- Environmental Epigenetics and Genetics Group, Department of Horticulture, Martin-Gatton College of Agriculture, Food and Environment, University of Kentucky, Lexington, KY, United States
Climate change is expected to increase the occurrence of extreme environmental conditions. Viticulture, as agriculture in general, is highly dependent on climatic conditions, not only for yield but also for fruit quality, which is the most important factor affecting produce value at the farm-gate. This demands the development of novel plant breeding techniques that will lead to the accelerated production of more resilient grape varieties, as conventional breeding programs for perennials are often prolonged. Recent research has suggested that environmental conditions can activate a memory of stress that could result in a primed response to subsequent stress events. This is a process capable of increasing plant’s resilience to abiotic stimuli, allowing plants to better adapt to extreme environmental conditions. While the effect of priming has been observed in many plants, the underlying mechanisms are puzzling and seldom studied in perennial crops. A large body of research has been developed in the last decade linking response to stress, stress priming, and memory of stress with epigenetic mechanisms. This understanding of plant epigenetics has opened the door to the application of epigenetics to crop improvement, such as the use of epigenetic breeding for the generation of more resilient crops. Perennial crop agriculture in general, and viticulture in particular, would benefit from more in-depth knowledge on epigenetic memory of stress.
1 Introduction
Wine grapes are considered the most important fruit crop in the world in terms of production and economic importance (Alston and Sambucci, 2019). It has been reported that there are nearly 8 million hectares of vineyards worldwide and the global annual production have reached approximately 90 million tons (http://faostat.fao.org). In the United States alone, which ranks fourth in the volume of wine production behind Italy, France, and Spain (Stevenson, 2005), wine, grapes, and grape products contribute $276 billion to the economy in 2022 (https://wineamerica.org/economic-impact-study/2022-american-wine-industry-methodology/). The importance of grape cultivation for wine production, however, goes beyond its bare contribution to the economy. Wine consumption has moved from a source of nutrition to a cultural phenomenon with a large tourist industry associated with it. For this reason, the wine industry has helped fix local populations in rural areas by diversifying the job markets in such regions (https://wineamerica.org/economic-impact-study/2022-american-wine-industry-methodology/). The majority of cultivated grapes belong to Vitis vinifera subsp. vinifera; but the cultivation of other Vitis sensu stricto species, including hybrids, and the related subgenus Muscadinia are also common in regions where the climate and/or disease pressure are not suitable for V. vinifera (Hickey et al., 2019), despite climate change. Early breeding efforts done in the Southern United States using American grape species, led to the production of many modern cultivars such as the muscadine and bunch grapes, that can still be found today (Stafne et al., 2015), and more recently to the modern rootstocks being used worldwide (Ollat et al., 2016; Santos et al., 2020). However, many bunch grape hybrids derived from V. vinifera, and other non-resistant species are susceptible to Pierce’s disease (PD). Thus, the breeding focus has mainly been on producing resistant cultivars, such as those released by the University of California-Davis (https://ucdavis.app.box.com/s/dte06een1orc7uzfucccwdaqwepqp90y).
Climate change is expected to severely affect the major viticultural regions of the world by reducing the areas where most grapevine cultivars can be cultivated economically, due to an increase in abiotic stress pressure (Diffenbaugh et al., 2011), and in the incidence of pests and diseases (Gullino et al., 2018). The long domestication and breeding history of V. vinifera in particular, for wine and fresh and dried fruit consumption has led to desirable traits such as berry color, sugar content, and berry size (Aradhya et al., 2003; Myles et al., 2011; Zhou et al., 2017). Traditional breeding attempts to cross wild non-vinifera species with V. vinifera has generated some resistant/tolerant genotypes (Morales-Cruz et al., 2021; Ruiz-García et al., 2021), although these genotypes can be compromised by negatively perceived flavors, prominently in wine production (Liu et al., 2015; Yang et al., 2016). While this is only true in F1 crosses between vinifera and wild species, due to the protracted nature of traditional grapevine breeding programs, the future success of the wine industry will require the utilization of new breeding technologies for the development of novel varieties better suited to the climatic conditions predicted under the scenario of climate change (Töpfer and Trapp, 2022).
Plants have acquired many adaptation strategies, activated and controlled by changes in gene expression and nuclear organization (Budak et al., 2015) to cope with ever-changing environmental conditions. Progress in plant molecular biology has enable the identification of major stress response pathways, leading into a deeper understanding of the plant responses that constitute such strategies (Hirayama and Shinozaki, 2010). The availability of the complete grapevine genome sequence has allowed the identification and characterization of various stress-inducible genes, cis-regulatory elements and transcription factors (Jaillon et al., 2007). More recent studies have shown that epigenetic mechanisms, some with the potential to be inherited, play an important role in plant response to environmental stress (Miryeganeh, 2021). Although the current knowledge on the role of epigenetic regulation in response to the environment in the grapevine is still limited, the demonstration of the involvement of epigenetic mechanisms in model plants has led to an increased interest in their role in crop resilience to environmental stress (Varotto et al., 2020).
Here we summarize the current knowledge on, environmental factors that affect grape and wine qualities, transcriptomic approaches that have been utilized to study the effect of environmental factors on grapevine, and finally recent studies focusing on epigenetic mechanisms, particularly those involved in plant response to environmental changes, which have led to proposing epigenetic breeding as a new tool for the generation of climate resilient grapevines.
2 Factors affecting grape and wine quality
Fruit and wine quality are determined by the interaction between the cultivar(s) (including the interaction between rootstock and scion), the local environmental conditions (climate, topography, soil, etc.), and the viticultural and enological practices implemented to grow the grapes and produce the wine (Van Leeuwen et al., 2004). Such interaction has been traditionally termed terroir (Seguin, 1986) (Figure 1).
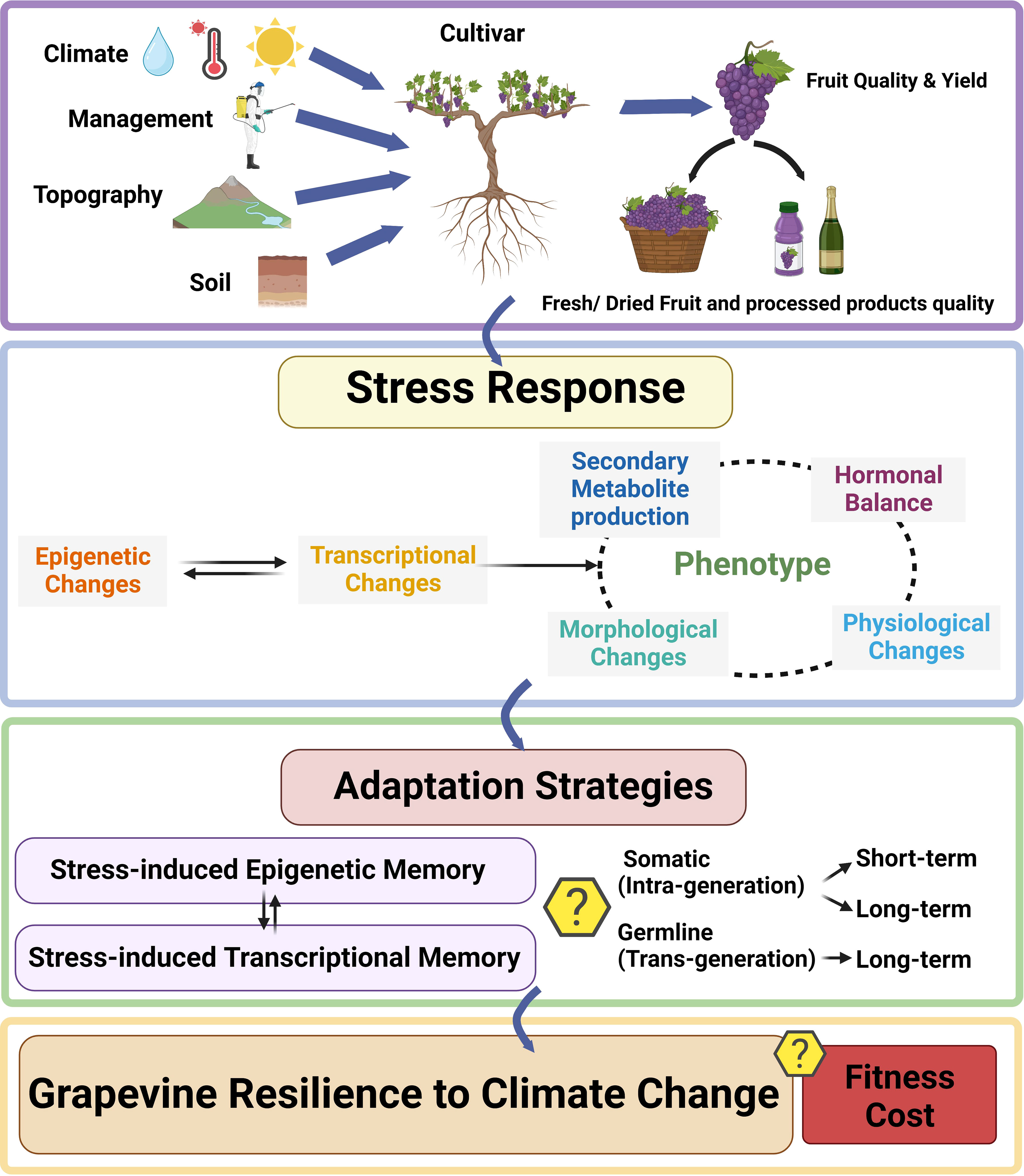
Figure 1 Grapevine response to the environment. Top panel represents the different factors (climate, soil, topography, management, and planted rootstock/scion genotype) contributing to grapevine growth and development, berry composition, fruit quality and yield. Middle panel represents the stress response triggered at a molecular level leading to a change in phenotype. Panel 3 represents the adaptation strategies employed by grapevine to establish different types of memory, leading to grapevine resilience to environmental stress. Question marks in panels 3 and 4 denote the current limited knowledge about the establishment and maintenance of epigenetic memory in grapevine, and of the potential deleterious fitness cost of epigenetic priming.
2.1 Climate
Among those factors, climate conditions determine the suitability to grow a particular variety, as the most desirable composition of grapes requires certain climatic conditions (Gladstones, 1992). Common climate factors that are important for grape and wine quality are temperature, radiation, and rainfall (Romero et al., 2016) (Figure 1).
2.1.1 Temperature
Temperature is widely accepted to affect grapevine phenology, vegetative cycles, grape quality, and the timing of grape harvest (Winkler, 1974; Jones and Alves, 2012; Cook and Wolkovich, 2016). Photosynthesis is among the first physiological functions to be directly affected by temperature variations, as it is reduced before other symptoms appear when the temperature rises above an optimum limit, which differs among species (Luo et al., 2011; Xiao et al., 2017; Sharma et al., 2020). Most of the physiological processes decline at below 10°C and heat acclimation mechanisms are activated at temperatures over 35°C (Bernardo et al., 2018). At extreme high temperature, i.e., above 40°C, the photosynthetic apparatus is disrupted (Venios et al., 2020). Elevated temperature during berry growth and maturation largely impacts size and composition (Carbonell-Bejerano et al., 2013). More specifically, higher temperatures lower the acidity and increase the sugar content of berries, resulting in unbalanced wines with higher alcohol content and deprived of freshness and aromatic complexity (Martínez-Lüscher et al., 2016). More sugar and less organic acids in berries, and altered secondary metabolites composition, mainly in aroma precursors, have been observed with increased temperature (Van Leeuwen and Destrac-Irvine, 2017). It has been reported that berry size and weight are reduced at temperatures above 30°C (Hale and Buttrose, 1974), while metabolic processes and sugar accumulation may completely stop (Downey et al., 2006). In addition, despite tartaric acid being relatively stable with regards to temperature, malic acid levels are tightly dependent on maturity and temperature, as higher temperature leads to lower malic acid content (Santos et al., 2020). In general, elevated temperature is associated with increased potassium levels and decreased total acidity, and thus is associated with increased pH levels (De Orduna, 2010). Higher temperatures also modify the biosynthesis and accumulation of flavonoids in berries. Temperatures above 30°C lead to lower anthocyanin synthesis (Spayd et al., 2002; Tarara et al., 2008), which can be completely and irreversibly inhibited at 37°C (Yang et al., 2018). This suggests that in warm climates, grapevine berries can suffer from the inhibition of anthocyanin formation and hence reduce grape color (Downey et al., 2006). Conversely, low temperature leads to an increase in anthocyanin accumulation and total soluble solids (Mori et al., 2005). It is important to consider, however, the degree to which high temperature affects the anthocyanin to sugar ratio is believed to be cultivar dependent, due to different sensitivity of berry anthocyanin to critical ranges of temperature (Fernandes de Oliveira et al., 2015).
2.1.2 Radiation
Solar radiation, along with temperature and thermal amplitude are highly influential for grape phenological stages (Zapata et al., 2017). In general, higher levels of radiation are likely accompanied by higher temperatures, which leads to a higher photosynthetic rate and increased metabolic activity (Jackson and Lombard, 1993; Arias et al., 2022). Additionally, photosynthesis can be inhibited when the radiation intensity is too high and accompanied by elevated temperatures (Iacono and Sommer, 1996). The natural intensity of ultraviolet (UV) radiation can alter grapevine physiology (Núñez-Olivera et al., 2006), and change grape production and composition (Kolb et al., 2003; Berli et al., 2011). In general, Ultraviolet B (UV-B) radiation at high-altitude can reduce shoot length, leaf expansion, photosynthesis and stomatal conductance; and augmented leaf thickness, photoprotective pigments, proline accumulation and the antioxidant capacity of leaves (Berli et al., 2013; Martínez-Lüscher et al., 2016). Moreover, UV-B is associated with flavonols accumulation in berries (Gregan et al., 2012; Marfil et al., 2019). However, increased levels of UV-B can have a potentially damaging effect on grapevine leaves and berries (Kolb et al., 2003), e.g., total amino acid concentration and total carotenoid pigment content both reduced by exposure to ambient level UV-B (Schultz, 2000). Conversely, UV-C radiation induces the synthesis of stilbene, via the phenylpropanoid pathway (Bais et al., 2000). Stilbenes are important for their defensive roles in plants, pharmacological value and beneficial effects on human health (Vannozzi et al., 2012; Kiselev et al., 2019).
2.1.3 Water
Rainfall or water available for grape production is a crucial factor that affects grapevine characteristics. Water management can be used to manipulate vine and berry attributes (Smart and Coombe, 1983), as changes in water status at critical phenological stages have a direct effect on grape composition and quality attributes by influencing vegetative growth, yield, canopy microclimate, and fruit metabolism (Pellegrino et al., 2005; Van Leeuwen and Seguin, 2006; Ezzahouani et al., 2007). Vine water stress is thought to enhance fruit quality for wine production (Jackson and Lombard, 1993), but it can at the same time reduce berry size and therefore lower yield (Salón et al., 2005). This has been exemplified by studies where water stress reduced the berry size but increased the phenolic compounds, soluble solids, and the berry anthocyanin concentration at harvest (e.g., Deluc et al., 2009; Savoi et al., 2017). However, significant changes in anthocyanin levels under water stress have not been observed in some of the studies, indicating that this response is common but not universal (Bonada et al., 2015; Brillante et al., 2018). Contrasting results have also been reported among studies on the impact of water stress on tannins (e.g., Castellarin et al., 2007; Deluc et al., 2009; Casassa et al., 2015; Savoi et al., 2017). Similar inconsistencies have been observed for stilbene accumulation (e.g., Vezzulli et al., 2007; Deluc et al., 2011; Hochberg et al., 2015). Still, a strong relationship has been observed between improved grape quality and water stress before veraison (Van Leeuwen et al., 2004). Other studies found that in addition to reduced berry size, sugar content and total acidity were also lowered with water stress. Under mild water stress, grape aroma potential was highest in vines, while severe water stress limits such potential (Des Gachons et al., 2005). A recent meta-analysis indicated that sugars and organic acids negatively and positively correlated, respectively, with grapevine stem water potential (Mirás-Avalos and Intrigliolo, 2017). To conclude, it should be important to note that different varieties respond differently to water deficit and that season conditions affect their responses (Herrera et al., 2017; Gambetta et al., 2020).
2.2 Cultivar
The cultivar has a significant impact on berry composition at maturity. A study conducted by Van Leeuwen et al. (2004), found that fruit composition (e.g., malate, sugar, and Potassium content) is especially dependent on the cultivar. Although the same study also showed that the impact of climate and soil was greater than that of cultivars on vine development and berry composition, the impact of cultivars is still a crucial factor to consider. Ripening speed is another crucial factor contributing to fruit composition that varies among cultivars (Costantini et al., 2008). As previously observed, different cultivars also respond to different environmental factors differently. For example, different cultivars respond differently to water stress, where the impact of water stress on anthocyanin accumulation was greater in Shiraz and Cabernet Sauvignon berries (Hochberg et al., 2015), subsequently influencing the yield and the quality of the berry (Dal Santo et al., 2016).
2.3 Soil
Grapevines can be grown on a large variety of soils, and one type of soil might be ideal for vine growth but not ideal for winemaking. For example, vines are vigorous and highly productive in deep, and rich soils, but better wines are generally produced when the vines are cultivated on poor soils (Van Leeuwen and Seguin, 2006). The complex effect of soil on vine and berry composition is due to factors such as vine mineral nutrition, water uptake, rooting depth, and the temperature in the root zone. Among the minerals found within the soil, nitrogen is believed to be one of the most influential regarding vine vigor, yield, and grape maturation (Van Leeuwen and Seguin, 2006). Soil structure and chemistry are believed to influence grapevine composition and wine quality (Mackenzie and Christy, 2005). Analysis of the effects of vine water and nitrogen status, linked to soil type for grafted Cabernet Sauvignon suggested that limited nitrogen uptake is associated with decreased vine vigor, berry weight, and yield, and also with increased sugar, anthocyanin, and tannin accumulation, which consequently increased quality in red wine production (Chone et al., 2001). In addition, Van Leeuwen et al. (2004) found that berry weight is mainly influenced by the soil type, and that grape quality is higher under moderate water stress, especially on clayey soils where water stress occurs early in the season.
Finally, soils contain the richer and more functionally active of all the plant’s microbiota (Rodriguez et al., 2019). It is now well stablished that soil microbial communities provide multiple benefits to plants, including better access to nutrients, enhanced growth, and improved tolerance to stress (Corbin et al., 2020). Moreover, soil microbial communities have also been linked to terroir at a local (Zhou et al., 2021) and global scale (Gobbi et al., 2022).
2.4 Topography
Topography variation is one of the main causes of vineyard variability, such variability can affect the yield (Bramley and Hamilton, 2004), vegetative development (Johnson et al., 2003; Acevedo-Opazo et al., 2008), and grape composition (Bramley, 2005). Different terrain attributes are factors causing topography variations, such as slope, elevation, and aspect/exposure (Yau et al., 2013). Those factors are then impacting soil depth, water holding capacity, air and soil temperature, radiation exposure, and others (Victorino et al., 2017). The elevation is a good example of how multiple agronomically important environmental conditions can be impacted by a single topographic factor. Vineyard elevation has been linked to vigor, as low elevation induced higher vigor vineyards due to higher temperatures (Fraga et al., 2014), while at the same time affect berry metabolomic profiles (Tarr et al., 2013) as elevation can have a profound effect on the UV levels experienced by vines (approximately 1% increase every 70 m gain in altitude) (Xie et al., 2017).
2.5 Management practices
Management practices refer to the idea of human factors at the vineyard level affecting fruit quality, as suggested by Van Leeuwen and Seguin (2006). Different management practices such as canopy management (Dry, 2000), floor management (Tesic et al., 2007; Guerra and Steenwerth, 2012), which includes practices such as soil management (Muganu et al., 2013; Likar et al., 2015), and weed management (Sanguankeo et al., 2009), have been shown to affect grapevine growth, yield, and berry quality traits. For example, the use of cover crops can increase juice soluble solids, anthocyanins, and other phenolic components and decrease acidity and pH (Guerra and Steenwerth, 2012). In addition, mineral composition varies significantly between differently managed vineyards, e.g., increased bioaccumulation of potassium and phosphorus is associated with sustainably managed vineyards (which utilizes biodynamic or organic farming practices to minimize environmental impacts and ensure economic viability), while increased zinc bioaccumulation is associated with conventional vineyards (Likar et al., 2015). This is significant since the soil concentrations of potassium, iron, and copper, organic matter content, and vesicular colonization, strongly affect the mineral composition of the grapes. Moreover, differences in soil management have also been associated with vine growth, bud break time, and total soluble solids and anthocyanin contents (Muganu et al., 2013). The goal of canopy management practices is to optimize sunlight interception, photosynthetic capacity, and fruit microclimate. The combination of these factors has been shown to affect the berry composition of red and white grape cultivars, where the combination of leaf removal and either shoot thinning or cluster thinning resulted in higher total soluble solids and anthocyanin content, and lower malic acid and potassium content (Satisha et al., 2013).
To conclude, the concept of terroirs is dynamic, and will most likely be affected by climate change (Brillante et al., 2020), similar to other agronomical important crops. Environmental variability can be managed by deeper understandings of the vine/environment interactions, and through the application of innovative agriculture techniques designed to make grapevines more resilient to environmental challenges (Brillante et al., 2020).
3 Effects of climate change related stress on grape quality, yield, and wine production
Stress can be classified into biotic and abiotic. Biotic stresses are caused by biological agents such as fungi, bacteria, viruses, and insects, whereas abiotic stresses are caused by physical environmental factors. Common abiotic factors unfavorable for plant growth and crop yield include drought, saline soils and irrigation, heat, and cold. Worldwide, extensive agricultural losses result from heat stress, often in combination with drought (Vogel et al., 2019). It is expected that the effects of combined drought and heat stress will become more severe as the climate continues to warm (Zhao et al., 2017; Raza et al., 2019), as it is predicted that an increase in global temperature of 1.5°C will cause more extremely hot days on land, and an increase in the intensity and frequency of drought and precipitation deficits (IPCC, 2018).
Agriculture is highly dependent on climatic conditions. Climate determines the ability to successfully grow a particular variety and can greatly affect the value of the fruit produced (Jones and Davis, 2000; Jones, 2006; Bai et al., 2022). Grape production is vulnerable to environmental stress as the environmental conditions occurring during one growing season contribute to the quality and yield of the next vintage (Mullins et al., 1992; Edwards and Clingeleffer, 2013; Martínez-Lüscher and Kurtural, 2021). Viticulture is commonly practiced in regions with a Mediterranean climate (Cs climate according to the updated Koppen-Geiger climate classification (Peel et al., 2007)), where the growing season is characterized by low rainfall, the majority occurring in winter, and by high air temperature and evaporative demand (Fraga et al., 2012). In addition to the coastal regions of the Mediterranean Sea, this includes, the West coast of the Iberia Peninsula, the Pacific coast of Chile and the United States, Cape Town region in South Africa, and portions of the West and South Coast of Australia (Peel et al., 2007). Recent studies have shown that temperature rise is highly correlated with an earlier onset of many growth stages in the grapevine (Alikadic et al., 2019). It has been proposed that an increase in ambient temperatures will constitute the primary cause of water shortages for viticulture due to increased evaporative demand (Schultz, 2010), and may eliminate production in many areas (White et al., 2006; Diffenbaugh et al., 2011). On the other hand, temperature rise is not without benefits, Bunting et al. (2021) showed that changes in climate in Michigan (MI), United States has helped the state to overcome the challenge for grape cultivation due to low growing season temperature, short growing seasons, and excessive precipitation. Similarly, Cabré et al. (2016) suggested that Argentina has a great potential for expansion into new suitable vineyards due to climate change. Climate change is also expected to affect plant-pathogen interactions causing severe damage to grapevine and leading to extensive yield and quality losses (Yu et al., 2012; Gullino et al., 2018). The maintenance of stable and high-quality supplies of grapes and derived products will demand the implementation of measures such as relocation of vineyards to northern zones or higher altitude areas with lower average temperature (White et al., 2006) or the development of novel and faster breeding programs.
4 Transcriptomic approaches to understand the response of grapevine to abiotic stress
Studying the regulation of gene expression can provide a deeper understanding of the molecular regulation of the physiological and metabolic mechanisms used by grapevine to respond to various stresses such as elevated temperatures (heat) or drought. Earlier efforts included the use of Expressed Sequence Tags (ESTs), which resulted in the development of a microarray containing a set of 3,200 Unigenes from V. vinifera to study grape development (Terrier et al., 2001; Terrier et al., 2005). The number of unigenes present on the microarray rapidly increased with newer technologies such as the Operon (Camps et al., 2010) or Affymetrix (Deluc et al., 2009) grape arrays. The complete sequence of the grapevine genome became available after the sequencing and assembly of the PN40024 line (Jaillon et al., 2007). With that being available, NimbleGen microarrays were utilized to study grape transcriptome (Pastore et al., 2017). With the advance of technology, full coverage of the grapevine transcriptome was made possible by next-generation sequencing, named RNA-sequencing (Zenoni et al., 2010). Since then, both genome wide-microarrays and RNA-sequencing have been widely used to characterize the response of grapevine to various stress. Some examples include heat (i.e., Rienth et al., 2016), drought (i.e., Berdeja et al., 2015), and UV-B stress (Du Plessis et al., 2017). The high-throughput sequencing technology has been proven useful in revealing potential key stress response genes, which could be highly beneficial for breeding new grape cultivars that can better adapt to the changing environment. Examples of the key genes that have been characterized as playing a role in grapevine stress response, include leafy cotyledon1-like (LEC1) and somatic embryogenesis receptor kinase (SERK) (VvL1L and VvSERK, respectively in grapevine), which are key regulators of grapevine development and stress response (Maillot et al., 2009). Abscisic acid-insensitive 3 (ABI3), a gene that is involved in abscisic acid (ABA) signaling and drought response (Mittal et al., 2014; Rattanakon et al., 2016). Various calcium-dependent protein kinases (CDPKs), such as VaCPK20 and VaCPK29 identified from V. amurensis have been shown to involved in drought and cold tolerance, and to heat and osmotic stresses respectively, when being overexpressed in transgenic grape cell cultures and in Arabidopsis thaliana (Dubrovina et al., 2015; Dubrovina et al., 2017). Several dehydration responsive protein associated genes and transcription factors regulated by ABA, including dehydration responsive element-binding protein1a (DREB1A), have been identified as regulators of stress-responsive genes against drought tolerance (Cardone et al., 2019), while apoptosis related-proteins genes were shown to be involved in the regulation of programmed cell death and defense against biotic stress (Repka, 2006). The exact role and mechanism of action of these genes can vary depending on the type of stress and the grapevine genotypes being studied and that they are often a part of a much more complex stress signaling pathways. Additionally, Zha et al. (2020) used transcriptomic analysis to study grapevine response to heat stress and identified two important genes central to grapevine’s response to heat stress, heat shock factor a2 and a7 (VvHSFA2 and VvHSFA7, respectively). Cochetel et al. (2020) showed that more drought tolerant wild genotypes are more responsive transcriptionally in terms of ABA signaling and biosynthesis than less drought tolerant ones. The authors also identified core genes to drought stress as well as gene clusters and sub-networks that are associated with drought tolerance in grapevine.
The experimental designs for transcriptomic analyses are not without limitations. Rienth et al. (2014) showed that the transcriptome of grapevine plants under heat stress can vary drastically depending on the time of the day the stress is being applied. The results from this study suggested that future grapevine transcriptomic analyses should rely standardized experimental designs. Additionally, the quantitation of the applied stress factor and the physiological impact on the plant should be measured carefully (Berdeja et al., 2015). Moreover, a large body of research has suggested the need to go beyond classical differentially expressed gene (DEG) analysis, more detailed tools and analyses such as weighted gene co-expression network (WGCNA) and cluster analysis. Those will provide more in-depth knowledge on stress response by revealing co-regulated gene modules and potential master switch/hub genes that might be key for abiotic stress responses in plants (Palumbo et al., 2014; Hopper et al., 2016; Cochetel et al., 2017). Moreover, although stress conditions in the natural environment often occur in combination (e.g., heat and drought stress tend to occur simultaneously in grapevine cultivating regions), a majority of grapevine transcriptomic studies deal with only one abiotic stress factor, where such a factor is often applied in controlled or semi-controlled conditions. Therefore, it has been suggested that transcriptomic studies should integrate stress combinations in their experimental design (Gomès et al., 2021). We integrated these recommendations in our most recent global transcriptomic and gene co-expression network analysis to reveal core genes central to grapevine response to combined heat and drought stress (Tan et al., 2023). Interestingly, this work also identified that epigenetic chromatin modifications may play an important role in grapevine responses to combined drought and heat stress through the establishment of an epigenetic memory of stress.
5 Epigenetic mechanisms in the context of plant adaptation to stress
Plants have developed various mechanisms to adapt to daily environmental conditions, and the regulation of gene expression through both transcriptional and post-transcriptional regulation is particularly important to their survival. Among those strategies are a suit of molecular mechanisms studied under the umbrella term of epigenetics. Waddington (1942) first proposed the term epigenetics, referring to the study of the interactions between genes and the environment. The current definition of the term refers to potentially heritable changes in gene function without changes to their underlying DNA sequence (Wu and Morris, 2001) that are usually mediated by three main types of changes: DNA methylation, histone post-translational modifications (PTMs), and the expression of certain small RNAs (sRNAs) (Agarwal et al., 2020).
5.1 DNA methylation
DNA methylation generally refers to the addition of a methyl group to carbon 5 of cytosine bases, thus forming 5-methylcytosine or 5mC. Although other forms of DNA methylation have been detected in plants, including N6-methyladenine (6mA), and 5-hydroxymethylcytosine (5hmC) (Kumar and Mohapatra, 2021), this section will focus on 5mC. The establishment and maintenance of plant cytosine methylation depends on the cytosine sequence context (i.e., CG, CHG, or CHH, H = a nucleotide other than G), and is catalyzed by DNA methyltransferases. CG and CHG methylation is regulated by METHYLTRANSFERASE 1 (MET1) and CHROMOMETHYLASE 3 (CMT3), respectively (Zhang et al., 2018a), while CHH methylation is maintained by either DOMAINS REARRANGED METHYLASE 2 (DRM2) or CHROMOMETHYLASE 2 (CMT2) (Zemach et al., 2013) depending on the genomic region. In general, cytosine methylation impacts genome stability and influences chromatin structure, thus also controlling the accessibility of genetic information (Jin et al., 2011; Bouyer et al., 2017; Zhang et al., 2018a). The effect of cytosine-methylation on gene expression is proposed to be determined by its genic context, i.e., cytosine methylation occurring within the promoter usually act to repress transcription, although in some cases it promotes gene transcription (Zhang et al., 2018a). On the other hand, gene-body methylation and transcription has been observed to be positively associated at some level (Yang et al., 2014), however, its function remains at large (Bewick and Schmitz, 2017).
Studies have examined the potential roles of cytosine methylation in plant response to biotic and abiotic stress factors, including but not limited to heat, cold, drought, salinity, and pathogen infections (e.g., Eichten and Springer, 2015; Liu et al., 2017; Konate et al., 2018). Many early studies have shown that stress not only induces genome-wide cytosine methylation and/or demethylation patterns but also loci specific changes, and that these changes in cytosine methylation may be associated with the transcriptional regulation of genes involved in plant stress response (Khan et al., 2013; Yong-Villalobos et al., 2015; Zhang et al., 2018b).
5.2 Histone post-translational modifications
Histone PTMs, such as phosphorylation, lysine acetylation, arginine and lysine methylation, ubiquitylation, proline isomerization, ADP ribosylation, arginine citrullination, SUMOylation, carbonylation, and, with some controversy, biotinylation, are essential elements of the chromatin signaling pathway (Seet et al., 2006; Arnaudo and Garcia, 2013). Among those, histone acetylation/deacetylation and histone methylation/demethylation are well characterized. Their effect depends on the type of modification and on the histone residues being modified, for example, di-methylation and tri-methylation on lysines 9 and 27 of Histone 3 (H3K9 and H3K27 respectively) result in gene expression repression, compared to the gene transcription activating mono-methylated forms, while acetylation of those residues is associated with transcription activation. Moreover, the repressive transcriptional state of transposable elements and repetitive sequence-enriched heterochromatic regions are maintained by H3K9 monomethylation and dimethylation (H3K9me1 and H3K9me2, respectively) in plants. Heterochromatin regions are also associated with H3K27me1, while the repression found in euchromatin regions is associated with H3K27 trimethylation (H3K27me3) (Liu et al., 2010). The involvement of histone modification in regulating plant responses to stresses by mediating gene expression has been extensively studied. Some examples include the involvement of histone acetyltransferase (HATs), deacetylases (HDACs), and demethylases (HDMs), which play important roles in response to various stress in a variety of plants (e.g., Ueda and Seki, 2020).
5.3 Non-coding RNA-mediated gene regulation
The third main epigenetic mechanism involves two species of RNA molecules, i.e., small-interfering RNAs (siRNAs) and microRNAs (miRNAs), which have been shown to regulate gene expression at transcriptional and post-transcriptional levels (Wei et al., 2017). In general, miRNAs are processed from single-stranded RNA (ssRNA) stem-loop precursors by DICER-LIKE 1 (DCL1) ribonucleases (Axtell, 2013) and when loaded into Argonaute (AGO) proteins to form the RNA-induced silencing complex (RISC), they regulate gene expression post-transcriptionally, by directing mRNA degradation and translational repression (Rogers and Chen, 2013). While siRNAs are processed from double-stranded RNA (dsRNA) precursors and can be further classified into multiple subclasses depending on their size (i.e., 21, 22, or 24 nucleotides (nt) long). The 21-nt and 22-nt siRNAs are associated with mRNA cleavage, while 24-nt siRNAs regulate DNA methylation, with those participating in the RNA-directed DNA methylation (RdDM) pathway being the most abundant (Matzke and Mosher, 2014). Based on the number of nucleotides, those siRNAs either participate in canonical RdDM pathway (24-nt siRNAs) that target transposable elements (TEs) and other repeats to induce DNA methylation and reinforce their transcriptional silencing (Matzke and Mosher, 2014; Du et al., 2015) or participate in noncanonical RdDM pathway (a small fraction of 21-22nt siRNAs) to establish the silencing of novel TEs at new target loci, both transcriptionally and post-transcriptionally (Nuthikattu et al., 2013). The functional outcome of a specific 21-22nt siRNA depends on the associating AGO protein, the association with AGO4, AGO6, and AGO9 will result in a noncanonical RdDM pathway and DNA methylation, while the association with other AGOs will result in post-transcription gene silencing (PTGS) through the cleavage of mRNAs (Matzke and Mosher, 2014; Cuerda-Gil and Slotkin, 2016).
The involvement of miRNA and siRNA in plant stress response by regulating gene expression has been studied extensively. A large number of miRNAs and putative siRNAs such as miRNA156 have been shown to play important roles in stress response in plants (e.g., Sunkar and Zhu, 2004; Ito et al., 2011).
In conclusion, these epigenetic mechanisms are thought to be acting together to coordinate gene activity at the transcriptional level and regulate different cellular processes and responses to environmental stimuli (Bartels et al., 2018) despite having their own regulatory mechanisms.
6 Epigenetics in grapevine
Fortes and Gallusci (2017) proposed grapevine as a model to study epigenomics in perennial woody plants of agricultural importance due to its characteristics, including a genome and methylome more alike to other crops than those of the most widely used model plant, Arabidopsis thaliana (Lee and Kim, 2014), in addition to a set of important agronomic characteristics which have been previously associated with epigenetic mechanisms: (1) grapevine is considered one of the models for non-climacteric fruit development (Fortes et al., 2015); (2) the usage of grafting and vegetative propagation (Lewsey et al., 2016); (3) vine age and vineyard location (Grigg, 2017; Xie et al., 2017; Grigg et al., 2018) have been traditionally associated with fruit production and quality; and (4) grapevine flower development has been shown to be programmed and affected by the environmental conditions one year in advance (Guilpart et al., 2014).
Although multiple studies have shown that the main driver of DNA methylation variability in grapevine is the genotype (Dal Santo et al., 2018; Varela et al., 2021), recent studies have suggested that the growing environment can have a significant effect on the methylome, and that such environmentally induced epigenetic changes could be the molecular basis of terroir in grapevine. In Xie et al., 2017 showed that the main contributor to differences in DNA between 22 V. vinifera cv. Shiraz vineyards in six sub-regions of South Australia was geographic distance (with 9% of the identified differentially methylated genes being associated with response to environmental stimulus), followed by vineyard management and altitude. A later study comparing DNA methylation patterns in two V. vinifera cultivars (i.e., Merlot and Pinot Noir) grown in contrasting climatic regions showed that a significant amount of DNA methylation variability (roughly 80% and 71% of Merlot and Pinot Noir, respectively) was associated to geographical location (Baránková et al., 2021).
The regulation of the biosynthesis of metabolites and the accumulation of phenolic compounds in grapevine is also found to be associated with epigenetic mechanisms. In V. amurensis cell cultures treated with 5-Azacytidine, a demethylating agent, the methylation level of a stilbene synthase gene was significantly reduced, while the gene expression of the same gene and the synthesis of resveratrol was significantly increased, which led to high level of resveratrol compared to the control cell culture, suggesting that the DNA methylation may be involved in the control of resveratrol biosynthesis during in vitro culture (Kiselev et al., 2013). Although these results might not be directly comparable to what happens in vivo, DNA methylation has also been reported to have a role in the production of anthocyanins, a pathway that competes with the biosynthesis of resveratrol (He et al., 2010), during berry maturation (Jia et al., 2020). In addition, UV-B was associated with flavonol accumulation in V. vinifera cv. Malbec berries and hydroxycinnamic acids in early fruit shoots, and those changes can be DNA methylation-dependent (Marfil et al., 2019). Interestingly, in a study that analyzed ten different grape varieties, a negative correlation between gene body methylation and gene expression variation between grapevine varieties was observed. The authors proposed that a higher number of transposable elements (TEs) within the grapevine genes may be responsible for this negative association between gene body methylation and expression (Magris et al., 2019). Pereira et al. (2022) were able to characterize nine grapevine DNA methyltransferase genes and suggested that changes in grapevine genome methylation are associated with the establishment of compatible and incompatible interactions with Plasmopara viticola. A following study by Azevedo et al. (2022) observed that DNA methylation is affected by P. viticola inoculation and that differences in the DNA methylation levels are related to the different susceptibility to P. viticola. These studies provided useful insights into the role of epigenetic mechanisms in grapevine defense against downy mildew and their potential implications for future breeding programs such as improving tolerance to powdery mildew in grapevine and reducing the massive current and recurring use of chemicals. Additionally, the use of DNA methyltransferases blockers (including but not limited to 5-azacytidine, 5-aza-2’-deoxycytidine, 1-beta-D-arabinofuranosyl-5-azacytosine and dihydro-5-azacytidine) has been proposed as an approach to generate epigenetic variation for crop improvement (Amoah et al., 2012), as recently implemented in the development of drought-tolerant sugarcane epimutants (Koetle et al., 2022).
6.1 Stress memory, priming, and epi-breeding
Similar to other crop breeding, classical grapevine breeding relies on the transfer of desirable traits by crossing and recurrent selection of genetic variants. Epigenetic mechanisms, on the other hand, play an essential role in the interactions between genes and the environment (Bräutigam et al., 2013; Baulcombe and Dean, 2014). As the study of epigenetics has advanced, it has provided novel directions to drive plant-breeding strategies by exploiting epigenetic variation and/or manipulating the epigenome to improve adaptation to various environmental stresses and ensure yield and quality (Rodríguez López and Wilkinson, 2015; Tirnaz and Batley, 2019; Pecinka et al., 2020; Gupta and Salgotra, 2022) (Figure 2). Indeed, studies have revealed the relevance of epigenetic regulation of stress response in many model and crop species such as A. thaliana (e.g., Tricker et al., 2012), barley (e.g., Konate et al., 2018), maize (e.g., Steward et al., 2002), rice (e.g., Zheng et al., 2017), soybean (e.g., Song et al., 2012), tomato (e.g., González et al., 2013), and wheat (e.g., Wang et al., 2016). Some additional examples include: Lämke et al. (2016) have described the methylation of histone H3 lysine 4 (H3K4) is involved in the heat stress-induced genes. Moreover, Surdonja et al. (2017) showed that DNA methylation and target gene repression by small non-coding RNAs were involved in the drought stress response in barley. Similarly, the presence of possible epi-marks that are drought inducible and inheritable across generation were observed in rice and that multigenerational drought exposure improved the adaptability of rice plants to drought conditions (Zheng et al., 2017). Taken collectively, these showed that epigenetic modifications play important roles in stress response and the long-term adaptation to changing environmental conditions (Zheng et al., 2017). Moreover, the study on natural and artificial epigenetic diversity could contribute to and improve current breeding programs, via multiple strategies. Including the identification of epigenetic biomarkers capable of predicting plant performance in a given environment (Kakoulidou et al., 2021); and the selection of epigenetic variability in genomic regions that modulate gene expression of traits of interest, after the validation of the temporal stability and functional association between a given epiallele and a given trait. The origin of such variability can be genotype dependent (Rodríguez López and Wilkinson, 2015), or exogenously generated through the application of chemicals capable of randomly altering the epigenetic profile of the target genome (Amoah et al., 2012) and via targeted gene editing approaches (Vojta et al., 2016). Moreover, the plastic and potentially heritable dual nature of environmentally induced epigenetic variability can generate epigenetically controlled adaptive traits to accelerate crop breeding (Rodríguez López and Wilkinson, 2015) (Figure 2).
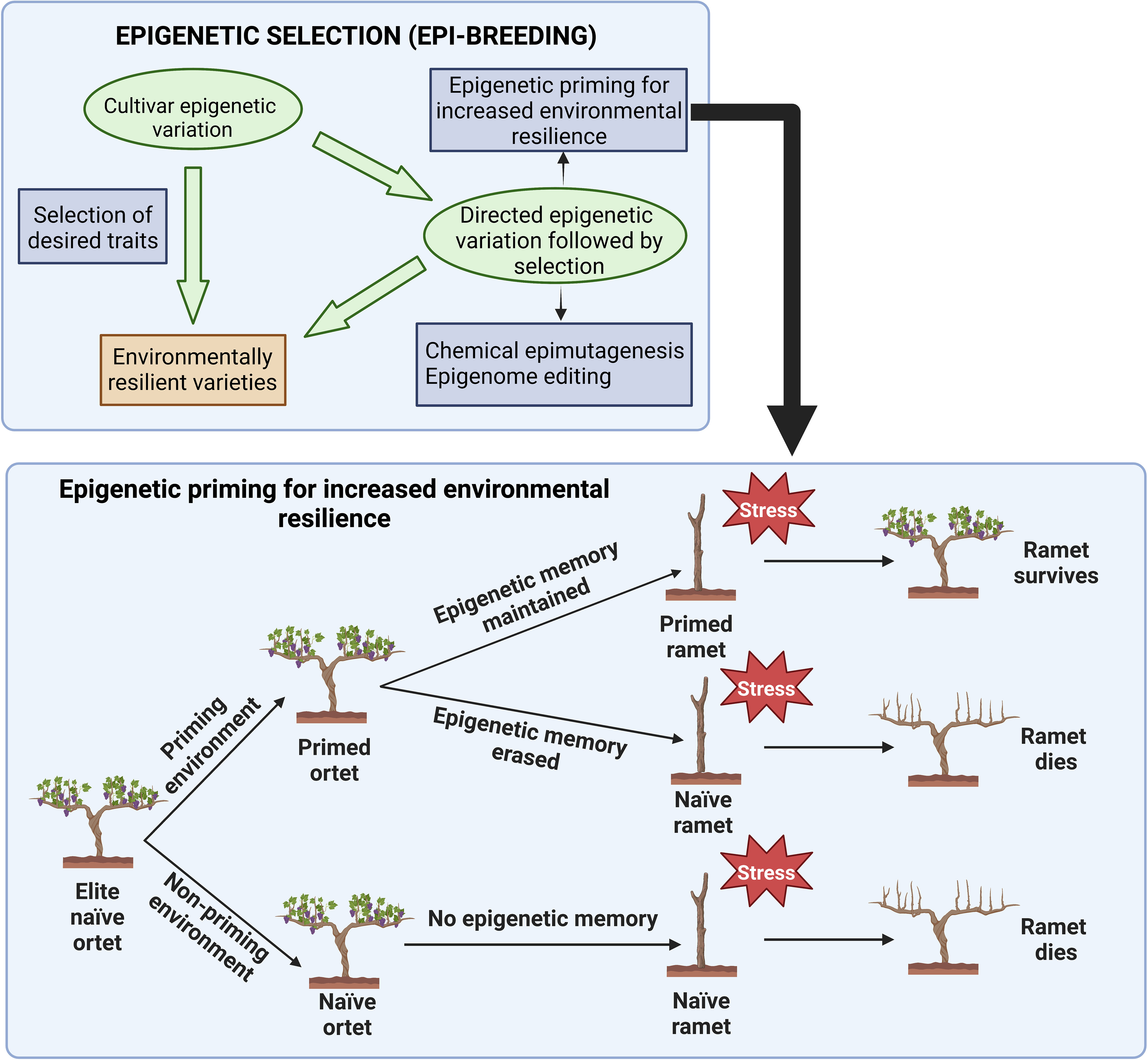
Figure 2 Epigenetic priming for the production of environmentally resilient grapevine cultivars. The top box shows two approaches for the production of environmentally resilient grapevine varieties via the selection of epigenetic variants of agronomic interest (adapted from Rodríguez López and Wilkinson, 2015). The bottom box shows the proposed method to enhance stress tolerance through epigenetic priming maintenance in perennial crops (modified from Rodriguez Lopez et al., 2019).
Stress and environmental stimuli can induce epigenetic variation in the genome, leading to phenotypic plasticity, where different phenotypes can arise from the same genome due to alterations in the epigenetic marks (Asensi-Fabado et al., 2017; Fortes and Gallusci, 2017). The acclimation and response process are thought to be related to the development of stress memory in plants (Figure 1). Stress memory is often associated with a phenomenon called stress priming, which is trigged by extreme conditions that inhibit normal growth and development. Priming has occurred when a plant shows a modified response to stress, after an initial exposure to stimulus, in comparison to a plant in the naïve (unprimed) state (Aranega-Bou et al., 2014). Priming is evidenced by positive effects like stronger or faster response to stress (Bruce et al., 2007; Conrath, 2009; Crisp et al., 2016). Studies have shown that plants have a memory of the first (priming) stress and are able to retrieve the remembered information upon encounter with a later stress when there is a period of no stress between the two stress events (Hilker and Schmülling, 2019). Additionally, studies have shown that priming is effective at various stages of the plant life cycle, starting from seed (i.e., seed priming) to seedlings and subsequent adult stages (Mozgova et al., 2019). While this priming and subsequent stress memory has provided valuable information on approaches to generate more vigorous crops via various products and techniques (e.g., Brzezinka et al., 2016), the underlying molecular mechanisms that establish, regulate, and even erase such memory has been puzzling (Iwasaki, 2015; Roberts and López Sánchez, 2019; Varotto et al., 2020).
Studies have, however, identified several mechanisms of storage and retrieval of this stress memory, which include epigenetic regulation, transcriptional priming, the primed conformation of proteins, or specific hormonal or metabolic signatures (Heil and Karban, 2010; Ding et al., 2012; Crisp et al., 2016; He and Li, 2018; Hake and Romeis, 2019). It is important to consider that specific transcriptional responses are triggered when plants are exposed to stress. After physiological recovery, the previously stressed plant enters the primed state, during which the transcription of the majority of stress-responsive genes will return to their original expression levels. The degree and time of recovery depends on the environmental cue (Avramova, 2015). The encounter of a second stress will trigger a different response than that shown by unprimed plants. The triggered response can be faster, stronger, more sensitive, and/or different (altered) than the first one (Lämke and Bäurle, 2017).
Some stress-inducible genes are linked to establishing a memory of stress, and they do not necessarily revert to their non-stress transcriptional state and are therefore termed stress memory genes (Charng et al., 2006; Charng et al., 2007; Ding et al., 2012; Lämke et al., 2016; Liu et al., 2018). Currently, memory genes are classified into two groups based on their transcriptional profile: Type I – those which change in expression pattern persists through the recovery phase, and Type II – those which response is modified during second exposure compared to initial stress response (reviewed in Bäurle (2018), and Bäurle and Trindade (2020)) and it is usually stronger and faster (Mozgova et al., 2019; Roberts and López Sánchez, 2019) (Figure 3). Built upon this knowledge, more evidence suggests that stress memory and the modified transcriptional response are heavily epigenetic-based and involve mechanisms such as chromatin remodeling, DNA methylation, nucleosome position, histone modification, and noncoding RNA-mediated regulation (Liu et al., 2022).
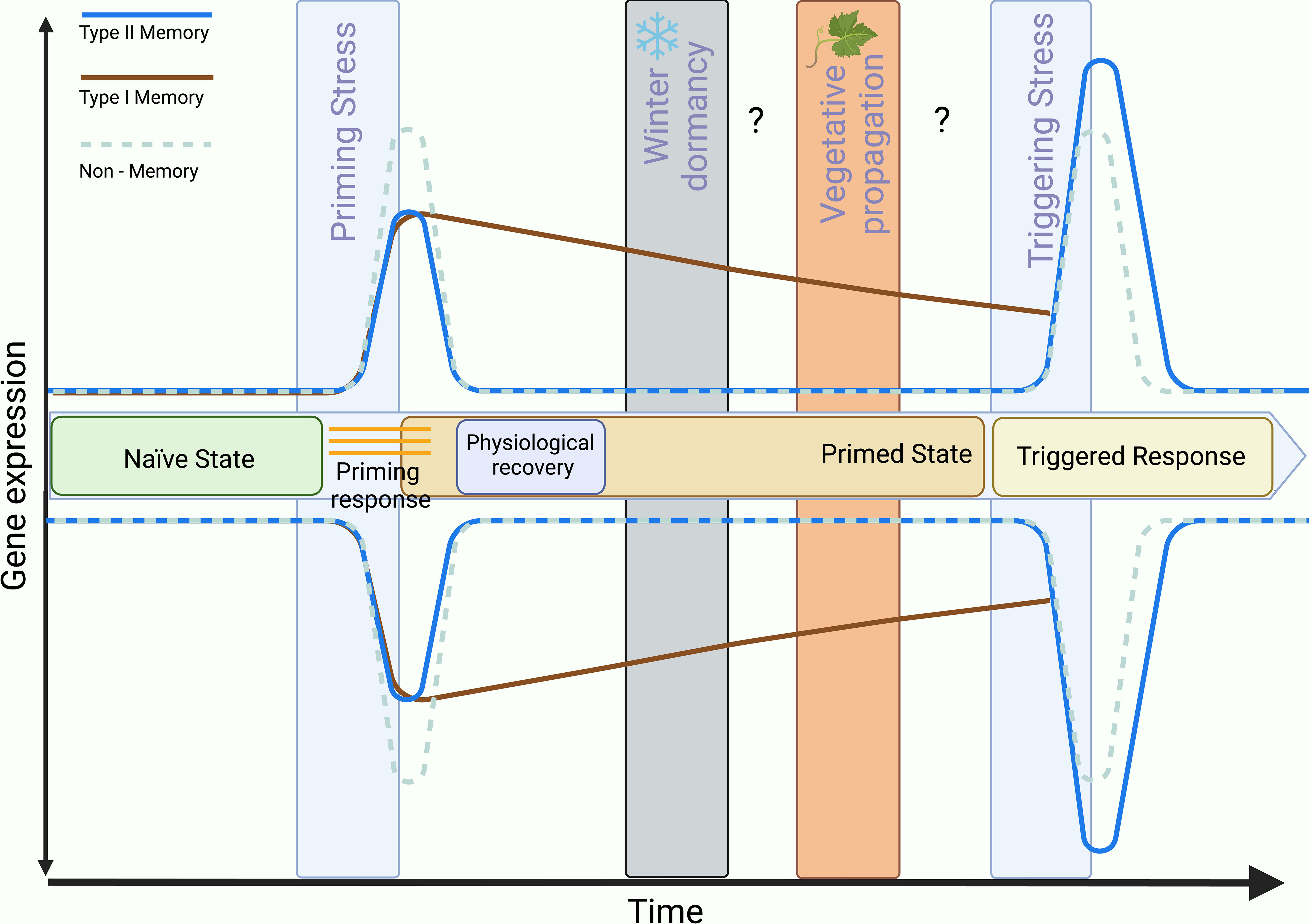
Figure 3 Effect of somatic memory of environmental stress on plant gene transcription in the context of perennial vegetatively propagated plants. Solid and dashed lines represent the transcriptional changes of stress-responsive genes triggered by the first stress encountered by naïve plants (priming stress), and by subsequent stress (triggering stress) encountered by primed plants. Stress-responsive genes can be classified into three categories based on their transcriptional profiles during priming and triggering stress events: (1) Non-memory genes, the stress-induced transcriptional changes are identical in naïve and primed plants; (2) Type I memory genes, the stress-induced transcriptional changes are sustained after stress removal and through physiological recovery; (3) Type II memory genes, the magnitude of the stress-induced transcriptional changes is larger in primed than in naïve plants (Bäurle, 2018). Current research in annual plants suggests that the primed state is maintained for a finite period within the same generation (somatic memory) and that it can also be inherited by the offspring of primed plants (inter-/transgenerational memory, not shown here), however, the effect of winter dormancy and vegetative propagation on the maintenance of priming has not been sufficiently studied in perennial plants.
Although the mechanisms underlying the stable status of epigenetic traits are not fully understood, stress induced epigenetic traits can be stable and therefore be inherited by the next generation as part of an adaptive form of memory (Johnson and Tricker, 2010). The effect of this stress memory can be observed through the physiological, transcriptional, and biochemical modifications occurring in the plant when re-expose to the stress, resulting in the plant becoming more resilient (or sensitive) to the same stress (Tricker et al., 2013a; de Freitas Guedes et al., 2018; Perrone and Martinelli, 2020) or a different stress (Tricker et al., 2013b). The duration of this memory varies from days to weeks or months for somatic memory (intergenerational), but it can be stable and inherited within offspring to one or more stress-free generations (transgenerational) (Blödner et al., 2007; Tricker et al., 2013a; Lämke and Bäurle, 2017; Bäurle, 2018). In annual plants, the key to keeping the transcriptional state associated with the primed response across generations, is the repeating stress in the progeny (Boyko et al., 2010; Wibowo et al., 2016), and a stress recovery phase of the mother plants (López Sánchez et al, 2021).
The potential importance of persistent stress for establishing DNA methylation-dependent stress memory through priming in plants has been highlighted and studied in annual plants, such as A. thaliana (e.g., Ding et al., 2012; Tricker et al., 2013a; Tricker et al., 2013b), maize (e.g., Forestan et al., 2020), and rice (e.g., Cong et al., 2019). How this translates to perennial plant species, which can be exposed many times during their life span, has not been studied to the same level. Studies on the effect of priming and establishment of stress memory on grapevine have been limited. However, multiple studies have focused on other perennials such as poplar (reviewed in Le Gac et al., 2018; Sow et al., 2018; Amaral et al., 2020). Other recent studies have addressed the effect of stress on the epigenome of different perennial plant species, including coffee (de Freitas Guedes et al., 2018), the perennial grass species tall fescue (Bi et al., 2021), and wild strawberry (López et al., 2022). Taken collectively, these studies show that the plant epigenome is versatile and plastic in response to environmental stress, and that the resulting change could potentially prime the plants against future stress (López et al., 2022). Viticulture could benefit from a deeper understanding of how this memory of stress is established, maintained, and even reset, leading to the production of more resilient grape varieties, via epigenetic selection, priming, epimutagenesis, and targeted epigenome editing.
7 Gaps in knowledge, potential challenges, and future prospects
As described in this review, there is growing evidence that epigenetic mechanisms play an important role in increasing crop resilience to stresses and therefore may be an important tool in the development of more resilient grapevine cultivars. However, among the plethora of epigenetic memory of stress and priming studies done in plants, only a small amount of them is perennial-focused – even less on grapevine specifically. Contrary to the limited studies on epigenetic memory of stress and priming, there is no lack of observations of stress priming in grapevine. Some of the more recent studies that observed physiological, transcriptional, and biochemical modifications that are potentially indicative of established stress memory in grapevine include, Babajamali et al. (2022), which showed that drought stress priming improved freezing tolerance in shoot and root tissues of both drought-tolerant and sensitive grapevine cultivars. In addition, a study performed on dry-grown Cabernet Sauvignon suggested the more drought-resilient grapevines with superior vine water status, leaf gas exchange and berry size are likely due to long-term drought stress adaptation via stress priming (Pagay et al., 2022). Spray-induced gene silencing (SIGS) that targets a putative grape glutathione S-transferase (GST) gene (VvGST40) has been shown to prime vines resulting in increased resilience to severe drought (Nerva et al., 2022). In the response to salinity stress, it has been shown that 6-Benzylaminopurine (BAP) primes salt tolerance in V. vinifera, with BAP-primed plants exhibiting higher intrinsic water use efficiency, photosystem-II efficiency, and growth (Montanaro et al., 2022). Moreover, grapevines infected with Grapevine fanleaf virus (GFLV) are more resilient to mild water stress than healthy vines, suggesting that the biotic stress can potentially induce priming in grapevine (Jež-Krebelj et al., 2022). Many more studies including biotic stress priming (e.g., Trouvelot et al., 2008; Verhagen et al., 2010; Perazzolli et al., 2011) and abiotic stress priming (e.g., Tombesi et al., 2018) are good indicators of the familiarity of priming effects in grapevine. Even with the ever-growing research on epigenetic regulations in the grapevine, to date, a limited amount of research is available on how this memory of stress and its underlying epigenetic mechanisms are established, maintained, and even reset. The long lifespan of woody perennials could be used to address some of the prevailing concerns in studies with annual plants, such as whether the period of vegetative growth between the priming treatment and the second stress treatment is long enough to test whether the stress is phenotypically effective and whether the changes in the epigenome are induced by priming treatment (Sani et al., 2013). Therefore, providing valuable insights into how long-term somatic memory is established and if it can be maintained past winter dormancy. Similarly, the connection between vegetative propagation and epigenetic memory of stress establishment and maintenance should also be considered. The use of vegetative propagation (i.e., propagated through cutting or layering) in woody perennials could reveal novel and useful information on how permanent or transient long-term somatic memory is after vegetative propagation (Perrone and Martinelli, 2020). Viticulture could benefit greatly from the understanding of transient or stable modification to the epigenome of stress memory, as it may contribute to developing novel molecular approaches such as targeted, gene-specific modifications for stress adaptation through plant breeding, leading to the production of more resilient grape varieties. It should be considered, when using epigenetic and epigenomics to develop stress resilient crop, that the negative effects of stress memory on breeding in general, as the obtained stress memory could inhibit normal plant growth (Chinnusamy and Zhu, 2009). The prediction and assessment of the impact of stable epigenetic variation on plant phenotype and performance should be explored further, via machine learning and model training as demonstrated in several studies (Colicchio et al., 2015; Hu et al., 2015; N'Diaye et al., 2020).
Some challenges to the utilization of epigenomics to design environment resilient grapevine, such as the stability and heritability of the epigenetic variation, are important for the potential transmission to the progeny (Eichten et al., 2014; Iwasaki and Paszkowski, 2014; Vriet et al., 2015). Most of the stress-induced epigenetic modifications return to basal levels when the stress is removed, but some of the modifications can be inherited mitotically and meiotically in plants (Sudan et al., 2018). Such epigenetically mediated stress memory can later lead to longer-term adaptation, an indication of the possibility of using epigenetics as a tool to combat environmental stress. It is important to note, however, further study is needed for understanding various factors that might affect epiallele stability in order to avoid inducing epialleles that might be unstable during the breeding process (Hofmeister et al., 2017). Moreover, epigenetic variation can also be maladaptive and become an epigenetic trap (Consuegra and Rodríguez López, 2016), not only if the changes they induce do not match the environment experienced by the offspring, but also due to the energetic cost associated to the maintenance of the acquired epigenetic state, which could negatively impact the plant growth and development, and ultimately affect crop yield (Chinnusamy and Zhu, 2009).
Another major challenge in creating epigenetic populations in crops is the uncertainty of whether epigenetic changes (i.e., alteration of DNA methylation patterns) induced by approaches developed in model species such as A. thaliana can be transferable to crops, since so few to none viable equivalent mutants have been produced in crop species (Hu et al., 2014; Li et al., 2014; Kawakatsu and Ecker, 2019). An alternative approach such as epimutagenesis and targeted epigenome editing can be utilized, as demonstrated in A. thaliana (Johnson et al., 2014; Springer and Schmitz, 2017). However, it will require advancement and innovation in both technical and biological disciplines to bring out the full potential of epigenomic variants and use them efficiently in the breeding of better stress-adapted crops (Varotto et al., 2020). Similarly, for the integration of epigenetics and epigenomics in crop, or more specifically, grapevine breeding, more knowledge needs to be acquired on stress induced epigenetic memory in perennials. Such acquisition of knowledge should move beyond describing the correlation between epigenetic variation and the desired trait, towards the demonstration of the functional association between acquired epialleles and enhanced tolerance to stress.
Despite the gap in knowledge in stress memory establishment and maintenance, the advancement in technology and the employment of multi-omics approaches have allowed epigenetic breeding (epi-breeding) to be successful in various steps of the process (Rajnović et al., 2020). Such as the generation of mutant lines (e.g., Yang et al., 2015), recurrent epi-selection (e.g., Hauben et al., 2009; Greaves et al., 2014), and epigenome editing (e.g., Park et al., 2016), as well as the usage of priming/stress memory (e.g., Lämke and Bäurle, 2017). One of the successful examples is through suppressing the nuclear-encoded MutS HOMOLOGUE 1 (MSH1). The success of the MSH1 system has been reported in A. thaliana and tomato, where the phenotypic changes that led to improved growth vigor and yield were linked to DNA methylation, as 5-AzaC can repress those improvements, while METHYLTRANSFERASE 1 (MET1) and HISTONE DEACETYLASE 6 (HDA6) played an important role in those phenotypic changes (Yang et al., 2015; Kundariya et al., 2020; Yang et al., 2020). In soybean, epigenetic selection has led to yield improvement for at least three generations (Raju et al., 2018). Moreover, after crossing the msh1 mutant to the wild type, the epi-population created was shown to possess multiple yield-related traits both in the greenhouse and in the field (Raju et al., 2018). Many other examples showing the potential of epi-breeding for plant adaptation to various stress including the usage of eustressors have been reviewed by Kakoulidou et al. (2021), and Villagómez-Aranda et al. (2022). For these successful examples to serve as future grapevine improvement strategies, its inherent characteristics (long-living perennial, highly heterozygous, high inbreeding depression) must be considered.
If the grape industry, and by extension other perennial crop industries, want to benefit from the potential use of epi-breeding approaches to produce climate resilient varieties, future multi-omics studies should be custom designed to unravel how environmentally induced epigenetic mechanisms interact with gene expression to affect the phenotype, and to determine if environmental stress is followed by the establishment and maintenance of a memory of stress in grapevine. Such studies will lay the foundation for the development of comprehensive models integrating plant response to stress, the establishment of transcriptional and epigenetic memory of stress, and their maintenance, over time and during vegetative propagation in perennial plants.
Author contributions
Both authors contributed to manuscript writing and revision, read, and approved the submitted version.
Funding
This work was supported by the National Institute of Food and Agriculture, AFRI Competitive Grant Program Accession number 1018617, and the National Institute of Food and Agriculture, United States Department of Agriculture, Hatch Program accession number 1020852.
Conflict of interest
The authors declare that the research was conducted in the absence of any commercial or financial relationships that could be construed as a potential conflict of interest.
The author CMRL declared that they were an editorial board member of Frontiers, at the time of submission. This had no impact on the peer review process and the final decision.
Publisher’s note
All claims expressed in this article are solely those of the authors and do not necessarily represent those of their affiliated organizations, or those of the publisher, the editors and the reviewers. Any product that may be evaluated in this article, or claim that may be made by its manufacturer, is not guaranteed or endorsed by the publisher.
References
Acevedo-Opazo C., Tisseyre B., Guillaume S., Ojeda H. (2008). The potential of high spatial resolution information to define within-vineyard zones related to vine water status. Precis. Agric. 9 (5), 285–302. doi: 10.1007/s11119-008-9073-1
Agarwal G., Kudapa H., Ramalingam A., Choudhary D., Sinha P., Garg V., et al. (2020). Epigenetics and epigenomics: underlying mechanisms, relevance, and implications in crop improvement. Funct. Integr. Genomics 20, 739–761. doi: 10.1007/s10142-020-00756-7
Alikadic A., Pertot I., Eccel E., Dolci C., Zarbo C., Caffarra A., et al. (2019). The impact of climate change on grapevine phenology and the influence of altitude: a regional study. Agric. For. Meteorology 271, 73–82. doi: 10.1016/j.agrformet.2019.02.030
Alston J. M., Sambucci O. (2019). Grapes in the world economy. in The grape genome. compendium of plant genomes. in Cantu, D., Walker, M. (eds) (Springer, Cham), 1–24. doi: 10.1007/978-3-030-18601-2_1
Amaral J., Ribeyre Z., Vigneaud J., Sow M. D., Fichot R., Messier C., et al. (2020). Advances and promises of epigenetics for forest trees. Forests 11, 976. doi: 10.3390/f11090976
Amoah S., Kurup S., Rodriguez Lopez C. M., Welham S. J., Powers S. J., Hopkins C. J., et al. (2012). A hypomethylated population of Brassica rapa for forward and reverse epi-genetics. BMC Plant Biol. 12, 1–17. doi: 10.1186/1471-2229-12-193
Aradhya M. K., Dangl G. S., Prins B. H., Boursiquot J.-M., Walker M. A., Meredith C. P., et al. (2003). Genetic structure and differentiation in cultivated grape, Vitis vinifera l. Genet. Res. 81, 179–192. doi: 10.1017/S0016672303006177
Aranega-Bou P., de la O Leyva M., Finiti I., García-Agustín P., González-Bosch C. (2014). Priming of plant resistance by natural compounds. hexanoic acid as a model. Front. Plant Sci. 5, 488. doi: 10.3389/fpls.2014.00488
Arias L. A., Berli F., Fontana A., Bottini R., Piccoli P. (2022). Climate change effects on grapevine physiology and biochemistry: benefits and challenges of high altitude as an adaptation strategy. Front. Plant Sci. 13. doi: 10.3389/fpls.2022.835425
Arnaudo A. M., Garcia B. A. (2013). Proteomic characterization of novel histone post-translational modifications. Epigenet. chromatin 6, 1–7. doi: 10.1186/1756-8935-6-24
Asensi-Fabado M.-A., Amtmann A., Perrella G. (2017). Plant responses to abiotic stress: the chromatin context of transcriptional regulation. Biochim. Biophys. Acta (BBA)-Gene Regul. Mech. 1860, 106–122. doi: 10.1016/j.bbagrm.2016.07.015
Avramova Z. (2015). Transcriptional ‘memory’of a stress: transient chromatin and memory (epigenetic) marks at stress-response genes. Plant J. 83, 149–159. doi: 10.1111/tpj.12832
Axtell M. J. (2013). Classification and comparison of small RNAs from plants. Annu. Rev. Plant Biol. 64, 137–159. doi: 10.1146/annurev-arplant-050312-120043
Azevedo V., Daddiego L., Cardone M. F., Perrella G., Sousa L., Santos R. B., et al. (2022). Transcriptomic and methylation analysis of susceptible and tolerant grapevine genotypes following Plasmopara viticola infection. Physiologia Plantarum 174, e13771. doi: 10.1111/ppl.13771
Babajamali A., Gholami M., Baninasab B. (2022). Drought preconditioning improves freezing tolerance in drought-tolerant and-intolerant grape cultivars. Theor. Exp. Plant Physiol. 34, 395–407. doi: 10.1007/s40626-022-00252-2
Bai H., Gambetta G. A., Wang Y., Kong J., Long Q., Fan P., et al. (2022). Historical long-term cultivar × climate suitability data to inform viticultural adaptation to climate change. Sci. Data 9, 1–10. doi: 10.1038/s41597-022-01367-6
Bais A. J., Murphy P. J., Dry I. B. (2000). The molecular regulation of stilbene phytoalexin biosynthesis in Vitis vinifera during grape berry development. Funct. Plant Biol. 27, 723–723. doi: 10.1071/PP00007_CO
Baránková K., Nebish A., Tříska J., Raddová J., Baránek M. (2021). Comparison of DNA methylation landscape between Czech and Armenian vineyards show their unique character and increased diversity. Czech J. Genet. Plant Breed. 57, 67–75. doi: 10.17221/90/2020-CJGPB
Bartels A., Han Q., Nair P., Stacey L., Gaynier H., Mosley M., et al. (2018). Dynamic DNA methylation in plant growth and development. Int. J. Mol. Sci. 19, 2144. doi: 10.3390/ijms19072144
Baulcombe D. C., Dean C. (2014). Epigenetic regulation in plant responses to the environment. Cold Spring Harbor Perspect. Biol. 6, a019471. doi: 10.1101/cshperspect.a019471
Bäurle I. (2018). Can't remember to forget you: chromatin-based priming of somatic stress responses. Semin. Cell Dev. Biol. 83, 133–139. doi: 10.1016/j.semcdb.2017.09.032
Bäurle I., Trindade I. (2020). Chromatin regulation of somatic abiotic stress memory. J. Exp. Bot. 71, 5269–5279. doi: 10.1093/jxb/eraa098
Berdeja M., Nicolas P., Kappel C., Dai Z. W., Hilbert G., Peccoux A., et al. (2015). Water limitation and rootstock genotype interact to alter grape berry metabolism through transcriptome reprogramming. Horticulture Res. 2, 15012. doi: 10.1038/hortres.2015.12
Berli F. J., Alonso R., Bressan-Smith R., Bottini R. (2013). UV-B impairs growth and gas exchange in grapevines grown in high altitude. Physiologia Plantarum 149, 127–140. doi: 10.1111/ppl.12012
Berli F. J., Fanzone M., Piccoli P., Bottini R. (2011). Solar UV-b and ABA are involved in phenol metabolism of Vitis vinifera l. increasing biosynthesis of berry skin polyphenols. J. Agric. Food Chem. 59, 4874–4884. doi: 10.1021/jf200040z
Bernardo S., Dinis L.-T., Machado N., Moutinho-Pereira J. (2018). Grapevine abiotic stress assessment and search for sustainable adaptation strategies in Mediterranean-like climates. a review. Agron. Sustain. Dev. 38, 1–20. doi: 10.1007/s13593-018-0544-0
Bewick A. J., Schmitz R. J. (2017). Gene body DNA methylation in plants. Curr. Opin. Plant Biol. 36, 103–110. doi: 10.1016/j.pbi.2016.12.007
Bi A., Wang T., Wang G., Zhang L., Wassie M., Amee M., et al. (2021). Stress memory gene FaHSP17. 8-CII controls thermotolerance via remodeling PSII and ROS signaling in tall fescue. Plant Physiol. 187, 1163–1176. doi: 10.1093/plphys/kiab205
Blödner C., Goebel C., Feussner I., Gatz C., Polle A. (2007). Warm and cold parental reproductive environments affect seed properties, fitness, and cold responsiveness in Arabidopsis thaliana progenies. Plant Cell Environ. 30, 165–175. doi: 10.1111/j.1365-3040.2006.01615.x
Bonada M., Jeffery D. W., Petrie P. R., Moran M. A., Sadras V. O. (2015). Impact of elevated temperature and water deficit on the chemical and sensory profiles of barossa Shiraz grapes and wines. Aust. J. Grape Wine Res. 21, 240–253. doi: 10.1111/ajgw.12142
Bouyer D., Kramdi A., Kassam M., Heese M., Schnittger A., Roudier F., et al. (2017). DNA Methylation dynamics during early plant life. Genome Biol. 18, 1–12. doi: 10.1186/s13059-017-1313-0
Boyko A., Blevins T., Yao Y., Golubov A., Bilichak A., Ilnytskyy Y., et al. (2010). Transgenerational adaptation of Arabidopsis to stress requires DNA methylation and the function of dicer-like proteins. PloS One 5, e9514. doi: 10.1371/annotation/726f31b5-99c4-44e9-9cd6-b8d66b3f6038
Bramley R. (2005). Understanding variability in winegrape production systems 2. within vineyard variation in quality over several vintages. Aust. J. Grape Wine Res. 11, 33–42. doi: 10.1111/j.1755-0238.2005.tb00277.x
Bramley R., Hamilton R. (2004). Understanding variability in winegrape production systems: 1. within vineyard variation in yield over several vintages. Aust. J. Grape Wine Res. 10, 32–45. doi: 10.1111/j.1755-0238.2004.tb00006.x
Bräutigam K., Vining K. J., Lafon-Placette C., Fossdal C. G., Mirouze M., Marcos J. G., et al. (2013). Epigenetic regulation of adaptive responses of forest tree species to the environment. Ecol. Evol. 3, 399–415. doi: 10.1002/ece3.461
Brillante L., Bonfante A., Bramley R. G., Tardaguila J., Priori S. (2020). Unbiased scientific approaches to the study of terroir are needed! Front. Earth Sci. 8, 539377. doi: 10.3389/feart.2020.539377
Brillante L., Martínez-Lüscher J., Kurtural S. K. (2018). Applied water and mechanical canopy management affect berry and wine phenolic and aroma composition of grapevine (Vitis vinifera l., cv. syrah) in central California. Scientia Hortic. 227, 261–271. doi: 10.1016/j.scienta.2017.09.048
Bruce T. J., Matthes M. C., Napier J. A., Pickett J. A. (2007). Stressful “memories” of plants: evidence and possible mechanisms. Plant Sci. 173, 603–608. doi: 10.1016/j.plantsci.2007.09.002
Brzezinka K., Altmann S., Czesnick H., Nicolas P., Gorka M., Benke E., et al. (2016). Arabidopsis FORGETTER1 mediates stress-induced chromatin memory through nucleosome remodeling. elife 5, e17061. doi: 10.7554/eLife.17061.037
Budak H., Hussain B., Khan Z., Ozturk N. Z., Ullah N. (2015). From genetics to functional genomics: improvement in drought signaling and tolerance in wheat. Front. Plant Sci. 6, 1012. doi: 10.3389/fpls.2015.01012
Bunting E. L., Wanyama D., Goodwin R., Weil N., Sabbatini P., Andresen J. (2021). Vitis vinifera production in Michigan: factors and trends driving cultivation patterns. Front. Plant Sci. 12. doi: 10.3389/fpls.2021.704690
Cabré M. F., Quénol H., Nuñez M. (2016). Regional climate change scenarios applied to viticultural zoning in Mendoza, Argentina. Int. J. Biometeorology 60, 1325–1340. doi: 10.1007/s00484-015-1126-3
Camps C., Kappel C., Lecomte P., Léon C., Gomès E., Coutos-Thévenot P., et al. (2010). A transcriptomic study of grapevine (Vitis vinifera cv. Cabernet-sauvignon) interaction with the vascular ascomycete fungus Eutypa lata. J. Exp. Bot. 61, 1719–1737. doi: 10.1093/jxb/erq040
Carbonell-Bejerano P., Santa María E., Torres-Pérez R., Royo C., Lijavetzky D., Bravo G., et al. (2013). Thermotolerance responses in ripening berries of Vitis vinifera l. cv Muscat Hamburg. Plant Cell Physiol. 54, 1200–1216. doi: 10.1093/pcp/pct071
Cardone M., Perniola R., Catacchio C., Alagna F., Rotunno S., Crupi P., et al. (2019). Grapevine adaptation to drought: new candidate genes for the genotype-dependent response. Bio Web Conf. 15, 1016. doi: 10.1051/bioconf/20191501016
Casassa L. F., Keller M., Harbertson J. F. (2015). Regulated deficit irrigation alters anthocyanins, tannins and sensory properties of Cabernet sauvignon grapes and wines. Molecules 20, 7820–7844. doi: 10.3390/molecules20057820
Castellarin S. D., Matthews M. A., Di Gaspero G., Gambetta G. A. (2007). Water deficits accelerate ripening and induce changes in gene expression regulating flavonoid biosynthesis in grape berries. Planta 227, 101–112. doi: 10.1007/s00425-007-0598-8
Charng Y. Y., Liu H. C., Liu N. Y., Chi W. T., Wang C. N., Chang S. H., et al. (2007). A heat-inducible transcription factor, HsfA2, is required for extension of acquired thermotolerance in Arabidopsis. Plant Physiol. 143, 251–262. doi: 10.1104/pp.106.091322
Charng Y. Y., Liu H. C., Liu N. Y., Hsu F. C., Ko S. S. (2006). Arabidopsis Hsa32, a novel heat shock protein, is essential for acquired thermotolerance during long recovery after acclimation. Plant Physiol. 140, 1297–1305. doi: 10.1104/pp.105.074898
Chinnusamy V., Zhu J. K. (2009). Epigenetic regulation of stress responses in plants. Curr. Opin. Plant Biol. 12, 133–139. doi: 10.1016/j.pbi.2008.12.006
Chone X., Van Leeuwen C., Dubourdieu D., Gaudillère J. P. (2001). Stem water potential is a sensitive indicator of grapevine water status. Ann. Bot. 87, 477–483. doi: 10.1006/anbo.2000.1361
Cochetel N., Escudié F., Cookson S. J., Dai Z., Vivin P., Bert P.-F., et al. (2017). Root transcriptomic responses of grafted grapevines to heterogeneous nitrogen availability depend on rootstock genotype. J. Exp. Bot. 68, 4339–4355. doi: 10.1093/jxb/erx224
Cochetel N., Ghan R., Toups H. S., Degu A., Tillett R. L., Schlauch K. A., et al. (2020). Drought tolerance of the grapevine, Vitis champinii cv. ramsey, is associated with higher photosynthesis and greater transcriptomic responsiveness of abscisic acid biosynthesis and signaling. BMC Plant Biol. 20, 1–25. doi: 10.1186/s12870-019-2012-7
Colicchio J. M., Miura F., Kelly J. K., Ito T., Hileman L. C. (2015). DNA Methylation and gene expression in Mimulus guttatus. BMC Genomics 16, 1–15. doi: 10.1186/s12864-015-1668-0
Cong W., Miao Y., Xu L., Zhang Y., Yuan C., Wang J., et al. (2019). Transgenerational memory of gene expression changes induced by heavy metal stress in rice (Oryza sativa l.). BMC Plant Biol. 19, 1–14. doi: 10.1186/s12870-019-1887-7
Conrath U. (2009). Priming of induced plant defense responses. Adv. Botanical Res. 51, 361–395. doi: 10.1016/S0065-2296(09)51009-9
Consuegra S., Rodríguez López C. M. (2016). Epigenetic-induced alterations in sex-ratios in response to climate change: an epigenetic trap? BioEssays 38 (10), 950–958. doi: 10.1002/bies.201600058
Cook B. I., Wolkovich E. M. (2016). Climate change decouples drought from early wine grape harvests in France. Nat. Climate Change 6, 715–719. doi: 10.1038/nclimate2960
Corbin K. R., Bolt B., Rodríguez López C. M. (2020). Breeding for beneficial microbial communities using epigenomics. Front. Microbiol. 11, 937. doi: 10.3389/fmicb.2020.00937
Costantini L., Battilana J., Lamaj F., Fanizza G., Grando M. S. (2008). Berry and phenology-related traits in grapevine (Vitis vinifera l.): from quantitative trait loci to underlying genes. BMC Plant Biol. 8, 1–17. doi: 10.1186/1471-2229-8-38
Crisp P. A., Ganguly D., Eichten S. R., Borevitz J. O., Pogson B. J. (2016). Reconsidering plant memory: intersections between stress recovery, RNA turnover, and epigenetics. Sci. Adv. 2, e1501340. doi: 10.1126/sciadv.1501340
Cuerda-Gil D., Slotkin R. K. (2016). Non-canonical RNA-directed DNA methylation. Nat. Plants 2, 1–8. doi: 10.1038/nplants.2016.163
Dal Santo S., Palliotti A., Zenoni S., Tornielli G. B., Fasoli M., Paci P., et al. (2016). Distinct transcriptome responses to water limitation in isohydric and anisohydric grapevine cultivars. BMC Genomics 17, 1–19. doi: 10.3389/fpls.2016.00970
Dal Santo S., Zenoni S., Sandri M., De Lorenzis G., Magris G., De Paoli E., et al. (2018). Grapevine field experiments reveal the contribution of genotype, the influence of environment and the effect of their interaction (G× e) on the berry transcriptome. Plant J. 93, 1143–1159. doi: 10.1111/tpj.13834
de Freitas Guedes F. A., Nobres P., Ferreira D. C. R., Menezes-Silva P. E., Ribeiro-Alves M., Correa R. L., et al. (2018). Transcriptional memory contributes to drought tolerance in coffee (Coffea canephora) plants. Environ. Exp. Bot. 147, 220–233. doi: 10.1016/j.envexpbot.2017.12.004
Deluc L. G., Decendit A., Papastamoulis Y., Merillon J.-M., Cushman J. C., Cramer G. R. (2011). Water deficit increases stilbene metabolism in Cabernet sauvignon berries. J. Agric. Food Chem. 59, 289–297. doi: 10.1021/jf1024888
Deluc L. G., Quilici D. R., Decendit A., Grimplet J., Wheatley M. D., Schlauch K. A., et al. (2009). Water deficit alters differentially metabolic pathways affecting important flavor and quality traits in grape berries of Cabernet sauvignon and Chardonnay. BMC Genomics 10, 1–33. doi: 10.1186/1471-2164-10-212
De Orduna R. M. (2010). Climate change associated effects on grape and wine quality and production. Food Res. Int. 43, 1844–1855. doi: 10.1016/j.foodres.2010.05.001
Des Gachons C. P., Leeuwen C. V., Tominaga T., Soyer J. P., Gaudillère J. P., Dubourdieu D. (2005). Influence of water and nitrogen deficit on fruit ripening and aroma potential of Vitis vinifera l cv sauvignon blanc in field conditions. J. Sci. Food Agric. 85, 73–85. doi: 10.1002/jsfa.1919
Diffenbaugh N. S., White M. A., Jones G. V., Ashfaq M. (2011). Climate adaptation wedges: a case study of premium wine in the western united states. Environ. Res. Lett. 6, 024024. doi: 10.1088/1748-9326/6/2/024024
Ding Y., Fromm M., Avramova Z. (2012). Multiple exposures to drought train transcriptional responses in arabidopsis. Nat. Commun. 3, 1–9. doi: 10.1038/ncomms1732
Downey M. O., Dokoozlian N. K., Krstic M. P. (2006). Cultural practice and environmental impacts on the flavonoid composition of grapes and wine: a review of recent research. Am. J. Enology Viticulture 57, 257–268. doi: 10.5344/ajev.2006.57.3.257
Dry P. R. (2000). Canopy management for fruitfulness. Aust. J. Grape Wine Res. 6, 109–115. doi: 10.1111/j.1755-0238.2000.tb00168.x
Du J., Johnson L. M., Jacobsen S. E., Patel D. J. (2015). DNA Methylation pathways and their crosstalk with histone methylation. Nat. Rev. Mol. Cell Biol. 16, 519–532. doi: 10.1038/nrm4043
Dubrovina A. S., Kiselev K. V., Khristenko V. S., Aleynova O. A. (2015). VaCPK20, a calcium-dependent protein kinase gene of wild grapevine Vitis amurensis rupr., mediates cold and drought stress tolerance. J. Plant Physiol. 185, 1–12. doi: 10.1016/j.jplph.2015.05.020
Dubrovina A. S., Kiselev K. V., Khristenko V. S., Aleynova O. A. (2017). The calcium-dependent protein kinase gene VaCPK29 is involved in grapevine responses to heat and osmotic stresses. Plant Growth Regul. 82, 79–89. doi: 10.1007/s10725-016-0240-5
Du Plessis K., Young P. R., Eyéghé-Bickong H. A., Vivier M. A. (2017). The transcriptional responses and metabolic consequences of acclimation to elevated light exposure in grapevine berries. Front. Plant Sci. 8, 1261. doi: 10.3389/fpls.2017.01261
Edwards E., Clingeleffer P. R. (2013). Interseasonal effects of regulated deficit irrigation on growth, yield, water use, berry composition and wine attributes of Cabernet sauvignon grapevines. Aust. J. Grape Wine Res. 19, 261–276. doi: 10.1111/ajgw.12027
Eichten S. R., Schmitz R. J., Springer N. M. (2014). Epigenetics: beyond chromatin modifications and complex genetic regulation. Plant Physiol. 165, 933–947. doi: 10.1104/pp.113.234211
Eichten S. R., Springer N. M. (2015). Minimal evidence for consistent changes in maize DNA methylation patterns following environmental stress. Front. Plant Sci. 6, 308. doi: 10.3389/fpls.2015.00308
Ezzahouani A., Valancogne C., Pieri P., Amalak T., Gaudillère J.-P. (2007). Water economy by italia grapevines under different irrigation treatments in a Mediterranean climate. OENO One 41, 131–139. doi: 10.20870/oeno-one.2007.41.3.845
Fernandes de Oliveira A., Mercenaro L., Del Caro A., Pretti L., Nieddu G. (2015). Distinctive anthocyanin accumulation responses to temperature and natural UV radiation of two field-grown Vitis vinifera l. cultivars. Molecules 20, 2061–2080. doi: 10.3390/molecules20022061
Forestan C., Farinati S., Zambelli F., Pavesi G., Rossi V., Varotto S. (2020). Epigenetic signatures of stress adaptation and flowering regulation in response to extended drought and recovery in Zea mays. Plant Cell Environ. 43, 55–75. doi: 10.1111/pce.13660
Fortes A. M., Gallusci P. (2017). Plant stress responses and phenotypic plasticity in the epigenomics era: perspectives on the grapevine scenario, a model for perennial crop plants. Front. Plant Sci. 8, 82. doi: 10.3389/fpls.2017.00082
Fortes A. M., Teixeira R. T., Agudelo-Romero P. (2015). Complex interplay of hormonal signals during grape berry ripening. Molecules 20, 9326–9343. doi: 10.3390/molecules20059326
Fraga H., Malheiro A. C., Moutinho-Pereira J., Cardoso R. M., Soares P. M., Cancela J. J., et al. (2014). Integrated analysis of climate, soil, topography and vegetative growth in Iberian viticultural regions. PloS One 9, e108078. doi: 10.1371/journal.pone.0108078
Fraga H., Malheiro A. C., Moutinho-Pereira J., Santos J. A. (2012). An overview of climate change impacts on European viticulture. Food Energy Secur. 1, 94–110. doi: 10.1002/fes3.14
Gambetta G. A., Herrera J. C., Dayer S., Feng Q., Hochberg U., Castellarin S. D. (2020). The physiology of drought stress in grapevine: towards an integrative definition of drought tolerance. J. Exp. Bot. 71, 4658–4676. doi: 10.1093/jxb/eraa245
Gobbi A., Acedo A., Imam N., Santini R. G., Ortiz-Álvarez R., Ellegaard-Jensen L., et al. (2022). A global microbiome survey of vineyard soils highlights the microbial dimension of viticultural terroirs. Commun. Niology 5, 1–9. doi: 10.1038/s42003-022-03202-5
Gomès É., Maillot P., Duchêne É. (2021). Molecular tools for adapting viticulture to climate change. Front. Plant Sci. 12, 633846. doi: 10.3389/fpls.2021.633846
González R. M., Ricardi M. M., Iusem N. D. (2013). Epigenetic marks in an adaptive water stress-responsive gene in tomato roots under normal and drought conditions. Epigenetics 8, 864–872. doi: 10.4161/epi.25524
Greaves I. K., Groszmann M., Wang A., Peacock W. J., Dennis E. S. (2014). Inheritance of trans chromosomal methylation patterns from Arabidopsis F1 hybrids. Proc. Natl. Acad. Sci. 111, 2017–2022. doi: 10.1073/pnas.1323656111
Gregan S., Wargent J., Liu L., Shinkle J., Hofmann R., Winefield C., et al. (2012). Effects of solar ultraviolet radiation and canopy manipulation on the biochemical composition of sauvignon blanc grapes. Aust. J. Grape Wine Res. 18, 227–238. doi: 10.1111/j.1755-0238.2012.00192.x
Grigg D. P. (2017). An investigation into the effect of grapevine age on vine performance, grape and wine composition, sensory evaluation and epigenetic characterisation (University of Adelaide, School of Agriculture, Food and Wine). doi: 10.25909/5b3d6e8347ffc
Grigg D., Methven D., De Bei R., Rodríguez López C., Dry P., Collins C. (2018). Effect of vine age on vine performance of Shiraz in the barossa valley, Australia. Aust. J. Grape Wine Res. 24, 75–87. doi: 10.1111/ajgw.12312
Guerra B., Steenwerth K. (2012). Influence of floor management technique on grapevine growth, disease pressure, and juice and wine composition: a review. Am. J. Enology Viticulture 63, 149–164. doi: 10.5344/ajev.2011.10001
Guilpart N., Metay A., Gary C. (2014). Grapevine bud fertility and number of berries per bunch are determined by water and nitrogen stress around flowering in the previous year. Eur. J. Agron. 54, 9–20. doi: 10.1016/j.eja.2013.11.002
Gullino M. L., Pugliese M., Gilardi G., Garibaldi A. (2018). Effect of increased CO2 and temperature on plant diseases: a critical appraisal of results obtained in studies carried out under controlled environment facilities. J. Plant Pathol. 100, 371–389. doi: 10.1007/s42161-018-0125-8
Gupta C., Salgotra R. K. (2022). Epigenetics and its role in effecting agronomical traits. Front. Plant Sci. 13. doi: 10.3389/fpls.2022.925688
Hake K., Romeis T. (2019). Protein kinase-mediated signalling in priming: immune signal initiation, propagation, and establishment of long-term pathogen resistance in plants. Plant Cell Environ. 42, 904–917. doi: 10.1111/pce.13429
Hale C., Buttrose M. (1974). Effect of temperature on ontogeny of berries of Vitis vinifera l. cv. Cabernet Sauvignon1. J. Am. Soc. Hortic. Sci. 99, 390–394. doi: 10.21273/JASHS.99.5.390
Hauben M., Haesendonckx B., Standaert E., van der Kelen K., Azmi A., Akpo H., et al. (2009). Energy use efficiency is characterized by an epigenetic component that can be directed through artificial selection to increase yield. Proc. Natl. Acad. Sci. 106, 20109–20114. doi: 10.1073/pnas.0908755106
He Y., Li Z. (2018). Epigenetic environmental memories in plants: establishment, maintenance, and reprogramming. Trends Genet. 34, 856–866. doi: 10.1016/j.tig.2018.07.006
He F., Mu L., Yan G. L., Liang N. N., Pan Q. H., Wang J., et al. (2010). Biosynthesis of anthocyanins and their regulation in colored grapes. Molecules 15 (12), 9057–9091. doi: 10.3390/molecules15129057
Heil M., Karban R. (2010). Explaining evolution of plant communication by airborne signals. Trends Ecol. Evol. 25, 137–144. doi: 10.1016/j.tree.2009.09.010
Herrera J. C., Hochberg U., Degu A., Sabbatini P., Lazarovitch N., Castellarin S. D., et al. (2017). Grape metabolic response to postveraison water deficit is affected by interseason weather variability. J. Agric. Food Chem. 65, 5868–5878. doi: 10.1021/acs.jafc.7b01466
Hickey C. C., Smith E. D., Cao S., Conner P. (2019). Muscadine (Vitis rotundifolia michx., syn. Muscandinia rotundifolia (Michx.) small): the resilient, native grape of the southeastern US. Agriculture 9, 131. doi: 10.1038/s41587-019-0152-9
Hilker M., Schmülling T. (2019). Stress priming, memory, and signalling in plants (Wiley online library). Plant Cell Environment 42, 753–761. doi: 10.1111/pce.13526
Hirayama T., Shinozaki K. (2010). Research on plant abiotic stress responses in the post-genome era: past, present and future. Plant J. 61, 1041–1052. doi: 10.1111/j.1365-313X.2010.04124.x
Hochberg U., Degu A., Cramer G. R., Rachmilevitch S., Fait A. (2015). Cultivar specific metabolic changes in grapevines berry skins in relation to deficit irrigation and hydraulic behavior. Plant Physiol. Biochem. 88, 42–52. doi: 10.1016/j.plaphy.2015.01.006
Hofmeister B. T., Lee K., Rohr N. A., Hall D. W., Schmitz R. J. (2017). Stable inheritance of DNA methylation allows creation of epigenotype maps and the study of epiallele inheritance patterns in the absence of genetic variation. Genome Biol. 18, 1–16. doi: 10.1186/s13059-017-1288-x
Hopper D. W., Ghan R., Schlauch K. A., Cramer G. R. (2016). Transcriptomic network analyses of leaf dehydration responses identify highly connected ABA and ethylene signaling hubs in three grapevine species differing in drought tolerance. BMC Plant Niology 16, 1–20. doi: 10.1186/s12870-016-0804-6
Hu L., Li N., Xu C., Zhong S., Lin X., Yang J., et al. (2014). Mutation of a major CG methylase in rice causes genome-wide hypomethylation, dysregulated genome expression, and seedling lethality. Proc. Natl. Acad. Sci. 111, 10642–10647. doi: 10.1073/pnas.1410761111
Hu Y., Morota G., Rosa G. J., Gianola D. (2015). Prediction of plant height in Arabidopsis thaliana using DNA methylation data. Genetics 201, 779–793. doi: 10.1534/genetics.115.177204
Iacono F., Sommer K. J. (1996). Photoinhibition of photosynthesis and photorespiration in Vitis vinifera under field conditions–effects of light climate and leaf position. Aust. J. Grape Wine Res. 2, 1–11. doi: 10.1111/j.1755-0238.1996.tb00089.x
IPCC (2018). "Global Warming of 1.5 °C" in An IPCC Special Report on the impacts of global warming of 1.5 °C above pre-industrial levels and related global greenhouse gas emission pathways, in the context of strengthening the global response to the threat of climate change, sustainable development, and efforts to eradicate poverty. Eds. V. Masson-Delmotte, P. Zhai, H. O. Pörtner, D. Roberts, J. Skea, P. R. Shukla, A. Pirani, W. Moufouma-Okia, C. Péan, R. Pidcock, S. Connors, J. B. R. Matthews, Y. Chen, X. Zhou, M. I. Gomis, E. Lonnoy, T. Maycock, M. Tignor, T. Waterfield.
Ito S., Shen L., Dai Q., Wu S. C., Collins L. B., Swenberg J. A., et al. (2011). Tet proteins can convert 5-methylcytosine to 5-formylcytosine and 5-carboxylcytosine. Science 333, 1300–1303. doi: 10.1126/science.1210597
Iwasaki M. (2015). Chromatin resetting mechanisms preventing transgenerational inheritance of epigenetic states. Front. Plant Sci. 6, 380. doi: 10.3389/fpls.2015.00380
Iwasaki M., Paszkowski J. (2014). Epigenetic memory in plants. EMBO J. 33, 1987–1998. doi: 10.15252/embj.201488883
Jackson D., Lombard P. (1993). Environmental and management practices affecting grape composition and wine quality-a review. Am. J. Enology Viticulture 44, 409–430. doi: 10.5344/ajev.1993.44.4.409
Jaillon O., Aury J. M., Noel B., Plicriti A., Clepet C., Casagrande A., et al. (2007). The grapevine genome sequence suggests ancestral hexaploidization in major angiosperm phyla. nature 449, 463–467. doi: 10.1038/nature06148
Jež-Krebelj A., Rupnik-Cigoj M., Stele M., Chersicola M., Pompe-Novak M., Sivilotti P. (2022). The physiological impact of GFLV virus infection on grapevine water status: first observations. Plants 11, 161. doi: 10.3390/plants11020161
Jia H., Zhang Z., Sadeghnezhad E., Pang Q., Li S., Pervaiz T., et al. (2020). Demethylation alters transcriptome profiling of buds and leaves in ‘Kyoho’grape. BMC Plant Biol. 20, 1–16. doi: 10.1186/s12870-020-02754-0
Jin B., Li Y., Robertson K. D. (2011). DNA Methylation: superior or subordinate in the epigenetic hierarchy? Genes Cancer 2, 607–617. doi: 10.1177/1947601910393957
Johnson L., Roczen D., Youkhana S., Nemani R., Bosch D. (2003). Mapping vineyard leaf area with multispectral satellite imagery. Comput. Electron. Agric. 38 (1), 33–44. doi: 10.1016/S0168-1699(02)00106-0
Johnson L. J., Tricker P. J. (2010). Epigenomic plasticity within populations: its evolutionary significance and potential. Heredity 105 (1), 113–121. doi: 10.1038/hdy.2010.25
Johnson L. M., Du J., Hale C. J., Bischof S., Feng S., Chodavarapu R. K., et al. (2014). SRA-And SET-domain-containing proteins link RNA polymerase V occupancy to DNA methylation. Nature 507, 124–128. doi: 10.1038/nature12931
Jones G. V. (2006). Climate and terroir: impacts of climate variability and change on wine. Geosci. Canada Reprint Ser. 9, 203–217.
Jones G. V., Alves F. (2012). Impact of climate change on wine production: a global overview and regional assessment in the douro valley of Portugal. Int. J. Global Warming 4, 383–406. doi: 10.1504/IJGW.2012.049448
Jones G. V., Davis R. E. (2000). Climate influences on grapevine phenology, grape composition, and wine production and quality for Bordeaux, France. Am. J. Enology Viticulture 51, 249–261. doi: 10.5344/ajev.2000.51.3.249
Kakoulidou I., Avramidou E. V., Baránek M., Brunel-Muguet S., Farrona S., Johannes F., et al. (2021). Epigenetics for crop improvement in times of global change. Biology 10, 766. doi: 10.3390/biology10080766
Kawakatsu T., Ecker J. R. (2019). Diversity and dynamics of DNA methylation: epigenomic resources and tools for crop breeding. Breed. Sci. 69, 191–204. doi: 10.1270/jsbbs.19005
Khan A. R., Enjalbert J., Marsollier A.-C., Rousselet A., Goldringer I., Vitte C. (2013). Vernalization treatment induces site-specific DNA hypermethylation at the VERNALIZATION-A1 (VRN-A1) locus in hexaploid winter wheat. BMC Plant Biol. 13, 1–16. doi: 10.1186/1471-2229-13-209
Kiselev K. V., Ogneva Z. V., Suprun A. R., Grigorchuk V. P., Dubrovina A. S. (2019). Action of ultraviolet-c radiation and p-coumaric acid on stilbene accumulation and expression of stilbene biosynthesis-related genes in the grapevine Vitis amurensis rupr. Acta Physiologiae Plantarum 41, 1–5. doi: 10.1007/s11738-019-2818-9
Kiselev K., Tyunin A., Karetin Y. (2013). Influence of 5-azacytidine and salicylic acid on demethylase gene expression in cell cultures of Vitis amurensis rupr. Acta Physiologiae Plantarum 35, 1843–1851. doi: 10.1007/s11738-013-1222-0
Koetle M. J., Snyman S. J., Rutherford R. S. (2022). Ex vitro morpho-physiological screening of drought tolerant sugarcane epimutants generated Via 5-azacytidine and imidacloprid treatments. Trop. Plant Biol. 15, 288–300. doi: 10.1007/s12042-022-09323-9
Kolb C. A., Kopecký J., Riederer M., Pfündel E. E. (2003). UV Screening by phenolics in berries of grapevine (Vitis vinifera). Funct. Plant Biol. 30, 1177–1186. doi: 10.1071/FP03076
Konate M., Wilkinson M. J., Mayne B. T., Pederson S. M., Scott E. S., Berger B., et al. (2018). Salt stress induces non-CG methylation in coding regions of barley seedlings (Hordeum vulgare). Epigenomes 2, 12. doi: 10.3390/epigenomes2020012
Kumar S., Mohapatra T. (2021). Dynamics of DNA methylation and its functions in plant growth and development. Front. Plant Sci. 12, 596236. doi: 10.3389/fpls.2021.596236
Kundariya H., Yang X., Morton K., Sanchez R., Axtell M. J., Hutton S. F., et al. (2020). MSH1-induced heritable enhanced growth vigor through grafting is associated with the RdDM pathway in plants. Nat. Commun. 11, 1–14. doi: 10.1038/s41467-020-19140-x
Lämke J., Bäurle I. (2017). Epigenetic and chromatin-based mechanisms in environmental stress adaptation and stress memory in plants. Genome Biol. 18, 1–11. doi: 10.1186/s13059-017-1263-6
Lämke J., Brzezinka K., Altmann S., Bäurle I. (2016). A hit-and-run heat shock factor governs sustained histone methylation and transcriptional stress memory. EMBO J. 35, 162–175. doi: 10.15252/embj.201592593
Lee S.-I., Kim N.-S. (2014). Transposable elements and genome size variations in plants. Genomics Inf. 12, 87–97. doi: 10.5808/GI.2014.12.3.87
Le Gac A.-L., Lafon-Placette C., Chauveau D., Segura V., Delaunay A., Fichot R., et al. (2018). Winter-dormant shoot apical meristem in poplar trees shows environmental epigenetic memory. J. Exp. Bot. 69, 4821–4837. doi: 10.1093/jxb/ery271
Lewsey M. G., Hardcastle T. J., Melnyk C. W., Molnar A., Valli A., Urich M. A., et al. (2016). Mobile small RNAs regulate genome-wide DNA methylation. Proc. Natl. Acad. Sci. 113, E801–E810. doi: 10.1073/pnas.1515072113
Li Q., Eichten S. R., Hermanson P. J., Zaunbrecher V. M., Song J., Wendt J., et al. (2014). Genetic perturbation of the maize methylome. Plant Cell 26, 4602–4616. doi: 10.1105/tpc.114.133140
Likar M., Vogel-Mikuš K., Potisek M., Hančević K., Radić T., Nečemer M., et al. (2015). Importance of soil and vineyard management in the determination of grapevine mineral composition. Sci. Total Environ. 505, 724–731. doi: 10.1016/j.scitotenv.2014.10.057
Liu H., Able A. J., Able J. A. (2022). Priming crops for the future: rewiring stress memory. Trends Plant Sci. 27, 699–716. doi: 10.1016/j.tplants.2021.11.015
Liu H.c., Lämke J., Lin S.y., Hung M. J., Liu K. M., Charng Y.y., et al. (2018). Distinct heat shock factors and chromatin modifications mediate the organ-autonomous transcriptional memory of heat stress. Plant J. 95, 401–413. doi: 10.1111/tpj.13958
Liu T., Li Y., Duan W., Huang F., Hou X. (2017). Cold acclimation alters DNA methylation patterns and confers tolerance to heat and increases growth rate in Brassica rapa. J. Exp. Bot. 68, 1213–1224. doi: 10.1093/jxb/erw496
Liu C., Lu F., Cui X., Cao X. (2010). Histone methylation in higher plants. Annu. Rev. Plant Biol. 61, 395–420. doi: 10.1146/annurev.arplant.043008.091939
Liu B., Xu X.-Q., Cai J., Lan Y.-B., Zhu B.-Q., Wang J. (2015). The free and enzyme-released volatile compounds of distinctive Vitis amurensis var. zuoshanyi grapes in China. Eur. Food Res. Technol. 240, 985–997. doi: 10.1007/s00217-014-2403-9
López M. E., Roquis D., Becker C., Denoyes B., Bucher E. (2022). DNA Methylation dynamics during stress response in woodland strawberry (Fragaria vesca). Horticulture Res. 9, uhac174. doi: 10.1093/hr/uhac174
López Sánchez A., Pascual-Pardo D., Furci L., Roberts M. R., Ton J. (2021). Costs and benefits of transgenerational induced resistance in Arabidopsis. Front. Plant Sci. 12, 644999. doi: 10.3389/fpls.2021.644999
Luo H.-B., Ma L., Xi H.-F., Duan W., Li S.-H., Loescher W., et al. (2011). Photosynthetic responses to heat treatments at different temperatures and following recovery in grapevine (Vitis amurensis l.) leaves. PloS One 6, e23033. doi: 10.1371/journal.pone.0023033
Mackenzie D., Christy A. (2005). The role of soil chemistry in wine grape quality and sustainable soil management in vineyards. Water Sci. Technol. 51, 27–37. doi: 10.2166/wst.2005.0004
Magris G., Di Gaspero G., Marroni F., Zenoni S., Tornielli G. B., Celii M., et al. (2019). Genetic, epigenetic and genomic effects on variation of gene expression among grape varieties. Plant J. 99, 895–909. doi: 10.1111/tpj.14370
Maillot P., Lebel S., Schellenbaum P., Jacques A., Walter B. (2009). Differential regulation of SERK, LEC1-like and pathogenesis-related genes during indirect secondary somatic embryogenesis in grapevine. Plant Physiol. Biochem. 47, 743–752. doi: 10.1016/j.plaphy.2009.03.016
Marfil C., Ibañez V., Alonso R., Varela A., Bottini R., Masuelli R., et al. (2019). Changes in grapevine DNA methylation and polyphenols content induced by solar ultraviolet-b radiation, water deficit and abscisic acid spray treatments. Plant Physiol. Biochem. 135, 287–294. doi: 10.1016/j.plaphy.2018.12.021
Martínez-Lüscher J., Kizildeniz T., Vučetić V., Dai Z., Luedeling E., van Leeuwen C., et al. (2016). Sensitivity of grapevine phenology to water availability, temperature and CO2 concentration. Front. Environ. Sci. 4, 48. doi: 10.3389/fenvs.2016.00048
Martínez-Lüscher J., Kurtural S. K. (2021). Same season and carry-over effects of source-sink sdjustments on grapevine yields and non-structural carbohydrates. Front. Plant Sci. 12, 695319. doi: 10.3389/fpls.2021.695319
Matzke M. A., Mosher R. A. (2014). RNA-Directed DNA methylation: an epigenetic pathway of increasing complexity. Nat. Rev. Genet. 15, 394–408. doi: 10.1038/nrg3683
Mirás-Avalos J. M., Intrigliolo D. S. (2017). Grape composition under abiotic constrains: water stress and salinity. Front. Plant Sci. 8, 851. doi: 10.3389/fpls.2017.00851
Miryeganeh M. (2021). Plants’ epigenetic mechanisms and abiotic stress. Genes 12, 1106. doi: 10.3390/genes12081106
Mittal A., Gampala S. S., Ritchie G. L., Payton P., Burke J. J., Rock C. D. (2014). Related to ABA-Insensitive3 (ABI 3)/Viviparous1 and At ABI 5 transcription factor coexpression in cotton enhances drought stress adaptation. Plant Biotechnol. J. 12, 578–589. doi: 10.1111/pbi.12162
Montanaro G., Briglia N., Lopez L., Amato D., Panara F., Petrozza A., et al. (2022). A synthetic cytokinin primes photosynthetic and growth response in grapevine under ion-independent salinity stress. J. Plant Interact. 17, 789–800. doi: 10.1080/17429145.2022.2102259
Morales-Cruz A., Aguirre-Liguori J. A., Zhou Y., Minio A., Riaz S., Walker A.M., et al. (2021). Introgression among north American wild grapes (Vitis) fuels biotic and abiotic adaptation. Genome Biol. 22 (1), 1–27. doi: 10.1186/s13059-021-02467-z
Mori K., Sugaya S., Gemma H. (2005). Decreased anthocyanin biosynthesis in grape berries grown under elevated night temperature condition. Scientia Hortic. 105, 319–330. doi: 10.1016/j.scienta.2005.01.032
Mozgova I., Mikulski P., Pecinka A., Farrona S. (2019). “Epigenetic mechanisms of abiotic stress response and memory in plants,” in Epigenetics in plants of agronomic importance: fundamentals and applications. Eds. Alvarez-Venegas R., De-la-Peña C., Casas-Mollano J. (Cham: Springer). doi: 10.1007/978-3-030-14760-0_1
Muganu M., Paolocci M., Gnisci D., Barnaba F., Bellincontro A., Mencarelli F., et al. (2013). Effect of different soil management practices on grapevine growth and on berry quality assessed by NIR-AOTF spectroscopy. Acta Hortic. 978, 117–125. doi: 10.17660/ActaHortic.2013.978.12
Mullins M. G., Bouquet A., Williams L. E. (1992). Biology of the grapevine (Cambridge University Press).
Myles S., Boyko A. R., Owens C. L., Brown P. J., Grassi F., Aradhya M. K., et al. (2011). Genetic structure and domestication history of the grape. Proc. Natl. Acad. Sci. 108, 3530–3535. doi: 10.1073/pnas.1009363108
N'Diaye A., Byrns B., Cory A. T., Nilsen K. T., Walkowiak S., Sharpe A., et al. (2020). Machine learning analyses of methylation profiles uncovers tissue-specific gene expression patterns in wheat. Plant Genome 13, e20027. doi: 10.1002/tpg2.20027
Nerva L., Guaschino M., Pagliarani C., De Rosso M., Lovisolo C., Chitarra W. (2022). Spray-induced gene silencing targeting a glutathione s-transferase gene improves resilience to drought in grapevine. Plant Cell Environ. 45, 347–361. doi: 10.1111/pce.14228
Núñez-Olivera E., Martínez-Abaigar J., Tomás R., Otero S., Arróniz-Crespo M. (2006). Physiological effects of solar ultraviolet-b exclusion on two cultivars of Vitis vinifera l. from la rioja, Spain. Am. J. Enology Viticulture 57, 441–448. doi: 10.5344/ajev.2006.57.4.441
Nuthikattu S., McCue A. D., Panda K., Fultz D., DeFraia C., Thomas E. N., et al. (2013). The initiation of epigenetic silencing of active transposable elements is triggered by RDR6 and 21-22 nucleotide small interfering RNAs. Plant Physiol. 162, 116–131. doi: 10.1104/pp.113.216481
Ollat N., Bordenave L., Tandonnet J. P., Boursiquot J. M., Marguerit E. (2016). Grapevine rootstocks: origins and perspectives. Acta Hortic. 1136, 11–22. doi: 10.17660/ActaHortic.2016.1136.2
Pagay V., Furlan T. S., Kidman C. M., Nagahatenna D. (2022). Long-term drought adaptation of unirrigated grapevines (Vitis vinifera l.). Theor. Exp. Plant Physiol. 34, 215–225. doi: 10.1007/s40626-022-00243-3
Palumbo M. C., Zenoni S., Fasoli M., Massonnet M., Farina L., Castiglione F., et al. (2014). Integrated network analysis identifies fight-club nodes as a class of hubs encompassing key putative switch genes that induce major transcriptome reprogramming during grapevine development. Plant Cell 26, 4617–4635. doi: 10.1105/tpc.114.133710
Park M., Keung A. J., Khalil A. S. (2016). The epigenome: the next substrate for engineering. Genome Biol. 17, 1–17. doi: 10.1186/s13059-016-1046-5
Pastore C., Dal Santo S., Zenoni S., Movahed N., Allegro G., Valentini G., et al. (2017). Whole plant temperature manipulation affects flavonoid metabolism and the transcriptome of grapevine berries. Front. Plant Sci. 8, 929. doi: 10.3389/fpls.2017.00929
Pecinka A., Chevalier C., Colas I., Kalantidis K., Varotto S., Krugman T., et al. (2020). Chromatin dynamics during interphase and cell division: similarities and differences between model and crop plants. J. Exp. Bot. 71, 5205–5222. doi: 10.1093/jxb/erz457
Peel M. C., Finlayson B. L., McMahon T. A. (2007). Updated world map of the köppen-Geiger climate classification. Hydrology Earth system Sci. 11 (5), 1633–1644. doi: 10.5194/hess-11-1633-2007
Pellegrino A., Lebon E., Simonneau T., Wery J. (2005). Towards a simple indicator of water stress in grapevine (Vitis vinifera l.) based on the differential sensitivities of vegetative growth components. Aust. J. Grape Wine Res. 11, 306–315. doi: 10.1111/j.1755-0238.2005.tb00030.x
Perazzolli M., Roatti B., Bozza E., Pertot I. (2011). Trichoderma harzianum T39 induces resistance against downy mildew by priming for defense without costs for grapevine. Biol. control 58, 74–82. doi: 10.1016/j.biocontrol.2011.04.006
Pereira G., Pereira J., Santos R. B., Figueiredo A. (2022). Uncovering the role of DNA methyltransferases in grapevine–Plasmopara viticola interaction: from genome-wide characterization to global methylation patterns. Gene 837, 146693. doi: 10.1016/j.gene.2022.146693
Perrone A., Martinelli F. (2020). Plant stress biology in epigenomic era. Plant Sci. 294, 110376. doi: 10.1016/j.plantsci.2019.110376
Rajnović T., Vokurka A., Bolarić S. (2020). Epigenetics in plant breeding. J. Cent. Eur. Agric. 21, 56–61. doi: 10.5513/JCEA01/21.1.2765
Raju S. K. K., Shao M. R., Sanchez R., Xu Y. Z., Sandhu A., Graef G., et al. (2018). An epigenetic breeding system in soybean for increased yield and stability. Plant Biotechnol. J. 16, 1836–1847. doi: 10.1111/pbi.12919
Rattanakon S., Ghan R., Gambetta G. A., Deluc L. G., Schlauch K. A., Cramer G. R. (2016). Abscisic acid transcriptomic signaling varies with grapevine organ. BMC Plant Biol. 16, 1–14. doi: 10.1186/s12870-016-0763-y
Raza A., Razzaq A., Mehmood S. S., Zou X., Zhang X., Lv Y., et al. (2019). Impact of climate change on crops adaptation and strategies to tackle its outcome: a review. Plants 8, 34. doi: 10.3390/plants8020034
Repka V. (2006). Early defence responses induced by two distinct elicitors derived from a Botrytis cinerea in grapevine leaves and cell suspensions. Biol. Plantarum 50, 94–106. doi: 10.1007/s10535-005-0080-z
Rienth M., Torregrosa L., Luchaire N., Chatbanyong R., Lecourieux D., Kelly M. T., et al. (2014). Day and night heat stress trigger different transcriptomic responses in green and ripening grapevine (Vitis vinifera) fruit. BMC Plant Biol. 14, 1–18. doi: 10.1186/1471-2229-14-108
Rienth M., Torregrosa L., Sarah G., Ardisson M., Brillouet J.-M., Romieu C. (2016). Temperature desynchronizes sugar and organic acid metabolism in ripening grapevine fruits and remodels their transcriptome. BMC Plant Biol. 16, 1–23. doi: 10.1186/s12870-016-0850-0
Roberts M. R., López Sánchez A. (2019). “Plant epigenetic mechanisms in response to biotic stress,” in Epigenetics in plants of agronomic importance: fundamentals and applications (Springer), 65–113.
Rodriguez P. A., Rothballer M., Chowdhury S. P., Nussbaumer T., Gutjahr C., Falter-Braun P. (2019). Systems biology of plant-microbiome interactions. Mol. Plant 12, 804–821. doi: 10.1016/j.molp.2019.05.006
Rodríguez López C. M., Wilkinson M. J. (2015). Epi-fingerprinting and epi-interventions for improved crop production and food quality. Front. Plant Sci. 6, 397. doi: 10.3389/fpls.2015.00397
Rodríguez López C. M. (2019). Harnessing the power of epigenetic priming to improve stress tolerance in vegetative propagated perennial crops. in Plant breeding and genetics in SNA research conference. 63, 11–14.Available at: https://www.researchgate.net/publication/334399022_Harnessing_the_Power_of_Epigenetic_Priming_to_Improve_Stress_Tolerance_in_Vegetative_Propagated_Perennial_Crops.
Rogers K., Chen X. (2013). Biogenesis, turnover, and mode of action of plant microRNAs. Plant Cell 25, 2383–2399. doi: 10.1105/tpc.113.113159
Romero P., Fernandez-Fernandez J. I., Botia P. (2016). Interannual climatic variability effects on yield, berry and wine quality indices in long-term deficit irrigated grapevines, determined by multivariate analysis. Int. J. Wine Res. 8, 3–17. doi: 10.2147/IJWR.S107312
Ruiz-García L., Gago P., Martínez-Mora C., Santiago J. L., Fernádez-López D. J., Martínez M. C., et al. (2021). Evaluation and pre-selection of new grapevine genotypes resistant to downy and powdery mildew, obtained by cross-breeding programs in Spain. Front. Plant Sci. 12. doi: 10.3389/fpls.2021.674510
Salón J. L., Chirivella C., Castel J. R. (2005). Response of cv. bobal to timing of deficit irrigation in requena, Spain: water relations, yield, and wine quality. Am. J. Enology Viticulture 56, 1–8. doi: 10.5344/ajev.2005.56.1.1
Sanguankeo P. P., Leon R. G., Malone J. (2009). Impact of weed management practices on grapevine growth and yield components. Weed Sci. 57, 103–107. doi: 10.1614/WS-08-100.1
Sani E., Herzyk P., Perrella G., Colot V., Amtmann A. (2013). Hyperosmotic priming of arabidopsis seedlings establishes a long-term somatic memory accompanied by specific changes of the epigenome. Genome Biol. 14, 1–24. doi: 10.1186/gb-2013-14-6-r59
Santos J. A., Fraga H., Malheiro A. C., Moutinho-Pereira J., Dinis L.-T., Correia C., et al. (2020). A review of the potential climate change impacts and adaptation options for European viticulture. Appl. Sci. 10, 3092. doi: 10.3390/app10093092
Satisha J., Dasharath P. O., Amruta N. V., Smita R. M., Ajay K. S., Ramhari G. S. (2013). Influence of canopy management practices on fruit composition of wine grape cultivars grown in semi-arid tropical region of India. Afr. J. Agric. Res. 8, 3462–3472. doi: 10.5897/AJAR2013.7307
Savoi S., Wong D. C., Degu A., Herrera J. C., Bucchetti B., Peterlunger E., et al. (2017). Multi-omics and integrated network analyses reveal new insights into the systems relationships between metabolites, structural genes, and transcriptional regulators in developing grape berries (Vitis vinifera l.) exposed to water deficit. Front. Plant Dcience 8, 1124. doi: 10.3389/fpls.2017.01124
Schultz H. (2000). Climate change and viticulture: a European perspective on climatology, carbon dioxide and UV-b effects. Aust. J. Grape Wine Res. 6, 2–12. doi: 10.1111/j.1755-0238.2000.tb00156.x
Schultz H. R. (2010). Climate change and viticulture: research needs for facing the future. J. Wine Res. 21, 113–116. doi: 10.1080/09571264.2010.530093
Seet B. T., Dikic I., Zhou M.-M., Pawson T. (2006). Reading protein modifications with interaction domains. Nat. Rev. Mol. Cell Biol. 7, 473–483. doi: 10.1038/nrm1960
Seguin G. (1986). ‘Terroirs’ and pedology of wine growing. Experientia 42, 861–873. doi: 10.1007/BF01941763
Sharma A., Kumar V., Shahzad B., Ramakrishnan M., Singh Sidhu G. P., Bali A. S., et al. (2020). Photosynthetic response of plants under different abiotic stresses: a review. J. Plant Growth Regul. 39, 509–531. doi: 10.1007/s00344-019-10018-x
Smart R. E., Coombe B. G. (1983). Water relations of grapevines [Vitis]. Water deficits Plant Growth 7, 137–196.
Song Y., Ji D., Li S., Wang P., Li Q., Xiang F. (2012). The dynamic changes of DNA methylation and histone modifications of salt responsive transcription factor genes in soybean. PloS One 7, e41274. doi: 10.1371/journal.pone.0041274
Sow M. D., Allona I., Ambroise C., Conde D., Fichot R., Gribkova S., et al. (2018). Epigenetics in forest trees: state of the art and potential implications for breeding and management in a context of climate change. Adv. Botanical Res. 88, 387–453. doi: 10.1016/bs.abr.2018.09.003
Spayd S. E., Tarara J. M., Mee D. L., Ferguson J. (2002). Separation of sunlight and temperature effects on the composition of Vitis vinifera cv. merlot berries. American. J. Enology Viticulture 53, 171–182. doi: 10.5344/ajev.2002.53.3.171
Springer N. M., Schmitz R. J. (2017). Exploiting induced and natural epigenetic variation for crop improvement. . Nat. Rev. Genet 18, 563–575. doi: 10.1038/nrg.2017.45
Stafne E., Sleezer S., Clark J. (2015). “Grapevine breeding in the southern united states,” in Grapevine breeding programs for the wine industry (Elsevier), 379–410.
Stevenson T. (2005). The sotheby's wine encyclopedia, sotheby's wine encyclopedia series. edition 4 (Dorling Kindersley Publishers).
Steward N., Ito M., Yamaguchi Y., Koizumi N., Sano H. (2002). Periodic DNA methylation in maize nucleosomes and demethylation by environmental stress. J. Biol. Chem. 277, 37741–37746. doi: 10.1074/jbc.M204050200
Sudan J., Raina M., Singh R. (2018). Plant epigenetic mechanisms: role in abiotic stress and their generational heritability. 3 Biotech. 8, 1–12. doi: 10.1007/s13205-018-1202-6
Sunkar R., Zhu J.-K. (2004). Novel and stress-regulated microRNAs and other small RNAs from Arabidopsis. Plant Cell 16, 2001–2019. doi: 10.1105/tpc.104.022830
Surdonja K., Eggert K., Hajirezaei M.-R., Harshavardhan V. T., Seiler C., Von Wirén N., et al. (2017). Increase of DNA methylation at the HvCKX2. 1 promoter by terminal drought stress in barley. Epigenomes 1, 9. doi: 10.3390/epigenomes1020009
Tan J. W., Shinde H., Tesfamicael K., Hu Y., Fruzangohar M., Tricker P., et al. (2023). Global transcriptome and gene co-expression network analyses reveal regulatory and non-additive effects of drought and heat stress in grapevine. Front. Plant Sci. 14, 239. doi: 10.3389/fpls.2023.1096225
Tarara J. M., Lee J., Spayd S. E., Scagel C. F. (2008). Berry temperature and solar radiation alter acylation, proportion, and concentration of anthocyanin in merlot grapes. Am. J. Enology Viticulture 59, 235–247. doi: 10.5344/ajev.2008.59.3.235
Tarr P. T., Dreyer M. L., Athanas M., Shahgholi M., Saarloos K., Second T. P. (2013). A metabolomics based approach for understanding the influence of terroir in Vitis vinifera l. Metabolomics 9, 170–177. doi: 10.1007/s11306-013-0497-x
Terrier N., Ageorges A., Abbal P., Romieu C. (2001). Generation of ESTs from grape berry at various developmental stages. J. Plant Physiol. 158, 1575–1583. doi: 10.1078/0176-1617-00566
Terrier N., Glissant D., Grimplet J., Barrieu F., Abbal P., Couture C., et al. (2005). Isogene specific oligo arrays reveal multifaceted changes in gene expression during grape berry (Vitis vinifera l.) development. Planta 222, 832–847. doi: 10.1007/s00425-005-0017-y
Tesic D., Keller M., Hutton R. J. (2007). Influence of vineyard floor management practices on grapevine vegetative growth, yield, and fruit composition. Am. J. Enology Viticulture 58, 1–11. doi: 10.5344/ajev.2007.58.1.1
Tirnaz S., Batley J. (2019). DNA Methylation: toward crop disease resistance improvement. Trends Plant Sci. 24, 1137–1150. doi: 10.1016/j.tplants.2019.08.007
Tombesi S., Frioni T., Poni S., Palliotti A. (2018). Effect of water stress “memory” on plant behavior during subsequent drought stress. Environ. Exp. Bot. 150, 106–114. doi: 10.1016/j.envexpbot.2018.03.009
Töpfer R., Trapp O. (2022). A cool climate perspective on grapevine breeding: climate change and sustainability are driving forces for changing varieties in a traditional market. Theor. Appl. Genet. 135, 3947–3960. doi: 10.1007/s00122-022-04077-0
Tricker P. J., Gibbings J. G., Rodríguez López C. M., Hadley P., Wilkinson M. J. (2012). Low relative humidity triggers RNA-directed de novo DNA methylation and suppression of genes controlling stomatal development. J. Exp. Bot. 63, 3799–3813. doi: 10.1093/jxb/ers076
Tricker P. J., Rodríguez López C. M., Gibbings G., Hadley P., Wilkinson M. J. (2013a). Transgenerational, dynamic methylation of stomata genes in response to low relative humidity. Int. J. Mol. Sci. 14, 6674–6689. doi: 10.3390/ijms14046674
Tricker P. J., Rodríguez López C. M., Hadley P., Wagstaff C., Wilkinson M. J. (2013b). Pre-conditioning the epigenetic response to high vapor pressure deficit increases the drought tolerance of Arabidopsis thaliana. Plant Signaling Behav. 8, e25974. doi: 10.4161/psb.25974
Trouvelot S., Varnier A.-L., Allegre M., Mercier L., Baillieul F., Arnould C., et al. (2008). A β-1, 3 glucan sulfate induces resistance in grapevine against Plasmopara viticola through priming of defense responses, including HR-like cell death. Mol. Plant-Microbe Interact. 21, 232–243. doi: 10.1094/MPMI-21-2-0232
Ueda M., Seki M. (2020). Histone modifications form epigenetic regulatory networks to regulate abiotic stress response. Plant Physiol. 182, 15–26. doi: 10.1104/pp.19.00988
Van Leeuwen C., Destrac-Irvine A. (2017). Modified grape composition under climate change conditions requires adaptations in the vineyard. Oeno One 51, 147–154. doi: 10.20870/oeno-one.2017.51.2.1647
Van Leeuwen C., Friant P., Chone X., Tregoat O., Koundouras S., Dubourdieu D. (2004). Influence of climate, soil, and cultivar on terroir. American. J. Enology Viticulture 55, 207–217. doi: 10.5344/ajev.2004.55.3.207
Van Leeuwen C., Seguin G. (2006). The concept of terroir in viticulture. J. Wine Res. 17, 1–10. doi: 10.1080/09571260600633135
Vannozzi A., Dry I. B., Fasoli M., Zenoni S., Lucchin M. (2012). Genome-wide analysis of the grapevine stilbene synthase multigenic family: genomic organization and expression profiles upon biotic and abiotic stresses. BMC Plant Biol. 12, 1–22. doi: 10.1186/1471-2229-12-130
Varela A., Ibañez V. N., Alonso R., Zavallo D., Asurmendi S., Gomez Talquenca S., et al. (2021). Vineyard environments influence Malbec grapevine phenotypic traits and DNA methylation patterns in a clone-dependent way. Plant Cell Rep. 40, 111–125. doi: 10.1007/s00299-020-02617-w
Varotto S., Tani E., Abraham E., Krugman T., Kapazoglou A., Melzer R., et al. (2020). Epigenetics: possible applications in climate-smart crop breeding. J. Exp. Bot. 71, 5223–5236. doi: 10.1093/jxb/eraa188
Venios X., Korkas E., Nisiotou A., Banilas G. (2020). Grapevine responses to heat stress and global warming. Plants 9, 1754. doi: 10.3390/plants9121754
Verhagen B. W., Trotel-Aziz P., Couderchet M., Höfte M., Aziz A. (2010). Pseudomonas spp.-induced systemic resistance to Botrytis cinerea is associated with induction and priming of defence responses in grapevine. J. Exp. Bot. 61, 249–260. doi: 10.1093/jxb/erp295
Vezzulli S., Civardi S., Ferrari F., Bavaresco L. (2007). Methyl jasmonate treatment as a trigger of resveratrol synthesis in cultivated grapevine. Am. J. Enology Viticulture 58, 530–533. doi: 10.5344/ajev.2007.58.4.530
Victorino G., Braga R., Lopes C. M. (2017). The effect of topography on the spatial variability of grapevine vegetative and reproductive components. Actas Portuguesas Horticultura 29, 510–516.
Villagómez-Aranda A. L., Feregrino-Pérez A. A., García-Ortega L. F., González-Chavira M., Torres-Pacheco I., Guevara-González R. (2022). Activating stress memory: eustressors as potential tools for plant breeding. Plant Cell Rep. 41, 1481–1498. doi: 10.1007/s00299-022-02858-x
Vogel E., Donat M. G., Alexander L. V., Meinshausen M., Ray D. K., Karoly D., et al. (2019). The effects of climate extremes on global agricultural yields. Environ. Res. Lett. 14, 054010. doi: 10.1088/1748-9326/ab154b
Vojta A., Dobrinić P., Tadić V., Bočkor L., Korać P., Julg B., et al. (2016). Repurposing the CRISPR-Cas9 system for targeted DNA methylation. Nucleic Acids Res. 44 (12), 5615–5628. doi: 10.1093/nar/gkw159
Vriet C., Hennig L., Laloi C. (2015). Stress-induced chromatin changes in plants: of memories, metabolites and crop improvement. Cell. Mol. Life Sci. 72, 1261–1273. doi: 10.1007/s00018-014-1792-z
Waddington C. H. (1942). Canalization of development and the inheritance of acquired characters. Nature 150, 563–565. doi: 10.1038/150563a0
Wang X., Xin C., Cai J., Zhou Q., Dai T., Cao W., et al. (2016). Heat priming induces trans-generational tolerance to high temperature stress in wheat. Front. Plant Sci. 7, 501. doi: 10.3389/fpls.2016.00501
Wei J.-W., Huang K., Yang C., Kang C.-S. (2017). Non-coding RNAs as regulators in epigenetics. Oncol. Rep. 37, 3–9. doi: 10.3892/or.2016.5236
White M. A., Diffenbaugh N., Jones G. V., Pal J., Giorgi F. (2006). Extreme heat reduces and shifts united states premium wine production in the 21st century. Proc. Natl. Acad. Sci. 103, 11217–11222. doi: 10.1073/pnas.0603230103
Wibowo A., Becker C., Marconi G., Durr J., Price J., Hagmann J., et al. (2016). Hyperosmotic stress memory in Arabidopsis is mediated by distinct epigenetically labile sites in the genome and is restricted in the male germline by DNA glycosylase activity. Elife 5, e13546. doi: 10.7554/eLife.13546.044
Wu C.-T., Morris J. R. (2001). Genes, genetics, and epigenetics: a correspondence. Science 293, 1103–1105. doi: 10.1126/science.293.5532.1103
Xiao F., Yang Z., Lee K. (2017). Photosynthetic and physiological responses to high temperature in grapevine (Vitis vinifera l.) leaves during the seedling stage. J. Hortic. Sci. Biotechnol. 92, 2–10. doi: 10.1080/14620316.2016.1211493
Xie H., Konate M., Sai N., Tesfamicael K. G., Cavagnaro T., Gilliham M., et al. (2017). Global DNA methylation patterns can play a role in defining terroir in grapevine (Vitis vinifera cv. Shiraz). Front. Plant Sci. 8, 1860. doi: 10.3389/fpls.2017.01860
Yang S., Fresnedo-Ramírez J., Sun Q., Manns D. C., Sacks G. L., Mansfield A. K., et al. (2016). Next generation mapping of enological traits in an F2 interspecific grapevine hybrid family. PloS One 11, e0149560. doi: 10.1371/journal.pone.0149560
Yang X., Han H., De Carvalho D. D., Lay F. D., Jones P. A., Liang G. (2014). Gene body methylation can alter gene expression and is a therapeutic target in cancer. Cancer Cell 26, 577–590. doi: 10.1016/j.ccr.2014.07.028
Yang X., Kundariya H., Xu Y.-Z., Sandhu A., Yu J., Hutton S. F., et al. (2015). MutS HOMOLOG1-derived epigenetic breeding potential in tomato. Plant Physiol. 168, 222–232. doi: 10.1104/pp.15.00075
Yang X., Sanchez R., Kundariya H., Maher T., Dopp I., Schwegel R., et al. (2020). Segregation of an MSH1 RNAi transgene produces heritable non-genetic memory in association with methylome reprogramming. Nat. Commun. 11, 1–17. doi: 10.1038/s41467-020-16036-8
Yang L., Wen K.-S., Ruan X., Zhao Y.-X., Wei F., Wang Q. (2018). Response of plant secondary metabolites to environmental factors. Molecules 23, 762. doi: 10.3390/molecules23040762
Yau I.-H., Davenport J. R., Rupp R. A. (2013). Characterizing inland pacific Northwest American viticultural areas with geospatial data. PloS One 8, e61994. doi: 10.1371/journal.pone.0061994
Yong-Villalobos L., González-Morales S. I., Wrobel K., Gutiérrez-Alanis D., Cervantes-Peréz S. A., Hayano-Kanashiro C., et al. (2015). Methylome analysis reveals an important role for epigenetic changes in the regulation of the Arabidopsis response to phosphate starvation. Proc. Natl. Acad. Sci. 112, E7293–E7302. doi: 10.1073/pnas.1522301112
Yu Y., Zhang Y., Yin L., Lu J. (2012). The mode of host resistance to Plasmopara viticola infection of grapevines. Phytopathology 102, 1094–1101. doi: 10.1094/PHYTO-02-12-0028-R
Zapata D., Salazar-Gutierrez M., Chaves B., Keller M., Hoogenboom G. (2017). Predicting key phenological stages for 17 grapevine cultivars (Vitis vinifera l.). Am. J. Enology Viticulture 68, 60–72. doi: 10.5344/ajev.2016.15077
Zemach A., Kim M. Y., Hsieh P.-H., Coleman-Derr D., Eshed-Williams L., Thao K., et al. (2013). The Arabidopsis nucleosome remodeler DDM1 allows DNA methyltransferases to access H1-containing heterochromatin. Cell 153, 193–205. doi: 10.1016/j.cell.2013.02.033
Zenoni S., Ferrarini A., Giacomelli E., Xumerle L., Fasoli M., Malerba G., et al. (2010). Characterization of transcriptional complexity during berry development in Vitis vinifera using RNA-seq. Plant Physiol. 152, 1787–1795. doi: 10.1104/pp.109.149716
Zha Q., Xi X., He Y., Jiang A. (2020). Transcriptomic analysis of the leaves of two grapevine cultivars under high-temperature stress. Scientia Hortic. 265, 109265. doi: 10.1016/j.scienta.2020.109265
Zhang H., Lang Z., Zhu J.-K. (2018a). Dynamics and function of DNA methylation in plants. Nat. Rev. Mol. Cell Biol. 19, 489–506. doi: 10.1038/s41580-018-0016-z
Zhang Q., Liang Z., Cui X., Ji C., Li Y., Zhang P., et al. (2018b). N6-methyladenine DNA methylation in Japonica and Indica rice genomes and its association with gene expression, plant development, and stress responses. Mol. Plant 11, 1492–1508. doi: 10.1016/j.molp.2018.11.005
Zhao C., Liu B., Piao S., Wang X., Lobell D. B., Huang Y., et al. (2017). Temperature increase reduces global yields of major crops in four independent estimates. Proc. Natl. Acad. Sci. 114, 9326–9331. doi: 10.1073/pnas.1701762114
Zheng X., Chen L., Xia H., Wei H., Lou Q., Li M., et al. (2017). Transgenerational epimutations induced by multi-generation drought imposition mediate rice plant’s adaptation to drought condition. Sci. Rep. 7, 1–13. doi: 10.1038/srep39843
Zhou J., Cavagnaro T. R., De Bei R., Nelson T. M., Stephen J. R., Metcalfe A., et al. (2021). Wine terroir and the soil bacteria: an amplicon sequencing–based assessment of the barossa valley and its sub-regions. Front. Microbiol. 3358. doi: 10.3389/fmicb.2020.597944
Keywords: review (article), grapevine, perennial crops, epigenomics, epi-breeding, stress memory, stress priming
Citation: Tan JW and Rodríguez López CM (2023) Epigenomics: a new tool for the generation of climate resilient grapevines. Front. Hortic. 2:1116866. doi: 10.3389/fhort.2023.1116866
Received: 05 December 2022; Accepted: 03 July 2023;
Published: 26 July 2023.
Edited by:
Carlos Poblete-Echeverría, Stellenbosch University, South AfricaReviewed by:
Shunli Wang, Chinese Academy of Agricultural Sciences (CAAS), ChinaManjul Dutt, University of Florida, United States
Copyright © 2023 Tan and Rodríguez López. This is an open-access article distributed under the terms of the Creative Commons Attribution License (CC BY). The use, distribution or reproduction in other forums is permitted, provided the original author(s) and the copyright owner(s) are credited and that the original publication in this journal is cited, in accordance with accepted academic practice. No use, distribution or reproduction is permitted which does not comply with these terms.
*Correspondence: Jia W. Tan, amlhLnRhbkB1a3kuZWR1