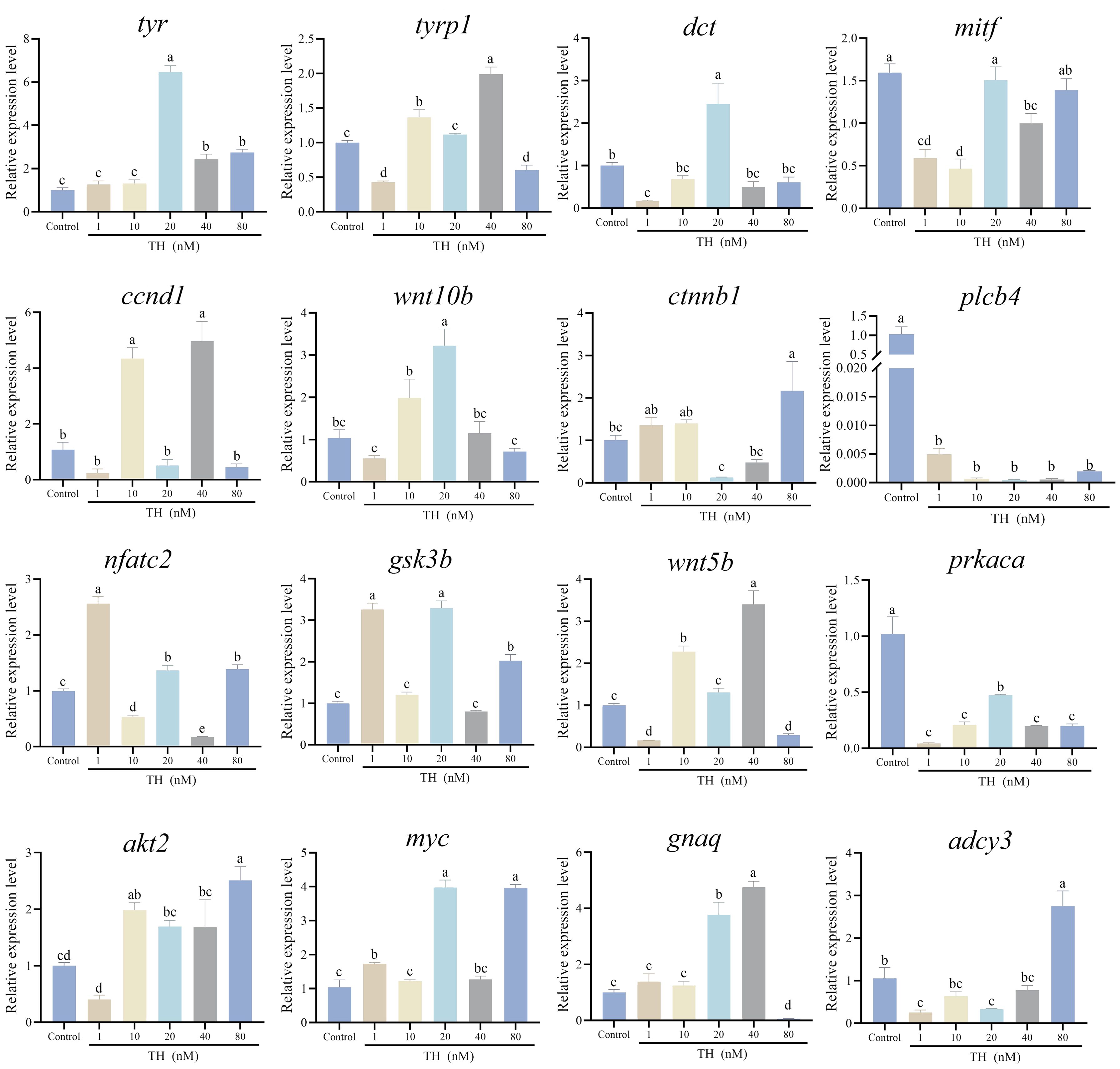
Figure 4. Thyroid hormone modified the expression levels of genes involved in melanin-based pigmentation. Significant differences among developmental stages are indicated by different letters above the error bars.
After TH treatment, the expression levels of 16 genes involved in melanin-based pigmentation were assessed. The key gene tyr, which is crucial in the tyrosine metabolic pathway, was significantly downregulated in the 20 nM group but upregulated in the 40 nM and 80 nM groups (P < 0.01). Similarly, the other two genes in the tyrosine metabolic pathway, tyrp1 and dct, were downregulated in the 1 nM group but upregulated with increasing TH concentration (P < 0.01). Ccnd1 and mitf, both associated with melanogenesis, exhibited different expression differing trends following TH treatment. Ccnd1 expression significantly increased in the 10 nM and 40 nM groups but decreased in the 1 nM, 20 nM, and 80 nM groups (P < 0.01). Mitf expression was downregulated in the 1 nM, 10 nM, and 40 nM groups (P < 0.01).
For the Wnt/β-Catenin signaling pathway, the expression of wnt5b, wnt10b, ctnnb1, plcb4, nfatc2, and gsk3b was measured. Ctnnb1 had the lowest expression in the 20 nM group and the highest in the 80 nM group, while wnt10b showed the opposite trend. Plcb4 expression significantly decreased with increasing TH concentration (P < 0.01). Nfatc2, gsk3b, and wnt5b displayed dynamic expression changes, while prkaca was significantly downregulated with increasing TH concentration (P < 0.01).
In the thyroid hormone signaling pathway, myc and gnaq expression levels increased with higher TH concentrations. For the PI3K-Akt signaling pathway, akt2 expression decreased in the 1 nM group and then increased with higher TH concentrations, especially in the 80 nM group. Adcy3, which is prominent in the cAMP-PKA pathway, had the highest expression in the 80 nM group and lower expression in the 1 nM and 20 nM groups (Figure 4).
3.4 Thyroid hormone-induced expression changes of genes involved in non-melanin-based pigmentation
Eight genes involved in non-melanin-based pigmentation were examined for their expression levels after TH treatment. For genes related to pteridine biosynthesis, pkm, xdh, and urah were all upregulated in the 20 nM group (P < 0.01). Bco1, associated with β-carotenoid absorption, also exhibited the highest expression level in the 20 nM group (P < 0.01), with no significant changes observed among the 1 nM, 40 nM, 80 nM, and control groups (Figure 5A).
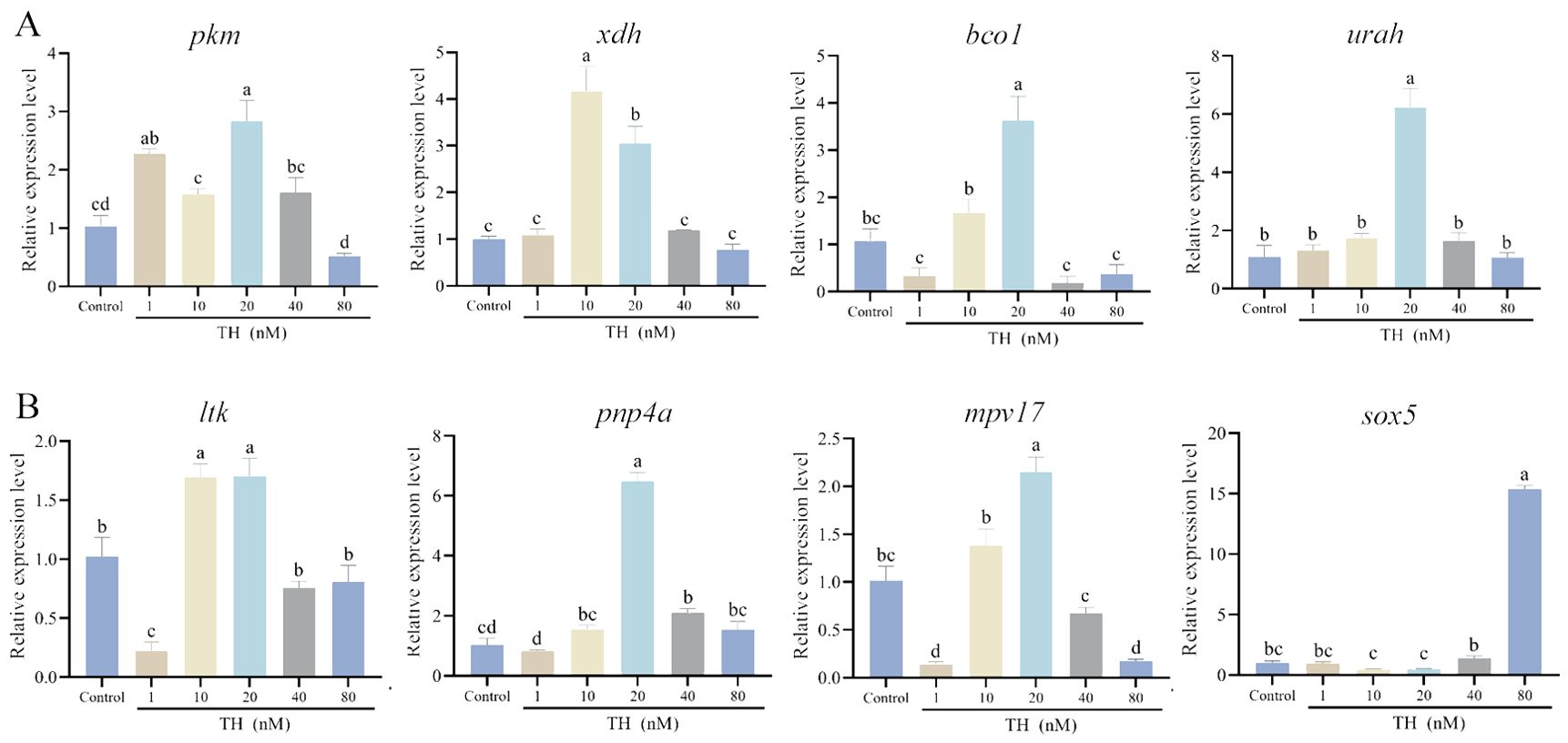
Figure 5. Thyroid hormone modified the expression levels of genes involved in non-melanin-based pigmentation. (A) the expression levels of xanthophores-related genes; (B) the expression levels of iridophores-related genes. Significant differences among developmental stages are indicated by different letters above the error bars.
Ltk, pnp4a, mpv17, and sox5 are key genes in guanine biosynthesis. Ltk expression significantly decreased in the 1 nM group (P < 0.01) and then significantly increased in the 10 nM and 20 nM groups (P < 0.05). Pnp4a was significantly upregulated in the 20 nM and 40 nM groups (P < 0.05), with no notable differences in the other groups. Mpv17 had the highest expression in the 20 nM group and the lowest in the 1 nM and 80 nM groups (P < 0.01). Sox5 expression significantly increased only in the 80 nM group (P < 0.01) (Figure 5B).
4 Discussion
4.1 Roles of endocrine hormones in fish pigmentation
Fish pigmentation is determined by genetic factors but is also influenced by hormones, diet, and environmental conditions (Luo et al., 2021). Extensive research on the genetic basis of pigmentation has enhanced our understanding of the molecular mechanisms underlying color and pattern formation (Du et al., 2019; Kon et al., 2020; Li et al., 2023; Wang et al., 2021b). Increasing evidence indicates that endocrine hormones, such as Mch (Madelaine et al., 2020), Msh (Kobayashi et al., 2012), Agouti-signalling protein 1 (Asip1) (Guillot et al., 2012), somatolactin (Fukamachi et al., 2004), melatonin (Sköld et al., 2008), and TH (McMenamin et al., 2014), play crucial roles in fish pigmentation. However, the regulatory mechanisms by which these hormones influence fish pigmentation remain unclear.
TH is a key hormone regulating the morphological and physiological changes that occur during metamorphosis in teleosts (Campinho, 2019). It is essential for color formation from the larval to juvenile stages, with its levels showing dynamic changes during this process (Prazdnikov and Shkil, 2023). TH can independently regulate different types of chromatophores in fish (Salis et al., 2021; Saunders et al., 2019). For instance, in Danio rerio and Danio albolineatus, TH affects the number of melanophores and xanthophores (McMenamin et al., 2014), while in Amphiprion ocellaris, TH acts only on iridophores (Salis et al., 2021). Interestingly, our research found that TH affects the pigment synthesis of melanophores, xanthophores, and iridophores in S. taeniatus, suggesting that TH can regulate all three types of chromatophores in this species, differing from previously reported fish species. This indicates that the influence of TH varies among chromatophores and shows species-specific differences in teleosts. In aquaculture, fishmeal-based diets often contain thyroxin, and dietary lipid and protein content can affect endogenous endocrine levels, including THs (Brown et al., 2014; Burel et al., 2001; Feist and Schreck, 1990). The goal of aquaculture management is to optimize the performance of aquatic animals under artificial conditions. We believe that exogenous THs hold promise for application in aquaculture to promote development, growth, reproduction, and body color modification in fish.
4.2 Effects of thyroid hormone on melanin-based pigmentation
Melanophores are recognized as the primary regulator of pigmentation in vertebrates (Ligon and McCartney, 2016). In our study, the density of melanophores in S. taeniatus significantly decreased after TH treatment, consistent with findings in zebrafish (Guillot et al., 2016). However, we found that the fish exhibited more heavily melanized skin under high TH concentrations compared to the control group. In zebrafish, single-cell RNA sequencing reveals that TH drives the terminal differentiation of melanophores, increasing their melanization (Saunders et al., 2019). In S. taeniatus, melanin content significantly increased after TH treatment. Hence, we speculate that TH promotes melanophores into a terminally differentiated state and stimulates their melanization, though further observation of cell status is needed.
The mechanisms of melanin-based pigmentation are rather complex since a series of genes are involved in these processes. To further explore the role of TH in melanogenesis, we examined expression levels of relevant under different hormonal regimes. After TH treatment in S. taeniatus, the highest and lowest expression levels of ctnnb1 and adcy3 were observed in the 80 nM and 20 nM groups, respectively, while wnt10b showed the reverse pattern. These expression changes suggest that ctnnb1, wnt10b, and adcy3 are key genes responsive to TH in S. taeniatus. Ctnnb1 and wnt10b are enriched in the Wnt/β-Catenin signaling pathway, and adcy3 belongs to the cAMP-PKA signaling pathway (Yuan et al., 2023). Ctnnb1 (encoding β-catenin) is needed for melanocyte differentiation and melanin synthesis (Bellei et al., 2011). Adcy3 plays a crucial role in mediating cAMP-PKA signaling for regulating melanin synthesis and melanosome dispersion (Zhang et al., 2022). Adcys are the enzymes responsible for converting ATP to cAMP (Devasani and Yao, 2022), and β-catenin is phosphorylated and stabilized by cAMP (Hino et al., 2005). This may explain the similar expression trends of ctnnb1 and adcy3 in the 20 nM and 80 nM groups after TH treatment. Mitf, a master transcriptional regulator, is crucial for melanophor development, differentiation and melanin synthesis (Liu and Fisher, 2010; Vachtenheim and Borovanský, 2010). In this study, after TH treatment, mitf expression significantly decreased in the 1 nM, 10 nM, and 40 nM groups, with no obvious change between the 20 nM, 80 nM, and control groups. Mitf regulates a series of melanogenic genes, including tyr, trp-1, and dct, which encode melanosome enzymes to regulate melanin synthesis (Costin et al., 2005; Fang et al., 2002; Lim et al., 2021; Park et al., 2018). After TH treatment, the expression levels of tyr, trp-1, and dct exhibited dynamic transcriptional changes that did not, align observed phenotypic or melanin content changes in S. taeniatus. The cAMP-PKA and Wnt/β-catenin pathways are upstream regulators of Mitf (Nguyen and Fisher, 2019; Syed et al., 2011). Therefore, we speculate that TH may not primarily act on mitf and its downstream genes but rather affect its upstream regulators in S. taeniatus.
4.3 Effects of thyroid hormone on non-melanin-based pigmentation
Apart from melanophores, other types of chromatophores also play critical roles in fish pigmentation. In zebrafish, TH not only limits the expansion of melanophore populations but also increases the number of xanthophores and enhances visibility of unstained xanthophores by promoting the accumulation of pteridine (McMenamin et al., 2014). Pteridines and carotenoids are the chromogenic substances of xanthophores, with carotenoids obtained from the diet. Xdh is a dehydrogenase involved in pteridine pigment synthesis in fish (Ziegler et al., 2000) and is essential for xanthophore development (Parichy et al., 2000). In this study, the content of pteridines and the expression level of xdh significantly increased after TH treatment, suggesting that TH can regulate xdh expression to alter pteridine synthesis in S. taeniatus. Bco1, a carotenoid metabolizing enzyme, converts a limited number of provitamin A carotenoids to retinaldehyde (Palczewski et al., 2016). The expression level of bco1 significantly increased in the 20 nM group, which corresponds to the decline in carotenoid content with rising TH concentration.
Recent research found that TH also affects the development of iridophores, delaying white bar formation, but does not influence melanophores and xanthophores in Amphiprion percula (Salis et al., 2021). In this study, we found that TH also impacts iridophores in S. taeniatus. The content of guanine, a chromogenic substance in iridophores, significantly increased with rising TH concentration, indicating that TH promotes guanine synthesis. Genes involved in guanine synthesis, such as ltk, pnp4a, and mpv17, exhibited higher expression levels after TH treatment, especially in the 20 nM group. This suggests that TH may regulate these genes to control guanine synthesis. Sox5 is crucial for the fate specification of melanocytes, iridophores, and xanthophores in zebrafish, while it affects xanthophore/leucophore progenitors in medaka (Nagao et al., 2018). In this study, the expression level of sox5 sharply increased in the 80 nM group. We speculate that sox5 may simultaneously influence all types of chromatophores, but the detailed mechanisms require further research.
5 Conclusion
In this study, low-concentration TH at 20 nM induced lighter skin pigmentation, while high-concentration of TH at 40 nM and 80 nM resulted in darker skin pigmentation in S. taeniatus. Additionally, we observed that TH affects both melanin-based and non-melanin-based pigmentation in S. taeniatus by regulating the pigment content synthesized by three types of chromatophores. Specifically, TH promoted the accumulation of pigments synthesized by melanophores and iridophores while inhibiting pteridines content produced by xanthophores. Additionally, we examined the effect of TH on the expression levels of genes involved in both melanin-based and non-melanin-based pigmentation, providing valuable insights into the regulatory network of TH on fish pigmentation. Overall, TH regulates pigment synthesis across all three types of chromatophores in S. taeniatus. However, the mechanism by which these chromatophores respond asymmetrically to TH remains unclear and warrants further research.
Data availability statement
The datasets presented in this study can be found in online repositories. The names of the repository/repositories and accession number(s) can be found in the article/Supplementary Material.
Ethics statement
The animal studies were approved by The Institutional Animal Care and Use Committee of Southwest University. The studies were conducted in accordance with the local legislation and institutional requirements. Written informed consent was obtained from the owners for the participation of their animals in this study.
Author contributions
XL: Data curation, Investigation, Methodology, Software, Writing – original draft. SW: Data curation, Formal analysis, Investigation, Writing – original draft. QF: Methodology, Validation, Writing – original draft. LC: Formal analysis, Validation, Writing – original draft. RT: Resources, Validation, Writing – original draft. LW: Resources, Validation, Writing – original draft. YW: Writing – review & editing. DY: Investigation, Project administration, Supervision, Writing – original draft, Writing – review & editing. ZW: Funding acquisition, Project administration, Writing – review & editing.
Funding
The author(s) declare financial support was received for the research, authorship, and/or publication of this article. This work was supported by the Chongqing Technology Innovation and Application Development Special Key Project (Grant No. CSTB2023TIAD-KPX0023), and the Special Fund for Youth Team of the Southwest University (Grant No. SWU-XJPY202302), and the Fundamental Research Funds for the Central Universities (Grant No. SWU-KQ22016).
Acknowledgments
We gratefully acknowledge the helpful suggestions of Dr. Chenxu Wang and Dr. Baoyue Lu.
Conflict of interest
The authors declare that the research was conducted in the absence of any commercial or financial relationships that could be construed as a potential conflict of interest.
Generative AI statement
The author(s) declare that no Generative AI was used in the creation of this manuscript.
Publisher’s note
All claims expressed in this article are solely those of the authors and do not necessarily represent those of their affiliated organizations, or those of the publisher, the editors and the reviewers. Any product that may be evaluated in this article, or claim that may be made by its manufacturer, is not guaranteed or endorsed by the publisher.
Supplementary material
The Supplementary Material for this article can be found online at: https://www.frontiersin.org/articles/10.3389/fmars.2024.1482306/full#supplementary-material
References
Baxter L. L., Watkins-Chow D. E., Pavan W. J., Loftus S. K. (2019). A curated gene list for expanding the horizons of pigmentation biology. Pigment Cell melanoma Res. 32, 348–358. doi: 10.1111/pcmr.2019.32.issue-3
Bellei B., Pitisci A., Catricalà C., Larue L., Picardo M. (2011). Wnt/β-catenin signaling is stimulated by α-melanocyte-stimulating hormone in melanoma and melanocyte cells: implication in cell differentiation. Pigment Cell melanoma Res. 24, 309–325. doi: 10.1111/j.1755-148X.2010.00800.x
Brown C. L., Urbinati E. C., Zhang W., Brown S. B., McComb-Kobza M. (2014). Maternal thyroid and glucocorticoid hormone interactions in larval fish development, and their applications in aquaculture. Rev. Fisheries Sci. Aquac. 22, 207–220. doi: 10.1080/23308249.2014.918086
Burel C., Boujard T., Kaushik S., Boeuf G., Mol K., van der Geyten S., et al. (2001). Effects of rapeseed meal-glucosinolates on thyroid metabolism and feed utilization in rainbow trout. Gen. Comp. Endocrinol. 124, 343–358. doi: 10.1006/gcen.2001.7723
Caetano-Lopes J., Henke K., Urso K., Duryea J., Charles J. F., Warman M. L., et al. (2020). Unique and non-redundant function of csf1r paralogues in regulation and evolution of post-embryonic development of the zebrafish. Development 147, dev181834. doi: 10.1242/dev.181834
Cal L., Suarez-Bregua P., Cerdá-Reverter J. M., Braasch I., Rotllant J. (2017). Fish pigmentation and the melanocortin system. Comp. Biochem. Physiol. Part A: Mol. Integr. Physiol. 211, 26–33. doi: 10.1016/j.cbpa.2017.06.001
Campinho M. A. (2019). Teleost metamorphosis: the role of thyroid hormone. Front. Endocrinol. 10, 448915. doi: 10.3389/fendo.2019.00383
Costin G.-E., Valencia J. C., Wakamatsu K., Ito S., Solano F., Milac A. L., et al. (2005). Mutations in dopachrome tautomerase (Dct) affect eumelanin/pheomelanin synthesis, but do not affect intracellular trafficking of the mutant protein. Biochem. J. 391, 249–259. doi: 10.1042/BJ20042070
Deal C. K., Volkoff H. (2020). The role of the thyroid axis in fish. Front. Endocrinol. 11, 596585. doi: 10.3389/fendo.2020.596585
Devasani K., Yao Y. (2022). Expression and functions of adenylyl cyclases in the CNS. Fluids Barriers CNS 19, 23. doi: 10.1186/s12987-022-00322-2
Du J., Chen X., Wang J., Chen H., Yue W., Lu G., et al. (2019). Comparative skin transcriptome of two Oujiang color common carp (Cyprinus carpio var. color) varieties. Fish Physiol. Biochem. 45, 177–185. doi: 10.1007/s10695-018-0551-8
Fang D., Tsuji Y., Setaluri V. (2002). Selective down-regulation of tyrosinase family gene TYRP1 by inhibition of the activity of melanocyte transcription factor, MITF. Nucleic Acids Res. 30, 3096–3106. doi: 10.1093/nar/gkf424
Feist G., Schreck C. B. (1990). Hormonal content of commercial fish diets and of young coho salmon (Oncorhynchus kisutch) fed these diets. Aquaculture 86, 63–75. doi: 10.1016/0044-8486(90)90222-9
Fukamachi S., Sugimoto M., Mitani H., Shima A. (2004). Somatolactin selectively regulates proliferation and morphogenesis of neural-crest derived pigment cells in medaka. Proc. Natl. Acad. Sci. 101, 10661–10666. doi: 10.1073/pnas.0401278101
Guillot R., Ceinos R. M., Cal R., Rotllant J., Cerda-Reverter J. M. (2012). Transient ectopic overexpression of agouti-signalling protein 1 (asip1) induces pigment anomalies in flatfish. PloS One 7, e48526. doi: 10.1371/journal.pone.0048526
Guillot R., Muriach B., Rocha A., Rotllant J., Kelsh R. N., Cerdá-Reverter J. M. (2016). Thyroid hormones regulate zebrafish melanogenesis in a gender-specific manner. PloS One 11, e0166152. doi: 10.1371/journal.pone.0166152
Hino S.-i., Tanji C., Nakayama K. I., Kikuchi A. (2005). Phosphorylation of β-catenin by cyclic AMP-dependent protein kinase stabilizes β-catenin through inhibition of its ubiquitination. Mol. Cell. Biol. 25, 9063–9072. doi: 10.1128/MCB.25.20.9063-9072.2005
Inagaki H., Koga A., Bessho Y., Hori H. (1998). The tyrosinase gene from medakafish: transgenic expression rescues albino mutation. Pigment Cell Res. 11, 283–290. doi: 10.1111/j.1600-0749.1998.tb00737.x
Kimura T., Takehana Y., Naruse K. (2017). pnp4a is the causal gene of the medaka iridophore mutant guanineless. G3: Genes Genomes Genet. 7, 1357–1363. doi: 10.1534/g3.117.040675
Kobayashi Y., Mizusawa K., Saito Y., Takahashi A. (2012). Melanocortin systems on pigment dispersion in fish chromatophores. Front. Endocrinol. 3, 15832. doi: 10.3389/fendo.2012.00009
Kon T., Omori Y., Fukuta K., Wada H., Watanabe M., Chen Z., et al. (2020). The genetic basis of morphological diversity in domesticated goldfish. Curr. Biol. 30, 2260–2274. e2266. doi: 10.1016/j.cub.2020.04.034
Krauss J., Astrinides P., Frohnhöfer H. G., Walderich B., Nüsslein-Volhard C. (2013). transparent, a gene affecting stripe formation in Zebrafish, encodes the mitochondrial protein Mpv17 that is required for iridophore survival. Biol. Open 2, 703–710. doi: 10.1242/bio.20135132
Lema S. C. (2020). Hormones, developmental plasticity, and adaptive evolution: endocrine flexibility as a catalyst for ‘plasticity-first’phenotypic divergence. Mol. Cell. Endocrinol. 502, 110678. doi: 10.1016/j.mce.2019.110678
Li B., Chen L., Yan M., Zou X., Bai Y., Xue Y., et al. (2023). Intercross population study reveals that co-mutation of mitfa genes in two subgenomes induces red skin color in common carp (Cyprinus carpio wuyuanensis). Zoological Res. 44, 276. doi: 10.24272/j.issn.2095-8137.2022.388
Ligon R. A., McCartney K. L. (2016). Biochemical regulation of pigment motility in vertebrate chromatophores: a review of physiological color change mechanisms. Curr. Zool. 62, 237–252. doi: 10.1093/cz/zow051
Lim D., Lee K.-J., Kim Y., Kim M., Ju H.-M., Kim M.-J., et al. (2021). A basic domain-derived tripeptide inhibits MITF activity by reducing its binding to the promoter of target genes. J. Invest. Dermatol. 141, 2459–2469. doi: 10.1016/j.jid.2021.01.037
Liu J. J., Fisher D. E. (2010). Lighting a path to pigmentation: mechanisms of MITF induction by UV. Pigment Cell melanoma Res. 23, 741–745. doi: 10.1111/j.1755-148X.2010.00775.x
Liu N., Cheng X., Yang Y., Liu J., Gu H., Wang Z. (2022). Effects of low temperature on the ovarian development of Sinibrama taeniatus. J. Fisheries China 46, 1389–1402. doi: 10.11964/jfc.20210112582
Liu Q., Hu J., Lin Y., Wu X., Feng Y., Ye J., et al. (2024). Effects of exogenous steroid hormones on growth, body color, and gonadal development in the Opsariichthys bidens. Fish Physiol. Biochem. 50 (2), 449–461. doi: 10.1007/s10695-023-01275-5
Logan D. W., Burn S. F., Jackson I. J. (2006). Regulation of pigmentation in zebrafish melanophores. Pigment Cell Res. 19, 206–213. doi: 10.1111/j.1600-0749.2006.00307.x
Lu B., Wang C., Liang G., Xu M., Kocher T. D., Sun L., et al. (2022). Generation of ornamental Nile tilapia with distinct gray and black body color pattern by csf1ra mutation. Aquac. Rep. 23, 101077. doi: 10.1016/j.aqrep.2022.101077
Luo M., Lu G., Yin H., Wang L., Atuganile M., Dong Z. (2021). Fish pigmentation and coloration: Molecular mechanisms and aquaculture perspectives. Rev. Aquac. 13, 2395–2412. doi: 10.1111/raq.12583
Madelaine R., Ngo K. J., Skariah G., Mourrain P. (2020). Genetic deciphering of the antagonistic activities of the melanin-concentrating hormone and melanocortin pathways in skin pigmentation. PloS Genet. 16, e1009244. doi: 10.1371/journal.pgen.1009244
McMenamin S. K., Bain E. J., McCann A. E., Patterson L. B., Eom D. S., Waller Z. P., et al. (2014). Thyroid hormone–dependent adult pigment cell lineage and pattern in zebrafish. Science 345, 1358–1361. doi: 10.1126/science.1256251
Miyadai M., Takada H., Shiraishi A., Kimura T., Watakabe I., Kobayashi H., et al. (2023). A gene regulatory network combining Pax3/7, Sox10 and Mitf generates diverse pigment cell types in medaka and zebrafish. Development 150 (19), dev202114. doi: 10.1242/dev.202114
Nagao Y., Takada H., Miyadai M., Adachi T., Seki R., Kamei Y., et al. (2018). Distinct interactions of Sox5 and Sox10 in fate specification of pigment cells in medaka and zebrafish. PloS Genet. 14, e1007260. doi: 10.1371/journal.pgen.1007260
Nguyen N. T., Fisher D. E. (2019). MITF and UV responses in skin: From pigmentation to addiction. Pigment Cell melanoma Res. 32, 224–236. doi: 10.1111/pcmr.2019.32.issue-2
Nugegoda D., Kibria G. (2017). Effects of environmental chemicals on fish thyroid function: Implications for fisheries and aquaculture in Australia. Gen. Comp. Endocrinol. 244, 40–53. doi: 10.1016/j.ygcen.2016.02.021
Palczewski G., Widjaja-Adhi M. A., Amengual J., Golczak M., Von Lintig J. (2016). Genetic dissection in a mouse model reveals interactions between carotenoids and lipid metabolism. J. Lipid Res. 57, 1684–1695. doi: 10.1194/jlr.M069021
Parichy D. M., Ransom D. G., Paw B., Zon L. I., Johnson S. L. (2000). An orthologue of the kit-related gene fms is required for development of neural crest-derived xanthophores and a subpopulation of adult melanocytes in the zebrafish, Danio rerio. Development 127, 3031–3044. doi: 10.1242/dev.127.14.3031
Park J., Jung H., Kim K., Lim K. M., Kim J. Y., Jho E. H., et al. (2018). D-tyrosine negatively regulates melanin synthesis by competitively inhibiting tyrosinase activity. Pigment Cell melanoma Res. 31, 374–383. doi: 10.1111/pcmr.2018.31.issue-3
Power D. M., Llewellyn L., Faustino M., Nowell M. A., Björnsson B. T., Einarsdóttir I. E., et al. (2001). Thyroid hormones in growth and development of fish. Comp. Biochem. Physiol. Part C: Toxicol. Pharmacol. 130, 447–459. doi: 10.1016/S1532-0456(01)00271-X
Prazdnikov D. V. (2021). Role of thyroid hormones in color diversity of male guppies: experimental data on Endler’s guppy (Poecilia wingei) Thyroid hormones and guppy color diversity. Environ. Biol. Fishes 104, 675–688. doi: 10.1007/s10641-021-01102-x
Prazdnikov D. V., Shkil F. N. (2023). The role of thyroid hormones in the development of coloration of two species of Neotropical cichlids. J. Exp. Biol. 226, jeb245710. doi: 10.1242/jeb.245710
Rawls J. F., Mellgren E. M., Johnson S. L. (2001). How the zebrafish gets its stripes. Dev. Biol. 240, 301–314. doi: 10.1006/dbio.2001.0418
Salis P., Roux N., Huang D., Marcionetti A., Mouginot P., Reynaud M., et al. (2021). Thyroid hormones regulate the formation and environmental plasticity of white bars in clownfishes. Proc. Natl. Acad. Sci. 118, e2101634118. doi: 10.1073/pnas.2101634118
Saunders L. M., Mishra A. K., Aman A. J., Lewis V. M., Toomey M. B., Packer J. S., et al. (2019). Thyroid hormone regulates distinct paths to maturation in pigment cell lineages. Elife 8, e45181. doi: 10.7554/eLife.45181.040
Schartl M., Larue L., Goda M., Bosenberg M. W., Hashimoto H., Kelsh R. N. (2016). What is a vertebrate pigment cell? Pigment Cell melanoma Res. 29, 8–14. doi: 10.1111/pcmr.12409
Schmittgen T. D., Livak K. J. (2008). Analyzing real-time PCR data by the comparative CT method. Nat. Protoc. 3, 1101–1108. doi: 10.1038/nprot.2008.73
Sköld H. N., Amundsen T., Svensson P. A., Mayer I., Bjelvenmark J., Forsgren E. (2008). Hormonal regulation of female nuptial coloration in a fish. Hormones Behav. 54, 549–556. doi: 10.1016/j.yhbeh.2008.05.018
Sköld H. N., Aspengren S., Cheney K. L., Wallin M. (2016). Fish chromatophores—from molecular motors to animal behavior. Int. Rev. Cell Mol. Biol. 321, 171–219. doi: 10.1016/bs.ircmb.2015.09.005
Subkhankulova T., Camargo Sosa K., Uroshlev L. A., Nikaido M., Shriever N., Kasianov A. S., et al. (2023). Zebrafish pigment cells develop directly from persistent highly multipotent progenitors. Nat. Commun. 14, 1258. doi: 10.1038/s41467-023-36876-4
Syed D. N., Afaq F., Maddodi N., Johnson J. J., Sarfaraz S., Ahmad A., et al. (2011). Inhibition of human melanoma cell growth by the dietary flavonoid fisetin is associated with disruption of Wnt/β-catenin signaling and decreased Mitf levels. J. Invest. Dermatol. 131, 1291–1299. doi: 10.1038/jid.2011.6
Tachibana M. (2000). MITF: a stream flowing for pigment cells. Pigment Cell Res. 13, 230–240. doi: 10.1034/j.1600-0749.2000.130404.x
Tian X., Shan Y., Peng N., Ma X., Shi X., Li H., et al. (2024). Sepiapterin reductase (Spra and Sprb) regulate carotenoid and pteridine metabolism influencing the koi carp (Cyprinus carpio L.) coloration. Aquac. Rep. 34, 101900. doi: 10.1016/j.aqrep.2023.101900
Vachtenheim J., Borovanský J. (2010). Transcription physiology” of pigment formation in melanocytes: central role of MITF. Exp. Dermatol. 19, 617–627. doi: 10.1111/j.1600-0625.2009.01053.x
Vissio P. G., Darias M. J., Di Yorio M. P., Sirkin D. I. P., Delgadin T. H. (2021). Fish skin pigmentation in aquaculture: The influence of rearing conditions and its neuroendocrine regulation. Gen. Comp. Endocrinol. 301, 113662. doi: 10.1016/j.ygcen.2020.113662
Wang C., Kocher T. D., Liu H., Wen Z., Yao J., Wang D. (2024). Knockout of Hermansky-Pudlak syndrome 5 (hps5) leads to red tilapia with reduced melanophores and iridophores. Aquaculture 582, 740496. doi: 10.1016/j.aquaculture.2023.740496
Wang C., Kocher T. D., Lu B., Xu J., Wang D. (2022). Knockout of hermansky-pudlak syndrome 4 (hps4) leads to silver-white tilapia lacking melanosomes. Aquaculture 559, 738420. doi: 10.1016/j.aquaculture.2022.738420
Wang C., Lu B., Li T., Liang G., Xu M., Liu X., et al. (2021a). Nile tilapia: a model for studying teleost color patterns. J. Heredity 112, 469–484. doi: 10.1093/jhered/esab018
Wang L., Sun F., Wan Z. Y., Ye B., Wen Y., Liu H., et al. (2021b). Genomic basis of striking fin shapes and colors in the fighting fish. Mol. Biol. Evol. 38, 3383–3396. doi: 10.1093/molbev/msab110
Yuan D., Liu X., Wang S., Wang H., Li J., Zhao Z., et al. (2023). Identification of key regulatory pathways and genes involved in the dynamic progression of pigmentation in Sinibrama taeniatus. Front. Mar. Sci. 10, 1202846. doi: 10.3389/fmars.2023.1202846
Zhang L., Wan M., Tohti R., Jin D., Zhong T. P. (2022). Requirement of zebrafish Adcy3a and Adcy5 in melanosome dispersion and melanocyte stripe formation. Int. J. Mol. Sci. 23, 14182. doi: 10.3390/ijms232214182
Zhao Z., Zhao Q., Wang H., Wei L., Wang S., Li S., et al. (2023). Integrated transcriptomic and metabolomic analyses identify key factors in the vitellogenesis of juvenile Sichuan bream (Sinibrama taeniatus). Front. Mar. Sci. 10, 1243767. doi: 10.3389/fmars.2023.1243767
Keywords: body color, chromophores, Cyprinidae fish, pigmentation, thyroid hormone
Citation: Liu X, Wang S, Feng Q, Cheng L, Teng R, Wei L, Wu Y, Wang Z and Yuan D (2024) Thyroid hormone regulates both melanin and non-melanin pigmentation in Sinibrama taeniatus via three types of chromophores. Front. Mar. Sci. 11:1482306. doi: 10.3389/fmars.2024.1482306
Received: 18 August 2024; Accepted: 02 October 2024;
Published: 17 October 2024.
Edited by:
Vikash Kumar, Central Inland Fisheries Research Institute (ICAR), IndiaReviewed by:
Mónica Lopes-Marques, University of Porto, PortugalSoumya Prasad Panda, Central Inland Fisheries Research Institute (ICAR), India
Sofia Priyadarsani Das, National Taiwan Ocean University, Taiwan
Copyright © 2024 Liu, Wang, Feng, Cheng, Teng, Wei, Wu, Wang and Yuan. This is an open-access article distributed under the terms of the Creative Commons Attribution License (CC BY). The use, distribution or reproduction in other forums is permitted, provided the original author(s) and the copyright owner(s) are credited and that the original publication in this journal is cited, in accordance with accepted academic practice. No use, distribution or reproduction is permitted which does not comply with these terms.
*Correspondence: Zhijian Wang, wangzj1969@126.com; Dengyue Yuan, yuandengyue@163.com
†These authors have contributed equally to this work