- 1Estonian Marine Institute, University of Tartu, Tallinn, Estonia
- 2Department of Functional and Evolutionary Ecology, Faculty of Life Sciences, University of Vienna, Vienna, Austria
- 3College of Oceanography and Ecological Science, Shanghai Ocean University, Shanghai, China
- 4Department of Marine Science, University of Otago, Dunedin, New Zealand
- 5Centre of Research Excellence, University of Otago, Dunedin, New Zealand
The extracellular release of dissolved organic carbon (DOC) from marine macroalgae supports coastal ecosystem function by supplying photosynthetically fixed carbon to higher trophic levels via the microbial loop. Despite its widely acknowledged biogeochemical importance, DOC is not typically included in primary production estimates of coastal systems. Additionally, little is known about how changes in species composition and coverage will affect the supply of DOC to coastal systems. Within the context of the Baltic Sea, anthropogenic forces are driving a decline in habitat forming kelp species (Fucus vesiculosus) which is superseded by filamentous/turfing algal species, a pattern of change observed globally in numerous other aquatic systems. To evaluate how the drivers of this change may impact the flow of carbon within the Baltic Sea coastal system, the production of DOC by the filamentous algae (Ectocarpus siliculosus) was examined and its rate of release determined (0.095 mg C · g DW−1 · h−1 in light and 0.070 mg C · g DW−1 · h−1 in dark). In addition, bioassays were used to assess the short-term DOC use by bacteria as a proxy of the lability of the released products, with the majority (28.7% released in light and 18.6% released in dark) of the products remaining after 120 hours. This data was linked with long term macroalgae biomass and coverage surveys in order to assess changes in macroalgae community structure through time and to produce standing stock estimates of F. vesiculosus. DOC production as a metric of algal coverage was used to make a preliminary estimation of how changes in community structure may impact the flow of carbon within the system. Our results suggest that decreased levels of DOC released by filamentous algae relative to F. vesiculosus will act to reduce microbial activity. Our model estimates the presence of approximately 150,000 tonnes of F. vesiculosus (dry weight) within the Estonian coastal system translating to an annual release of 7,391 tonnes of DOC. Our study indicates that filamentous-dominated systems have likely altered carbon flow, impacting the broader ecology of the Baltic Sea. Consequently, the loss of kelp species and the expansion of filamentous algae may alter carbon dynamics, with important ecological consequences for other coastal systems globally.
1 Introduction
It is now well established that numerous species of macroalgae release a fraction of their photosynthetic products as dissolved organic matter (DOM) (Paine et al., 2021; Hall et al., 2022; Abdullah and Fredriksen, 2004). This, coupled with their high rate of photosynthetic activity, implicates them as an important group involved in the transfer of carbon from primary producers to higher trophic levels via the release of this matter. DOM transfer has therefore been suggested to play a central role in driving microbial function in nearshore coastal ecosystems (Pomeroy et al., 2007). Despite the recognized need to develop more comprehensive models for the flux of carbon in coastal ecosystems, a lack of primary information regarding release rates and the lability of these products presents a substantial challenge for the accurate evaluation of these systems. Specifically, we lack baseline estimates of DOC release rates for the vast majority of macroalgae species which is further confounded by a lack of temporal information regarding how seasonality and life history influence DOC release rates. Few studies report the lability of released products and the degree to which they are consumed by heterotrophic bacteria, thus limiting our ability to assess the importance of macroalgal DOC in the microbial loop (Egea et al., 2023; Xie et al., 2024). A need therefore arises to relate measured values of DOC release and consumption with macroalgae biomass estimates as means to assess the magnitude of carbon transfer. Therefore, it is important to assess the ecology of macroalgae not only at the species level but also at the community level, which is still inadequately understood.
The Baltic Sea represents a proper model area for examining the flux of dissolved organic nutrients within an aquatic context. The sea’s naturally low salinity (Snoeijs-Leijonmalm, 2017; HELCOM Balance, 2007 from http://balance-eu.org/publications/index.html) also presents a substantial physiological challenge for the organisms that inhabit their as osmotic pressure acts to restrict a combination of both marine and freshwater organisms (Snoeijs-Leijonmalm and Andrén, 2017). Consequently, the Baltic Sea displays a strong trend of low diversity but high biomass relative to other fully marine systems (Kautsky et al., 2017; Snoeijs-Leijonmalm, 2017; Snoeijs-Leijonmalm and Andrén, 2017). Additionally, the Baltic Sea’s relatively low volume combined with slow water exchange with North Sea constricted by the Danish Straits results in a residence time in the range of 30 to 40 years (Snoeijs-Leijonmalm and Andrén, 2017). Consequently, the Baltic Sea is particularly susceptible to anthropogenic forces, which have acted to yield substantial change in the sea’s underlying water chemistry (Elmgren, 2001; Andersson et al., 2015). This is particularly evident with the influx of high nutrient loads beginning in the early 20th century associated with industrial agricultural producing a marked increase in both nitrogen and phosphorous loads (Elmgren, 2001; Fonselius and Valderrama, 2003; Gustafsson et al., 2012; Andersson et al., 2015). Regime shifts have been documented for the Baltic Sea ecosystem during the second half of the 20th century, involving different components of ecosystem (Alheit et al., 2005). Notably, the Baltic Sea has been observed to have undergone drastic shifts in both the distribution and presence of its phytobenthic communities (Eriksson et al., 1998; Kovtun et al., 2009; Eveleens Maarse et al., 2020).
In the Baltic Sea, Fucus vesiculosus Linnaeus acts as a phytobenthic ecosystem engineer (Malm and Isæus, 2005; Kautsky et al., 2017, 2019). F. vesiculosus’ high productivity coupled with its substantial biomass within the littoral rocky photic zone, colloquially named the ‘Fucus belt’, plays an important role in structuring coastal habitats by providing complex three-dimensional structure that acts to positively affect biodiversity by supporting numerous other organisms, including both invertebrates and fishes e.g. Haage, 1975, 1976; Kautsky and Kautsky, 1989; Wallentinus, 1991; Wikström and Kautsky, 2007. Therefore, any changes to the health or structure of the Fucus belt are likely to dramatically affect the system as a whole. Beginning in the early to mid-20th century, F. vesiculosus has declined in abundance throughout the Baltic Sea as a consequence of a variety of anthropogenic factors including both point source pollution affecting local populations as well as a more generalized decline due to sea wide eutrophication (Lehtinen et al., 1988; Cederwall, 1990; Vogt and Schramm, 1991; Schramm, 1996; Nilsson et al., 2004; Torn et al., 2006; Rinne and Salovius-Laurén, 2020). While numerous studies have investigated the consequences of Fucus spp. decline, including F. vesiculosus, on coastal ecosystem function such as habitat loss (Airoldi et al., 2008; Rinne and Salovius-Laurén, 2020; Rinne et al., 2022), fish or fisheries decline (Rajasilta et al., 1999; Kautsky and Kautsky, 2000; Mattsson, 2019), and nutrient uptake (Duarte et al., 2015), none have yet to explore how this loss may act to alter carbon flow through changes in the quantities of DOC released by macroalgae. Such a question is relevant as DOC is released across the lifetime of the plant rather than just during senescence. Depending upon the lability of the released products, this DOC represents an under accounted for and likely important source of carbon which may support the wider food web via the microbial loop or alternatively act as an important carbon sink (Paine et al., 2021; Dolliver and O’Connor, 2022).
The ecophysiological properties a given algal species possesses underpins its growth rate and when a resource such as space, light, or nutrients, is limited, determines its ability to out compete others (Olson and Lubchenco, 1990; Edwards and Connell, 2012). Within the context of the Baltic Sea, eutrophication has been demonstrated to favor filamentous brown algal species for this reason (Kiirikki and Blomster 1996; Bonsdorff et al., 1997). Such species compete with and displace larger perennial species like F. vesiculosus through the formation of dense matts which prevent settlement and attachment of fucoids to hard substrate (Kiirikki, 1996a). Additionally, filamentous brown algae attach epiphytically to existing fucoids, smothering the host reducing overall fitness (Berger et al., 2003; Isæus et al., 2004). Moreover, benthic filamentous algae act to entrain large amounts of sediment, further suppressing the settlement and fitness of benthic algae post settlement (Isæus et al., 2004). In the Northeastern Baltic Sea, two dominant species of filamentous ‘turfing’ algae are present, Pyaiella littoralis and Ectocarpus siliculosus, which can be distinguished only by examination of the reproductive structures or through genetic tests. Both P. littoralis and E. siliculosus are capable of taking advantage of elevated nutrient levels or otherwise large nutrient pulses, particularly during the spring months (Kiirikki, 1996b). Consequently, these species can efficiently compete for substrates which in turn excludes slower growing perennial species like F. vesiculosus (Berger et al., 2004; Nilsson et al., 2004). Thus, eutrophication has led to a clear pattern of F. vesiculosus decline which is supplanted by brown filamentous turfing algal species (Figure 1).
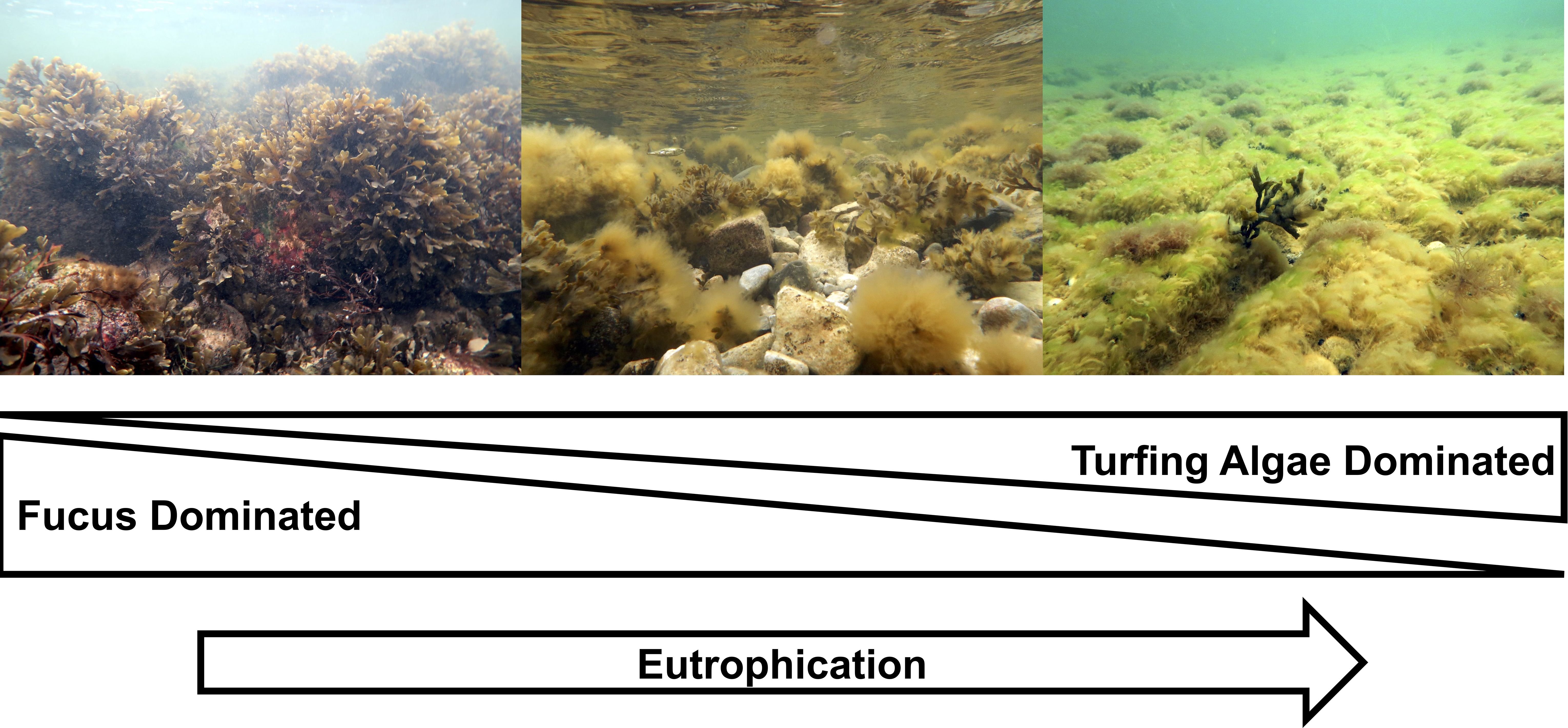
Figure 1 Schematized diagram depicting the generalized transition from F. vesiculosis dominated habitats to turfing algal-dominated habitats in the shallow coastal waters of the Estonian coastal system and the Baltic Sea proper, correlating with increasing eutrophication. Photo source: Estonian Marine Institute database.
The Baltic Sea is undergoing increased change due to a convergence of factors in addition to eutrophication, this includes: climate change, ocean acidification, and overfishing, among others (Österblom et al., 2007; Korpinen et al., 2012; Andersen et al., 2017). The impact these factors have on marine ecosystems has been studied in relation to physical–biogeochemical–carbonate models (e.g., Neumann, 2010; Meier et al., 2011a, b; Neumann et al., 2012; Omstedt et al., 2012) as well as food web models (e.g., Niiranen et al., 2013). However, our ability to link lower trophic levels with higher ones remains limited and our knowledge of how shifts in communities as a result of anthropogenic forces complicates these matters. Hall et al. (2022) measured the DOC release rates of three ecologically significant species in the Baltic Sea: the perennial habitat-forming Fucus vesiculosus and Furcellaria lumbricalis, and the seasonal, fast-growing Ulva intestinalis under light and dark treatments. The results reported that both F. vesiculosus and U. intestinalis released significant quantities of DOC (Hall et al., 2022). To date, no studies have examined specifically the release, nor the lability of photosynthetic products released by brown turfing algal species found within the Baltic. However, a review into the topic of macroalgae DOC release by Paine et al. (2021) identified filamentous algae as having the highest range of DOC release rates globally, relative to other functional groups. It is suggested that elevated levels of release are related to filamentous algae’s underlying high growth rate and opportunistic life strategy combined with their high surface to volume ratio (Hurd et al., 2014). Such a finding implicates brown filamentous turfing algae as potentially having a disproportionate role in supporting coastal ecosystem microbial activity function by contributing to the microbial loop through the release of DOC.
In this study, we investigate the decline of F. vesiculosus populations and the concurrent rise of brown turfing algae, with a particular focus on the DOC release process. We quantify the rate at which DOC is released by the fast-growing brown turfing algae E. siliculosus in both light and dark conditions. Furthermore, we assess the extent to which heterotrophic marine bacteria consume these released DOC to assess the short-term DOC use by bacteria as a proxy for the lability of the released products. By integrating this data with previously documented macroalgae DOC release rates, we establish connections with the historical evolution of macroalgae biomass along the Estonian coastline. This approach enables us to estimate the flow of DOC within the coastal Baltic Sea ecosystem over time. Additionally, we discuss whether anthropogenic factors have altered phytobenthic communities and suggest how shifts in these communities has and will continue to alter carbon flow within the system.
2 Methods
2.1 Study area
The Estonian marine environment, located in the NE of the Baltic Sea, is characterized by complex coastal morphology formed by abrupt changes in sea level during the Holocene, encompasses sandy shores, rocky outcrops, and estuarine areas (Snoeijs-Leijonmalm, 2017). The brackish nature of the Baltic Sea imparts salinity usually below 8 (PSU), with pronounced salinity gradients near major river outflows (Snoeijs-Leijonmalm, 2017). Benthic substrate variations include moving sand, granite boulder fields, gravel, and limestone cliffs/craters (Snoeijs-Leijonmalm, 2017). Environmental conditions are influenced by strong seasonal patterns, impacting the marine ecosystem, with winters leading to the formation of sea ice and warm summers affecting overall water temperature (Snoeijs-Leijonmalm, 2017). Phytobenthic communities, include macroalgae and seagrasses, which provide complex three-dimensional habitat representing spaces high in biodiversity (Kautsky et al., 2017).
2.2 Macrophytobenthos data
The macrophytobenthos data used in this study originates from the macrobenthos database of the Estonian Marine Institute, University of Tartu. This extensive database, which dates back to the 1950s, contains over 57,000 georeferenced benthic samples collected from various projects, including basic scientific research, national marine monitoring, benthic habitat mapping, and environmental impact assessments. For this study, data from two macrophytobenthos sampling methodologies was selected: 1) diver-collected quantitative biomass samples and 2) divers’ visual coverage estimates. Quantitative biomass samples are collected by SCUBA divers using 20 × 20 cm quadrats placed on the seabed, where all macroscopic fauna and flora within the frame is collected. The samples are stored at -18°C for later analysis. In the laboratory, these samples are sorted, and all macrobenthic organisms are identified under a microscope. The dry weights of all taxa are measured after drying for two weeks at 60°C, and the values converted to biomass per square meter. The coverage estimates are obtained by SCUBA divers visually assessing the percentage cover of macrophyte species on the seabed.
Data from the long term national phytobenthos monitoring transects was used for the trend analysis. The Estonian phytobenthos monitoring method is based on the HELCOM COMBINE guidelines (www.helcom.fi). Monitoring is carried out along an imaginary transect line placed perpendicular to the shoreline from a predetermined starting point. Observations are carried out once inside a 1 m depth interval to the deepest limit of vegetation. When the deepest limit is reached, possible occurrence of vegetation deeper is checked by an underwater drop video camera. A continuous transect line, often suggested for observations along Scandinavian rocky shores, is not used because of the small slope and the resulting length of transects. For a quantitative description of phytobenthic communities the biomass samples are obtained from each different community type. Depending on the length of the transect biomass samples are taken from 5-7 depth intervals. Most commonly samples from depths 0.2, 0.5, 1-2, 2-3, 4-5 and 6-8 meters were collected. Quantitative biomass samples are taken always in three replicates, 20 × 20 cm frames with attached bag are used. Samples are stored in a deep-freezer and later sorted and determined to species level in a laboratory. Each species is dried separately at 60°C until constant weight is reached and the dry weight is measured with 0.0001 g accuracy.
For the trend analysis, data from the following long term (1995-2021) phytobenthos monitoring transects was extracted: Liu transect in the Pärnu Bay, Kõiguste transect in the Gulf of Riga, Küdema transect in the Baltic Proper, Tallinn transect in the Gulf of Finland, Eru transect in the Gulf of Finland (Figure 2). The data was further filtered by constraining water depth range of samples between 0.5 m and the maximum depth of the occurrence of Fucus spp. The maximum depth of Fucus was assessed separately on each transect over the full range of years. The species and groups of species for the trend analysis were:
1. 1) Fucus spp.: summed group of F. vesiculosus and F. radicans;
2. 2) Summed group of Pylaiella littoralis and Ectocarpus siliculosus (hereafter “PylaiellaEctocarpus”). The species are morphologically highly similar and determination between the species is only possible under microscope when reproductive organs are present. In situ visual distinguishing between the two species by a diver is not possible;
3. 3) Summed group of opportunistic algae as defined in the Estonian national phytobenthos monitoring method (Torn et al., 2014): Capsosiphon fulvescens, Chaetomorpha linum, Cladophora spp., Ectocarpus siliculosus, Monostroma balticum, Oedogonium sp., Percursaria percursa, Pylaiella littoralis, Rhizoclonium riparium, Spongomorpha aeruginosa, Stigeoclonium sp., Ulothrix sp., Ulva spp., Urospora penicilliformis.
As an input for trend analysis, mean cover of Fucus spp, ‘Pylaiella-Ectocarpus’ and ‘opportunistic algae’ was calculated for each transect and year.
For modeling the distribution of biomass of Fucus, data from biomass samples collected in the Estonian marine area from the years 2005-2021 was used. Samples from that period represent a methodologically coherent dataset with good spatial coverage. Temporal replicates from sampling sites that were visited several times, were removed and only data from the most recent sampling event were retained in the dataset. The application of these temporal and spatial filters resulted in Fucus biomass data from 1469 sites (Figure 2). Data from replicate biomass samples (n = 3) of each sampling site was averaged.
2.3 Environmental variables
A total of 15 environmental variables, including topographical (depth, slope of seabed), hydrodynamic (wave exposure, currents, ice conditions), geological (seabed substrate), physico-chemical (temperature, salinity, nutrients), and biological (water chlorophyll concentration) variables (Table 1), were used for spatial prediction of the biomass of F. vesiculosus. Mean values were used for variables with time series, because general long-term spatial distribution, not temporal variation (seasonal, annual) of the biomass of F. vesiculosus was addressed in this study. All environmental variables were available as georeferenced raster layers in GeoTIFF format. The extents of raster layers fully covered the study area. Raster cell size was 10 m for depth and seabed slope and 50 m for all the other variables. The environmental variables were selected based on previous knowledge on potential relationships with the distribution of the studied macrophytes and data availability.
2.4 Macrophytobenthos data analysis
Mann-Kendall test was used to identify trends in the time series of coverage of Fucus, PylaiellaEctocarpus, and opportunistic algae. The Mann-Kendall test is a nonparametric test for monotonic trends. The test results in numeric estimates of statistical significance (p-values) and Kendall’s tau coefficient (τ). Kendall’s tau is a measure of correlation, ranging from minus one to one. Negative values indicate decreasing trends and positive values denote increasing trends.
Species distribution modeling (SDM) framework (Elith and Leathwick, 2009) was applied to generate seamless distribution estimation of the biomass of Fucus in the Estonian marine waters. SDMs are numerical tools that relate in situ observations of species occurrence or abundance with environmental estimates using mathematical models. These models are then used to predict the occurrence or abundance of a species in a larger spatial extent enabling to produce seamless maps. A modeling algorithm boosted regression trees (BRT) was used to model the spatial distribution of the biomass of F. vesiculosus. BRT is a non-parametric machine-learning method that can handle non-linear interactions between predictor variables. It combines the strength of two algorithms: regression trees and boosting. BRT was chosen as it has been shown to have high predictive performance in many previous studies (e.g. Elith et al., 2006; Hill et al., 2014; Herkül et al., 2016; Valavi et al., 2021). The BRT model was trained using a learning rate of 0.005 and a maximum tree complexity of five. These settings were selected based on recommendations made by Elith et al. (2008). The model was trained in a stepwise manner by adding trees iteratively. Ten-fold cross-validation was applied at each iteration to test if the model’s predictive accuracy increased with the addition of trees. Iterations were stopped when no further improvement of the model was detected. Cross-validation based Pearson correlation coefficient (r) of the model of the final iteration was used to report the prediction accuracy of the BRT model. The final model that was further used for predictions, was fitted using 100% of the data.
Biomass of F. vesiculosus (g m-2 dry weight) in the selected sampling sites (n = 1469) was used as a response variable in the BRT model. The values of the 15 environmental variables (Table 1) served as predictor variables. The values of all predictor variables in each sampling site together with the biomass values of F. vesiculosus formed the model training data table. The final model was then used to generate spatial distribution prediction of biomass of F. vesiculosus. Predictions were calculated for each grid point in the prediction dataset (n = 4 249 989) covering the whole Estonian marine area from the coastline to the depth of 20 m with 50 m rectangular equispaced grid. Deeper areas were removed from the prediction grid for practical reasons as Fucus never occurs deeper than 15 m. The values of all environmental variables were available for each grid point in the prediction dataset. Based on the predicted biomass values in all grid points, the total standing stock biomass (dry weight) of Fucus in the Estonian marine area was calculated.
R programming language version 4.1.3 (R Core Team, 2022) in the development environment RStudio (RStudio Team 2021) was used for data preparation, analysis, and modeling. In addition to the base R functionality, the following packages were used: raster (Hijmans, 2022) for handling spatial raster data, sf (Pebesma, 2018) for handling spatial vector data, gbm (Greenwell et al., 2020) and dismo (Hijmans et al., 2022) for fitting BRT models, tidyverse (Wickham et al., 2019) for tabular data manipulation and plotting. ArcGIS Pro software (ESRI, 2023) was used to generate map layouts.
2.5 Macroalgae sample collection and pre-treatment
The collection of macroalgal specimens for experimentation occurred during the summer season. The species Ectocarpus siliculosus was selected for study as it is representative of numerous fast growing, opportunistic, filamentous brown algal species and is one of two species dominant within Estonian coastal waters. The site selected for sampling consisted of complex three-dimensional rocky habitat interspersed with shallow sandy patches, typical of Northern Baltic temperate macroalgae habitat (59.55019° N, 24.86928° E). Individual macroalgae specimens were collected for incubation via wading to depths not greater than 1.5 meters and no shallower than 0.2 meters. E. siliculosus biomass was collected and stored in 20-L clear plastic bins containing site water with free-flowing air providing aeration and water motion. The collected individuals were transported to the laboratory of the Estonian Marine Institute and stored in a climate-controlled room set to the ambient seawater temperature of the sample site for a period of 24 h for the macroalgae individuals to acclimatize. Prior to experimentation, individuals were gently cleaned in order to remove any epiphytic algae or animals. Experimentation consisted of two phases: (1) the measurement of DOC production and (2) the assimilation of DOC by heterotrophic marine bacteria.
2.6 DOC release
The release of DOC by E. siliculosus was quantified in the same manner as Hall et al. (2022), but replacing the filtered seawater with artificial seawater as a medium to reduce the potential influence natural bacterial communities had and to reduce the detection of background DOC. The experiment consisted of 10 biological replicates comprised of ~35 g of wet weight material which were incubated in separate 4-L acid washed glass chambers. The experiment consisted of two treatments, each comprising of five replicates. The first treatment consisted of saturating light designed to stimulate photosynthesis (PAR measured using Li-Cor light meter), and the second treatment consisted of total darkness. The incubations were conducted for 12 hours in a temperature-controlled environment reflecting the seawater temperature at the time of sample collection. Each incubation chamber received aeration and water motion from free-flowing bubblers. 50 ml water samples were taken at 0, 3, 6, 9, and 12-hour intervals from the incubation chambers using a sterile plastic lure lock tipped syringe equipped with 0.45-micron G/F sterile syringe filters (Minisart). These samples were transferred to individual 50-ml acid-washed glass bottles and promptly frozen for subsequent analysis. At the conclusion of the incubation period, the algal material was retained to obtain dry weights and the water medium retained to conduct lability testing. By standardizing algae biomass to dry weight, production rates can be compared with other DOC release trials. The measurement of dissolved organic carbon (DOC) in the seawater samples were performed using high-temperature catalytic oxidation as outlined by Sugimura and Suzuki (1988). A total organic carbon analyzer (Shimadzu Corp.) featuring a platinum catalyst was employed to determine the concentration and reported as micrograms per liter.
2.7 Bioassay of bacterial DOC utilization
The consumption of E. siliculosus derived DOC by heterotrophic bacteria was evaluated using seawater from the DOC release trial. A total of 10 microcosm incubations were prepared by distributing 180 milliliters of retained macroalgae seawater from each replicate into separate acid-washed glass vessels. Each microcosm received a 20 mL inoculation of seawater containing ambient bacteria from the sample site. To prevent nutrient limitations, the microcosms were supplemented with 16.43 mL of NaNO3 (1.06 mmol l−1), 10.53 mL of KH2PO4 (0.077 mmol l−1), and 0.78 mL of NH4Cl (0.172 mmol l−1). The microcosms were placed on a shaker table to induce turbulent motion and incubated in a light-restricted, temperature-controlled room for period of 120 hours. At the conclusion of the incubation, DOC water samples were taken and analyzed in the same manner as described previously.
2.8 Statistics
Statistical analyses were carried out using the statistical software JMP software (Version 16.2.0) and R (Version 3.6.0). DOC release was assessed using repeated measures analysis of variance (ANOVA) for the factor of treatment (light/dark) with the repeated measure being time. Shapiro Wilkes test was used to test for normality and was not violated. The assumed sphericity was tested using Mauchly’s sphericity test of which the assumptions were met. Post-hoc testing was conducted using Tukey HSD (Table 2). Changes in DOC concentration released under light and dark conditions after the 120-h incubation period was tested using Pair-wise analysis. The significance level for all statistical tests was set at 5% (α = 0.05).
3 Results
3.1 Macrophytobenthos
Analysis of the macrophytobenthos time series using Mann-Kendall test revealed several statistically significant (p < 0.05) trends. An upward trend in opportunistic algae was identified in the Eru, Liu, and Tallinn transects. Pylaiella and Ectocarpus exhibited an increasing trend in Eru but a decreasing trend in the Kõiguste transect. No statistically significant trends were discerned in the Küdema transect. Fucus displayed no discernible trends in any of the transects (Figure 3).
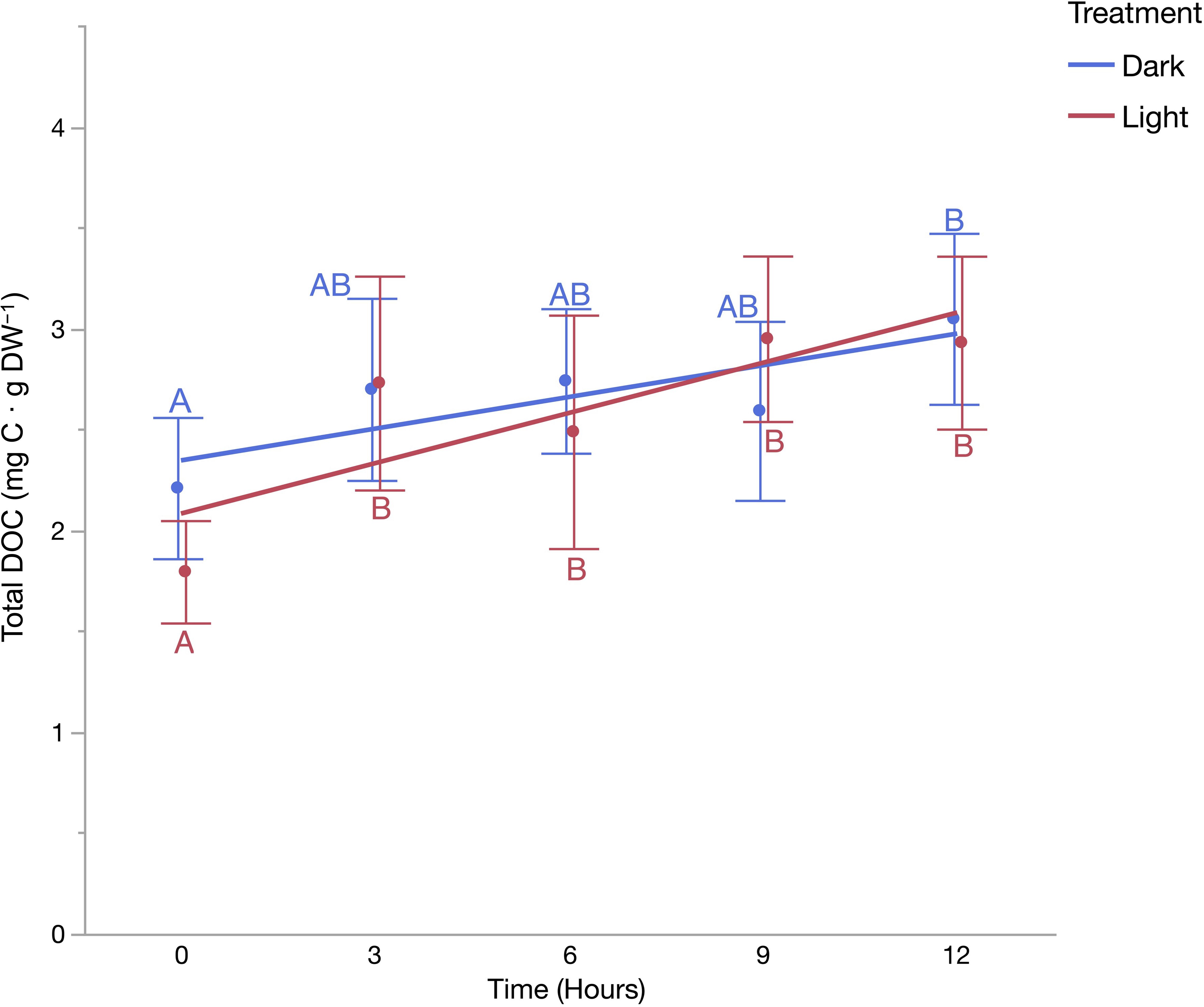
Figure 3 Total dissolved organic carbon concentration per milligram of Ecotcarpus siliculosus dry weight material over a 12-h period under light (red) and dark (blue) treatments. Different letters indicate significantly different DOC concentrations for each treatment (mean ± 1 SE, n = 5) (Tukey’s HSD, a = 0.05).
The distribution of biomass of Fucus spp., as predicted by the BRT model, is shown on Figure 4. The Pearson correlation value for the BRT model, determined through 10-fold cross-validation, was 0.61. Based on the spatial prediction of the distribution of biomass, the standing stock biomass of Fucus spp. within Estonian marine waters was estimated to be ca. 150 000 tonnes (dry weight).
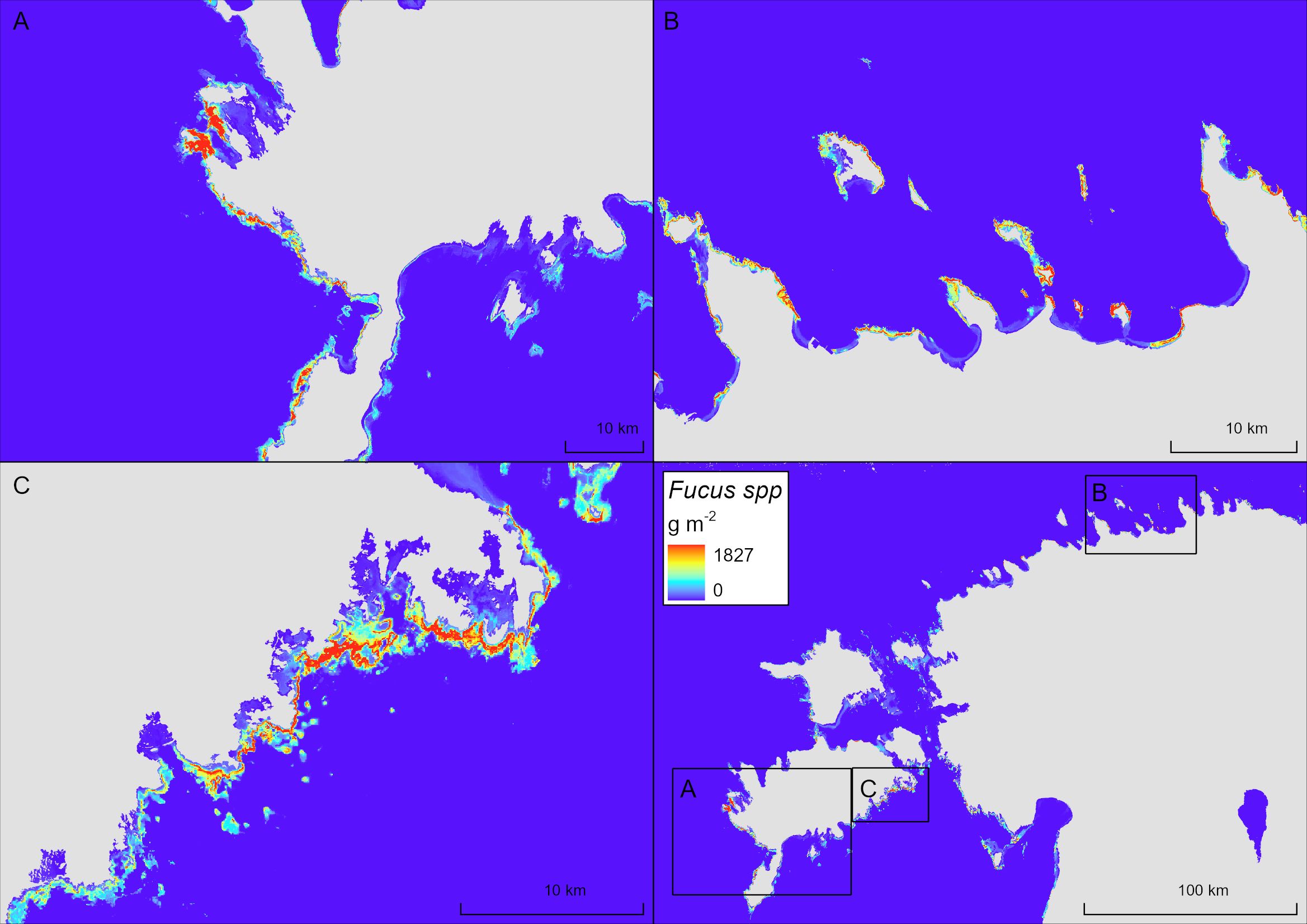
Figure 4 Distribution of biomass (g m-2 dry weight) of Fucus spp. based on BRT modeling. Three zoomed-in areas (A–C) are shown to illustrate small scale distribution patterns.
3.2 E. siliculosus DOC release and consumption
Mean E. siliculosus DOC concentration incubated under both light and dark treatments increased significantly from the starting concentration relative to the final concentration at the conclusion of the 12-hour incubation. In total, E. siliculosus released a mean value of 1.14 mg C per gram of dry weight material for the light treatment within the 12-h incubation period, achieving a production rate of 0.095 mg C · g DW−1 · h−1. In contrast, the dark treatment released 0.84 mg C · g DW−1 achieving a production rate of 0.070 mg C · g DW−1 · h−1. The degree to which released DOC products were consumed by heterotrophic bacteria were assessed over a 120-hour time course (Figure 5). Initial mean DOC concentration produced by E. siliculosus under the light treatment decreased significantly after 120 h from 8.42 mg C · l−1 to 6.00 mg C · l−1, representing a decrease of 28.7% for the light treatment, and 7.2 mg C · l−1 to 5.86 mg C · l−1, representing an 18.6% decrease for the dark treatment (Figure 6).
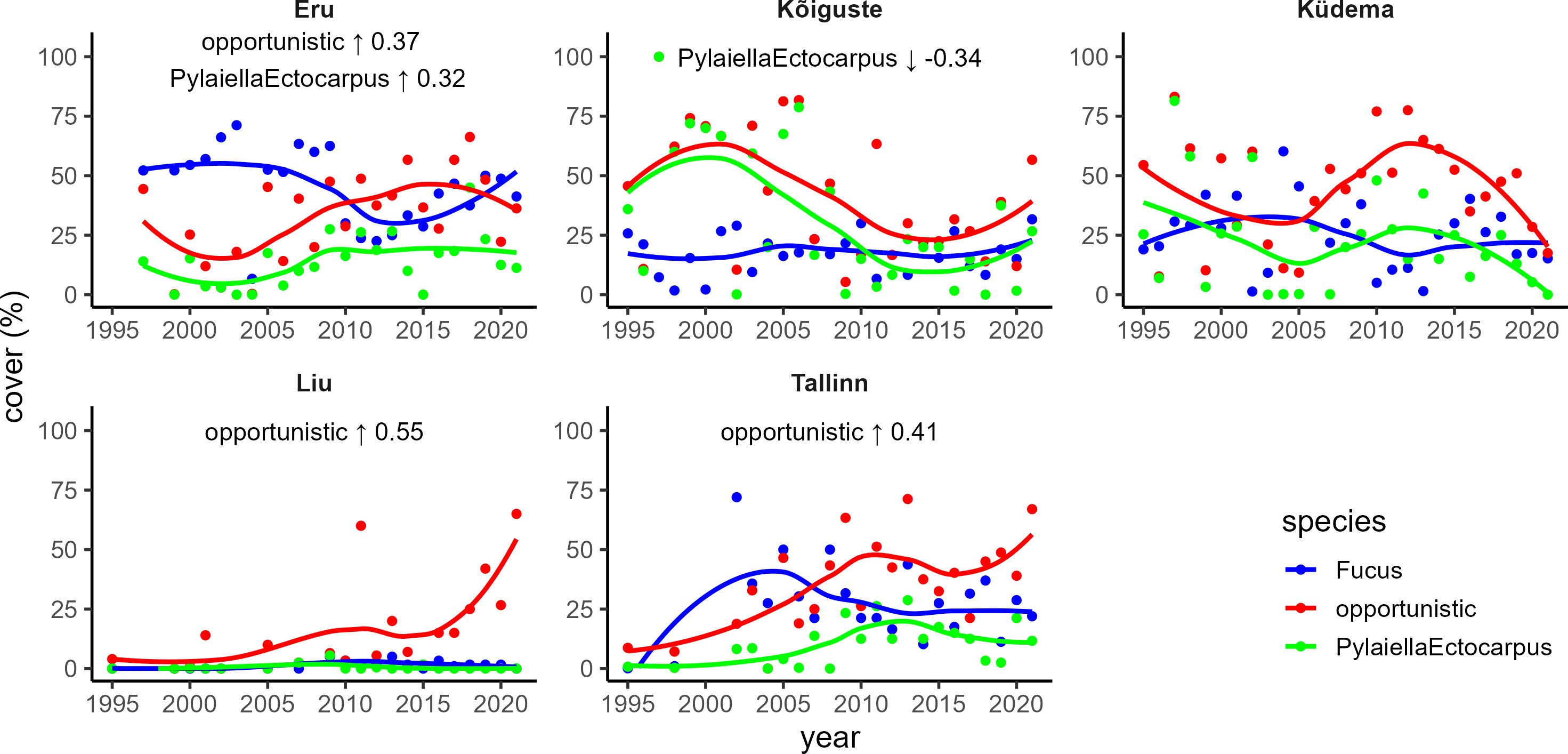
Figure 5 Time series of macrophyte cover. Statistically significant (p < 0.05) trends based on Mann-Kendall test are marked in the upper part of the plots: arrow – direction of the trend; number – τ coefficient.
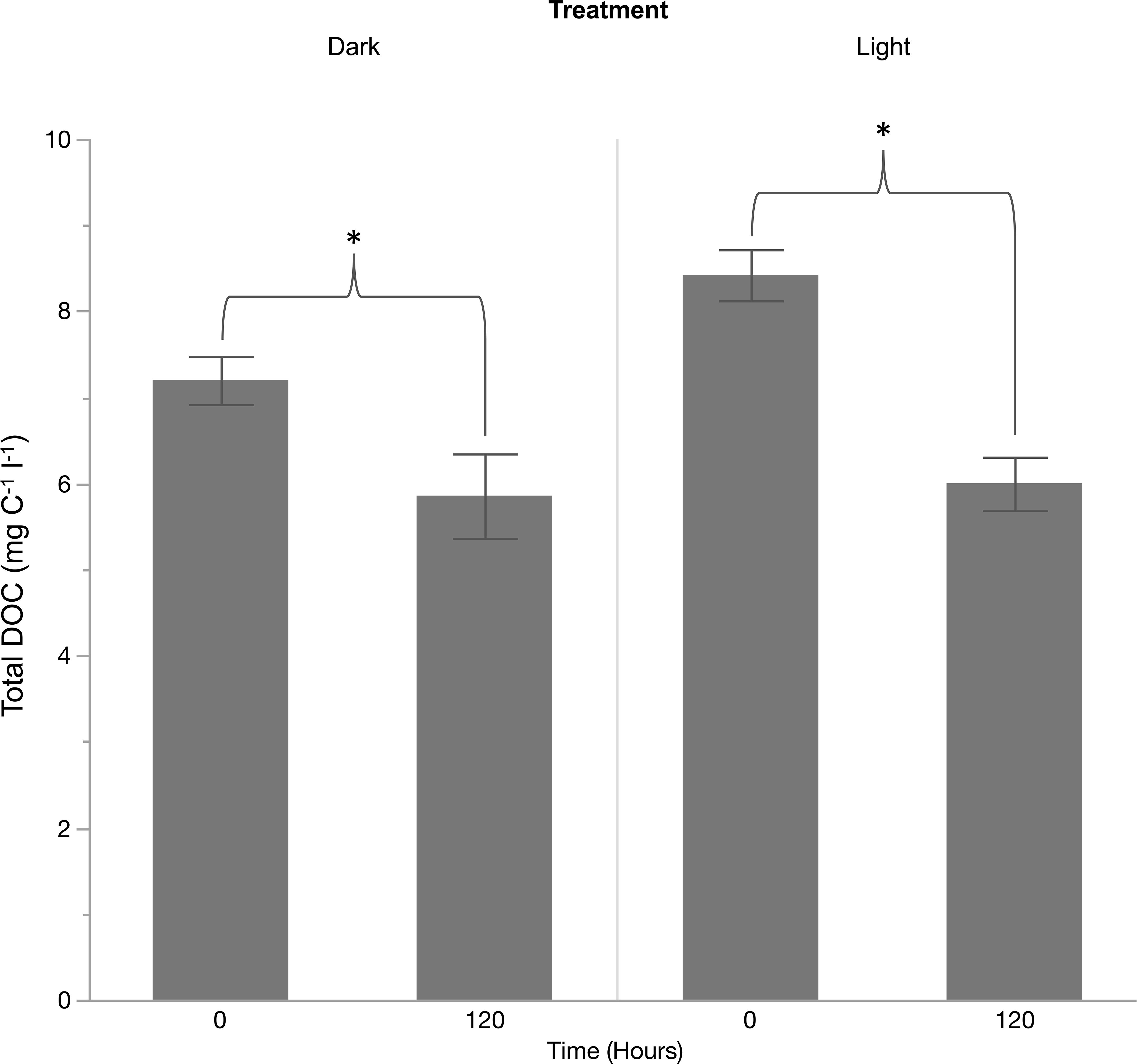
Figure 6 Consumption of total dissolved organic carbon released by Ecotcarpus siliculosus under light and dark treatments after a period of 120 h measured as milligrams of carbon per liter. * = significant difference (<0.05).
4 Discussion
During the latter half of the 20th century profound shifts in the phytobenthic communities of the Baltic Sea have been observed, primarily attributed to eutrophication (Evaleens Maarse et al., 2020; Kautsky et al., 1986; Eriksson et al., 1998; Rönnberg and Mathiesen, 1998; Kovtun et al., 2009; Eveleens Maarse et al., 2020). An analysis of phytobenthos coverage time series of years the 1995-2021 revealed several distinct patterns. Firstly, and in contrast to earlier historical patterns, Fucus spp. did not exhibit statistically significant trends (Figure 5). These observations suggest that the broader loss of F. vesiculosus in Estonian coastal waters, and to a larger extent the Baltic Sea, have reached their conclusion under current eutrophic conditions. However, point source pollution may still drive local decline further, as observed at the ‘Tallinn’ site, with pollutants from the Tallinn Municipality and Pirita river likely constituting a significant and localized stressor for the populations in that area (Erm et al., 2014; Maharjan et al., 2016).
Vahteri and Vuorinen (2016) examined the decline of F. vesiculosus in the Northern Baltic based on diver surveys from 1993-1997. They concluded that F. vesiculosus populations in the region have declined sharply, and that the species is no longer capable of actively competing for living space and as such is struggling to persist. This finding is supported by Rinne and Salovius-Laurén (2020), which examined the status of Fucus spp. within the neighboring Finnish marine area using a more recent data set but also concluded the groups status to be poor. Despite substantial efforts to curtail nutrient loading in the Baltic Sea by all coastal nations since the 1980s, which have succeeded in reducing water column nutrient loads, the issue of eutrophication persists and continues being problematic. The outcome of such initiatives indicate a decline in nitrogen inputs of approximately 30% since 1995, whereas phosphorus inputs, following a notable decrease of 25% between 1995 and 2000, have since maintained a relatively stable level in the western Baltic region (Kuss et al., 2020). Other research has demonstrated that, in the majority of Baltic Sea regions, the total nitrogen (TN) pool in the water column increased from the 1970s until the early 1990s, at which point it stabilized and, in many instances, it has either remained constant or commenced a decline during the early 2000s (Lønborg and Markager, 2021). Additionally, further reductions in TN pollution are scheduled for implementation, with specific reduction targets assigned to the Baltic Sea coastal nations under the framework of the Baltic Sea Action Plan (Wulff et al., 2014). This study provides evidence that reductions in the main nutrient drivers for eutrophication in the Baltic Sea may have resulted in a stabilization of F. vesiculosus beds, perhaps representing the first steps in the recovery of this important habitat.
With F. vesiculosus having declined throughout the Baltic Sea, other opportunistic species have filled the newly available ecological niche. Analysis of the biomass and coverage time series data revealed brown turfing algae (E. siliculosus and P. littoralis) as well as other opportunistic species have both increased in abundance across the majority of the measured sites, including Tallinn, Eru and Liu. Only Kõiguste showed a decrease in brown turfing algae and opportunistic species. The observed trends confirm a predicted rise in the abundance of these species with filamentous brown algae and other opportunistic species supplanting the previously F. vesiculosus dominated system via higher growth rates and the ability to respond more rapidly to newly available substrate (Kiirikki, 1996a; Kiirikki and Blomster 1996; Bonsdorff et al., 1997). Whilst many aspects of F. vesiculosus loss have been intensely studied including, none have evaluated how this loss/shift will alter carbon flow in marine systems as a consequence of changes in DOC release.
From the analysis of the biomass and coverage time series data, carbon flow derived from released photosynthetic products by macroalgae has likely been altered on two levels. Firstly, changes in biomass and species composition have altered the total amount of DOC released on a per area basis. And secondly, the relative ‘quality’ of the released products has likely changed in terms of its lability or refractories. Based on the incubations conducted here, it is confirmed that E. siliculosus releases dissolved organic products into the water column, under both photosynthetic (light) and non-photosynthetic (dark) conditions (Figure 5). Further, the rate of release under the light treatment was 30.3% higher than that of the dark treatment implicating photosynthesis as process that enhances E. siliculosus carbon release. As discussed in the 2021 review by Paine et al., DOC release is mainly hypothesized to be a consequence of either passive cell diffusion, whereby low weight molecular compounds passively ‘leak’ from the macroalgae’s cell membranes leading to a consistent loss of DOC, or as a factor of photosynthate storage (stoichiometric overflow); whereby under photosynthetic conditions photosynthate that cannot be efficiently stored or consumed is released as a means to avoid cell damage (Hatcher et al., 1977; Fogg, 1983; Bjørrisen, 1988; Møller et al., 2003; Saba et al., 2011; Agusti and Duarte, 2013; Thornton, 2014; Livanou et al., 2019). The DOC release pattern observed here suggests that both of these factors influence the release of DOC by E. siliculosus, aligning with the release patterns observed in other filamentous macroalgae species in analogous studies investigating DOC release (Paine et al., 2021). To ensure comparability and minimize variation in DOC release rates, our experimental conditions were aligned as closely as possible with those of Hall et al. (2022). Baltic Sea F. vesiculosus produces labile DOC under photosynthetic conditions at a rate 2.84 (0.27 mg C·g DW−1·h−1) times greater than that of E. siliculosus (Hall et al., 2022). In contrast to E. siliculosus, F. vesiculosus does not appear to release DOC passively under non photosynthetic conditions (Hall et al., 2022). Nevertheless, the total release of E. siliculosus would still not exceed that of F. vesiculosus after a 24-hour light/dark cycle (assuming; 12 hours dark, 12 hours saturating light) with the cumulative release of E. siliculosus at 1.98 mg C·g DW−1 compared to F. vesiculosus releasing 3.24 mg C·g DW−1 (constituting 48.28% more DOC in total). As coastal DOC supply hinges on the given light regime and the time of year, it could lead to one species contributing a greater amount of DOC per unit of biomass than the other. Within the Estonian context, a region that has a long winter period of low light and slow growth followed by high growth summer long light summer period, it could be assumed that F. vesiculosus dominated communities produce an outsized proportion of DOC particularly during the summer when biomass and growth is high relative to that of E. siliculosus. It is therefore concluded that the decline of F. vesiculosus and its supplanting by E. siliculosus and other similar brown turfing algae, could have reduced the total quantity of DOC released into the Baltic Sea coastal system. Such findings are consistent with other studies on community shifts in phytobenthic ecosystems, such as seagrass meadows dominated by Cymodocea nodosa. Jiménez-Ramos et al. (2022) highlighted the role of C. nodosa in carbon metabolism and dissolved organic carbon (DOC) fluxes, noting its substantial production of both labile and recalcitrant DOC, predominantly during winter and summer, respectively. In contrast, areas invaded by the alga Halimeda incrassata showed reduced DOC production and a shift towards DOC consumption in winter. These observations underscore the potential impact of invasive algae on altering carbon dynamics and function within coastal ecosystems.
A given species DOC release metrics in isolation do not allow for the proper assessment of the broader ecological implications of this release as the factor of DOC consumption by heterotrophic bacteria and the subsequent remineralization or sequestration of these products must also be evaluated in conjunction. Results from the 120-hour microcosm experiment used to assess the short-time DOC use by bacteria as a proxy of the lability of the released E. siliculosus products showed a significant decrease in DOC concentration at its conclusion for both the light and dark treatments, indicating E. siliculosus DOC is at a minimum partially labile (Figure 6). As labile DOC is almost instantly acted upon by water column microbes it can be stated that 28.7% of DOC released by E. siliculosus under photosynthetic conditions and 18.6% of DOC released under non-photosynthetic conditions is highly labile and is readily up taken by heterotopic bacteria acting to enhance the microbial loop. Over longer time scales, a substantial portion of the remaining DOC will likely be consumed, as chemical and photodegradation act to increase the lability of the products. It should be noted that measuring DOC for 120 hours may not fully capture the complete consumption dynamics of DOC, only allowing for speculation regarding its potential lability. Information on the true recalcitrant level of DOC requires long-term monitoring periods to comprehensively understand DOC consumption dynamics (Xie et al., 2024; Yamuza-Magdaleno et al., 2024). When, compared to F. vesiculosus DOC consumption measured to be 20.7% after 120 hours, a remarkably similar value to the ones reported here for E. siliculosus. However, and with total DOC release rates in mind, a shift from F. vesiculosus dominated habitats to one of brown turfing algae typified by E. siliculosus, likely acts to weaken the microbial loop.
The underlying reasons for the pattern of DOC consumption reported here can be attributed to the chemical properties of the released DOC and/or the bacterial community’s ability to remineralize these products. A reduction in the release of labile carbon products into the water column has implications for carbon cycling and energy flux to higher trophic level organisms. In the water column, heterotrophic bacteria play a pivotal role by utilizing up to 50% of the dissolved organic matter (DOM) released by primary producers and it is this bacterial biomass, that in turn, serves as a food source for flagellates and other higher-level organisms (Azam et al., 1983; Azam and Malfatti, 2007; Cherrier et al., 1996). This dynamic process is essential for the energy flow within the aquatic food web, as it converts biologically inaccessible DOM into biologically available biomass (Azam et al., 1983; Cherrier et al., 1996; Kirchman, 1990; Pomeroy et al., 2007). Therefore, it can be stated that the loss and decline of F. vesiculosus in the Baltic Sea has likely resulted in a decrease in an important source of labile DOC for which heterotrophic water column bacteria utilize and assimilate into biomass particularly during the summer growth period. In essence, the replacement of F. vesiculosus by filamentous brown alga has acted to modify the efficiency of Baltic Sea food webs by reducing carbon flow from primary producer to higher order trophic levels via the microbial loop.
4.1 Estimate of total Estonian F. vesiculosus release
Variations in the dissolved organic carbon (DOC) release rates between F. vesiculosus and E. siliculosus signify that any alterations between these two species will impact coastal carbon flow, primarily by reducing DOC release from F. vesiculosus. The complexity of the system makes estimations of total carbon release highly difficult, but approximations can be made. Utilizing the boosted regression tree model, we estimate that the Estonian maritime area contains a standing stock biomass of 150,000 tonnes dry weight of F. vesiculosus. This standing stock estimate is within same order of magnitude as early estimates (Estonian Marine Institute, 1999). Assuming all factors remain constant and maximal photosynthetic activity is attained, we can project that in the F. vesiculosus would release a potential maximum of 40.5 tonnes of DOC per hour. This estimation is derived by applying the measured release rate of 0.27 mg C·g DW−1·h−1 to the modeled 150,000 tonnes of F. vesiculosus dry weight. Alternatively, this would translate to an annual release of approximately 7,391.25 tonnes of DOC, given the assumption of saturating light for 50% of the year. Such a number would almost certainly never be achieved for numerous reasons, the first and foremost being that never at one time would the entire population of F. vesiculosus ever simultaneously be under saturating light conditions for reasons such as self-shading and sub optimal water clarity. However, exploring these values is important as approximations allows us to assess the potential significance this source of DOC has in the relevant carbon budgets relative to that of other values.
5 Summary statement
Our current knowledge regarding the rate and fate of dissolved organic matter released by macroalgae is still inadequately understood. This knowledge gap presents a significant challenge when it comes to establishing carbon budgets for coastal marine systems. The findings from this study underscore that shifts in macrophytobenthic communities are likely to have a substantial impact on carbon flow in coastal ecosystems, potentially reshaping the structure of marine food webs or altering its dynamics as a carbon sink. This research emphasizes the significance of DOC in marine food webs and highlights the considerable amount of carbon that is released but often goes unaccounted for. It is therefore imperative to assess individual species with a substantial presence and biomass for both their DOC release rate and the labile nature of the DOC. These findings should be complemented by in-depth investigations into shifts in community structure, particularly in the context of our rapidly changing world. Such work holds important implications for the expanding body of literature that now acknowledges seaweeds as valuable ecosystems for the marine carbon cycle and ocean carbon sequestration. Currently, much research lacks consideration for the lability of DOC and the pathways it takes, highlighting limitations in developing comprehensive coastal marine carbon budgets. By advancing our understanding of the pathways through which macroalgae derived carbon moves, we can develop a more cohesive understanding of coastal food webs, blue carbon and coastal ecology.
Data availability statement
The raw data supporting the conclusions of this article will be made available by the authors, without undue reservation.
Author contributions
JH: Conceptualization, Data curation, Formal analysis, Funding acquisition, Investigation, Methodology, Project administration, Resources, Validation, Writing – original draft, Writing – review & editing. KH: Data curation, Formal analysis, Investigation, Software, Supervision, Writing – original draft, Writing – review & editing. FB: Conceptualization, Methodology, Supervision, Writing – review & editing. CH: Conceptualization, Supervision, Writing – review & editing. GM: Conceptualization, Funding acquisition, Resources, Supervision, Writing – review & editing.
Funding
The author(s) declare financial support was received for the research, authorship, and/or publication of this article. Funding for this study was provided through the basic research and infrastructure fund of the Estonian Marine Institute, University of Tartu, Estonia.
Acknowledgments
The authors would like to thank all of those who have aided us in the completion of this work. Specifically, we would like to thank Trude Taevere and Kaire Kaljurand for assistance with algal sample collection, and Irma Lubiene, Klaipeda University, Marine Research Institute, Lithuania, for assistance in DOC sample analysis. Additionally, we would like to thank all of those who have contributed to the macrobenthos database of the Estonian Marine Institute, University of Tartu. We also wish to thank the reviewers of this manuscript who provided insightful and constructive feedback.
Conflict of interest
The authors declare that the research was conducted in the absence of any commercial or financial relationships that could be construed as a potential conflict of interest.
The author(s) declared that they were an editorial board member of Frontiers, at the time of submission. This had no impact on the peer review process and the final decision.
Publisher’s note
All claims expressed in this article are solely those of the authors and do not necessarily represent those of their affiliated organizations, or those of the publisher, the editors and the reviewers. Any product that may be evaluated in this article, or claim that may be made by its manufacturer, is not guaranteed or endorsed by the publisher.
References
Abdullah M. I., Fredriksen S. (2004). Production, respiration and exudation of dissolved organic matter by the kelp Laminaria hyperborea along the west coast of Norway. J. Mar. Biol. Assoc. United Kingdom 84 (5), 887–894. doi: 10.1017/S002531540401015Xh
Agusti S., Duarte C. M. (2013). Phytoplankton lysis predicts dissolved organic carbon release in marine plankton communities. Biogeosciences 10, 1259–1264. doi: 10.5194/bg-10-1259-2013
Airoldi L., Balata D., Beck M. W. (2008). The gray zone: relationships between habitat loss and marine diversity and their applications in conservation. J. Exp. Mar. Biol. Ecol. 366, 8–15. doi: 10.1016/j.jembe.2008.07.034
Alheit J., Möllmann C., Dutz J., Kornilovs G., Loewe P., Mohrholz V., et al. (2005). Synchronous ecological regime shifts in the central Baltic and the North Sea in the late 1980s. ICES J. Mar. Sci. 62, 1205–1215. doi: 10.1016/j.icesjms.2005.04.024
Andersen J. H., Carstensen J., Conley D. J., Dromph K., Fleming-Lehtinen V., Gustafsson B. G., et al. (2017). Long-term temporal and spatial trends in eutrophication status of the Baltic Sea. Biol. Rev. 92, 135–149. doi: 10.1111/brv.12221
Andersson A., Meier H. M., Ripszam M., Rowe O., Wikner J., Haglund P., et al. (2015). Projected future climate change and Baltic Sea ecosystem management. Ambio 44, 345–356. doi: 10.1007/s13280-015-0654-8
Azam F., Fenchel T., Field J. G., Gray J. S., Meyerreil L. A., Thingstad F. (1983). The ecological role of water-column microbes in the sea. Mar. Ecol. Prog. Ser. 10, 257–263. doi: 10.1126/science.1175309
Azam F., Malfatti F. (2007). Microbial structuring of marine ecosystems. Nat. Rev. Microbiol. 5, 782–791. doi: 10.1038/nrmicro1747
Bendtsen J., Gustafsson K. E., Söderkvist J., Hansen J. L. (2009). Ventilation of bottom water in the North Sea–Baltic Sea transition zone. J. Mar. Syst. 75, 138–149. doi: 10.1016/j.jmarsys.2008.08.006
Berger R., Bergström L., Granéli E., Kautsky L. (2004). “How does eutrophication affect different life stages of Fucus vesiculosus in the Baltic Sea?—a conceptual model,” in Biology of the baltic sea: proceedings of the 17th BMB symposium, 25–29 november 2001 (Springer Netherlands, Stockholm, Sweden), 243–248. doi: 10.1007/978-94-017-0920-0_22
Berger R., Henriksson E., Kautsky L., Malm T. (2003). Effects of filamentous algae and deposited matter on the survival of Fucus vesiculosus L. germlings in the Baltic Sea. Aquat. Ecol. 37, 1–11. doi: 10.1023/A:1022136900630
Björkqvist J. V., Lukas I., Alari V., van Vledder G. P., Hulst S., Pettersson H., et al. (2018). Comparing a 41-year model hindcast with decades of wave measurements from the Baltic Sea. Ocean Eng. 152, 57–71. doi: 10.1016/j.oceaneng.2018.01.048
Bjørrisen P. K. (1988). Phytoplankton exudation of organic matter: Why do healthy cells do it? 1. Limnology Oceanog. 33, 151–154. doi: 10.4319/lo.1988.33.1.0151
Bonsdorff E., Blomqvist E. M., Mattila J., Norkko A. (1997). Long-term changes and coastal eutrophication. Examples from the Aland Islands and the Archipelago Sea, northern Baltic Sea. Oceanolica Acta 20, 319–329.
Cherrier J., Bauer J. E., Druffel E. R. M. (1996). Utilization and turnover of labile dissolved organic matter by bacterial heterotrophs in eastern north pacific surface waters. Mar. Ecol. Prog. Ser. 139, 267–279. doi: 10.3354/meps139267
Cederwall H. (1990). Biological effects of eutrophication in the Baltic Sea, particularly the coastal zone. Ambio 19, 109–112.
Dolliver J., O’Connor N. (2022). Whole system analysis is required to determine the fate of macroalgal carbon: A systematic review. J. Phycol. 58, 364–376. doi: 10.1111/jpy.13251
Duarte L., Rossi F., Docal C., Viejo R. M. (2015). Effects of alga Fucus serratus decline on benthic assemblages and trophic linkages at its retreating southern range edge. Mar. Ecol. Prog. Ser. 527, 87–103. doi: 10.3354/meps11248
Edwards M. S., Connell S. D. (2012). “Competition, a major factor structuring seaweed communities,” in Seaweed biology: Novel insights into ecophysiology, ecology and utilization (Springer Berlin Heidelberg, Berlin, Heidelberg), 135–156. doi: 10.1007/978-3-642-28451-9_7
Egea L. G., Jiménez-Ramos R., Romera-Castillo C., Casal-Porras I., Bonet-Melià P., Yamuza-Magdaleno A., et al. (2023). Effect of marine heat waves on carbon metabolism, optical characterization, and bioavailability of dissolved organic carbon in coastal vegetated communities. Limnology Oceanog. 68, 467–482. doi: 10.1002/lno.12286
Elith J., Leathwick J. R., Hastie T. (2008). A working guide to boosted regression trees. J. Anim. Ecol. 77, 802–813. doi: 10.1111/j.1365-2656.2008.01390.x
Elith J., Leathwick J. R. (2009). Species distribution models: ecological explanation and prediction across space and time. Annu. Rev. Ecol. Evol. Systemat. 40, 677–697. doi: 10.1146/annurev.ecolsys.110308.120159
Elith J., Graham C. H., Anderson R. P., Dudík M., S. F., Guisan A., et al. (2006). Novel methods improve prediction of species’ distributions from occurrence data. Ecography 29, 129–151. doi: 10.1111/j.2006.0906-7590.04596.x
Elmgren R. (2001). Understanding human impact on the Baltic ecosystem: changing views in recent decades. AMBIO: A J. Hum. Environ. 30, 222–231. doi: 10.1579/0044-7447-30.4.222
Eriksson B. K., Johansson G., Snoeijs P. (1998). Long-term changes in the sublittoral zonation of brown algae in the southern Bothnian Sea. Eur. J. Phycol. 33, 241–249. doi: 10.1080/09670269810001736743
Erm A., Maljutenko I., Buschmann F., Suhhova I., Meerits A. (2014). “Stormwater impact on the coastal area of the Tallinn Bay,” in 2014 IEEE/OES Baltic International Symposium (BALTIC) . 1–15 (IEEE). doi: 10.1109/BALTIC.2014.6887867
ESRI (2023). ArcGIS pro: version 3.1.2 (Redlands, CA, USA: Environmental Systems Research Institute).
Estonian Marine Institute (1999). Assessment of the amount of commercially useable bladder wrack in Estonian coastal sea (in Estonian), Report. 39.
Eveleens Maarse F., Salovius-Laurén S., Snickars M. (2020). Long-term changes in the phytobenthos of the southern Åland Islands, northern Baltic Sea. Nordic J. Bot. 38. doi: 10.1111/njb.02751
Fogg G. E. (1983). The ecological significance of extracellular products of phytoplankton photosynthesis. Bot. Mar. 26, 3–14. doi: 10.1515/botm.1983.26.1.3
Fonselius S., Valderrama J. (2003). One hundred years of hydrographic measurements in the Baltic Sea. J. Sea Res. 49, 229–241. doi: 10.1016/S1385-1101(03)00035-2
Greenwell B., Boehmke B., Cunningham J., GBM Developers (2020). gbm: Generalized Boosted Regression Models. R package version 2.1.8. Available online at: https://CRAN.R-project.org/package=gbm.
Gustafsson B. G., Schenk F., Blenckner T., Eilola K., Meier H. M., Müller-Karulis B., et al. (2012). Reconstructing the development of Baltic Sea eutrophication 1850–2006. Ambio 41, 534–548. doi: 10.1007/s13280-012-0318-x
Haage P. (1975). Quantitative Investigations of the Baltic Fucus Belt Macrofauna: Quantitative seasonal fluctuations (Contrib Askö Lab, Stockholm University), 1–88.
Haage P. (1976). Quantitative investigations of the Baltic Fucus belt macrofauna, 3: Seasonal variation in biomass, reproduction and population dynamics of the dominant taxa Vol. 10 (Contrib Askö Lab, Stockholm University), 1–84.
Hall J. R., Albert G., Twigg I. M., Baltar F., Hepburn C. D., Martin G. (2022). The production of dissolved organic carbon by macroalgae and its consumption by marine bacteria: Implications for coastal ecosystems. Front. Mar. Sci. 9. doi: 10.3389/fmars.2022.934229
Hatcher B. G., Chapman A. O., Mann K. H. (1977). An annual carbon budget for the kelp Laminaria longicruris. Mar. Biol. 44, 85–96. doi: 10.1007/BF00386909
HELCOM Balance (2007). Baltic Sea Management — Nature Conservation and Sustainable Development of the Ecosystem through Spatial Planning project. Available online at: https://balance-eu.org/publications/index.html.
Herkül K., Lauringson V., Kotta J. (2016). Specialization among amphipods: the invasive Gammarus tigrinus has narrower niche space compared to native gammarids. Ecosphere 7, e01306. doi: 10.1002/ecs2.1306
Hijmans R. J. (2022). raster: Geographic Data Analysis and Modeling. R package version 3.5-29. Available online at: https://CRAN.R-project.org/package=raster.
Hijmans R. J., Phillips S., Leathwick J., Elith J. (2022). dismo: Species Distribution Modeling. R package version 1.3-8. Available online at: https://CRAN.R-project.org/package=dismo.
Hill N. A., Lucieer V., Barrett N. S., Anderson T. J., Williams S. B. (2014). Filling the gaps: Predicting the distribution of temperate reef biota using high resolution biological and acoustic data. Estuar. Coast. Shelf Sci. 147, 137–147. doi: 10.1016/j.ecss.2014.05.019
Hurd C. L., Harrison P. J., Bischof K., Lobban C. S. (2014). Seaweed Ecology and Physiology, 2nd Edn (Cambridge: Cambridge University Press). doi: 10.1017/CBO9781139192637
Isæus M. (2004). Factors structuring Fucus communities at open and complex coastlines in the Baltic Sea (Ph.D. thesis, Stockholm University, Stockholm: Botaniska institutionen).
Isæus M., Malm T., Persson S., Svensson A. (2004). Effects of filamentous algae and sediment on recruitment and survival of Fucus serratus (Phaeophyceae) juveniles in the eutrophic Baltic Sea. Eur. J. Phycol. 39, 301–307. doi: 10.1080/09670260410001714732
Jiménez-Ramos R., Tomàs F., Reynés X., Romera-Castillo C., Pérez-Lloréns J. L., Egea L. G. (2022). Carbon metabolism and bioavailability of dissolved organic carbon (DOC) fluxes in seagrass communities are altered under the presence of the tropical invasive alga Halimeda incrassata. Sci. Tot. Environ. 839, 156325. doi: 10.1016/j.scitotenv.2022.156325
Kautsky H., Martin G., Snoeijs-Leijonmalm P. (2017). “The phytobenthic zone,” in Biological Oceanography of the Baltic Sea, eds Snoeijs-Leijonmalm P., Schubert H., Radziejewska T. (Dordrecht: Springer), 387–455. doi: 10.1007/978-94-007-0668-2_11
Kautsky L., Kautsky H. (1989). Algal species diversity and dominance along gradients of stress and disturbance in marine environments. Vegetatio 83, 259–267. doi: 10.1007/BF00031698
Kautsky L., Kautsky N. (2000). The baltic sea, including bothnian sea and bothnian bay. Seas at millennium: an Environ. Eval. 1, 121–133.
Kautsky L., Qvarfordt S., Schagerström E. (2019). Fucus vesiculosus adapted to a life in the Baltic Sea: impacts on recruitment, growth, re-establishment and restoration. Botanica Marina 62, 17–30. doi: 10.1515/bot-2018-0026
Kautsky N., Kautsky H., Kautsky U., Waern M. (1986). Decreased depth penetration of Fucus vesiculosus (L.) since the 1940’s indicates eutrophication of the Baltic Sea. Mar. Ecol. Prog. Ser. 28, 1–8. doi: 10.3354/meps028001
Kiirikki M., Blomster J. (1996). Wind induced upwelling as a possible explanation for mass occurrences of epiphytic Ectocarpus siliculosus (Phaeophyta) in the northern Baltic Proper. Mar. Biol. 127, 353–358. doi: 10.1007/BF00942120
Kiirikki M. (1996a). Dynamics of macroalgal vegetation in the northern Baltic Sea – evaluating the effects of weather and eutrophication. Walter Andrée Nottbeck Foundation Sci. Rep. 12, 1–15.
Kiirikki M. (1996b). Mechanisms affecting macroalgal zonation in the northern Baltic Sea. Eur. J. Phycol. 31, 225–232. doi: 10.1080/09670269600651421
Kirchman D. (1990). Limitation of bacterial growth by dissolved organic matter in the subarctic Pacific. Mar. Ecol. Prog. Ser. 62, 47–54. doi: 10.3354/meps062047
Korpinen S., Meski L., Andersen J. H., Laamanen M. (2012). Human pressures and their potential impact on the Baltic Sea ecosystem. Ecol. Indic. 15, 105–114. doi: 10.1016/j.ecolind.2011.09.023
Kovtun A., Torn K., Kotta J. (2009). Long-term changes in a northern Baltic macrophyte community. Estonian J. Ecol. 58, 270–285. doi: 10.3176/eco.2009.4.03
Kuss J., Nausch G., Engelke C., Weber M. V., Lutterbeck H., Naumann M., et al. (2020). Changes of nutrient concentrations in the western Baltic Sea in the transition between inner coastal waters and the central basins: Time series from 1995 to 2016 with source analysis. Front. Earth Sci. 8. doi: 10.3389/feart.2020.00106
Lehtinen K.-J., Notini M., Mattsson J., Landner L. (1988). Disappearance of bladder-wrack (Fucus vesiculosus L.) in the baltic sea: relation to pulp-mill chlorate. Ambio 17, 387–393.
Livanou E., Lagaria A., Psarra S., Lika K. (2019). A DEB-based approach of modeling dissolved organic matter release by phytoplankton. J. Sea Res. 143, 140–151. doi: 10.1016/j.seares.2018.07.016
Lønborg C., Markager S. (2021). Nitrogen in the Baltic Sea: long-term trends, a budget and decadal time lags in responses to declining inputs. Estuar. Coast. Shelf Sci. 261, 107529. doi: 10.1016/j.ecss.2021.107529
Maharjan B., Pachel K., Loigu E. (2016). Trends in urban storm water quality in Tallinn and influences from stormflow and baseflow. J. Water Secur. (JWS) 2. doi: 10.15544/jws.2016.001
Malm T., Isæus M. (2005). “Distribution of macroalgal communities in the central Baltic Sea,” in Annales botanici fennici (Finnish Zoological and Botanical Publishing Board), 257–266. Available at: https://www.jstor.org/stable/23727375.
Mattsson E. (2019). Importance of Fucus vesiculosus (bladderwrack) for coastal fish communities in the Baltic Sea (Stockholm University).
Møller E. F., Thor P., Nielsen T. G. (2003). Production of DOC by Calanus finmarchicus, C. glacialis and C. hyperboreus through sloppy feeding and leakage from fecal pellets. Mar. Ecol. Prog. Ser. 262, 185–191. doi: 10.3354/meps262185
Meier H. E. M., Andersson H. C., Eilola K., Gustafsson B. G., Kuznetsov I., Müller-Karulis B., et al. (2011a). Hypoxia in future climates: a model ensemble study for the Baltic Sea (Geophys). doi: 10.1029/2011GL049929
Meier H. E. M., Eilola K., Almroth E. (2011b). Climate-related changes in marine ecosystems simulated with a three-dimensional coupled biogeochemical-physical model of the Baltic Sea. Clim. Res. 48, 31–55. doi: 10.3354/cr00968
Neumann T. (2010). Climate-change effects on the Baltic Sea ecosystem: a model study. J. Mar. Syst. 8, 213–224. doi: 10.1016/j.jmarsys.2009.12.001
Neumann T., Eilola K., Gustafsson B., Muller-Karulis B., Kuznetsov I., Meier H. E. M., et al. (2012). Extremes of temperature, oxygen and blooms in the Baltic sea in a changing climate. Ambio 41, 574–585. doi: 10.1007/s13280-012-0321-2
Niiranen S., Yletyinen J., Tomczak M. T., Blenckner T., Hjerne O., MacKenzie B. R., et al (2013). Combined effects of global climate change and regional ecosystem drivers on an exploited marine food web. Glob. Change Biol. 19, 3327–3342. doi: 10.1111/gcb.12309
Nilsson J., Engkvist R., Persson L. E. (2004). Long-term decline and recent recovery of Fucus populations along the rocky shores of southeast Sweden, Baltic Sea. Aquat. Ecol. 38, 587–598. doi: 10.1007/s10452-004-5665-7
Olson A. M., Lubchenco J. (1990). Competition in seaweeds: linking plant traits to competitive outcomes. J. Phycol. 26, 1–6. doi: 10.1111/j.0022-3646.1990.00001.x
Omstedt A., Edman M., Claremar B., Frodin P., Gustafsson E., Humborg C., et al. (2012). Future changes in the Baltic Sea acid–base (pH) and oxygen balances. Tellus B Chem. Phys. Meteorol. 64, 19586. doi: 10.3402/tellusb.v64i0.19586
Österblom H., Hansson S., Larsson U., Hjerne O., Wulff F., Elmgren R., et al. (2007). Human-induced trophic cascades and ecological regime shifts in the Baltic Sea. Ecosystems 10, 877–889. doi: 10.1007/s10021-007-9069-0
Paine E. R., Schmid M., Boyd P. W., Diaz-Pulido G., Hurd C. L. (2021). Rate and fate of dissolved organic carbon release by seaweeds: a missing link in the coastal ocean carbon cycle. J. Phycol. 57, 1375–1391. doi: 10.1111/jpy.13198
Pebesma E. J. (2018). Simple features for R: standardized support for spatial vector data. R J. 10, 439. doi: 10.32614/RJ-2018-009
Pomeroy L. R., leB. Williams P. J., Azam F., Hobbie J. E. (2007). The microbial loop. Oceanography 20, 28–33. doi: 10.5670/oceanog
Rajasilta M., Mankki J., Ranta-Aho K., Vuorinen I. (1999). Littoral fish communities in the Archipelago Sea, SW Finland: a preliminary study of changes over 20 years. Hydrobiologia 393, 253–259. doi: 10.1023/A:1003523308309
R Core Team (2022). R: A language and environment for statistical computing (Vienna, Austria: R Foundation for Statistical Computing). Available at: https://www.R-project.org/.
Rinne H., Blanc J. F., Salo T., Nordström M. C., Salmela N., Salovius-Laurén S. (2022). Variation in Fucus vesiculosus associated fauna along a eutrophication gradient. Estuar. Coast. Shelf Sci. 275, 107976. doi: 10.1016/j.ecss.2022.107976
Rinne H., Salovius-Laurén S. (2020). The status of brown macroalgae Fucus spp. and its relation to environmental variation in the Finnish marine area, northern Baltic Sea. Ambio 49, 118–129. doi: 10.1007/s13280-019-01175-0
Rönnberg O., Mathiesen L. (1998). Long-term changes in the marine macroalgae of Lågskär, Åland Sea (N Baltic). Nordic J. Bot. 18, 379–384. doi: 10.1111/j.1756-1051.1998.tb01894.x
RStudio Team (2021). RStudio: integrated development environment for R (Boston, MA, USA: RStudio, PBC). Available at: http://www.rstudio.com/.
Saba G. K., Steinberg D. K., Bronk D. A. (2011). The relative importance of sloppy feeding, excretion, and fecal pellet leaching in the release of dissolved carbon and nitrogen by Acartia tonsa copepods. J. Exp. Mar. Biol. Ecol. 404, 47–56. doi: 10.1016/j.jembe.2011.04.013
Schramm W. (1996). “The Baltic Sea and its transition zones,” in Marine benthic vegetation: recent changes and the effects of eutrophication (Springer Berlin Heidelberg, Berlin, Heidelberg), 131–163. doi: 10.1007/978-3-642-61398-2_6
Snoeijs-Leijonmalm P. (2017). “Patterns of biodiversity,”, in Biological Oceanography of the Baltic Sea, eds Snoeijs-Leijonmalm P., Schubert H., Radziejewska T. (Dordrecht: Springer). doi: 10.1007/978-94-007-0668-2-4
Snoeijs-Leijonmalm P., Andrén E. (2017). “Why is the Baltic Sea so special to live in?,” in Biological Oceanography of the Baltic Sea, eds Snoeijs-Leijonmalm P., Schubert H., Radziejewska T. (Dordrecht: Springer), 23–84. doi: 10.1007/978-94-007-0668-2_2
Sugimura Y., Suzuki Y. (1988). A high-temperature catalytic oxidation method for the determination of non-volatile dissolved organic carbon in seawater by direct injection of a liquid sample. Mar. Chem. 24 (2), 105–131. doi: 10.1016/0304-4203(88)90043-6
Thornton D. C. (2014). Dissolved organic matter (DOM) release by phytoplankton in the contemporary and future ocean. Eur. J. Phycol. 49, 20–46. doi: 10.1080/09670262.2013.875596
Torn K., Krause-Jensen D., Martin G. (2006). Present and past depth distribution of bladderwrack (Fucus vesiculosus) in the Baltic Sea. Aquat. Bot. 84, 53–62. doi: 10.1016/j.aquabot.2005.07.011
Torn K., Martin G., Rostin L. (2014). Testing and development of different metrics and indexes describing submerged aquatic vegetation for the assessment of the ecological status of semi-enclosed coastal water bodies in the NE Baltic Sea. Estonian J. Ecol. 63, 262. doi: 10.3176/eco.2014.4.05
Uiboupin R., Pärn O. (2018). Mereala planeeringu alusuuring: jääolude analüüs ja kaartide koostamine (in Estonian) (Tallinn University of Technology).
Vahteri P., Vuorinen I. (2016). Continued decline of the bladderwrack, Fucus vesiculosus, in the Archipelago Sea, northern Baltic proper. Boreal Environ. Res. 21, 373–386.
Valavi R., Guillera-Arroita G., Lahoz-Monfort J. J., Elith J. (2021). Predictive performance of presence-only species distribution models: a benchmark study with reproducible code. Ecol. Monogr. 92, e01486. doi: 10.1002/ecm.1486
van der Meijs F., Isaeus M. (2020). Wave exposure calculations for the Gulf of Finland. AquaBiota Rep. 13, 1–26.
Vogt H., Schramm W. (1991). Conspicuous decline of Fucus in Kiel Bay (Western Baltic): What are the causes? Mar. Ecol. Prog. series. Oldendorf 69, 189–194. doi: 10.3354/meps069189
Wallentinus I. (1991). “The baltic sea gradient,” in Intertidal and littoral ecosystems, vol. 24 . Eds. Mathieson A. C., Nienhuis P. H. (Elsevier, Amsterdam), 83–108.
Wickham H., Averick M., Bryan J., Chang W., McGowan L. D. A., François R., et al. (2019). Welcome to the tidyverse. J. Open Source software 4, 1686. doi: 10.21105/joss.01686
Wikström S. A., Kautsky L. (2007). Structure and diversity of invertebrate communities in the presence and absence of canopy-forming Fucus vesiculosus in the Baltic Sea. Estuar. Coast. Shelf Sci. 72, 168–176. doi: 10.1016/j.ecss.2006.10.009
Wulff F., Humborg C., Andersen H. E., Blicher-Mathiesen G., Czajkowski M., Elofsson K., et al. (2014). Reduction of Baltic Sea nutrient inputs and allocation of abatement costs within the Baltic Sea catchment. Ambio 43, 11–25. doi: 10.1007/s13280-013-0484-5
Xie Y., Su J., Shao K., Hu T., Ming H., Shi T., et al. (2024). Long-term response of the microbial community to the degradation of DOC released from Undaria pinnatifida. Mar. Environ. Res. 194, 106313. doi: 10.1016/j.marenvres.2023.106313
Keywords: seaweed, microbial loop, coastal ecosystem function, blue carbon, ocean carbon biogeochemical cycle, eutrophication, algae physiology, community shift
Citation: Hall JR, Herkül K, Baltar F, Hepburn CD and Martin G (2024) Shifts in macroalgae composition alters carbon flow in Coastal Baltic Sea ecosystems: implications for dissolved organic carbon bioavailability and flux. Front. Mar. Sci. 11:1384165. doi: 10.3389/fmars.2024.1384165
Received: 08 February 2024; Accepted: 01 July 2024;
Published: 19 July 2024.
Edited by:
Alberto Basset, University of Salento, ItalyCopyright © 2024 Hall, Herkül, Baltar, Hepburn and Martin. This is an open-access article distributed under the terms of the Creative Commons Attribution License (CC BY). The use, distribution or reproduction in other forums is permitted, provided the original author(s) and the copyright owner(s) are credited and that the original publication in this journal is cited, in accordance with accepted academic practice. No use, distribution or reproduction is permitted which does not comply with these terms.
*Correspondence: Jack R. Hall, amFjay5oYWxsQHV0LmVl