- 1School of Bioengineering and Biosciences, Lovely Professional University, Phagwara, Punjab, India
- 2Indian Institute of Technology, Roorkee, Uttrakhand, India
- 3School of Agricultural Sciences, Baddi University of Emerging Sciences and Technologies, Baddi, Himachal Pradesh, India
- 4Department of Biological Sciences, Al Hussein Bin Talal University Ma’an, Ma’an, Jordan
- 5Department of Biomedical Sciences, Institute of Health, Jimma University, Jimma, Ethiopia
Heavy metal and Persistent Organic Pollutants (POPs) pollution stemming from industrialization, intensive agriculture, and other human activities pose significant environmental and health threats. These contaminants persist in the air, soil, and water, particularly in industrialized nations, adversely affecting human health and ecosystems. While physical and chemical methods exist for detoxifying contaminated soil, they often have drawbacks such as high cost and technical complexity. Bioremediation, utilizing plants and microbes, offers a promising solution. Certain microorganisms like Streptomyces, Aspergillus and plant species such as Hibiscus and Helianthus show high metal adsorption capacities, making them suitable for bioremediation. However, plants’ slow growth and limited remediation efficiency have been challenges. Recent advancements involve leveraging plant-associated microbes to enhance heavy metal removal. Additionally, nanotechnology, particularly nano-bioremediation, shows promise in efficiently removing contaminants from polluted environments by combining nanoparticles with bioremediation techniques. This review underscores bioremediation methods for heavy metals using plants and microbes, focusing on the role of Plant Growth Promoting Rhizobacteria (PGPR) in promoting phytoremediation. It also explores the implementation of nanotechnologies for eliminating metals from polluted soil, emphasizing the significance of soil microbiomes, nanoparticles, and contaminant interactions in developing effective nano-remediation strategies for optimizing agriculture in contaminated fields.
1 Introduction
Plenty of biochemical interactions in plants and animals require heavy metals (HMs), making them essential to manufacturing. When present in high concentrations, these heavy metals (HMs)/pollutants can disrupt essential metabolic activities in living beings, which makes them severely harmful pollutants in ecologically vulnerable regions (Ding et al., 2022; Mustapha and Halimoon, 2022). Natural disasters like volcanic eruptions and human activity have also contributed to this pollution of the environment. Municipal garbage, agricultural waste, and industrial activities like mining and electroplating all contain high amounts of heavy metals like lead (Pb), mercury (Hg), zinc (Zn), copper (Cu), cadmium (Cd), nickel (Ni), cobalt (Co), and chromium (Cr) (Hananingtyas et al., 2022; Mitra et al., 2022). Even though heavy metals, unlike organic materials, do not naturally degrade, the toxicity of heavy metals can be reduced through several different mechanisms, one of which is bioremediation (Kumar et al., 2022). Bioremediation has emerged as the most popular and effective solution for treating metal-polluted locations. Bioremediation refers to employing microorganisms to break down harmful contaminants in soil or water into less hazardous byproducts (Riyazuddin et al., 2022; Vanisree et al., 2022; Zaynab et al., 2022).
Plants (phytoremediation) (Gavrilescu, 2022) and microorganisms (rhizo-remediation) (Husain et al., 2022) working together in the root zone are crucial components of biological remediation (Sharma et al., 2021; Cepoi et al., 2022; Pande et al., 2022). Both in-situ and ex-situ technologies may be employed, depending on the specificity of the contaminated area. In-situ technologies are used for passive, non-invasive clean-up, while ex-situ technologies are used for cost-effective and safe clean-up (Sharma et al., 2021). In In-situ biodegradation, naturally occurring microorganisms are prompted to break down organic pollutants by supplying nutrients and oxygen via the uniform circulation of aqueous solutions extracted from soil containing hazardous heavy metals (Reddy and Parupudi, 1997). Groundwater and soil both respond well to in-situ bioremediation. Several bioremediation techniques, such as land farming and composting, are employed in ex-situ bioremediation (Concetta et al., 2013; Bandaru et al., 2020; Tufail et al., 2022), which entails the removal of contaminated soil from its original location. Table 1 outlines various in-situ and ex-situ bioremediation techniques employed for the effective removal of heavy metals and persistent organic pollutants (POPs), showcasing diverse methods essential in addressing environmental contamination challenges.
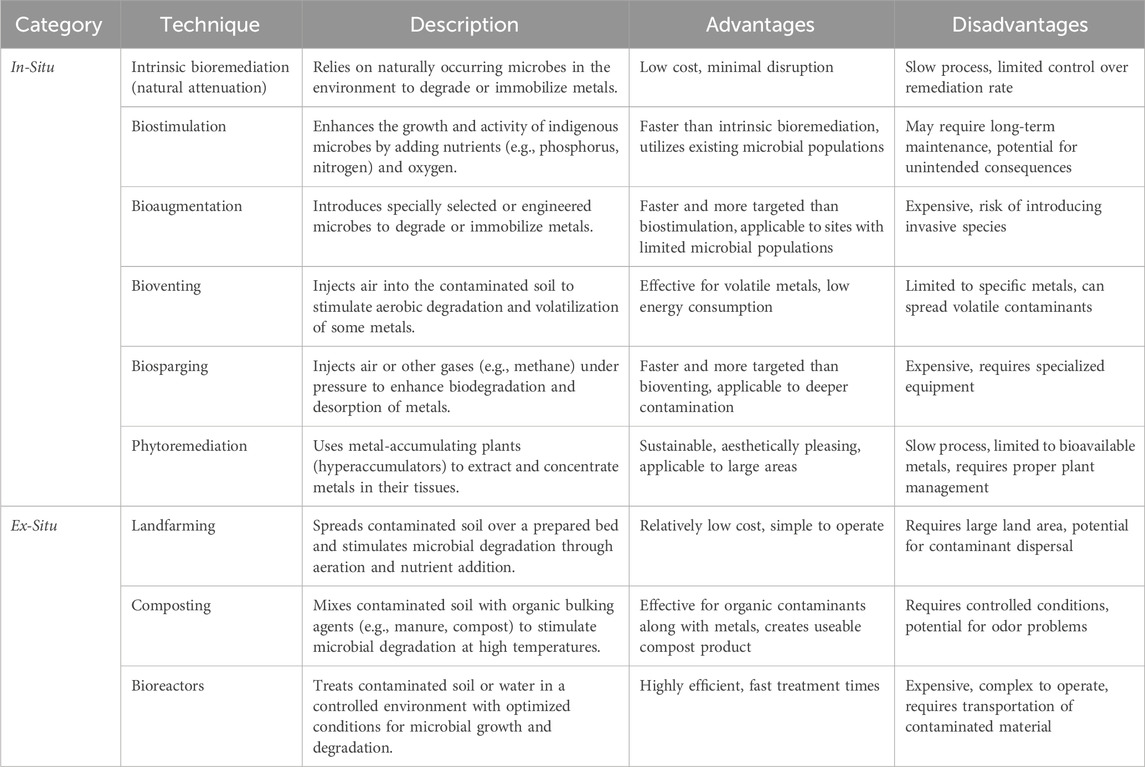
Table 1. In-situ and Ex-situ bioremediation techniques used for remediation of heavy metals and POPs (RoyChowdhury et al., 2018; DalCorso et al., 2019; Ravindra and Mor, 2019; Zwolak et al., 2019; Sharma et al., 2021; Cepoi et al., 2022; Pande et al., 2022).
Various variables and factors in bioremediation, including electron acceptors, soil type, nutrients, oxygen presence, temperature, and pH, play an important role (Zhai et al., 2018). They can thrive in various temperatures and humidity levels, and microbes are extensively versatile for these environmental conditions. The versatility of microbes and other biological agents makes them capable of removing or remediating heavy metal pollutants in the environment. However, a deeper understanding of microbial ecology and bioremediation methods is essential to fully harness their potential. While enhancing bioremediation processes holds promise, the intricate interactions between microorganisms and heavy metal contamination can present challenges that may impede successful outcomes (Jabbar et al., 2022). Several methods, like protein engineering, whole-transcriptome profiling, metabolic engineering, and rhizo-remediation, can aid bioremediation by improving heavy metal binding for detoxification and xenobiotic chemical degradation. For example, genetically altered Deinococcus geothermalis expressed the mer-operon responsible for reducing Hg (II) after being inserted from Escherichia coli. This allowed the bacteria to reduce Hg (II) contamination (Brim et al., 2003). In addition, the gram-negative strain of Ralstonia eutropha underwent genetic engineering to express a mouse protein known as metallothionein on the cell surface. This rendered the strain resistant to heavy metals (Valls et al., 2000), and when added to Cadmium-contaminated soil, bacteria have shown a significant improvement in tobacco plant growth.
2 Heavy metal and POPS pollution: threats to soil health, plant growth, and human wellbeing
Polluting the environment is a major problem that has emerged as a major obstacle in the modern world. Heavy metals (about 65) pose the greatest environmental concern (Tufail et al., 2022). Metals with densities of more than 5 g/cm3, known as “heavy metals,” are one of the world’s greatest dangers because of their widespread discharge into the environment (Roy et al., 2018; DalCorso et al., 2019; Ravindra and Mor, 2019; Zwolak et al., 2019). Various metals and metalloids like Cr, Cd, Cu, Pb, Zn, Ni, Hg, and As are discharged into the environment through sewage disposal, smelting, fertilizer applications, and industrial waste (Zwolak et al., 2019; Briffa et al., 2020; Sall et al., 2020). The available evidence indicates that a majority, exceeding 50%, of the total 10 million contaminated sites, equivalent to a land area exceeding 20 million hectares, are found to be contaminated with these substances (RoyChowdhury et al., 2018). A few HM concentrations available in soil are mentioned in Table 2.
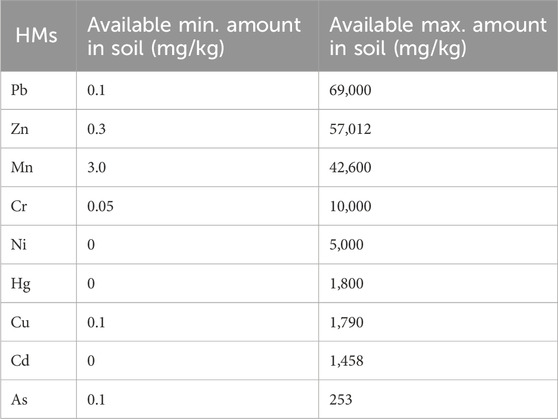
Table 2. Heavy metal minimum and maximum concentrations in soil documented worldwide (shorted as per Maximum concentration) (Saleem et al., 2022).
Adsorption and desorption affect the availability of these metals in soil; these processes are, in turn affected by a wide range of soil parameters, including calcium carbonate, clay mineral, oxidation-reduction status, cation exchange, organic matter content, pH, Mn, and Fe oxide concentrations (Islam et al., 2017; Chen and Li, 2018; Sall et al., 2020). As a result of these factors, there is a range of heavy metal types and concentrations present in soils across diverse agro-climatic regions, each with its unique features and functions. The persistent, bioaccumulative, and inert nature of these metals in the soil-plant system contributes to complex issues related to their toxicity and long half-life (Wang Q. et al., 2018). Two significant issues are predicted to arise due to heavy metal accumulation in soils: i) a decrease in soil nutrition due to changes in the microbiota (Xie et al., 2016) and ii) a decrease in human health through plants and the food chain at and near contaminated locations (Zwolak et al., 2019). Besides causing damage to the soil, even low levels of metals can pose a significant risk to plant ecosystems due to their strong reactivity. Therefore, it is essential to swiftly comprehend how metals interact with the soil, microbiome, and plants’ systems to enhance crop yields in stress-prone soils. Moreover, affordable approaches to stress management should be devised. To prevent and eliminate metal pollution, stringent regulations must be enforced by the government or private entities, and detoxification procedures must be implemented to regulate the release of heavy metals from various sources (Baruah et al., 2019; Liu et al., 2019; Dhaliwal, Singh, Taneja and Mandal, 2020). Removing heavy metals relies on or follows the regulatory standards set for soil heavy metal levels in numerous countries. These criteria can differ from one location to another and vary depending on the specific type of heavy metal in question. Also, such standards aid in developing more effective solutions for removing heavy metals from contaminated locations (Zhan et al., 2019). This review will briefly describe the toxic effects of a few metal pollutants on plants and microorganisms and how these effects can be mitigated. From a biological point of view, HMs can be broken down into two categories: necessary and harmful (Ullah et al., 2022). Micronutrients for plants and animals are called “essential metals” or “metalloids” and include elements like zinc, iron, nickel, and copper (Manoj et al., 2020). Toxic metals, classified as non-essential metals, pose severe risks even in minute quantities. Essential and non-essential metals exist in the environment as trace elements but accumulate in specific regions due to human activities like urbanization, industrialization, mining, agriculture, and smelting (Houri et al., 2020). The increased presence of Heavy Metals (HMs) in soil and the environment has garnered significant attention recently due to their widespread distribution, non-degradability, toxicity, accumulation potential, and persistence. Extensive evidence suggests that HMs adversely affect various soil properties, including physical, biological, and chemical characteristics. As a result of their prolonged presence in soil, HMs pose a significant threat to human health by enabling harmful metals to enter the food chain (Mao et al., 2019; Mitra et al., 2022). The widespread destruction of existing vegetation and the establishment of new vegetation exacerbate the adverse effects of HMs on soil surface, structure, fertility, nutrient cycles, and microbial communities. HMs indirectly impact soil enzymatic activities by altering soil microbial communities’ size, composition, and activity (Ding et al., 2022). These chemicals interfere with essential metabolic functions like respiration, denitrification, and enzyme activity, leading to a reduction in the abundance of certain microbial populations. Furthermore, HMs negatively affect the development of cell membranes, hindering microbial cell division, transcription, and protein denaturation (Sobolev and Begonia, 2008; Abdu, Abdullahi and Abdulkadir, 2017). The composition of soil, encompassing factors such as texture, clay content, organic matter, pH, as well as the presence of inorganic anions, cations, and metal speciation, all play significant roles in shaping the impact of metals on soil biology. Similarly, heavy metal (HM) contamination significantly affects soil quality, fertility, plant health, and yield. HM contamination disrupts crucial biological processes including seed germination, water regulation, photosynthesis, electron transport, stomatal conductance, CO2 assimilation, antioxidant defense mechanisms, solute balance, mineral uptake, and overall plant growth. Such disruptions can ultimately lead to plant mortality (Asati et al., 2016; Kalaivanan and Ganeshamurthy, 2016; Riyazuddin et al., 2022). Furthermore, HM toxicity impedes plant development and metabolism by causing oxidative damage to cellular structures and interfering with cytoplasmic enzymes (Kalaivanan and Ganeshamurthy, 2016). Reduced yields resulting from impaired plant growth contribute to escalating food insecurity. The extensive presence of HMs in soil poses risks to human health as these contaminants can leach into other environmental compartments such as groundwater, rivers, and crops (Briffa et al., 2020). Water exceeding allowable HM concentrations loses its quality, rendering it unsuitable for drinking and irrigation (Tan et al., 2016). HMs can enter the human body through various routes including ingestion, skin contact, food consumption, and water intake. Prolonged exposure to certain metals can have detrimental health effects, which may not manifest until years after exposure begins. The duration and intensity of exposure are critical determinants of toxicity levels (Jia et al., 2018; Mao et al., 2019; Briffa et al., 2020; Sall et al., 2020; Ding et al., 2022).
Persistent Organic Pollutants (POPs) are synthetic chemicals that endure in the environment, accumulate in organisms, and pose potential risks to human health and ecosystems (Adebusuyi et al., 2022; Tufail et al., 2022). Primarily human-made, these substances can traverse vast distances via air and water, impacting remote regions. The escalating apprehension over POPs’ adverse impacts on human and plant health is evident (refer to Table 3), underscoring the global challenge of controlling and managing these pollutants.
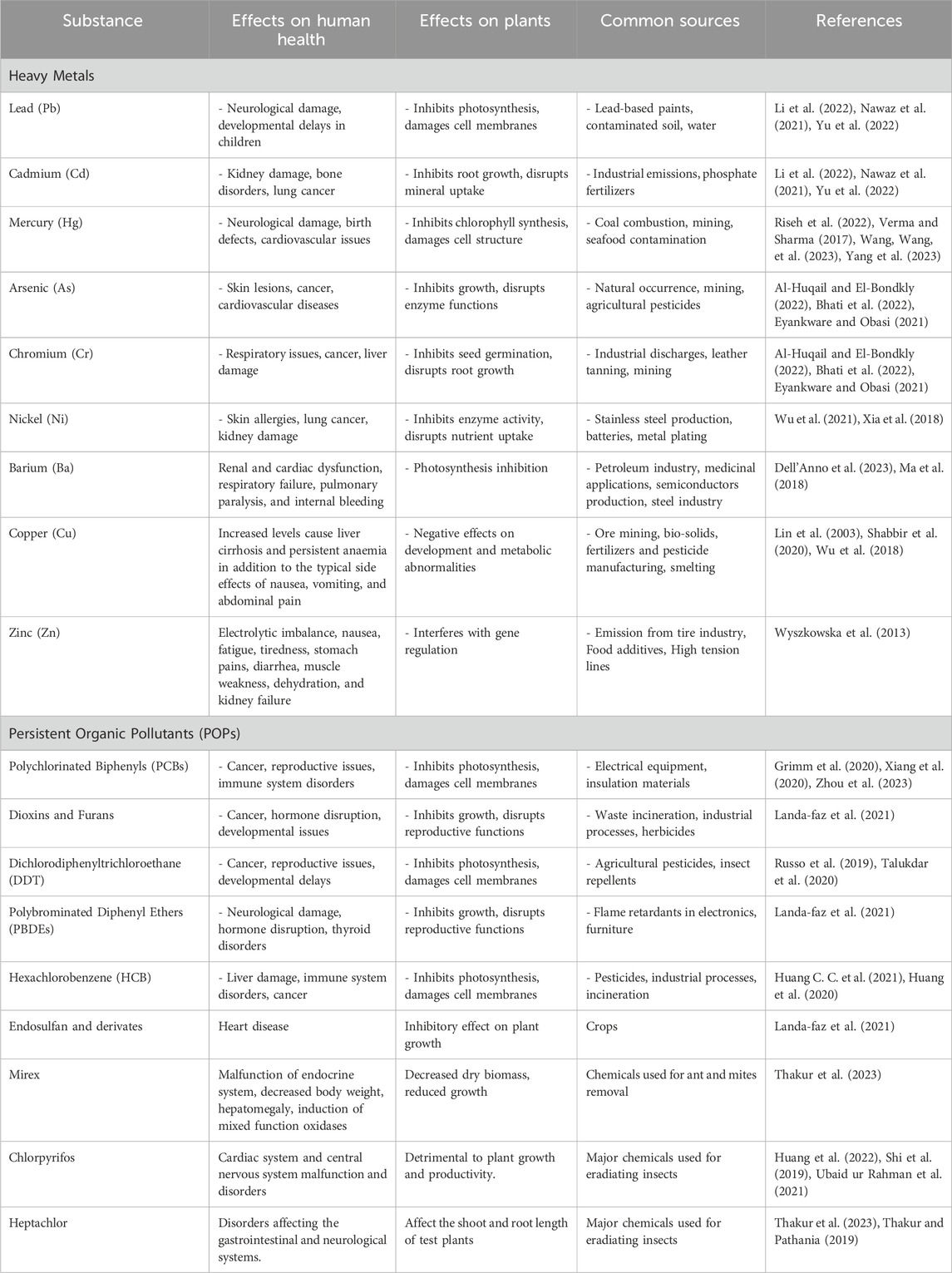
Table 3. A comprehensive overview of the effects of various heavy metals and persistent organic pollutants on both human health and plants, along with their common sources.
POPs infiltrate the human body through consumption of contaminated food, water, and air. They are lipophilic and accumulate in fatty tissues, where they can stay for years, leading to chronic exposure (Devi, 2020). POPs are linked to a wide range of health problems, including cancer, reproductive disorders, immune system dysfunction, neurodevelopmental disorders, and endocrine disruption, as mentioned in Table 3. Exposure to POPs during pregnancy can also have adverse effects on fetal development, leading to congenital disabilities and developmental delays. One of the most well-known and widely studied POPs is polychlorinated biphenyls (PCBs) (Barker and Bryson, 2002; Grimm et al., 2020). These chemicals were previously utilized in various applications, such as electrical equipment, transformers, and hydraulic fluids, until their prohibition in the 1970s. Despite the ban, they persist in the environment, potentially accumulating in the food chain and posing risks to human exposure. PCBs have been associated with a spectrum of health issues, including cancer, immune system dysfunction, and developmental delays. Another category of Persistent Organic Pollutants (POPs) receiving recent attention is per- and polyfluoroalkyl substances (PFAs) (Wang et al., 2023). These compounds are used in various products, including non-stick cookware, water-resistant clothing, and firefighting foam. PFAs exhibit high persistence in the environment and can accumulate in the human body, leading to adverse health effects similar to PCBs, including cancer, immune system dysfunction, and developmental delays (Saibu et al., 2023). Dioxins and furans, also categorized as POPs, are byproducts of industrial processes like waste incineration and paper bleaching. Exposure to these chemicals can result in adverse health effects, such as cancer, reproductive disorders, and immune system dysfunction.
Additionally, children exposed to dioxins and furans may experience developmental delays and cognitive impairment. In response to growing concerns regarding the detrimental impacts of POPs, the international community has initiated measures to control and manage these chemicals. The Stockholm Convention on Persistent Organic Pollutants, enforced in 2004, aims to eliminate or restrict the production and usage of POPs. Currently, the convention identifies 28 chemicals as POPs, including PCBs, dioxins, and furans (Yanitch et al., 2020; Saibu et al., 2023; Zhou et al., 2023).
3 Exploring the rhizosphere: importance and significance
The rhizosphere soil region is nutrient-rich, where plant roots stimulate chemical and biological processes (Barra et al., 2021). The plant and many macro- and microorganisms interact uniquely in the rhizosphere, such as viruses, bacteria, fungi, protozoa, algae, nematodes, and microarthropods. These interactions contribute to the plant’s overall development (Ahkami et al., 2017; Abedinzadeh et al., 2019; Pathania et al., 2020; Adeyemi et al., 2021). Rhizospheric microorganisms that help plant growth can regulate plant development and yield. Rhizobacteria play a crucial role in the rhizosphere’s functioning and significantly impact plant physiology and growth (Barra et al., 2021). Three distinct zones make up the rhizosphere (McGrath et al., 2001), The rhizosphere, the small zone of soil surrounding plant roots, is a dynamic environment characterized by intricate interactions between plants and microorganisms. Understanding the relevance of each rhizospheric zone is critical for comprehending the functions of microbial activity and plant interaction (Barra et al., 2021). The rhizosphere is divided into three zones: the endorhizosphere, the rhizoplane, and the ectorhizosphere. Each zone has a specific role in microbial activity and plant contact.
3.1 Endorhizosphere
The endorhizosphere refers to the plant root’s interior tissues, including the cortex and vascular system. This zone is crucial for several reasons:
- Microbial Colonization: Beneficial microorganisms, such as endophytic bacteria and fungi, inhabit the endorhizosphere, providing improved nutrient uptake and growth promotion without harming the plant (Abedinzadeh et al., 2019).
- Nutrient Exchange: Close proximity to the plant’s vascular system allows microbes to efficiently exchange nutrients, such as nitrogen from endophytic nitrogen-fixing bacteria.
- Disease Resistance: Endophytic microorganisms help resist diseases by producing antimicrobial compounds or inducing systemic resistance (Adeyemi et al., 2021).
3.2 Rhizoplane
The rhizoplane is the root surface where plant roots interact with the soil environment. This zone is significant for:
- Microbial Adhesion: Microorganisms, including bacteria, fungi, and archaea, attach to the root surface, often facilitated by root exudates (McGrath et al., 2001).
- Nutrient Utilization: Rhizoplane microbes utilize root exudates, which contain nutrients like sugars, amino acids, and organic acids, for their development and activity (Adeyemi et al., 2021).
- Biofilm Formation: Microbes on the rhizoplane often form biofilms, enhancing microbial viability, nutrient exchange, and defense against environmental stressors.
3.3 Ectorhizosphere
The ectorhizosphere is the outer zone of soil surrounding the root, influenced by root exudates but excluding the root surface itself. It plays a crucial role in:
- Microbial Diversity: This zone supports a diverse microbial community, including free-living bacteria, fungi, protozoa, and nematodes, often with greater diversity than bulk soil (Zhou et al., 2023).
- Nutrient Cycling: Ectorhizosphere microbes contribute to nutrient cycling processes like nitrogen fixation, phosphorus solubilization, and organic matter decomposition, enhancing nutrient availability for plants (Pathania et al., 2020).
- Plant-Microbe Interactions: Beneficial microorganisms, such as mycorrhizal fungi and rhizobia, form symbiotic relationships with plant roots, promoting nutrient uptake and plant growth.
- Soil Structure: Microbial activity in the ectorhizosphere improves soil aggregation and structure, enhancing soil porosity and water retention.
Understanding each rhizospheric zone’s importance is crucial for optimizing agricultural practices and promoting sustainable farming. By managing rhizospheric microbial communities through strategies like crop rotation, cover cropping, and using biofertilizers and biopesticides, farmers can enhance plant health, improve nutrient uptake, and reduce reliance on chemical inputs. Additionally, insights into rhizospheric interactions can guide ecological restoration and natural ecosystem management, contributing to biodiversity conservation and soil health.
3.4 Plant growth-promoting rhizobacteria: key players in plant development and soil fertility
Rhizobacteria that promote plant development in addition to the plant’s natural defense mechanisms are referred to as plant growth-promoting rhizobacteria (PGPRs). Plant-PGPR interaction is crucial to plant development and soil fertility in the rhizosphere (Barnawal et al., 2017; Manoj et al., 2020; Zafar-ul-Hye et al., 2020; Shabaan et al., 2021). PGPRs are a class of rhizospheric bacteria that affect plant development and yield in economically important crops. Streptomyces, Serratia, Xanthomonas, Pseudomonas, Enterobacter, Klebsiella, Bacillus, Burkholderia, Azospirillum, Azotobacter, and Arthrobacter are a few of the genera found in PGPRs (Abbasi et al., 2013; Gururani et al., 2013). The following is an overview of the roles performed by PGPRs (Barnawal et al., 2017; Becze et al., 2021; Gulzar and Mazumder, 2022; Kuan et al., 2016; Sapre et al., 2021; Shabaan et al., 2021; Thokchom et al., 2017; Ullah and Bano, 2015):
1. The process of plant nutrient uptake
2. Plant development through the synthesis and production of amino acids and other compounds that stimulate plant development
3. Improved microorganisms required for plant development
4. Phytohormone production (auxins, gibberellins, and cytokinins)
5. Nutrient solubilization (Zn, PO4, Fe2+, and Fe3+)
6. Reduced metal toxicity
7. Stimulating metabolic processes in roots using bacteria's other process, i.e., biological nitrogen fixing
8. Enhanced plant disease resistance
Despite their small size, bacteria are the most prevalent organism in the rhizosphere. Pseudomonas, a Gram-negative bacterium genus, is a particularly efficient root colonizer with 108–1012 bacterial cells per gram of rhizosphere soil. In addition to their usefulness as bio-fertilizers, bioenergy generation, and bioremediation (Table 4), PGPRs are efficient plant root colonizers because of the abovementioned characteristics. The primary reason for the efficient colonization of plant roots by PGPRs is their motility and chemotaxis, which contribute to their positive effects (Belimov et al., 2020).
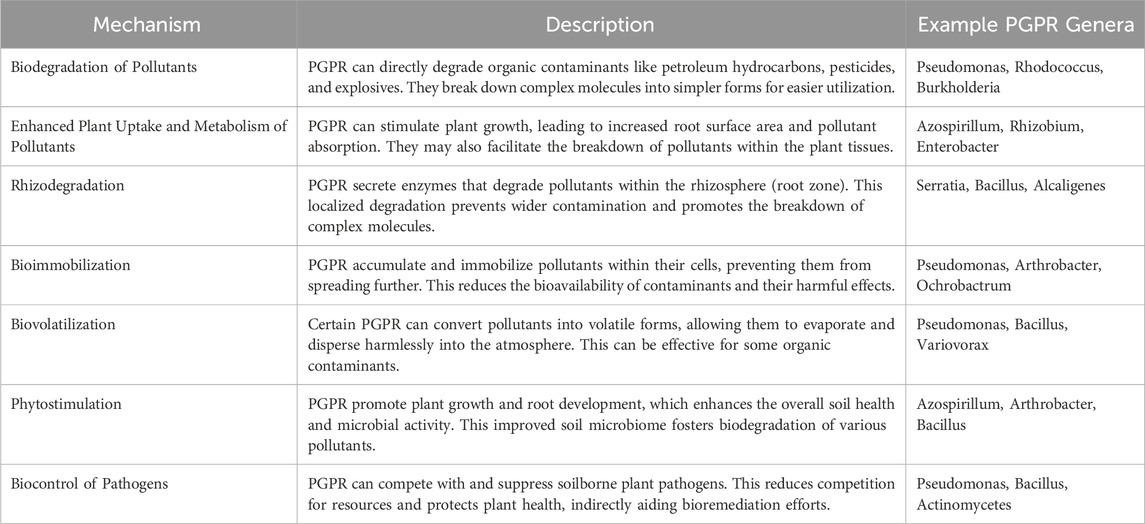
Table 4. Plant Growth-Promoting Rhizobacteria (PGPR) and their mechanisms in bioremediation (Abbasi et al., 2013; Gururani et al., 2013; Barnawal et al., 2017; Becze et al., 2021; Gulzar and Mazumder, 2022; Kuan et al., 2016; Sapre et al., 2021; Shabaan et al., 2021; Thokchom et al., 2017; Ullah and Bano, 2015).
Nitrogen (N) is a critical nutrient in agricultural production, playing a pivotal role in crop yield, particularly for grains such as rice, maize, potatoes, and wheat (Kuan et al., 2016; Rizvi and Khan, 2018). The application of nitrogen fertilizers significantly enhances the productivity of these crops. However, nitrogen utilization efficiency is often compromised due to ammonia volatilization, nitrogen leaching, and denitrification (Kumar and Saxena, 2019). Biological nitrogen fixation (BNF) presents a viable alternative to reduce chemical fertilizers applications. BNF responsible for the majority of Earth’s fixed nitrogen (∼60%). Given the increasing global food demand, optimizing BNF in agriculture is essential (Wickramasinghe et al., 2021). The nitrogen-fixing bacteria Kosakonia radicincitans was isolated from Pennisetum giganteum by Jia et al. (2020). They demonstrated that by combining this bacterium with chemical fertilisers, the total amount of fertiliser needed could be lowered by 25%. This integration significantly improved several plant characteristics, including alkali hydrolyzed nitrogen content, vitamin C and soluble protein amount, chlorophyll amount, soluble sugar amount, and available phosphorus amount. Similarly, Song et al. (2021) conducted a 2-year study assessing the replacement of urea with the cyanobacterium Anabaena azotica in rice cultivation. The investigation determined that using cyanobacteria in place of half of the urea had no negative effect on rice production.
Phosphorus (P) is another crucial macronutrient for plant growth and metabolic processes (Khan et al., 2009). Metal cations like calcium, iron, and aluminium quickly immobilise phosphorus in soils or bind it to mineral surfaces, reducing the amount of phosphorus that plants may access. Phosphates play vital roles in legume biological nitrogen fixation, crop maturation, flower and seed production, root and stem development, photosynthesis, and plant disease resistance (Nath et al., 2017; Wan et al., 2020). Therefore, phosphates are indispensable for agricultural productivity. In their 2020 study, Wan et al. tested eight different bacterial taxa for their ability to solubilize phosphorus. They found that Acinetobacter was the most effective, leading to improved soil fertility and quality. In addition, Liu et al. (2020) showed that specific bacteria release organic acids with low molecular weight, which dissolve inorganic phosphorus, alter soil characteristics, and indirectly affect the microbes in the rhizosphere. Iron, in its most common forms as Fe3+ and Fe2+, is a mineral that plants cannot function without (Belimov et al., 2020). Plants are unable to absorb iron from soils because the element is typically present in insoluble forms such hydroxides and oxyhydroxides, especially under aerobic circumstances. Iron is insoluble in water, but bacteria in the rhizosphere manufacture siderophores—small molecules with a strong affinity for iron—those plants can use (Da Silva et al., 2023). Rhizobium, Enterobacter, Pseudomonas, Azotobacter, Bacillus, and Azotobacter are among the plant growth-promoting rhizobacteria (PGPR) that create siderophores (Din et al., 2019). In situations where there is a shortage of iron, these molecules—which are present both within and outside of cells—help dissolve organic compounds and minerals containing iron. They can also form stable complexes with heavy metals and radioactive particles (Din et al., 2019). Soil heavy metal contamination can be reduced and plant growth can be enhanced by PGPR strains that produce siderophore. Phytohormones including gibberellin, cytokinin, and indole-3-acetic acid (IAA) are produced by plant growth-promoting bacteria (PGPB), which impact the hormone balance in plants (Chen et al., 2017). The amount of auxin available to the plant and its sensitivity to the hormone determine how IAA influences root growth. While low concentrations of bacterial auxin stimulate growth, high concentrations of auxin from PGPB, when added to the ideal levels of auxin found in nature, can stunt plant development (Sukul et al., 2021). By encouraging the development of adventitious and lateral roots and boosting the secretion of root exudates, bacterial IAA improves nitrogen absorption. Pseudomonas sp. isolated from soil near Vigna radiata (L.) produced growth-regulating compounds such IAA, 1-aminocyclopropane-1-carboxylate (ACC) deaminase, and siderophores, according to Al-Enazi et al. (2022) investigation of pesticide-resistant plant growth-promoting rhizobacteria strains. Even when exposed to increasingly high quantities of metalaxyl, carbendazim, and tebuconazole, the PGR-11 strain persisted in producing PGP compounds. High pesticide concentrations have a negative effect on plant development and physiological and biochemical features, according to tests conducted on V. radiata (L.).
3.5 Relationship between heavy metals and the microbiome
Bioremediation technology currently focuses on the interactions between metals and microbes, which can be explained differently. The presence of soil microorganisms in different soil regions is crucial in deciding the fate of heavy metals in the soil (Becze et al., 2021; Gulzar and Mazumder, 2022). These microorganisms are not evenly spread throughout the soil, and heavy metals can severely impact their cellular, biochemical, and molecular processes, putting their survival at risk. Although heavy metals generally have an inhibitory effect at high concentrations, some heavy metals, such as Cadmium, can harm soil microbiota even at low concentrations (Sengupta et al., 2021). Heavy metals are toxic because they inhibit cell growth and development by directly destroying or deactivating critical cellular components, among other things. For example, HMs can cause oxidative stress by ROS production (Husain et al., 2022), disrupting the structure (Camargo et al., 2018) and function of several active biomolecules like DNA, RNA, and proteins (Gulzar and Mazumder, 2022). For instance, metals like cadmium, mercury, and lead disrupt the ionic balance, harm cell membranes, and even denature proteins (Sobolev and Begonia, 2008; Abdu et al., 2017). In addition to causing ionic imbalance and enzyme inhibition in bacterial systems, copper, nickel, and zinc toxicity has been documented (Ding et al., 2022). Metal ions can block the activity of many enzymes, including superoxide dismutase, catalase, and ascorbate peroxidase (Mitra et al., 2022). In a similar vein, arsenic damages DNA, while mercury impedes the transcription process (Bobaker et al., 2019).
It has been suggested that microorganisms residing in metal-contaminated soil can transform toxic metals into less harmful molecules. The microbiome can dissolve or solubilize heavy metals, and transition metals can undergo oxidation or reduction without impacting the microbiome’s ability to promote plant growth (Hlihor et al., 2022). In addition, the soil microbiome replenishes the environment by binding, volatilizing, oxidizing, immobilizing, and converting harmful metals into less harmful forms. Consequently, the soil microbiome can remove, restore, precipitate, and detoxify metals by modifying their environmental characteristics and solubility (Barra et al., 2021). These ecological interactions between the microbiota and toxic metals are essential for regulating mobility and decontaminating polluted environments. Nevertheless, the effectiveness of microbial activities depends on various factors, including metal concentration and species, the composition and function of microbiomes, and the environmental state (Kim et al., 2020; Liu et al., 2019).
4 Remediation approach used for HMs and POPs
Understanding chemical characteristics and eliminating heavy metals and pollutants (POPs) from the soil requires efficient solutions. Several strategies have been tried to reduce the harmful effects of POPs, the most prevalent of which involves concentrating on HMs and PAHs found in soil and water, respectively (Tufail et al., 2022). Depending on whether the remediation is conducted on-site (in-situ) or away from the contaminated area (ex-situ), soil and water remediation techniques can be categorized as chemical (using chemicals), physical (using physical agents), or biological (using living organisms) (Cepoi et al., 2022; Kumar et al., 2022; Li et al., 2020). To alleviate POPs, modern techniques have been developed that incorporate the compounds’ molecular size, water solubility, polarity, and volatility, increasing extraction efficiency (Sun et al., 2017). Table 5 presents a compilation of methods utilized for HMs and POPs remediation from the environment, showcasing a summary of different approaches.
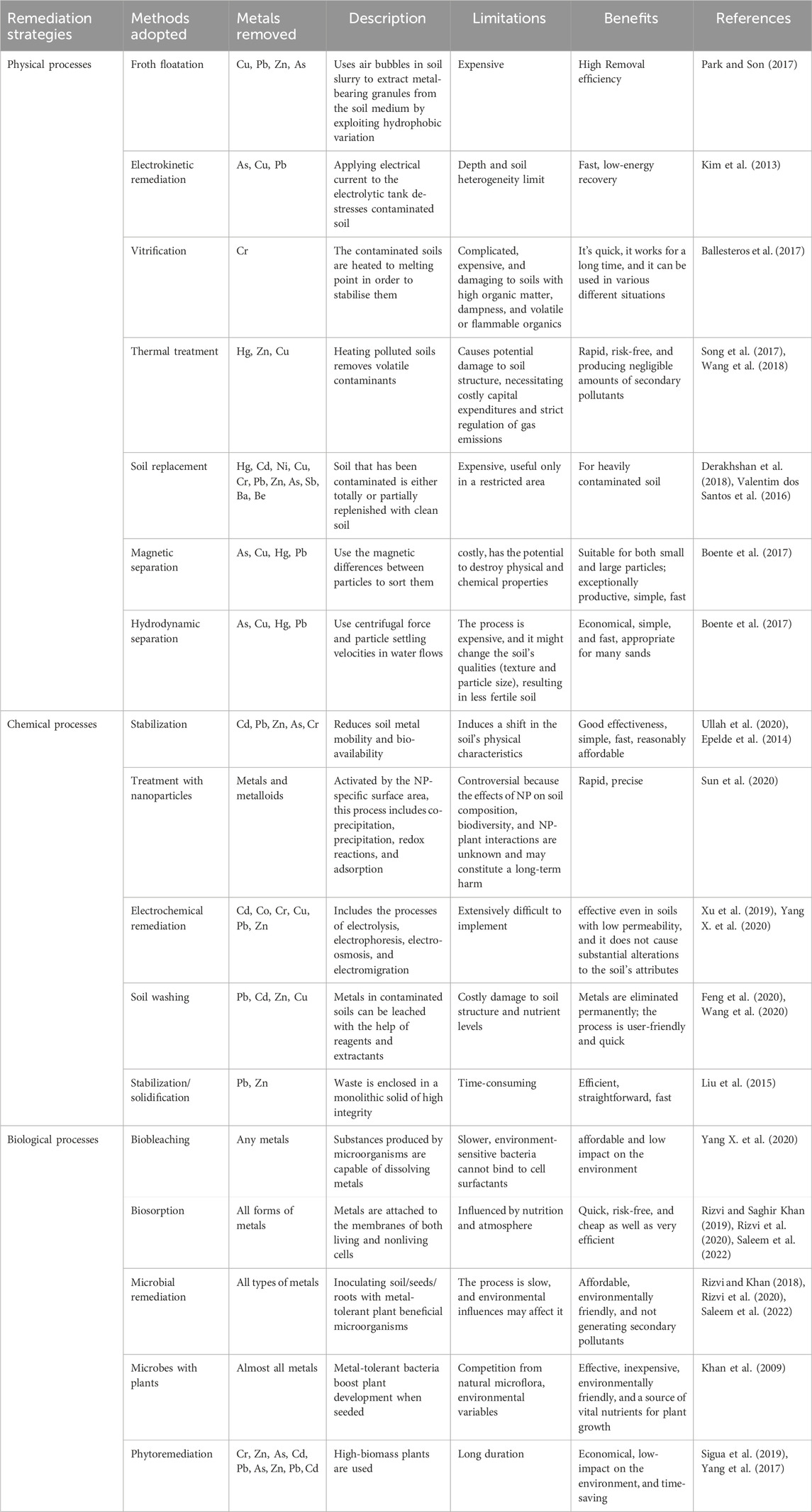
Table 5. Techniques for the physical, chemical, and biological remediation of metals at both laboratory and field scales.
4.1 Physicochemical approaches utilized for remediation
Ultrafiltration, solvent extraction, osmosis, nanofiltration, incineration, flotation, ion exchange, electrodialysis, fixation (i.e., nitrogen), conventional and advanced oxidation, coagulation, and precipitation are some of the physicochemical processes that have been used to get rid of HMs and POPs (Kadam et al., 2019). As a physicochemical technique, adsorption has great promise for eliminating HMs and POPs from the environment. Adsorption is widely used in industry because of its remarkable potential efficiency, low energy requirements, molecular level preference, malleability, and capacity to separate various chemical substances (Priyadarshanee and Das, 2021).
Another standard method of water purification is electro-coagulation (Bandaru et al., 2020; Titchou et al., 2021). Research studies (Bandaru et al., 2020) have shown the application of iron electro-coagulation to remove As (III) from the environment. After being subjected to microfiltration, the concentration of additional HMs (Cr, Pb, and Ni) in oily water dropped to 10 mg/L from 35 mg/L (Changmai et al., 2019). Commercial electro-coagulation systems maintain a steady current of 5–20 mA/cm2 for optimal effluent removal. Advanced oxidation processes (AOPs) and conventional oxidation processes (COPs) are two types of chemical treatments (Silvianti et al., 2020). Photocatalysis processes, solar-Fenton, electron-Fenton, and Ferrate ion, all AOPs, have greatly facilitated the remediation process of HMs (Mazumder et al., 2020). Nevertheless, ozonization, hydrogen peroxide (H2O2), chlorination, and photolysis are used in COPs to clean water, and their application will produce byproducts and radicals. Fruit waste, such as banana peel, egg shells, coconut husks, and nutshells, as well as tannin-rich materials like rice husk and fertilizer wastes, are all examples of bioadsorbents to treat water and soil (O’Connell et al., 2008; Zhao et al., 2018). Furthermore, several soil-derived adsorbents, such as fly ash and red mud (Dash et al., 2018), zeolites and clays (Titchou et al., 2021), new carbon nanomaterials (NMs), and metal oxides, showed improved removal effectiveness when tested on a variety of contaminants and HMs (Chakraborty et al., 2022).
4.2 Biological approaches utilized for remediation
Microbial bioreactors (MBRs) have been shown to effectively decontaminate wastewater using a combinatorial approach of microbial biodegradation and physical retention at the membrane surface (Tufail et al., 2022; Zhang et al., 2020). However, Membrane fouling makes it challenging to eliminate specific POPs. As a result, a hybrid technique involving an electrochemical MBR has been developed to remove nearly all toxins at a fixed electric potential from wastewater (Hube et al., 2020). Compared to controlled MBR, this approach is three times more reliable and may be used for three times as long. The build-up of pesticides, PAHs, and HMs in natural resources is a topic of discussion on a global scale. Pollutants remained in the soil for a long and leaked into the ground and surface water. In order to produce sustainable habitats, it is crucial to break down harmful chemicals in the soil (Russo et al., 2019; Rigoletto et al., 2020; Saha et al., 2021). Bioremediation is superior to the conventional physicochemical approach of removing pollutants from soil and water. Microbe-mediated biodegradation of HMs and organic pollutants is emerging as a modest but feasible answer to all these problems, often known as bioremediation (Tyagi and Kumar, 2020; Sreedevi et al., 2022; Tufail et al., 2022; Liaqat et al., 2023; Masotti et al., 2023; Saibu et al., 2023). Compared to chemical and physical approaches, this technology is more cost-effective, less invasive, environmentally benign, and long-lasting.
4.2.1 Bacterial-mediated bioremediation
Bacteria might adapt or be resistant to metal toxicity using various approaches, i.e., synthesis of metallothioneins, active transport or efflux mechanisms, morphological changes, synthesis of siderophores, biotransformation of toxic metals, and synthesis of exopolymeric compounds (Funtikova et al., 2023; Liaqat et al., 2023; Sevak et al., 2023; Wang et al., 2023; Zhou et al., 2023). Figure 1 presents the few microbial approaches used for HM remediation from the soil.
Bacteria are well-known for their ability to degrade or mineralize pollutants through enzyme-catalyzed catabolic action (Tables 6, 7). Numerous catabolic genes that rely on PAHs and are remarkably conserved have been identified during bioremediation studies (Barker and Bryson, 2002; Ali et al., 2022). These genes are present in gram-positive bacterial species and include phd, pdo, nid, and nar, and in gram-negative bacterial species and include phn, pah, ndo, nah, and nag (Sakshi and Haritash, 2020). Analysis showed that crude oil might be biodegraded by the bacteria Pseudoalteromonas agarivorans, Isoptericola chiayiensis, Rhodococcus soli, and Bacillus algicola in less than 2 weeks (Lee et al., 2018). Most small molecules with carbon atoms between C9 and C14 were entirely decomposed, while those with C15 and C20 were almost completely broken down. The larger-sized molecules, on the other hand, were substantially broken down. Another study reported that after 30 days of remediation, bacterial consortiums obtained from China’s Yangtze River Delta broke down 44.5% of total petroleum hydrocarbon (TPH) (Jia et al., 2018). Chlorpyrifos (CPF) breakdown was studied in two different bacterial species Bacteroides megaterium CM-Z19 and Pseudomonas syringae CM-Z6. Five days of incubation at 37°C resulted in a 92.6% and 99.1% degradation of chlorpyrifos-methyl-Z19 and chlorpyrifos-methyl-Z6, respectively, at an initial 100 mg/L concentration (Zhu et al., 2019). Nevertheless, 200 μg per liter of CPF can be degraded by Cupriavidus nantongensis X1T in about 48 h. C. nantongensis X1T species may survive in temperatures between 30°C and 42°C, and pH ranges between 5 and 9. It has a CPF tolerance of 500 mg/L (Shi et al., 2019). Cupriavidus sp. DT-1 has been shown to successfully degrade CPF in liquid media, mineralizing both CPF entirely after 14 h at pH 7°C and 30°C. In the same settings, 90% of chlorpyrifos in soil media degrades after 30 days (Lu et al., 2013). The most efficient microorganisms, P. putida MAS, can break down 90% of CPF in 24 h (Kamika and Momba, 2013). Recent studies indicate that aerobic bacteria, such as Sphingobium sp. strain BHC-A and Schistosoma japonicum UT26, can fully degrade lindane in an aerobic environment (Perera and Hemamali, 2022). Atrazine-contaminated soil from the Vetiver rhizosphere reported two bacterial species: Paenarthrobacter aurescens TC1 and Arthrobacter MCM B-436. Atrazine degradation in P. aurescens TC1 is controlled by trzN, atzC, and atzB genes. Arthrobacter sp CW-1 breaks down dimethyl phthalate (DMP) in anaerobic environments (Jia et al., 2021). Nicotine degradation in Arthrobacter nicotinovorans is mediated by the plasmid pAO1 (Guo et al., 2019). Rhodococcus pyridinivorans SS2 and Rhodococcus ruber SS1 can effectively remediate triCB and dichlorobiphenyl (diCB) (Xiang et al., 2020). Pseudomonas sp. breaks down - Hexabromocyclododecanes (HBCD) at concentrations as low as 50 mg/L in about 5 days, but at 640 mg/L, it takes 8 days to break down (Huang L. et al., 2021; Huang et al., 2022). Bacillus sp. can break down HBCDs at 320 mg/L in about 4 days (Huang et al., 2022). Pseudomonas aeruginosa HS9 can degrade 69% of 1.7 mg/L HBCDs in 14 days. Table 6 provides a detailed overview of diverse bioremediation strategies utilized by microorganisms to effectively eliminate Heavy Metals (HMs) and Persistent Organic Pollutants (POPs) from contaminated environments.
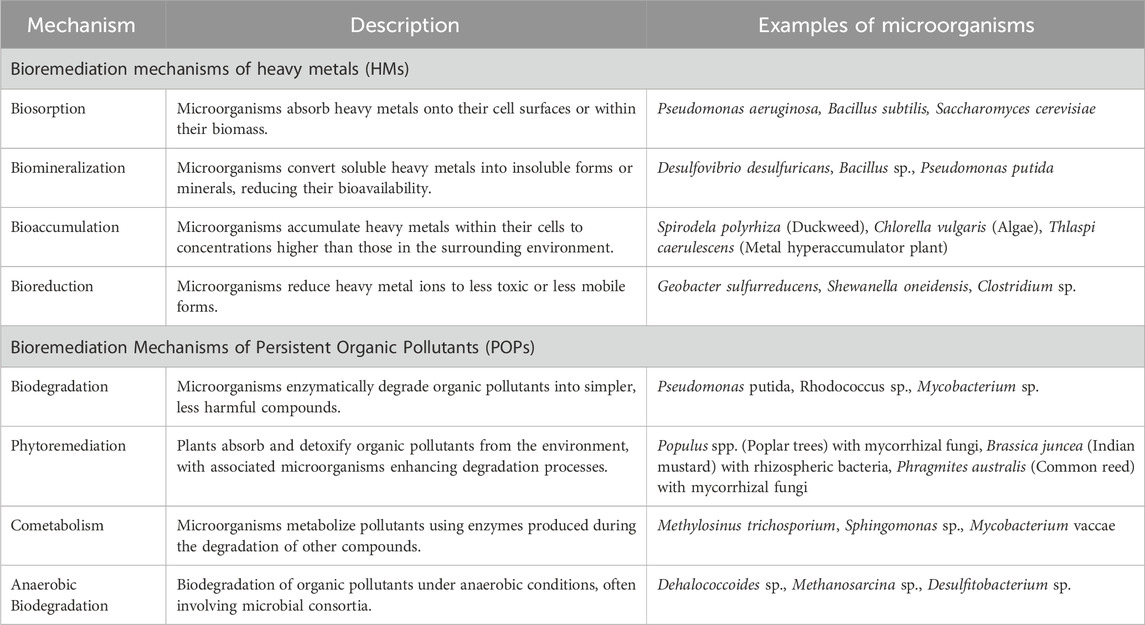
Table 6. A comprehensive breakdown of various bioremediation mechanisms employed by microorganisms for the removal of heavy metals (HMs) and persistent organic pollutants (POPs) (Guo et al., 2019; Huang et al., 2021; Jia et al., 2021; Huang et al., 2022).
Halophilic bacteria possess extremozymes that can operate effectively in severe conditions, which makes them a promising choice for bioremediation applications. These halophilic bacteria produce an extremozyme with unusual properties, including resistance to heat, acidity, organic solvents, and strong ions. Microprecipitation or proton exchange aids in attaching these bacteria to HMs via an extracellular polymeric material (Kaushik et al., 2021). The negative charge on cell surfaces can be attributed to various functional groups, including sulfate, carboxyl, phosphoryl, and amino functional groups. These functional groups possess negatively charged atoms or groups of atoms, such as oxygen or sulfur, which contribute to the overall negative charge of the cell surface (Dawwam et al., 2023; Syed et al., 2022). The negative charge of these functional groups plays an essential role in the interaction of biomass with metal ions. These groups serve as ion exchange sites and can bind metal ions through a process known as cation exchange. During cation exchange, metal ions, such as hydrogen ions, are exchanged for positively charged ions on the biomass surface (Kaushik et al., 2021). Microorganisms employ various mechanisms to eliminate heavy metals from contaminated soils. These include precipitation, biosorption by sequestering them in intracellular metal-binding proteins (metallothioneins), and converting them into harmless forms through an enzymatic transformation, as presented in Figure 2.
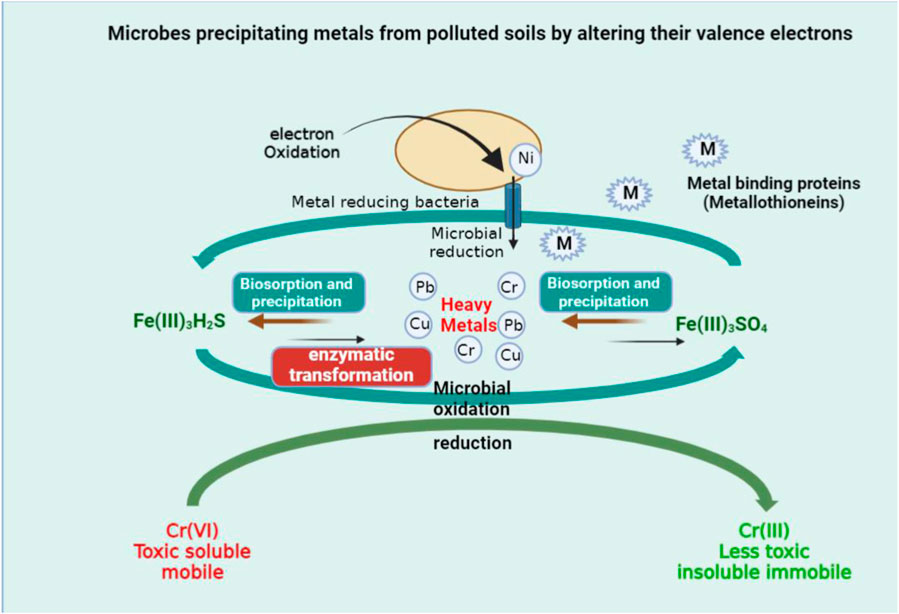
Figure 2. Precipitation, biosorption via sequestration by intracellular metal-binding proteins (metallothioneins), and enzyme-catalyzed metal conversion to nontoxic forms are all mechanisms used by microorganisms to remove heavy metals from contaminated soils (enzymatic transformation) (Ojuederie and Babalola, 2017).
In addition, the anionic functional groups in the cell walls of gram-positive and gram-negative bacteria have an essential function in binding metals. The negatively charged groups allow the bacteria to bind metal ions on the surface or within the cell wall. Binding metals is vital for bacteria’s survival as metal ions are necessary for many cellular processes, such as enzyme activity and energy metabolism (Pachaiappan et al., 2021). It has been reported that Methanothermobacter thermautotrophicus can convert chromium (VI) to chromium (III) and then immobilize chromium (III) as hydroxide or oxide. Bacillus cereus and Shewanella have been shown to decrease Cr (VI) and its immobilization (Chen et al., 2012) as shown in Figure 2. Table 7 enumerates diverse bacterial species employed in soil for the remediation of heavy metals (HMs), showcasing their effectiveness in mitigating environmental contamination.
4.2.2 Fungi-mediated bioremediation
Fungi are a diverse group of eukaryotic organisms that obtain their nutrients from organic matter in their environment, and they are often referred to as saprophytic organisms. Fungi have been present on Earth for millions of years, and they play essential roles in various ecological processes such as decomposition, nutrient cycling, and symbiosis. They are exceptionally efficient in decomposing PAHs and HMs due to their specificity for excessive refractory chemicals and survival potential in harsh natural habitats, i.e., elevated temperatures and reduced pH (Arwidsson et al., 2010; Bano et al., 2018; Chen et al., 2022; de Moura Dickel et al., 2022). Moreover, the fungus may digest PAHs and HMs “in situ” by producing extracellular enzymes (Liu et al., 2017), which can be done due to the extensively branched mycelia. Fungi have two main approaches for metal detoxification: biosorption (Bano et al., 2018), which includes adhering metals to the membrane, and bioaccumulation, which includes absorbing metals into the cell and metabolizing them (Soleimani et al., 2010). It has been shown that certain fungi, including Gloeophyllum sepiarium, Penicillium chrysogenum, Aspergillus versicolor, Aspergillus terreus, Aspergillus niger, Aspergillus fumigatus, and Rhizopus oryzae can breakdown PAHs and HMs (Hota et al., 2021). Recently, Chen et al. (2022) evaluated the potential of white rot fungus for the remediation of heavy metal contamination. However, heavy metal concentrations, organic pollutants, and unfavorable environmental conditions can slow this remediation process. Table 8 presents a comprehensive overview of various fungi, algae, and plants utilized for remediating heavy metals (HMs) in soil, emphasizing their mechanisms and target HMs. These organisms play crucial roles in bioremediation by absorbing, accumulating, or transforming HMs through mechanisms such as biosorption, bioaccumulation, and biotransformation. Understanding these biological agents and their specific interactions with HMs is essential for developing effective strategies for soil remediation and environmental protection.
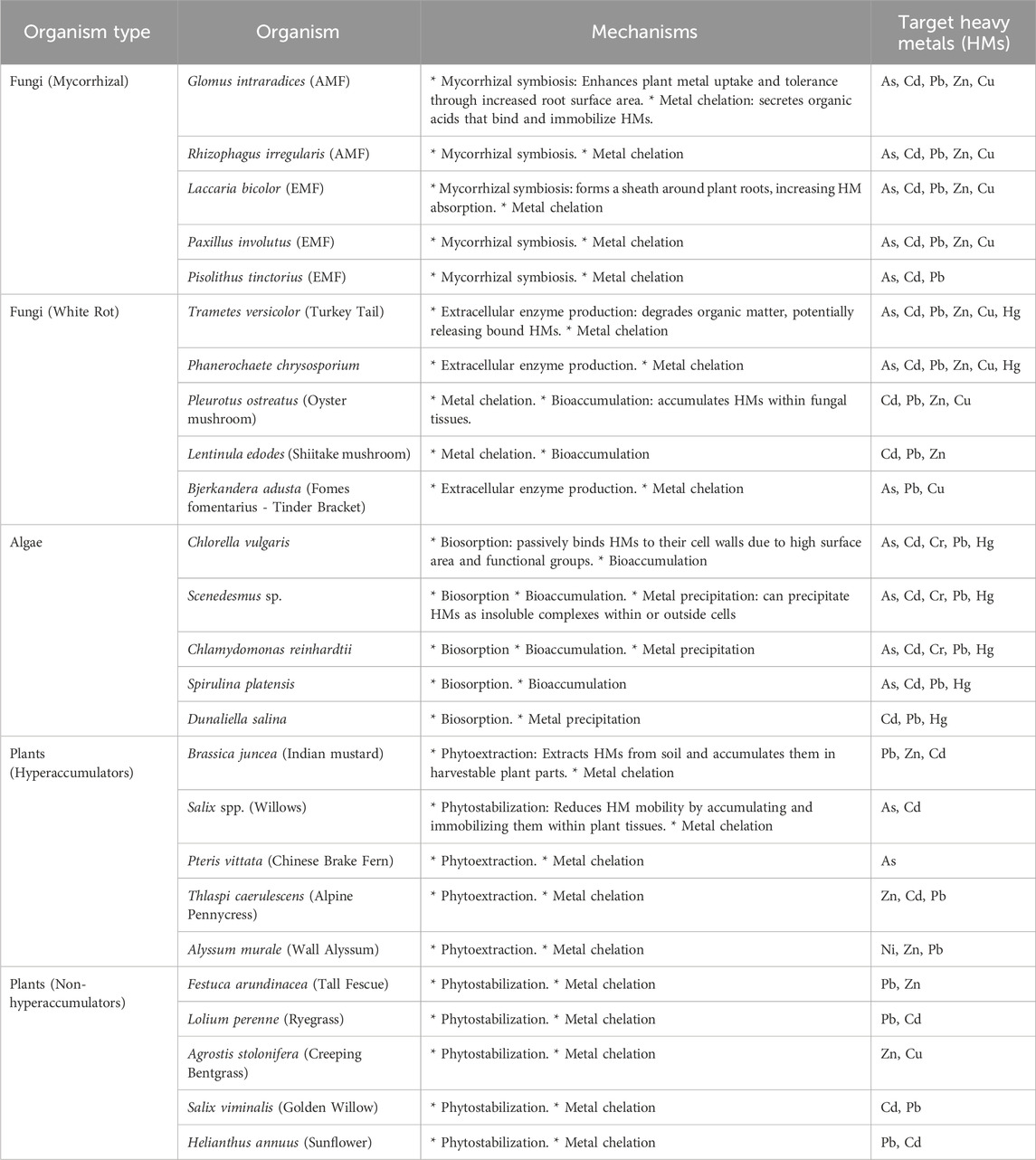
Table 8. A comprehensive details of various fungi, algae, and plants used for remediating heavy metals (HMs) in soil, highlighting their mechanisms and target HMs (Soleimani et al., 2010; Arwidsson et al., 2010; Bano et al., 2018; Hota et al., 2021; Chen et al., 2022; de Moura Dickel et al., 2022).
The remediation outcomes appear to be highly sensitive to the types of strains, the types of pollutants, and the reaction conditions. Concentrations of heavy metals or organic pollutants that are too high or too low, or reaction conditions that are too slow or too fast, might impede the cleaning process (Kumar et al., 2023; Palanivel et al., 2023; Tessaro et al., 2023). Zhuo and Fan (2021) have reported an in-depth analysis to evaluate the most current developments in using white rot fungus to degrade organic pollutants. In addition, they deduced that most current bioremediation investigations of white rot fungus are undertaken in controlled laboratory settings. Further research must consider the challenges associated with treating pollution in practice. The white-rot fungus most likely uses laccases, lipases, lignin peroxidase (LiP), manganese peroxidase (MnP), and cytochrome P450 versatile peroxidase to breakdown and decrease PAHs and HMs (El-Khoury et al., 2022; Sanchez-Hernandez et al., 2023; Syed et al., 2014). Lasiodiplodia theobromae, isolated from PAH-polluted soil in Beijing, China, removed 53% of benzo[a]pyrene (BaP) within 10 days of incubation (Punetha et al., 2022). Increasing the incubation period to 2 weeks enhanced the biodegradation potential of Peniophora incarnate strain KUC8836, resulting in the removal of 97.9% of pyrene, 95.3% of phenanthrene, and 95% of fluoranthene, attributed to elevated laccase, LiP, and MnP production (Lee et al., 2014). Within 30 days, Rhizoctonia zeae SOL3, Scopulariopsis brevicaulis, and Pleurotus pulmonarius FO43 achieved near-complete decomposition of pyrene at concentrations of 42%, 64%, and 99%, respectively (Bhattacharya et al., 2014; Mao and Guan, 2016). Aspergillus terreus, Trichoderma viride, Trichoderma longibrachiatum, and Aspergillus niger were observed to absorb Pb, Cd, Cr, and Ni at rates of 59.67 mg/g, 16.25 mg/g, 0.55 mg/g, and 0.55 mg/g, respectively (Dell’Anno et al., 2022; Kumar and Dwivedi, 2021). In another study, the highest remediation potentials for Cr (III), Pb (II), Cr (VI), and Cu (II) were found to be 226.6 mg/g, 208.5 mg/g, 207.3 mg/g, and 205.1 mg/g, respectively, when the fungus was immobilized in living form (Hanif et al., 2015). The fungus Aspergillus fumigatus (FS6) and Aspergillus flavus (FS4) eliminated almost 70% of the Cr (VI) from the liquid PDB medium. Cd (II) removal by Aspergillus fumigatus (FS9) was as high as 74% (Talukdar et al., 2020). Sterigmatomyces halophilus, A. restrictus, A. penicillioides, A. gracilis, and A. flavus and all of which are obligate halophilic fungi, showed efficient biosorption for cadmium, copper, ferrous, manganese, zinc, and lead (de Moura Dickel et al., 2022; Hota et al., 2021; Kumar and Singh, 2023; Tessaro et al., 2023). S. halophilus and A. flavus demonstrated the highest adsorption levels, averaging 83%–86%. The fungi Mucor alternans, Phanerochaete chrysosporium, Trichoderma viride, Rhizopus arrhizus FBL 578, Fusarium oxysporum, and Trichoderma hamatum FBL 587 are long-established as DDT degraders (Russo et al., 2019). When endosulfan is exposed to Trichoderma harzianum, it is oxidized to endosulfan sulfate and then degraded naturally (Landa-faz et al., 2021). In PAH-contaminated industrial soil, the fungus Irpex lacteus and Pleurotus ostreatus can break down the PAHs. In 5 days at 26.8°C and pH 6.5, Cladosporium cladosporioides degrades 50 mg/L CPF (Bhattacharya et al., 2014).
4.2.3 Microalgal-mediated bioremediation
Algae offers various advantages as a decontaminating agent, including low cost, easy handling, non-pollution, quick metal contamination removal for recovery, and no additional wastage. Microalgae exhibit the capability of bio-remediating environmental-contaminants (ECs) through three distinct approaches: bio-uptake, bio-adsorption, and bio-degradation (Table 8) (Goswami et al., 2022; Kashem et al., 2023; Tambat et al., 2023). Bio-adsorption is the process by which contaminants are adsorbed onto the surface of the microalgae cells without any cellular uptake or degradation. This process depends on the physicochemical properties of both the contaminant and the microalgae cells, and it can be affected by factors such as pH, temperature, salinity, and ionic strength (Goswami et al., 2022). Bio-adsorption can be an effective method for removing low concentrations of contaminants from the environment, but it is not a long-term solution, as the contaminants can be released back into the environment over time (Dubey et al., 2023). Bio-uptake is the process by which contaminants are taken into the microalgae cells and accumulated within the cells (Dubey et al., 2023). This process can occur through passive diffusion or active transport, depending on the contaminant’s physicochemical properties and the cellular membrane. Bio-uptake can be an effective method for removing moderate to high concentrations of contaminants from the environment, as the contaminants are sequestered within the cells and are not released back into the environment. Bio-degradation is the process of metabolizing contaminants and breaking them down by the microalgae cells into less toxic or non-toxic compounds (Cameron et al., 2018; Goswami et al., 2022). This process depends on the microalgae cells’ metabolic pathways and the contaminants’ nature. Bio-degradation can effectively remove complex or persistent contaminants from the environment, but it requires specific conditions and nutrients to support the growth and metabolism of the microalgae cells. Bio-adsorption occurs when environmental contaminants (ECs) link to organic substances released by cells or components of the cell wall (Das et al., 2022; Satya et al., 2023). Alternatively, bio-uptake occurs when pollutants bind to intracellular proteins and other substances and involve the subsequent intracellular transit through active transport, assisted diffusion, or simple diffusion. Microalgae use a catalytic metabolic process to break down the chemicals into their parts to biodegrade ECs. Bio-degradation is an essential approach for cleaning up hazardous toxins, which works more like a bioreactor than a biofilter by breaking down the contamination into less dangerous chemicals (Sher and Rehman, 2019; Leon-Vaz et al., 2021; Chebotaryova et al., 2023). It could take place inside cells, outside cells, or in a hybrid form. Spirulina, Scenedesmus, Phormidium, Oscillatoria, Nodularia, Desmodesmus, Cyanothece, Chlorella, Botryococcus, and Arthrospira are a few of the microalgal genera used in bioremediation (Dwivedi, 2012; Dubey et al., 2023). Chlorella vulgaris is effective at degrading acenaphthene and fluoranthene, according to research by Touliabah et al. (2022). The microbes Lyngbya digueti, Phormidium mucicola, Oscillatoria princeps, Anabaena variabilis, and Westiellopsis prolific were helpful in the reduction of quantity of various petroleum hydrocarbons in oil refinery effluent, which ranged from 24% to 92% reduction (Takáčová et al., 2014). It was reported that Chlorella kessleri could degrade 3,4-benzpyrene (29%) when exposed to light at a strength of 13.5 W per square meter. Similarly, Chlamydomonas reinhardtii was reported to decompose benz(a)anthracene at a rate of 10 mg/L in 11 days (Luo et al., 2020; Luo et al., 2020). The breakdown of homogentisate resulted in a rise of gene expression that encodes for ubiquinol oxidase, carboxy-methylene-butenolide, carboxylase/oxygenase (Rubisco), ribulose 1,5-bisphosphate, and homogentisate 1,2-dioxygenase (HGD) enzymes. Chlorella vulgaris biomass is an effective biosorbent for the removal of copper (Cu2+), Cadmium (Cd2+), and lead (Pb2+) from a mixed solution containing 50 mg dm3 of each metal ion (Goher et al., 2016). After being treated with Spirulina sp, Ca2+ was reduced by 98% and Cu2+ by 91% in municipal wastewater. Another study (Yang et al., 2015) found that Chlorella minutissima could remove 84% of Cu2+, 84% of Mn2+, 74% of Cd2+, and 62% of Zn2+ from municipal garbage. Microalgal biochar has been shown to remove Cr (VI) from water with 100 percent efficiency by Daneshvar et al. (2019), while Cheng et al. (2017) have studied the biosorption and kinetics of Cd (II) removal using both live and dead C. vulgaris. The research findings indicate that both viable and decaying cells of C. vulgaris exhibit a notable ability for adsorbing Cd, demonstrating efficiencies of 95.2% and 96.8% respectively.
4.2.4 Plant-mediated bioremediation
Plants are used in phytoremediation, a form of bioremediation, to clean up polluted environments such as soil, water, and air. Methods include phytotransformation, phytostabilization, rhizofiltration, phytostimulation, rhizodegradation, phytodegradation, phytovolatilization, and phytoextraction are all part of the broader field of phytoremediation (Nedjimi, 2021; Shabaan et al., 2021; Oladoye et al., 2022) as shown in Figure 3 and Table 8.
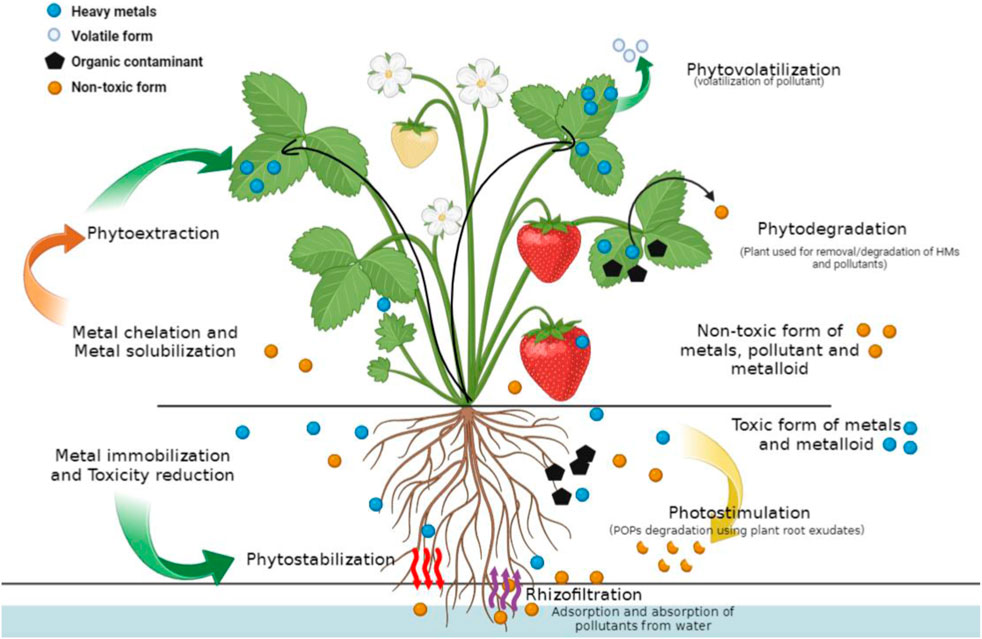
Figure 3. A diagrammatic depiction of various plant-based methods being investigated for phytoremediation purposes.
Many plant species can absorb, bioaccumulate, immobilize, and degrade environmental pollutants. Some plants that can be utilized for HMs and POPs phytoremediation of soil include Cucurbita pepo, Zea mays, Nicotiana tabacum, Medicago sativa, Alyssum murale, Achillea millefolium, Aeolanthus biformifolius, Arabis gemmifera, Phytolacca americana, and Pteris vittata (Kurniawan et al., 2022; Li et al., 2023; Rahman and Singh, 2020; Tauqeer et al., 2016). In order to effectively remove pollutants from water, certain plant species are utilized, i.e., Eichhornia crassipes, Ipomoea aquatica, Phragmites australis, Potamogeton natans, Ruppia maritima, Vallisneria americana, Hygrophila corymbosa, Nuphar lutea, Salvinia minima, Pistia stratiotes, and Lemna minor (Wei et al., 2021). The impact of certain bacterial species, which are associated with the growth of plants underground, can enhance plant development, promote metal translocation within the plant, alter the bioavailability of metals in the soil, and reduce metal phytotoxicity. This leads to an increase in the effectiveness of phytoremediation. Evidence from a few research shows that bacterial inoculations considerably alter the expression pattern of various metal transporters, including the ZIP, NRAMP, HMA, F-box, and AtALS3 gene families, employing these shared and unique growth-promoting functions (Dash and Osborne, 2023; Dash et al., 2018; Manobala et al., 2021; Dash and Osborne, 2023).
In Arabidopsis tissues, Bacillus amyloliquefaciens alters the transcriptional activity of the IRT1, FRO2, and FIT1 genes, increasing Fe and Cd accumulation (Sukweenadhi et al., 2015). It has been shown that the endophytic Pseudomonas fluorescens Sasm05 strain significantly increases Cd accumulation and tissue growth after inoculation, a process that mimics the overexpression of the SaHMAs, SaNRAMPs, and SaZIPs gene families (Chen et al., 2017). Much research has been conducted on the ZIP transporter gene family, which regulates zinc transportation through membranes and cytoplasmic concentrations in plant cells. Enterobacter cloacae–Zn solubilizing bacterial inoculation in rice plants had altered OsZIP1, OsZIP4, and OsZIP5 gene expression, resulting in enhanced Zn accumulation in plant tissues (Krithika and Balachandar, 2016). In Arabidopsis thaliana (Sukweenadhi et al., 2015), inoculations with Plant Growth-Promoting Rhizobacteria (PGRP) under Aluminum (Al) stress offer a promising strategy to alleviate heavy metal toxicity and enhance plant development. This is achieved by modulating the expression of key genes, including AtAIP, AtALMt1, and AtALS3. Notably, the activation of AtALS3 gene in response to Al stress results in synthesizing an ABC transporter-like protein within phloem cell membranes. This protein aids in effectively relocating Aluminum away from vulnerable areas, thus protecting the plant. While the specific role of the AtALP gene in Aluminum tolerance remains uncertain, it likely contributes to the overall adaptive response. Additionally, the collaboration between the HMA gene family and AtALS3 plays a vital role in facilitating the translocation of Heavy Metals (HMs) from the plant’s roots to the shoots, primarily accomplished through the xylem. This mechanism assists in regulating the distribution of HMs within the plant, ultimately supporting its resilience to metal-induced stress.
Unfortunately, plant cells do not include any natural transporters specific to organic environmental pollutants. Hence, they move around without actively doing anything. Root microbiome components such as rhizosphere bacteria and endophytes have long been appreciated for their role in the phytoremediation of PAHs. Diesel-contaminated soil remediation using petroleum hydrocarbons was less harmful due to the presence of endophytic microbial species such as Stenotrophomonas spp., Pseudomonas spp., Pantoea spp., and Flavobacterium spp (Agarwal et al., 2019; Pinel-Cabello et al., 2023). To remove organic pollutants from the environment, rhizobial symbiotic consortiums use organic molecules as a C and N source in phytoremediation. Rhizobium strains that nodulate the hyperaccumulator plant Leucaena have been shown to aid in rhizoremediation by using plant toxins (such as the aromatic chemical Mimosine) as C and N sources (Sytar et al., 2021). The bacterial species help plants detoxify HMs and PAHs by increasing their metabolic growth rate. Plant growth and metabolic gene regulation by PGPR inoculations facilitate the systematic development of plant physiology (specifically, “biomass, bushiness, lateral root production, lateral root number, surface area, and thickness”). Inoculating rice seedlings with Bacillus altitudinis, for instance, improves root architecture by regulating auxin metabolism and modulating the expression of OsIAA1, OsIAA4, OsIAA11, and OsIAA13 (Ambreetha et al., 2018). The antioxidant defenses of the host plant are also strengthened by PGPR inoculations, making them more effective in combating stress.
The improvement of Solanum tuberosum Zn tolerance by Bacillus isolates is achieved by adjusting the expression of SOD, GR, DHAR, CAT, and APX genes (Gururani et al., 2013). Moreover, the growth-promoting qualities of ACC-deaminase are critical in aiding hosts to resist the toxicity of petroleum hydrocarbons, which is just one of the many ways endophytic bacteria can help. The use of arbuscular mycorrhizal fungus (AMF) and endophytic fungi to bio-enhance plant growth is a current focus in phytoremediation (Kumar and Saxena, 2019; Ordookhani et al., 2010). The underground network of mycelium belonging to AMF aids in phytoremediation by expanding the rhizosphere, enabling plants to access contaminants and nutrients. This is facilitated by a symbiotic relationship between AMF species, such as Rhizophagus irregularis, Glomus versiforme, and Funneliformis mosseae, which can increase the GRSP (Glomalin related soil protein) in soil (González-Chávez et al., 2004). As a direct result of this, the levels of lead and cadmium in maize decrease while the pH of the soil rises.
To further reduce heavy metal toxicity on host plants, AMF secrete extracellular polymeric substances (EPS) from their fungal surface through surface precipitation, ion exchange, and chelation (More et al., 2014; Riaz et al., 2021). EPS can absorb minerals and elements that are smaller than plant roots. Recent research suggests that phosphate groups with negative charges can cause Cr (III) to precipitate on the surface of fungi (Wu et al., 2021). The importance of glomalin and organic acid excretion by fungi and plants cannot be overstated when it comes to immobilizing 85% of heavy metals (HMs) in soil. By manipulating endophytic fungi, it is possible to minimize metal toxicity to plants, and some of these fungi can even flourish in environments rich in metals. Endophytic fungi possess a range of tolerance mechanisms contributing to their effectiveness in phytoremediation. These mechanisms include extracellular metal sequestration and precipitation, internal metal sequestration and complexation, compartmentation, volatilization, and metal binding to fungal cell walls. Such diverse strategies bolster the potential of phytoremediation efforts (Aly et al., 2011; Sharma and Kumar, 2021). The Festuca pratensis and Festuca arundinacea, infested with endophytic fungi, grew more biomass in their roots and shoots while significantly degrading the petroleum hydrocarbons in the soil despite growing in ancient petroleum-contaminated soil (Soleimani et al., 2010). The gibberellin-producing endophyte Penicillium janthinellum LK5 protects host plants from Cd-induced oxidative stress and membrane damage by decreasing lipid peroxidation and electrolytes and increasing reduced glutathione content and catalase activity (Khan et al., 2014). Canola biomass and Cd extraction efficiency were both increased when the endophytic fungus Lasiodiplodia sp. MXSF31 was introduced to Portulaca oleracea stems grown in Pb and Cd-contaminated soils (Zanganeh et al., 2022).
4.3 Nanoparticle-soil systems
Nanotechnology has become an essential tool to overcome various agricultural restrictions, including improving nutrient utilization efficiency, reducing toxicity from heavy metals, and enhancing soil fertility through bio-nano formulations (Dave and Chopda, 2014). Sustainable nano-formulations have been shown to improve both plant health and yield. However, the disproportionate usage of nanoparticles (NPs) in numerous fields has contributed to the buildup of these particles in soils, which kill microbiota and plant systems like heavy metals (Malik et al., 2022). Despite these challenges, the soil’s physicochemical and biological properties can influence the microbiome’s ecophysiology and the stability, toxicity, complexation, and mobility of NPs. NPs accumulated in soil can undergo biological, chemical, and physical changes when interacting with soil systems’ inorganic and organic components. Physical phenomena such as aggregation can reduce the mobility of NPs in soils, whether through hetero or homo interactions between the ambient particles and NPs (Balusamy et al., 2021; Goswami et al., 2022; Malik et al., 2022; Chebotaryova et al., 2023).
Furthermore, chemical changes to NPs can occur through surface dissolution, coating degradation, surface modification, abiotic and biotic routes, oxidation, and reduction. These changes are crucial in understanding the behavior of NPs in the soil and their potential impact on plant and soil health. Overall, the use of nanotechnology in agriculture has promising benefits, but careful consideration and monitoring of the behavior of NPs in the soil are necessary to minimize any potential adverse effects (Usman et al., 2020). Soil organic matter (SOM) has a role in the stabilization and absorption of NPs, making it one of many elements that affect their nature (stability and mobility), aggregations, and cohesiveness. Nanoparticles (NPs) can have their potential impacts mitigated by being absorbed by SOM, reducing the NPs’ surface-active area. Soil organic matter (SOM) has been found to increase the solubility of NPs in soil; for instance, CuO NPs were more soluble after SOM addition (Fato et al., 2019; Hemlata et al., 2020).
4.3.1 In what ways do nanoparticles and metals interact?
Heavy metals (HMs) and nanoparticles (NPs) coexist in agricultural settings can have devastating consequences for the soil, crop yields, and microbiota. However, the behavior of NPs can be influenced by several biological and environmental factors due to their distinct physicochemical properties. Therefore, various biotic variables may affect the interaction between HMs and NPs (da Silva et al., 2023; Sabourian et al., 2020). Moreover, the uptake, transport, and accumulation of NPs in different plant organs are also influenced by biotic factors. When present in polluted areas, heavy metals interact with NPs through physical adsorption, chemical interactions, and electrostatic binding (Noman et al., 2020). Such interactions can significantly impact the environment, accumulating these harmful substances in the soil and the food chain. Therefore, it is essential to understand the complex interactions between HMs and NPs in agricultural settings to minimize their adverse effects on the environment and public health. Examples include the adsorption of Cd from the soil by FeO NPs, which were able to do so because of their unique qualities, including reactivity, electrostatic attraction, a wide surface area, and the ability to cap molecules (Manzoor et al., 2021). In this situation, Ca and Fe transporters bring Cd into plant cells. This results in a lower metal concentration within the plant tissues as Cd and FeO NPs compete to enter the plant systems via the same transporter channel (Ahmed et al., 2021).
Similarly, Noman et al. (2020) found that Cu-NPs reduced Cd translocation from soil to aerial parts of wheat because of their wide surface area, reactivity, and electrostatic attraction. Hence, the biogenic CuNPs’ capping molecules boosted soil Cd immobilization. As a result, the wheat’s development was aided by the plant’s ability to absorb Cu-bound nutrients. It functions as a coenzyme in essential reactions and stimulates plant growth and development in polluted soil. As another example, graphene oxide (GO), which has a similarly huge surface area, has been utilized to clean up HMs contaminated areas (Etemadi et al., 2017). For instance, graphene oxide sheets, which, due to their functional group, may conjugate with metals like Cr (VI), speed up the adsorption kinetics of HMs ions (Wang et al., 2017). In conclusion, NPs’ metal complexing abilities are anticipated to aid in elucidating how the NPs may effectively reduce metal toxicity.
4.3.2 Techniques for reducing exposure to hazardous metals using nano-bioremediation
Across the globe, researchers have employed multiple approaches, such as physical, chemical, and biological techniques - including phyto and microbial remediation - to decontaminate soil polluted with heavy metals (Yadav et al., 2017; Singh et al., 2020; Sunanda et al., 2022). This is done to ensure that the soil becomes suitable for farming, considering the risks heavy metals pose to diverse life forms. Nevertheless, most of these techniques have only been tested in the lab at bench scales, and those tested in real-world settings have met with scant success for various reasons. Physicochemical methods include excavation and landfill (Funtikova et al., 2023), chemical reduction, evaporation acid leaching, soil washing, soil flushing, precipitation, electrokinetic extraction, vitrification, thermal treatment, and surface capping pose significant issues as mentioned in Table 9 (Rahman and Singh, 2020). The adverse impacts on soil, microbiota, and plant ecosystems are a direct result of the production of secondary metabolites, which can be costly and difficult to eradicate (Gaur et al., 2014; Wang P. et al., 2018). Table 10 outlines the utilization of nanoparticles in the remediation of heavy metals (HMs) and persistent organic pollutants (POPs), showcasing their efficacy in tackling environmental contaminants through innovative nanotechnology-based solutions.
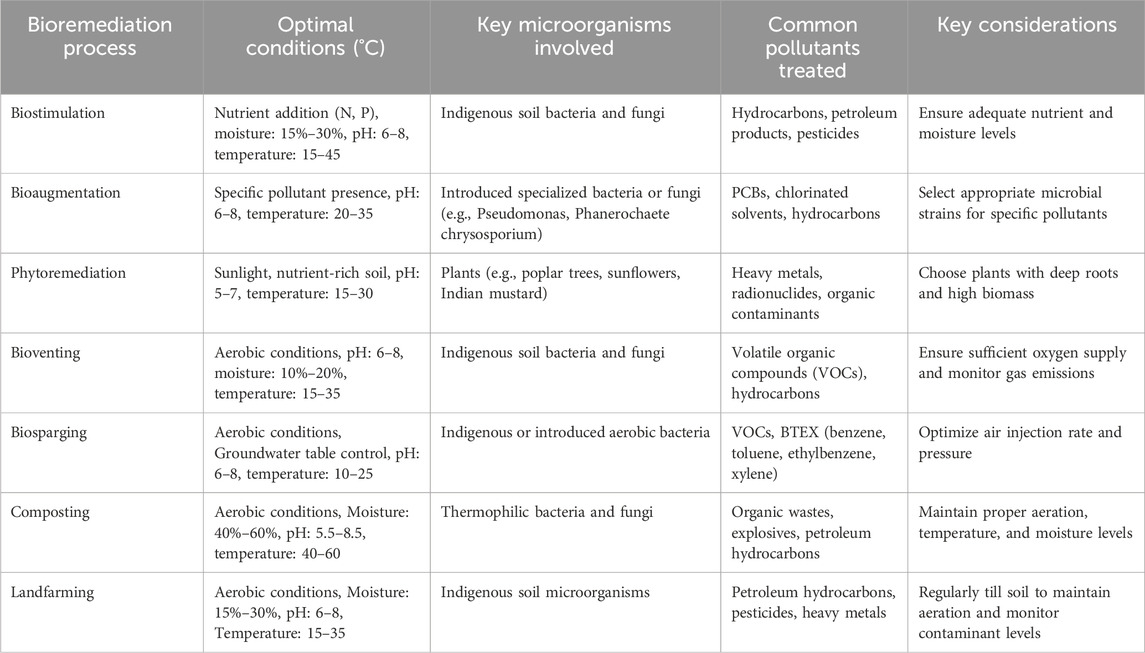
Table 9. Summary of the optimal conditions required for different types of bioremediation processes (Ali et al., 2022; Bhatt et al., 2022; Cepoi et al., 2022; Goswami et al., 2022; Chebotaryova et al., 2023).
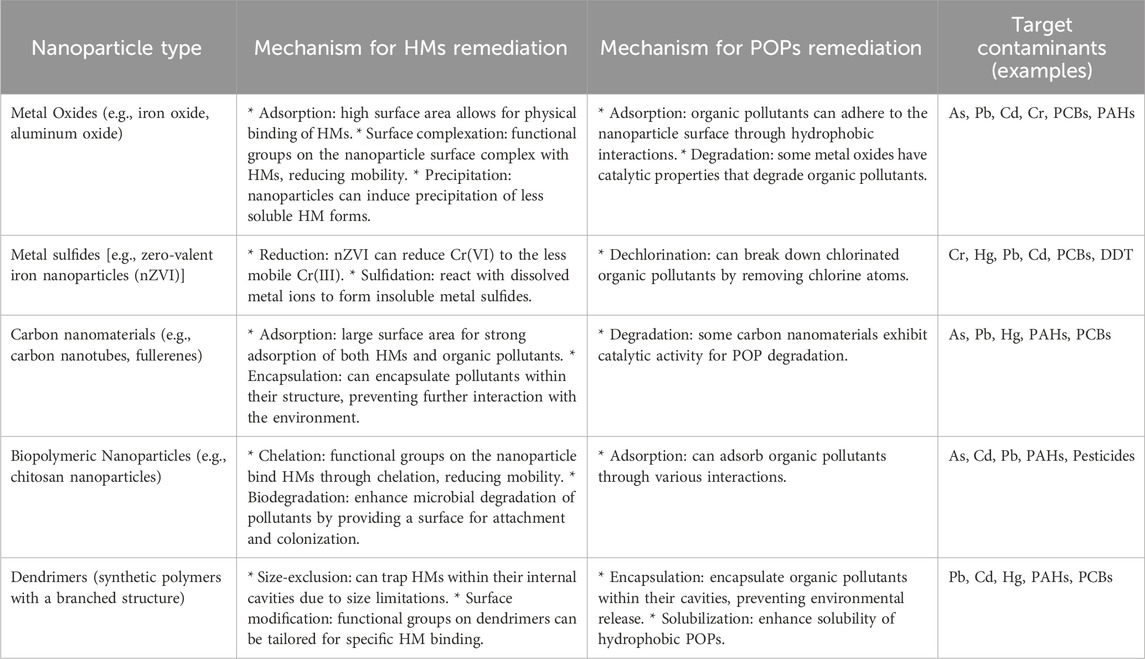
Table 10. Nanoparticles for remediation of heavy metals (HMs) and persistent organic pollutants (POPs) (Gaur et al., 2014; Wang et al., 2018; Singh et al., 2020; Sunanda et al., 2022; Yadav et al., 2017; Fulekar et al., 2012; Gavrilescu, 2022; Oladoye et al., 2022).
For instance, According to Lambert et al. (2000), FRTR (Federal Remediation-Technologies Roundtable) statistics suggest that excavation and disposal costs $270 to $460 per tonne (Feng et al., 2020; Hu et al., 2021). The United States Environmental Protection Agency (USEPA) estimates that, depending on the size of the polluted site, the cost of soil cleaning ranges from $150 per tonne up to $250 per tonne.
Selection and placement of plants, irrigation, soil amendment, field monitoring, harvesting, and residue management all add to the price tag of a treatment that relies on phytoextraction. The cost of remediation could range from $10 to $35 per ton of soil with low levels of toxins, which also depends on the contamination level and size of the site (Fulekar et al., 2012). Due to the plant-based nature of phytoremediation, the soil treatment process, which typically takes 3 months to 5 years, becomes more expensive and time-consuming (Gavrilescu, 2022; Oladoye et al., 2022). However, the clean-up has failed under natural field conditions due to reliance on specific pollutant characteristics, soil qualities, low efficiency, changing environments, and site conditions. Metal clean-up programs rely heavily on nanotechnology because of the unique physicochemical properties of nanosized particles (NPs) ranging in size from 1 to 100 nm. Furthermore, the nano remediation procedure has successfully removed heavy metals from soil ecosystems and other habitats by exploiting NPs’ potential mobility, reactivity (catalysis), and adsorption properties (Corsi et al., 2018; Baragaño et al., 2020; Del Prado-Audelo et al., 2021). Nanoremediation technology is one of the most promising remediation alternatives, and it removes toxic metals through a variety of mechanisms, including
(i) absorption,
(ii) oxide reduction to a stable metallic state,
(iii) heterogeneous catalysis,
(iv) deployment of electrical fields (electro-nano remediation),
(v) photodegradation, and
(vi) the use of biological materials (nano-bioremediation).
Various materials such as polymers, carbon-based compounds, metallic oxides, metals, and nanocomposites have remarkably removed metals (Baragaño et al., 2020). However, the type of metal and pollution source (e.g., biogenic) can affect the efficacy of these materials. Such materials include carbon nanoparticles (fullerenes), semiconductors, noble metals, and magnetic nanoparticles (such as zinc oxide and titanium dioxide). One specific example is Spirulina platensis supported PdNP, which removed between 12%–90% of Pd from polluted environments (Sayadi et al., 2018). On the other hand, an iron oxide nanoparticle-based on Geobacter sulfurreducens could remove chromium from chromium-polluted soils altogether (O’Neil et al., 2008). Overall, these findings highlight the potential of using different materials and approaches for effective metal removal, which could be tailored based on the type of pollutant and the specific environmental conditions. Nanoscale metal oxide particles (MONPs) made of iron, silver, nickel, and palladium have been remarkably successful in removing toxic metals and other chemicals from polluted areas. Detoxifying Cd stress in wheat plants using FeO NPs increased plant growth, antioxidants, and chlorophyll levels (Manzoor et al., 2021). Cd-phosphate production appears to be the leading cause for decreased bioaccumulation of Cd in soil, and treatment of the soil with Fe3 (PO4)2 NPs successfully immobilized the Cd by 70% (Gong et al., 2018).
Similarly, another study found that applying biochar-supported FeNPs reduced plant Cr bioavailability (Neeli et al., 2020). Wheat’s development and nutrient profile were found to be improved when Cu NPs were present, and vice versa (Noman et al., 2020). Notwithstanding the progress, new NPs that are effective in nano remediation technologies must be discovered. However, this requires researchers to collaborate with local governments, which can back innovations and fund research to identify sustainable nano-solutions for contaminated soils. Compared to traditional clean-up methods, nano remediation technology is typically swift, may be deployed over a broad contamination region, and costs less. According to the USEPA, about 70 potentially harmful trace elements have been effectively cleaned worldwide using nano-remediation techniques, considerably reducing time and operational costs (Feng et al., 2023; Tufail et al., 2022; Yang et al., 2023).
4.3.3 Microbiome-mediated nano-bioremediation of toxic metals
Microbes have long been used in various settings, including the medical, agricultural, and environmental sectors. However, as explained below, the application of microbiome to further optimize nanoparticle usage in the nano-bioremediation process has also shown promising results in detoxifying various inorganic contaminants, hence reducing the limiting potential of bioremediation (Bhatt et al., 2022). Microbiome-based nano-bioremediation has demonstrated substantial progress in detoxifying carcinogenic and mutagenic chromium by employing palladium nanoparticles (Alexakis, 2016). These nanoparticles are synthesized using Pd (II) ions, with the mediation of Clostridium pasteurianum. The process involves the conversion of hexavalent chromium into an insoluble trivalent form, resulting in hydrogen gas production.
Similarly, a matrix composed of carbon nanotubes (CNTs), sodium alginate, and polyvinyl alcohol (PVA) immobilized on P. aeruginosa has been shown to detoxify Cr (VI) selectively (Pang et al., 2011). At 80 mg/L Cr (VI), the immobilized bacterial cells converted 84% of the compound to the soluble Cr (III), and this process was completed within 24 h. Biotransformation of poisonous Cr (VI) into less harmful Cr (III) has been demonstrated by immobilised cells of Shewanella oneidensis stabilised with CNTs (Yan et al., 2013). Immobilized S. oneidensis and carbon nanotubes were four times more effective at removing hexavalent chromium from a solution than the test bacteria or calcium alginate beads alone. Based on these results, it is plausible that nano-bioremediation methods targeting habitats contaminated with inorganic pollutants could benefit from incorporating CNTs with bacteria. Magnetic iron oxide nanoparticles, known as MIONPs, have become popular for removing metals due to their extensive surface area, strong reactivity, adjustable features, distinctive magnetic qualities, potent reducing ability and capacity to soak up various dangerous metals and metalloids (Kumar et al., 2019; Verma et al., 2023). For instance, the Lysinibacillus sphaericus prepared magnetic oxide nanoparticles have been shown to release exopolysaccharides (EPS) that act as a complexing, stabilizing, and capping agent and have several binding sites for different metal ions. The EPS-functionalized magnetic oxide nanoparticles (VI) improve the ability to absorb Cr (Kumar et al., 2019).
Similarly, adding iron nanoparticles, produced by Chlorococcum sp. green algae, led to a 92% reduction of Cr (VI) to Cr (III). These nanoparticles were highly reactive, stable, and had a practical ability to reduce (Subramaniyam et al., 2015). Further strengthening algae’s role in detoxifying carcinogenic chromium is the incorporation of C. vulgaris as a functionalized agent in ultrafine bi-metallic (TiO2/Ag) chitosan nanofiber mats. According to this study, combining C. vulgaris algae with TiO2/Ag chitosan nanofiber mats significantly boosted the photocatalytic reduction of hexavalent chromium. The researchers observed that various organic compounds secreted by the algae played a crucial role in enhancing the process. As a result, the study implies that the synergistic effect between the algae and TiO2/Ag hybrid nanomaterial could offer a cost-effective solution for removing chromium from polluted environments (Goher et al., 2016; Awasthi et al., 2018). Rhodosporidium diobovatum was responsible for generating lead sulfide (PbS) nanoparticles, which effectively converted toxic Pb (II) ions into less harmful and advantageous compounds (Seshadri et al., 2011). Combining B. subtilis and nanohydroxyapatite and the production of CdS nanoparticles from P. aeruginosa (NHAP) successfully eliminated Cd from a Cd-contaminated environment. Implementing this remediation approach stimulated the rhizosphere community, leading to a notable rise in bacterial diversity in rapeseed (Brassica campestris L.) cultivated in previously contaminated soil (Liu et al., 2018).
4.3.4 Nanoparticles in remediation of POPs
Economic and technological variables must be considered when selecting a treatment technique for POP removal, as they significantly impact POP destiny, transport, and degradation (Zhou et al., 2023). With the rise of nanotechnology, a powerful tool is now available to tackle environmental problems, specifically in purifying polluted treatment solutions. Nanoremediation is a state-of-the-art method that may safely and effectively remove organic contaminants from the environment. Nanomaterials are highly beneficial in many fields due to their extraordinary electromagnetic, structural, mechanical, thermal, and optical capabilities, i.e., in wastewater treatment (Del Prado-Audelo et al., 2021). Various forms of nanomaterials can be created through different methods, including physical, chemical, or biological processes. Many researchers also utilize green chemistry principles to ensure environmentally friendly synthesis. Cutting-edge multifunctional nanomaterials such as nanowires, nanoflowers, and nanocomposites are designed to optimize performance and address existing obstacles (Corsi et al., 2018). Higher specific surface area (SVR) of nanomaterials enhances their reactivity with POPs. In the upcoming sections, we will delve into nanocatalysis, nano adsorbents, and nanomembranes in POP treatment.
4.3.4.1 Nanocatalysis
Traditional technologies have proven ineffective in completely breaking down and eliminating organic pollutants, leading to a need for a more sustainable approach that minimizes energy and chemical consumption. Exploring advanced oxidation processes (AOPs) as cost-effective solutions due to their powerful oxidizing radicals. Nanocatalysis has emerged as a promising approach for transforming contaminants into eco-friendly compounds by utilizing semiconducting wide-bandgap nanomaterials (Baragaño et al., 2020). Metal and metal-oxide nanomaterials are increasingly recognized for their potential in addressing persistent organic pollutants (POPs) sustainably. Different nanocatalysts, such as Fenton-based, electrocatalytic, and photocatalytic, are used to degrade POPs. Photocatalysis, a widely recognized advanced oxidation process (AOP), utilizes light to activate nanocatalysts, producing reactive oxygen species (ROS) that efficiently break down organic pollutants (Fei et al., 2022). This process is highly effective in dealing with volatile organic compounds (VOCs) such as Dioxins and polychlorinated biphenyls (PCBs) by producing free radicals. When certain nanocatalysts, like ZnO, TiO2, or WO3, are exposed to light and oxygen, they become excited and can break down POPs through photocatalysis. Currently, TiO2 and ZnO are the primary semiconductors employed to degrade POPs (Nandini et al., 2023). Their ability to effectively remove highly hydrophobic POPs is impressive. Important considerations for catalyst selection involve surface characteristics, pore volume, and material structure. Optimizing surface properties and crystal structure improves degradation efficiency. On the other hand, one downside of photocatalysis is the difficulty in eliminating nanomaterial after the reaction.
Lwin et al. (2019) previously produced a cube-shaped ZnO-SnO2 nanocomposite, showing that it effectively degraded tetracycline hydrochloride. This material showed exceptional photostability throughout its four cycles, which suggests it is suitable for its potential use in cleaning up organic pollutants like POPs. Amir et al. (2016) introduced a nanocatalyst designated MnFe2O4@PANI@Ag to break down azo dye in their investigation. This nanocatalyst has demonstrated sustained performance during numerous cycles and has the added benefit of being quickly separated with an external magnet. Khan et al. (2018) developed a magnetic Fe-ZnO nanocomposite that efficiently removed the insecticide Chlorpyrifos. Keeping its excellent stability and reusability, the nanocomposite showed remarkable performance, degrading the pesticide quickly. A recent study by Chen et al. (2022) focused on Mn-based nanocomposites and their ability to degrade bisphenol A. The researchers found that these nanocomposites exhibited impressive mineralization and BPA removal efficiency, maintaining their high performance even after multiple cycles. Photocatalysis is a popular choice for wastewater treatment due to its high efficiency and sustainability in combating a wide range of pollutants.
4.3.4.2 Nanoadsorption
With their extensive surface area, adjustable pore size, minimized intraparticle-diffusion distance, and powerful surface activity, nano adsorbents demonstrate exceptional sorption efficiency, effectively trapping a diverse range of pollutants. They can be easily tailored to target specific pollutants, which enhances their selectivity. This technology has effectively eliminated persistent organic pollutants (POPs) such as hydrocarbons, dyes, phenols, and pesticides (Chen et al., 2022). Nanoadsorption has proven to be a highly effective technique for POP remediation by utilizing electrostatic, hydrogen bonding, and hydrophobic interactions. Numerous nanomaterials, such as carbon-based nanomaterials, metal oxides, zeolite, and clay, are widely used in this process. Introducing innovative magnetic separation strategies, magnetic nanoparticles, especially iron oxide, play a crucial role. The microporous structure of activated carbon improves the efficiency of removing POPs, while nano adsorbents made from carbon can interact with contaminants. Carbon nanotubes can significantly boost their adsorption capacity with surface modifications, making them highly efficient in removing pollutants. The adsorption of cyanazine through iron nanocomposites produced using green technologies was examined in a study by Ali et al. (2022). The results showed that cyanazine was rapidly removed, which can be attributed to the short contact time. In recent years, Mahdavi et al. (2021) successfully applied magnetic-graphene oxide treated with amino-guanidine to eliminate chlorpyrifos pesticide. The researchers observed significant desorption through HPLC-MS analysis using a synthesized nano adsorbent. In a recent study, Izanloo et al. (2019) created a nano adsorbent (Fe3O4@SiO2@NH2@SH) that effectively removed 2,4-D and lead from contaminated environments. The researchers found that the pH level played a critical role in the adsorption of organic contaminants.
Additionally, the nano adsorbent demonstrated consistent desorption efficiency even after multiple cycles. In a recent study, Mohammadi et al. (2018) researched a modified magnetic nano adsorbent. They focused on its ability to rapidly separate pollutants and effectively remove phenoxy-acid herbicides such as 2,4-D and MCPA. Dehghani et al. (2019) evaluated the potential of multi-walled carbon nanotubes (MWCNTs) to remove the herbicide diazinon. They discovered that at pH 6, diazinon was completely removed after 15 min, demonstrating the efficacy of MWCNTs in pesticide cleanup. Utilizing nano adsorbents in wastewater treatment can provide a practical and environmentally friendly approach to removing heavy metals. Additionally, the magnetic variants of these adsorbents can be conveniently separated using external magnets, resulting in reduced operational expenses.
4.3.4.3 Nanofiltration
The introduction of nanofiltration membranes has significantly transformed water treatment systems, bringing about a revolution in nanotechnology. These membranes, along with microfiltration (MF), reverse osmosis (RO), and ultrafiltration (UF), provide highly efficient methods for wastewater treatment, offering alternatives to conventional techniques. Membrane processes are known for their remarkable removal efficiency, especially in organic micropollutants, although they can be quite expensive (Corina-Petronela and Teodosiu, 2007). Their functionality is significantly enhanced by incorporating nanoparticles into membranes using different techniques such as surface immobilization or blending. Electrospinning allows the creation of polymeric or composite nanofibrous membranes that provide incredibly precise filtration ranging from 10 to 1,000 nm. Micro/trace organic pollution can be effectively filtered using membrane techniques like reverse osmosis (RO) and nanofiltration (NF). NF, in particular, is known for its effectiveness thanks to its smaller pore sizes and user-friendly nature (Tibi et al., 2020). Nanofiltration membranes are constructed using a variety of polymers, some of which are naturally occurring and others of which are synthetic. These comprise polyvinyl fluoride, polypropylene, polyacrylonitrile, and cellulose acetate. With their stable adsorption structures, nanofibers effectively eliminate pesticides from wastewater through molecular propagation. Incorporating semiconducting materials into nanofibers enhances their efficiency in dye compound remediation by giving them photocatalytic properties (Oatley-Radcliffe et al., 2017). These nanocomposite nanofiber membranes, such as ZnO-cellulose acetate and TiO2-graphene, exhibit remarkable photocatalytic efficiency. In addition, combining magnetic nanoparticles with membranes and adding TiO2 can significantly improve the ability to remediate organic pollutants.
Different filtration techniques, such as ultrafiltration, microfiltration, and nanofiltration, are used to eliminate organic and inorganic pollutants effectively. When combined with biological or chemical methods, filtration can significantly improve the efficiency of remediation. However, the success of this approach depends on various factors, including the type of membrane, modules, composition, and how well it interacts with pollutants. Using pressure dynamics, nanofiltration efficiently targets compounds with low molecular weight (1–10 nm) and reduces the hardness of organic pollutants, decreasing ionic strength. Electrospinning creates nanofibrous membranes that are essential for achieving optimal filtration performance. Nanofiltration is an excellent method for removing arsenic from water because it can effectively separate soluble minerals and other ions.
Karimi-Shamsabadi et al. (2016) examined the efficacy of a thin-film composite poly-amide nanofiltration membrane in removing atrazine and diazinon from wastewater. The researchers found that the membrane had a higher rejection rate for diazinon than atrazine. The modified membranes showed improved water permeability and diazinon rejection, suggesting better pesticide removal performance. In a recent study, Wang et al. (2020) introduced a new type of nanocomposite catalyst. This catalyst, called Al-MOF/Fe3O4/PDA@Ag, contains silver nanoparticles and has shown impressive performance in eliminating organic pollutants such as CIP, NOR, and MO. One of the notable advantages of this catalyst is its ability to be easily separated using an external magnet. Additionally, it has demonstrated good reusability and stability, making it a promising option for future applications. Membrane filtration, especially nanofiltration, is widely acknowledged as a secure technology for effectively eliminating low-molecular-weight compounds and pesticides. However, the issue of membrane fouling remains a persistent challenge that can be overcome by utilizing blended techniques.
5 Factors affecting bioremediation
Bioremediation, the process of using living organisms to remove or neutralize contaminants from the environment, is influenced by many factors. These factors can significantly impact the efficiency and effectiveness of bioremediation efforts (Yang Y.-C. et al., 2020). Understanding these factors is crucial for designing and implementing successful bioremediation strategies. One of the primary factors affecting bioremediation is the type and concentration of contaminants present in the environment. Different contaminants require specific microbial communities and enzymatic pathways for degradation. For instance, hydrocarbon-degrading bacteria effectively remove petroleum-based pollutants, while heavy metal-contaminated sites may require metal-resistant bacteria or plants with metal-accumulating capabilities (Yetunde Mutiat et al., 2018).
Additionally, high concentrations of contaminants can inhibit microbial activity, so it is essential to optimize conditions to ensure microbial growth and activity. Environmental conditions such as temperature, pH, oxygen availability, and moisture content also play a critical role in bioremediation (Yadav et al., 2017). Most microbial activity occurs within specific temperature and pH ranges, and extreme conditions can hinder microbial growth and metabolism. Adequate oxygen levels are necessary for aerobic degradation processes, while anaerobic conditions may be required to reduce specific contaminants. Similarly, moisture content affects microbial activity and nutrient availability, with excessive dryness or water saturation inhibiting bioremediation processes (Wang et al., 2023).
The availability of nutrients such as carbon, nitrogen, and phosphorus is another critical factor influencing bioremediation (Tufail et al., 2022). Microorganisms require these nutrients for growth and metabolism, and their availability can limit microbial activity in contaminated environments. Supplementing nutrients through techniques like fertilization or bioaugmentation can enhance microbial growth and biodegradation rates, particularly in nutrient-poor environments. The microbial community present in the contaminated site also significantly influences bioremediation outcomes (Talukdar et al., 2020). Indigenous microorganisms may already possess the metabolic capabilities required for contaminant degradation, potentially reducing the need for external intervention. However, in some cases, the indigenous microbial community may be insufficient to effectively remediate contaminants, necessitating the introduction of specialized microbial consortia or genetically engineered microorganisms. The accessibility and permeability of the contaminated matrix also impact bioremediation efficiency. Contaminants within soil aggregates, pores, or dense matrices may be less accessible to microbial degradation, requiring physical or chemical pretreatment to enhance accessibility. Similarly, contaminants in groundwater or deep soil layers may be more challenging to reach and treat effectively (Sreedevi et al., 2022).
Furthermore, external factors such as regulatory requirements, public perception, and economic considerations can influence bioremediation project planning and implementation. Compliance with environmental regulations, stakeholder engagement, and cost-effectiveness are essential considerations in designing bioremediation strategies.
6 Conclusion
In conclusion, the issue of heavy metal and POPs pollution poses a significant threat to both the environment and human health. To address this problem, bioremediation utilizing microorganisms and plants has emerged as a promising technology to detoxify contaminated soil. Certain microorganisms and plants exhibit strong metal adsorption capabilities, making them well-suited for bioremediation efforts. However, the effectiveness of phytoremediation has been hindered by the slow growth of plants and low remediation efficiency. To overcome these limitations, using plant-associated microbes, particularly PGPR, can enhance the removal efficiency of heavy metals in contaminated soil.
Moreover, nanotechnology offers the potential to remediate hazardous metals, and integrating nanoparticles with bioremediation, known as nano-bioremediation, holds promise for removing harmful contaminants. Understanding the interactions between the soil microbiome, nanoparticles, and contaminants is pivotal for successfully implementing nano-remediation strategies and optimizing crops in contaminated fields. Overall, developing and implementing efficient and sustainable bioremediation strategies for heavy metal pollution are crucial in safeguarding the environment and human health.
Author contributions
AK: Conceptualization, Formal Analysis, Resources, Supervision, Writing–original draft. SM: Conceptualization, Formal Analysis, Resources, Writing–original draft, Validation. AD: Validation, Visualization, Writing–review and editing, Data curation. AA-T: Data curation, Writing–review and editing, Formal Analysis, Investigation, Visualization. TM: Resources, Validation, Writing–review and editing, Data curation, Formal Analysis.
Funding
The author(s) declare that no financial support was received for the research, authorship, and/or publication of this article.
Acknowledgments
I am obliged to conduct my research at Lovely Professional University, Phagwara, and Jimma University, Ethiopia.
Conflict of interest
The authors declare that the research was conducted in the absence of any commercial or financial relationships that could be construed as a potential conflict of interest.
Publisher’s note
All claims expressed in this article are solely those of the authors and do not necessarily represent those of their affiliated organizations, or those of the publisher, the editors and the reviewers. Any product that may be evaluated in this article, or claim that may be made by its manufacturer, is not guaranteed or endorsed by the publisher.
References
Abbasi, S., Zahedi, H., Sadeghipour, O., and Akbari, R. (2013). Effect of plant growth promoting rhizobacteria (PGPR) on physiological parameters and nitrogen content of soybean grown under different irrigation regimes. Res. Crops 14 (3), 798–803.
Abdu, N., Abdullahi, A. A., and Abdulkadir, A. (2017). Heavy metals and soil microbes. Environ. Chem. Lett. 15 (1), 65–84. doi:10.1007/s10311-016-0587-x
Abedinzadeh, M., Etesami, H., and Alikhani, H. A. (2019). Characterization of rhizosphere and endophytic bacteria from roots of maize (Zea mays L.) plant irrigated with wastewater with biotechnological potential in agriculture. Biotechnol. Rep. 21, e00305. doi:10.1016/j.btre.2019.e00305
Adebusuyi, A. T., Sojinu, S. O., and Aleshinloye, A. O. (2022). The prevalence of persistent organic pollutants (POPs) in West Africa – a review. Environ. Challenges 7, 100486. doi:10.1016/j.envc.2022.100486
Adeyemi, N. O., Atayese, M. O., Sakariyawo, O. S., Azeez, J. O., Abayomi Sobowale, S. P., Olubode, A., et al. (2021). Alleviation of heavy metal stress by arbuscular mycorrhizal symbiosis in Glycine max (L.) grown in copper, lead and zinc contaminated soils. Rhizosphere 18, 100325. doi:10.1016/j.rhisph.2021.100325
Agarwal, M., Rathore, R. S., Jagoe, C., and Chauhan, A. (2019). Multiple lines of evidences reveal mechanisms underpinning mercury resistance and volatilization by stenotrophomonas sp. MA5 isolated from the savannah river site (SRS), USA. Cells 8 (4), 309. doi:10.3390/cells8040309
Ahkami, A. H., Allen White, R., Handakumbura, P. P., and Jansson, C. (2017). Rhizosphere engineering: enhancing sustainable plant ecosystem productivity. Rhizosphere 3, 233–243. doi:10.1016/j.rhisph.2017.04.012
Ahmed, T., Noman, M., Manzoor, N., Shahid, M., Abdullah, M., Ali, L., et al. (2021). Nanoparticle-based amelioration of drought stress and cadmium toxicity in rice via triggering the stress responsive genetic mechanisms and nutrient acquisition. Ecotoxicol. Environ. Saf. 209, 111829. doi:10.1016/j.ecoenv.2020.111829
Al-Enazi, N. M., AlTami, M. S., and Alhomaidi, E. (2022). Unraveling the potential of pesticide-tolerant Pseudomonas sp. augmenting biological and physiological attributes of Vigna radiata (L.) under pesticide stress. RSC Adv. 12, 17765–17783. doi:10.1039/D2RA01570F
Alexakis, D. (2016). Human health risk assessment associated with Co, Cr, Mn, Ni and V contents in agricultural soils from a Mediterranean site. Archives Agron. Soil Sci. 62 (3), 359–373. doi:10.1080/03650340.2015.1062088
Al-Huqail, A. A., and El-Bondkly, A. M. A. (2022). Improvement of Zea mays L. growth parameters under chromium and arsenic stress by the heavy metal-resistant Streptomyces sp. NRC21696. Int. J. Environ. Sci. Technol. 19 (6), 5301–5322. doi:10.1007/s13762-021-03532-7
Ali, M., Song, X., Ding, D., Wang, Q., Zhang, Z., and Tang, Z. (2022). Bioremediation of PAHs and heavy metals co-contaminated soils: challenges and enhancement strategies. Environ. Pollut. 295, 118686. doi:10.1016/j.envpol.2021.118686
Aly, A. H., Debbab, A., and Proksch, P. (2011). Fungal endophytes: unique plant inhabitants with great promises. Appl. Microbiol. Biotechnol. 90 (6), 1829–1845. doi:10.1007/s00253-011-3270-y
Ambreetha, S., Chinnadurai, C., Marimuthu, P., and Balachandar, D. (2018). Plant-associated Bacillus modulates the expression of auxin-responsive genes of rice and modifies the root architecture. Rhizosphere 5, 57–66. doi:10.1016/j.rhisph.2017.12.001
Amir, M., Kurtan, U., Baykal, A., and Sözeri, H. (2016). MnFe2O4@PANI@Ag heterogeneous nanocatalyst for degradation of industrial aqueous organic pollutants. J. Mater. Sci. Technol. 32, 134–141. doi:10.1016/j.jmst.2015.12.011
Arif, M. S., Yasmeen, T., Shahzad, S. M., Riaz, M., Rizwan, M., Iqbal, S., et al. (2019). Lead toxicity induced phytotoxic effects on mung bean can be relegated by lead tolerant Bacillus subtilis (PbRB3). Chemosphere 234, 70–80. doi:10.1016/j.chemosphere.2019.06.024
Arwidsson, Z., Johansson, E., Von Kronhelm, T., Allard, B., and Van Hees, P. (2010). Remediation of metal contaminated soil by organic metabolites from fungi i-production of organic acids. Water, Air, Soil Pollut. 205 (1-4), 215–226. doi:10.1007/s11270-009-0067-z
Asati, A., Pichhode, M., and Nikhil, K. (2016). Effect of heavy metals on plants: an overview. IJAIEM 5 (3), 56–66.
Awasthi, S., Chauhan, R., Dwivedi, S., Srivastava, S., Srivastava, S., and Tripathi, R. D. (2018). A consortium of alga (Chlorella vulgaris) and bacterium (Pseudomonas putida) for amelioration of arsenic toxicity in rice: a promising and feasible approach. Environ. Exp. Bot. 150, 115–126. doi:10.1016/j.envexpbot.2018.03.001
Azzam, A. M., and Tawfik, A. (2015). Removal of heavy metals using bacterial bio-flocculants of Bacillus sp. and Pseudomonas sp. J. Environ. Eng. Landsc. Manag. 23 (4), 288–294. doi:10.3846/16486897.2015.1068781
Ballesteros, S., Rincón, J. M., Rincón-Mora, B., and Jordán, M. M. (2017). Vitrification of urban soil contamination by hexavalent chromium. J. Geochem. Explor. 174, 132–139. doi:10.1016/j.gexplo.2016.07.011
Balusamy, S. R., Karuppieh, S., Venkat, S., Thangavelu, L., Kim, Y. J., and Perumalsamy, H. (2021). “Biomedical applications of ginsenosides nanoparticles synthesized using microbes,” in Agri-waste and microbes for production of sustainable nanomaterials, 625–653.
Bandaru, S. R. S., Van Genuchten, C. M., Kumar, A., Glade, S., Hernandez, D., Nahata, M., et al. (2020). Rapid and efficient arsenic removal by iron electrocoagulation enabled with in situ generation of hydrogen peroxide. Environ. Sci. Technol. 54 (10), 6094–6103. doi:10.1021/acs.est.0c00012
Bano, A., Hussain, J., Akbar, A., Mehmood, K., Anwar, M., Hasni, M. S., et al. (2018). Biosorption of heavy metals by obligate halophilic fungi. Chemosphere 199, 218–222. doi:10.1016/j.chemosphere.2018.02.043
Baragaño, D., Forján, R., Welte, L., and Gallego, J. L. R. (2020). Nanoremediation of as and metals polluted soils by means of graphene oxide nanoparticles. Sci. Rep. 10 (1), 1896. doi:10.1038/s41598-020-58852-4
Baran, M. F., and Duz, M. Z. (2021). Removal of cadmium (II) in the aqueous solutions by biosorption of Bacillus licheniformis isolated from soil in the area of Tigris River. Int. J. Environ. Anal. Chem. 101 (4), 533–548. doi:10.1080/03067319.2019.1669583
Barker, A. V., and Bryson, G. M. (2002). Bioremediation of heavy metals and organic toxicants by composting. ScientificWorldJournal 2, 407–420. doi:10.1100/tsw.2002.91
Barnawal, D., Bharti, N., Pandey, S. S., Pandey, A., Chanotiya, C. S., and Kalra, A. (2017). Plant growth-promoting rhizobacteria enhance wheat salt and drought stress tolerance by altering endogenous phytohormone levels and TaCTR1/TaDREB2 expression. Physiol. Plant. 161 (4), 502–514. doi:10.1111/ppl.12614
Barra Caracciolo, A., and Terenzi, V. (2021). Rhizosphere microbial communities and heavy metals. Microorganisms 9 (7), 1462. doi:10.3390/microorganisms9071462
Baruah, N., Mondal, S. C., Farooq, M., and Gogoi, N. (2019). Influence of heavy metals on seed germination and seedling growth of wheat, pea, and tomato. Water, Air, Soil Pollut. 230 (12), 273. doi:10.1007/s11270-019-4329-0
Becze, A., Vincze, É. B., Varga, H. M., and Gyöngyvér, M. (2021). Effect of plant growth promoting rhizobacteria on zea mays development and growth under heavy metal and salt stress condition. Environ. Eng. Manag. J. 20 (4), 547–557. doi:10.30638/eemj.2021.053
Belimov, A. A., Shaposhnikov, A. I., Azarova, T. S., Makarova, N. M., Safronova, V. I., Litvinskiy, V. A., et al. (2020). Microbial consortium of PGPR, rhizobia and arbuscular mycorrhizal fungus makes pea mutant SGECdt comparable with indian mustard in cadmium tolerance and accumulation. Plants 9 (8), 975–1021. doi:10.3390/plants9080975
Bhati, R., Sreedharan, S. M., Rizvi, A., Khan, M. S., and Singh, R. (2022). An insight into efflux-mediated arsenic resistance and biotransformation potential of Enterobacter cloacae RSC3 from arsenic polluted area. Indian J. Microbiol. 62 (3), 456–467. doi:10.1007/s12088-022-01028-7
Bhatt, P., Pandey, S. C., Joshi, S., Chaudhary, P., Pathak, V. M., Huang, Y., et al. (2022). Nanobioremediation: a sustainable approach for the removal of toxic pollutants from the environment. J. Hazard. Mater. 427, 128033. doi:10.1016/j.jhazmat.2021.128033
Bhattacharya, S., Das, A., Prashanthi, K., Palaniswamy, M., and Angayarkanni, J. (2014). Mycoremediation of Benzo[a]pyrene by Pleurotus ostreatus in the presence of heavy metals and mediators. 3 Biotech. 4 (2), 205–211. doi:10.1007/s13205-013-0148-y
Bobaker, A. M., Alakili, I., Sarmani, S. B., Al-Ansari, N., and Yaseen, Z. M. (2019). Determination and assessment of the toxic heavy metal elements abstracted from the traditional plant cosmetics and medical remedies: case study of Libya. Int. J. Environ. Res. Public Health 16 (11), 1957. doi:10.3390/ijerph16111957
Boente, C., Sierra, C., Rodríguez-Valdés, E., Menéndez-Aguado, J. M., and Gallego, J. R. (2017). Soil washing optimization by means of attributive analysis: case study for the removal of potentially toxic elements from soil contaminated with pyrite ash. J. Clean. Prod. 142, 2693–2699. doi:10.1016/j.jclepro.2016.11.007
Briffa, J., Sinagra, E., and Blundell, R. (2020). Heavy metal pollution in the environment and their toxicological effects on humans. Heliyon 6 (9), e04691. doi:10.1016/j.heliyon.2020.e04691
Brim, H., Venkateswaran, A., Kostandarithes, H. M., Fredrickson, J. K., Daly, M. J. J. A., and microbiology, e. (2003). Engineering Deinococcus geothermalis for bioremediation of high-temperature radioactive waste environments. Appl. Environ. Microbiol. 69 (8), 4575–4582. doi:10.1128/aem.69.8.4575-4582.2003
Çabuk, A., Akar, T., Tunali, S., and Tabak, O. (2006). Biosorption characteristics of Bacillus sp. ATS-2 immobilized in silica gel for removal of Pb(II). J. Hazard. Mater. 136 (2), 317–323. doi:10.1016/j.jhazmat.2005.12.019
Camargo, F. P., Prado, P. F. D., Tonello, P. S., Dos Santos, A. C. A., and Duarte, I. C. S. (2018). Bioleaching of toxic metals from sewage sludge by co-inoculation of Acidithiobacillus and the biosurfactant-producing yeast Meyerozyma guilliermondii. J. Environ. Manage 211, 28–35. doi:10.1016/j.jenvman.2018.01.045
Cameron, H., Mata, M. T., and Riquelme, C. (2018). The effect of heavy metals on the viability of Tetraselmis marina AC16-MESO and an evaluation of the potential use of this microalga in bioremediation. PeerJ 6, e5295. doi:10.7717/peerj.5295
Cepoi, L., Zinicovscaia, I., Valuta, A., Codreanu, L., Rudi, L., Chiriac, T., et al. (2022). Bioremediation capacity of edaphic cyanobacteria Nostoc linckia for chromium in association with other heavy-metals-contaminated soils. Environ. - MDPI 9 (1), 1. doi:10.3390/environments9010001
Chakraborty, R., Asthana, A., Singh, A. K., Jain, B., and Susan, A. B. H. (2022). Adsorption of heavy metal ions by various low-cost adsorbents: a review. Int. J. Environ. Anal. Chem. 102 (2), 342–379. doi:10.1080/03067319.2020.1722811
Chamekh, A., Kharbech, O., Driss-Limam, R., Fersi, C., Khouatmeya, M., and Chouari, R. (2021). Evidences for antioxidant response and biosorption potential of Bacillus simplex strain 115 against lead. World J. Microbiol. Biotechnol. 37 (3), 44. doi:10.1007/s11274-021-03009-2
Changmai, M., Pasawan, M., and Purkait, M. K. (2019). Treatment of oily wastewater from drilling site using electrocoagulation followed by microfiltration. Sep. Purif. Technol. 210, 463–472. doi:10.1016/j.seppur.2018.08.007
Chebotaryova, S. P., Zakharova, O. V., Gusev, A. A., Baranchikov, P. A., Kolesnikov, E. A., Yakusheva, A. S., et al. (2023). Assessment of the tolerance of a chlorophyte Desmodesmus to CuO-NP for evaluation of the nanopollution bioremediation potential of this microalga. Nanomater. (Basel) 13 (4), 737. doi:10.3390/nano13040737
Chen, B., Luo, S., Wu, Y., Ye, J., Wang, Q., Xu, X., et al. (2017). The effects of the endophytic bacterium Pseudomonas fluorescens Sasm05 and IAA on the plant growth and cadmium uptake of Sedum alfredii hance. Front. Microbiol. 8 (DEC), 2538. doi:10.3389/fmicb.2017.02538
Chen, L., Zhang, X., Zhang, M., Zhu, Y., and Zhuo, R. (2022). Removal of heavy-metal pollutants by white rot fungi: mechanisms, achievements, and perspectives. J. Clean. Prod. 354, 131681. doi:10.1016/j.jclepro.2022.131681
Chen, W., and Li, H. (2018). Cost-effectiveness analysis for soil heavy metal contamination treatments. Water, Air, Soil Pollut. 229 (4), 126. doi:10.1007/s11270-018-3784-3
Chen, Y., Hu, K., and Chen, Y. (2019). The effect of biotic and abiotic environmental factors on Pd(II) adsorption and reduction by Bacillus megaterium Y-4. Chemosphere 220, 1058–1066. doi:10.1016/j.chemosphere.2019.01.011
Chen, Z., Huang, Z., Cheng, Y., Pan, D., Pan, X., Yu, M., et al. (2012). Cr(VI) uptake mechanism of Bacillus cereus. Chemosphere 87 (3), 211–216. doi:10.1016/j.chemosphere.2011.12.050
Cheng, J., Yin, W., Chang, Z., Lundholm, N., and Jiang, Z. (2017). Biosorption capacity and kinetics of cadmium(II) on live and dead Chlorella vulgaris. J. Appl. Phycol. 29 (1), 211–221. doi:10.1007/s10811-016-0916-2
Concetta Tomei, M., and Daugulis, A. J. (2013). Ex situ bioremediation of contaminated soils: an overview of conventional and innovative technologies. Crit. Rev. Environ. Sci. Technol. 43 (20), 2107–2139. doi:10.1080/10643389.2012.672056
Corina-Petronela, M., and Teodosiu, C. (2007). Removal of persistent organic pollutants from textile wastewater by membrane processes. Environmental Engineering and Management Journal (EEMJ) 6, 3.
Corsi, I., Winther-Nielsen, M., Sethi, R., Punta, C., Della Torre, C., Libralato, G., et al. (2018). Ecofriendly nanotechnologies and nanomaterials for environmental applications: key issue and consensus recommendations for sustainable and ecosafe nanoremediation. Ecotoxicol. Environ. Saf. 154, 237–244. doi:10.1016/j.ecoenv.2018.02.037
DalCorso, G., Fasani, E., Manara, A., Visioli, G., and Furini, A. (2019). Heavy metal pollution: state of the art and innovation in phytoremediation. Int. J. Mol. Sci. 20 (14), 3412. doi:10.3390/ijms20143412
Daneshvar, E., Zarrinmehr, M. J., Koutra, E., Kornaros, M., Farhadian, O., and Bhatnagar, A. (2019). Sequential cultivation of microalgae in raw and recycled dairy wastewater: microalgal growth, wastewater treatment and biochemical composition. Bioresour. Technol. 273, 556–564. doi:10.1016/j.biortech.2018.11.059
Das, S., Das, S., and Ghangrekar, M. M. (2022). Efficacious bioremediation of heavy metals and radionuclides from wastewater employing aquatic macro- and microphytes. J. Basic Microbiol. 62 (3-4), 260–278. doi:10.1002/jobm.202100372
Dash, D. M., and Osborne, W. J. (2023). A systematic review on the implementation of advanced and evolutionary biotechnological tools for efficient bioremediation of organophosphorus pesticides. Chemosphere 313, 137506. doi:10.1016/j.chemosphere.2022.137506
Dash, S., Chaudhuri, H., Gupta, R., and Nair, U. G. (2018). Adsorption study of modified coal fly ash with sulfonic acid as a potential adsorbent for the removal of toxic reactive dyes from aqueous solution: kinetics and thermodynamics. J. Environ. Chem. Eng. 6 (5), 5897–5905. doi:10.1016/j.jece.2018.05.017
da Silva, J. R. R., Gregorio, A., Portela-Castro, A. L. B., and Fernandes, C. A. (2023) “Genotoxicity and cytotoxicity of textile production effluents, before and after Bacillus subitilis bioremediation, in Astyanax lacustris (Pisces, Characidae). Astyanax lacustris (Pisces, Characidae) 886, 503588. doi:10.1016/j.mrgentox.2023.503588
Da Silva, L. I., Pereira, M. C., de Carvalho, A. M. X., Buttrós, V. H., Pasqual, M., and Dória, J. (2023). Phosphorus-solubilizing microorganisms: a key to sustainable agriculture. Agriculture 13, 462. doi:10.3390/agriculture13020462
Dave, P. N., and Chopda, L. V. (2014). Application of iron oxide nanomaterials for the removal of heavy metals. J. Nanotechnol. 2014, 1–14. doi:10.1155/2014/398569
Dawwam, G. E., Abdelfattah, N. M., Abdel-Monem, M. O., Jahin, H. S., Omer, A. M., Abou-Taleb, K. A., et al. (2023). An immobilized biosorbent from Paenibacillus dendritiformis dead cells and polyethersulfone for the sustainable bioremediation of lead from wastewater. Sci. Rep. 13 (1), 891. doi:10.1038/s41598-023-27796-w
Dehghani, M. H., Kamalian, S., Shayeghi, M., Yousefi, M., Heidarinejad, Z., Agarwal, S., et al. (2019). High-performance removal of diazinon pesticide from water using multi-walled carbon nanotubes. Microchemical Journal 145, 486–491.
Dell'Anno, F., Joaquim van Zyl, L., Trindade, M., Buschi, E., Cannavacciuolo, A., Pepi, M., et al. (2023). Microbiome enrichment from contaminated marine sediments unveils novel bacterial strains for petroleum hydrocarbon and heavy metal bioremediation. Environ. Pollut. 317, 120772. doi:10.1016/j.envpol.2022.120772
Dell'Anno, F., Rastelli, E., Buschi, E., Barone, G., Beolchini, F., and Dell'Anno, A. (2022). Fungi can Be more effective than bacteria for the bioremediation of marine sediments highly contaminated with heavy metals. Microorganisms 10 (5), 993. doi:10.3390/microorganisms10050993
Del Prado-Audelo, M. L., García Kerdan, I., Escutia-Guadarrama, L., Reyna-González, J. M., Magaña, J. J., and Leyva-Gómez, G. (2021). Nanoremediation: nanomaterials and nanotechnologies for environmental cleanup. Front. Environ. Sci. 9. doi:10.3389/fenvs.2021.793765
de Moura Dickel, J. D., Carvalho, J. K., Silveira, M. A. D., Menegotto Dos Santos, P., Rodrigues, M. L. F., Fagundes-Klen, M. R., et al. (2022). Aspergillus sclerotiorum lipolytic activity and its application in bioremediation of high-fat dairy wastewater environments. Environ. Sci. Pollut. Res. Int. 30, 35517–35527. doi:10.1007/s11356-022-24669-z
Derakhshan Nejad, Z., Jung, M. C., and Kim, K. H. (2018). Remediation of soils contaminated with heavy metals with an emphasis on immobilization technology. Environ. Geochem. Health 40 (3), 927–953. doi:10.1007/s10653-017-9964-z
Devi, N. L. (2020). Persistent organic pollutants (POPs): environmental risks, toxicological effects, and bioremediation for environmental safety and challenges for future research. Singapore: Bioremediation of Industrial Waste for Environmental Safety, 53–76.
Dhaliwal, S. S., Singh, J., Taneja, P. K., and Mandal, A. (2020). Remediation techniques for removal of heavy metals from the soil contaminated through different sources: a review. Environ. Sci. Pollut. Res. 27 (2), 1319–1333. doi:10.1007/s11356-019-06967-1
Din, M., Nelofer, R., Salman, M., Khan, F. H., Khan, A., Ahmad, M., et al. (2019). Production of nitrogen fixing Azotobacter (SR-4) and phosphorus solubilizing Aspergillus Niger and their evaluation on Lagenaria siceraria and Abelmoschus esculentus. Biotechnol. Rep. 22, e00323. doi:10.1016/j.btre.2019.e00323
Ding, C., Chen, J., Zhu, F., Chai, L., Lin, Z., Zhang, K., et al. (2022). Biological toxicity of heavy metal(loid)s in natural environments: from microbes to humans. Front. Environ. Sci. 10. doi:10.3389/fenvs.2022.920957
Dubey, S., Chen, C. W., Haldar, D., Tambat, V. S., Kumar, P., Tiwari, A., et al. (2023). Advancement in algal bioremediation for organic, inorganic, and emerging pollutants. Environ. Pollut. 317, 120840. doi:10.1016/j.envpol.2022.120840
Dwivedi, S. (2012). Bioremediation of heavy metal by algae: current and future perspective. J. Adv. Lab. Res. Biol. 3 (3), 195–199.
El-Khoury, R., Rak, M., Bénit, P., Jacobs, H. T., and Rustin, P. (2022). Cyanide resistant respiration and the alternative oxidase pathway: a journey from plants to mammals. Biochimica Biophysica Acta - Bioenergetics 1863 (6), 148567. doi:10.1016/j.bbabio.2022.148567
Epelde, L., Burges, A., Mijangos, I., and Garbisu, C. (2014). Microbial properties and attributes of ecological relevance for soil quality monitoring during a chemical stabilization field study. Appl. Soil Ecol. 75, 1–12. doi:10.1016/j.apsoil.2013.10.003
Etemadi, M., Samadi, S., Yazd, S. S., Jafari, P., Yousefi, N., and Aliabadi, M. (2017). Selective adsorption of Cr(VI) ions from aqueous solutions using Cr6+-imprinted Pebax/chitosan/GO/APTES nanofibrous adsorbent. Int. J. Biol. Macromol. 95, 725–733. doi:10.1016/j.ijbiomac.2016.11.117
Eyankware, M. O., and Obasi, P. N. (2021). A holistic review of heavy metals in water and soil in Ebonyi SE. Nigeria; with emphasis on its effects on human, aquatic organisms and plants. World News Nat. Sci. 38, 1–19.
Fato, F. P., Li, D. W., Zhao, L. J., Qiu, K., and Long, Y. T. (2019). Simultaneous removal of multiple heavy metal ions from river water using ultrafine mesoporous magnetite nanoparticles. ACS Omega 4 (4), 7543–7549. doi:10.1021/acsomega.9b00731
Fei, L., Bilal, M., Qamar, S. A., Imran, H. M., Riasat, A., Jahangeer, M., et al. (2022). Nano-remediation technologies for the sustainable mitigation of persistent organic pollutants. Environ. Res. 211, 113060. doi:10.1016/j.envres.2022.113060
Feng, J. R., Deng, Q. X., Han, S. K., and Ni, H. G. (2023). Use of nanoparticle-coated bacteria for the bioremediation of organic pollution: a mini review. Chemosphere 313, 137391. doi:10.1016/j.chemosphere.2022.137391
Feng, L., Yan, H., Dai, C., Xu, W., Gu, F., Zhang, F., et al. (2020). The systematic exploration of cadmium-accumulation characteristics of maize kernel in acidic soil with different pollution levels in China. Sci. Total Environ. 729, 138972. doi:10.1016/j.scitotenv.2020.138972
Fulekar, M. H., Sharma, J., and Tendulkar, A. (2012). Bioremediation of heavy metals using biostimulation in laboratory bioreactor. Environ. Monit. Assess. 184 (12), 7299–7307. doi:10.1007/s10661-011-2499-3
Funtikova, T. V., Akhmetov, L. I., Puntus, I. F., Mikhailov, P. A., Appazov, N. O., Narmanova, R. A., et al. (2023). Bioremediation of oil-contaminated soil of the republic of Kazakhstan using a new biopreparation. Microorganisms 11 (2), 522. doi:10.3390/microorganisms11020522
Furnholm, T., Rehan, M., Wishart, J., and Tisa, L. S. J. M. (2017). Pb2+ tolerance by Frankia sp. strain EAN1pec involves surface-binding. , 163(4), 472–487. doi:10.1099/mic.0.000439
Gabr, R. M., Hassan, S. H. A., and Shoreit, A. A. M. (2008). Biosorption of lead and nickel by living and non-living cells of Pseudomonas aeruginosa ASU 6a. Int. Biodeterior. Biodegrad. 62 (2), 195–203. doi:10.1016/j.ibiod.2008.01.008
Gaur, N., Flora, G., Yadav, M., and Tiwari, A. (2014). A review with recent advancements on bioremediation-based abolition of heavy metals. Environ. Sci. Process Impacts 16 (2), 180–193. doi:10.1039/c3em00491k
Gavrilescu, M. (2022). Enhancing phytoremediation of soils polluted with heavy metals. Curr. Opin. Biotechnol. 74, 21–31. doi:10.1016/j.copbio.2021.10.024
Gentry, T. J., Rensing, C., and Pepper, I. L. (2004). New approaches for bioaugmentation as a remediation technology. Crit. Rev. Environ. Sci. Technol. 34 (5), 447–494. doi:10.1080/10643380490452362
Goher, M. E., El-Monem, A. M. A., Abdel-Satar, A. M., Ali, M. H., Hussian, A. E. M., Napiórkowska-Krzebietke, A., et al. (2016). Biosorption of some toxic metals from aqueous solution using non-living algal cells of Chlorella vulgaris. J. Elem. 21 (3), 703–714. doi:10.5601/jelem.2015.20.4.1037
Gong, Y., Zhao, D., and Wang, Q. (2018). An overview of field-scale studies on remediation of soil contaminated with heavy metals and metalloids: technical progress over the last decade. Water Res. 147, 440–460. doi:10.1016/j.watres.2018.10.024
González-Chávez, M. C., Carrillo-González, R., Wright, S. F., and Nichols, K. A. (2004). The role of glomalin, a protein produced by arbuscular mycorrhizal fungi, in sequestering potentially toxic elements. Environ. Pollut. 130 (3), 317–323. doi:10.1016/j.envpol.2004.01.004
Goswami, R. K., Agrawal, K., Shah, M. P., and Verma, P. (2022). Bioremediation of heavy metals from wastewater: a current perspective on microalgae-based future. Lett. Appl. Microbiol. 75 (4), 701–717. doi:10.1111/lam.13564
Grimm, F. A., Klaren, W. D., Li, X., Lehmler, H. J., Karmakar, M., Robertson, L. W., et al. (2020). Cardiovascular effects of polychlorinated biphenyls and their major metabolites. Environ. Health Perspect. 128 (7), 77008–77013. doi:10.1289/EHP7030
Gulzar, A. B. M., and Mazumder, P. B. (2022). Helping plants to deal with heavy metal stress: the role of nanotechnology and plant growth promoting rhizobacteria in the process of phytoremediation. Environ. Sci. Pollut. Res. 29 (27), 40319–40341. doi:10.1007/s11356-022-19756-0
Guo, W., Pan, B., Sakkiah, S., Yavas, G., Ge, W., Zou, W., et al. (2019). Persistent organic pollutants in food: contamination sources, health effects and detection methods. Int. J. Environ. Res. Public Health 16 (22), 4361. doi:10.3390/ijerph16224361
Gururani, M. A., Upadhyaya, C. P., Baskar, V., Venkatesh, J., Nookaraju, A., and Park, S. W. (2013). Plant growth-promoting rhizobacteria enhance abiotic stress tolerance in Solanum tuberosum through inducing changes in the expression of ROS-scavenging enzymes and improved photosynthetic performance. J. Plant Growth Regul. 32 (2), 245–258. doi:10.1007/s00344-012-9292-6
Hananingtyas, I., Nuryanty, C. D., Karlinasari, L., Alikodra, H. S., Jayanegara, A., and Sumantri, A. (2022). The effects of heavy metal exposure in agriculture soil on chlorophyll content of agriculture crops: a meta-analysis approach. IOP Conf. Ser. Earth Environ. Sci. 951, 012044. Paper presented at the. doi:10.1088/1755-1315/951/1/012044
Hanif, A., Bhatti, H. N., and Hanif, M. A. (2015). Removal of zirconium from aqueous solution by Ganoderma lucidum: biosorption and bioremediation studies. Desalination Water Treat. 53 (1), 195–205. doi:10.1080/19443994.2013.837005
Hemlata, , Meena, P. R., Singh, A. P., and Tejavath, K. K. (2020). Biosynthesis of silver nanoparticles using cucumis prophetarum aqueous leaf extract and their antibacterial and antiproliferative activity against cancer cell lines. ACS Omega 5 (10), 5520–5528. doi:10.1021/acsomega.0c00155
Hlihor, R. M., Rosca, M., Hagiu-Zaleschi, L., Simion, I. M., Daraban, G. M., and Stoleru, V. (2022). Medicinal plant growth in heavy metals contaminated soils: responses to metal stress and induced risks to human health. Toxics 10 (9), 499. doi:10.3390/toxics10090499
Hota, S., Sharma, G. K., Subrahmanyam, G., Kumar, A., Shabnam, A. A., Baruah, P., et al. (2021). Fungal communities for bioremediation of contaminated soil for sustainable environments. Recent Trends Mycol. Res. Environ. Industrial Perspective 2, 27–42. doi:10.1007/978-3-030-68260-6_2
Houri, T., Khairallah, Y., Zahab, A. A., Osta, B., Romanos, D., and Haddad, G. (2020). Heavy metals accumulation effects on the photosynthetic performance of geophytes in mediterranean reserve. J. King Saud Univ. - Sci. 32 (1), 874–880. doi:10.1016/j.jksus.2019.04.005
Hu, X., Wang, J., Lv, Y., Liu, X., Zhong, J., Cui, X., et al. (2021). Effects of heavy metals/metalloids and soil properties on microbial communities in farmland in the vicinity of a metals smelter. Front. Microbiol. 12, 707786. doi:10.3389/fmicb.2021.707786
Huang, C. C., Liang, C. M., Yang, T. I., Chen, J. L., and Wang, W. K. (2021). Shift of bacterial communities in heavy metal-contaminated agricultural land during a remediation process. PLoS ONE 16 (7 July), e0255137. doi:10.1371/journal.pone.0255137
Huang, F., Zhou, H., Gu, J., Liu, C., Yang, W., Liao, B., et al. (2020). Differences in absorption of cadmium and lead among fourteen sweet potato cultivars and health risk assessment. Ecotoxicol. Environ. Saf. 203, 111012. doi:10.1016/j.ecoenv.2020.111012
Huang, L., Ni, J., Zhong, C., Xu, P., Dai, J., and Tang, H. (2022). Establishment of a salt-induced bioremediation platform from marine Vibrio natriegens. Commun. Biol. 5 (1), 1352. doi:10.1038/s42003-022-04319-3
Huang, L., Wang, W., Zanaroli, G., Xu, P., and Tang, H. (2021). Hexabromocyclododecanes are dehalogenated by CYP168A1 from Pseudomonas aeruginosa strain HS9. Appl. Environ. Microbiol. 87 (17), 00826211–e82711. doi:10.1128/AEM.00826-21
Hube, S., Eskafi, M., Hrafnkelsdóttir, K. F., Bjarnadóttir, B., Bjarnadóttir, M. Á., Axelsdóttir, S., et al. (2020). Direct membrane filtration for wastewater treatment and resource recovery: a review. Sci. Total Environ. 710, 136375. doi:10.1016/j.scitotenv.2019.136375
Husain, R., Vikram, N., Yadav, G., Kumar, D., Pandey, S., Patel, M., et al. (2022). “Microbial bioremediation of heavy metals by Marine bacteria,” in Development in wastewater treatment research and processes: microbial degradation of xenobiotics through bacterial and fungal approach, 177–203.
Imron, M. F., Kurniawan, S. B., and Abdullah, S. R. S. (2021). Resistance of bacteria isolated from leachate to heavy metals and the removal of Hg by Pseudomonas aeruginosa strain FZ-2 at different salinity levels in a batch biosorption system. Sustain. Environ. Res. 31 (1), 14. doi:10.1186/s42834-021-00088-6
Izanloo, M., Mehrpooya, M., and Delpisheh, M. (2021). Integrated thermochemical Mg-Cl-Na hydrogen production cycle, carbon dioxide capture, ammonia production, and methanation. International Journal of Energy Research 45 (7), 10719–10737.
Islam, M. N., Taki, G., Nguyen, X. P., Jo, Y. T., Kim, J., and Park, J. H. (2017). Heavy metal stabilization in contaminated soil by treatment with calcined cockle shell. Environ. Sci. Pollut. Res. 24 (8), 7177–7183. doi:10.1007/s11356-016-8330-5
Jabbar, N. M., Alardhi, S. M., Mohammed, A. K., Salih, I. K., and Albayati, T. M. (2022). Challenges in the implementation of bioremediation processes in petroleum-contaminated soils: a review. Environ. Nanotechnol. Monit. Manag. 18, 100694. doi:10.1016/j.enmm.2022.100694
Jia, W., Li, N., Yang, T., Dai, W., Jiang, J., Chen, K., et al. (2021). Bioaugmentation of atrazine-contaminated soil with paenarthrobacter sp. strain AT-5 and its effect on the soil microbiome. Front. Microbiol. 12, 771463. doi:10.3389/fmicb.2021.771463
Jia, Y., Liao, Z., Chew, H., Wang, L., Lin, B., Chen, C., et al. (2020). Effect of Pennisetum giganteum z.x. lin mixed nitrogen-fixing bacterial fertilizer on the growth, quality, soil fertility and bacterial community of pakchoi (Brassica chinensis L.). PLoS ONE 15, e0228709. doi:10.1371/journal.pone.0228709
Jia, Z., Li, S., and Wang, L. (2018). Assessment of soil heavy metals for eco-environment and human health in a rapidly urbanization area of the upper Yangtze Basin. Sci. Rep. 8 (1), 3256. doi:10.1038/s41598-018-21569-6
Kadam, A., Saratale, R. G., Shinde, S., Yang, J., Hwang, K., Mistry, B., et al. (2019). Adsorptive remediation of cobalt oxide nanoparticles by magnetized α-cellulose fibers from waste paper biomass. Bioresour. Technol. 273, 386–393. doi:10.1016/j.biortech.2018.11.041
Kalaivanan, D., and Ganeshamurthy, A. N. (2016). “Mechanisms of heavy metal toxicity in plants,” in Abiotic stress physiology of horticultural crops, 85–102.
Kamika, I., and Momba, M. N. (2013). Assessing the resistance and bioremediation ability of selected bacterial and protozoan species to heavy metals in metal-rich industrial wastewater. BMC Microbiol. 13, 28. doi:10.1186/1471-2180-13-28
Karimi-Shamsabadi, M., and Nezamzadeh-Ejhieh, A. (2016). Comparative study on the increased photoactivity of coupled and supported manganese-silver oxides onto a natural zeolite nano-particles. Journal of Molecular Catalysis A: Chemical 418, 103–114.
Kashem, A. H. M., Das, P., AbdulQuadir, M., Khan, S., Thaher, M. I., Alghasal, G., et al. (2023). Microalgal bioremediation of brackish aquaculture wastewater. Sci. Total Environ. 873, 162384. doi:10.1016/j.scitotenv.2023.162384
Kaushik, S., Alatawi, A., Djiwanti, S. R., Pande, A., Skotti, E., and Soni, V. (2021). Potential of extremophiles for bioremediation. Microb. Rejuvenation Polluted Environ. 1, 293–328. doi:10.1007/978-981-15-7447-4_12
Khan, A. A., Jilani, G., Akhtar, M. S., Naqvi, S. M. S., and Rasheed, M. (2009). Phosphorus solubilizing bacteria: occurrence, mechanisms and their role in crop production. J. Agric. Biol. Sci. 1 (1), 48–58.
Khan, A. L., Waqas, M., Hussain, J., Al-Harrasi, A., and Lee, I. J. (2014). Fungal endophyte Penicillium janthinellum LK5 can reduce cadmium toxicity in Solanum lycopersicum (Sitiens and Rhe). Biol. Fertil. Soils 50 (1), 75–85. doi:10.1007/s00374-013-0833-3
Khan, S. H., Pathak, B., and Fulekar, M. H. (2018). Synthesis, characterization and photocatalytic degradation of chlorpyrifos by novel Fe: ZnO nanocomposite material. Nanotechnology for Environmental Engineering 3, 1–14.
Kim, B. K., Park, G. Y., Jeon, E. K., Jung, J. M., Jung, H. B., Ko, S. H., et al. (2013). Field application of in situ electrokinetic remediation for as-Cu-and Pb-contaminated paddy soil. Water, Air, Soil Pollut. 224 (9), 1698. doi:10.1007/s11270-013-1698-7
Kim, H. W., Seok, Y. S., Cho, T. J., and Rhee, M. S. (2020). Risk factors influencing contamination of customized cosmetics made on-the-spot: evidence from the national pilot project for public health. Sci. Rep. 10 (1), 1561. doi:10.1038/s41598-020-57978-9
Krithika, S., and Balachandar, D. (2016). Expression of zinc transporter genes in rice as influenced by zinc-solubilizing enterobacter cloacae strain ZSB14. Front. Plant Sci. 7 (APR2016), 446. doi:10.3389/fpls.2016.00446
Kuan, K. B., Othman, R., Rahim, K. A., and Shamsuddin, Z. H. (2016). Plant growth-promoting rhizobacteria inoculation to enhance vegetative growth, nitrogen fixation and nitrogen remobilisation of maize under greenhouse conditions. PLoS ONE 11 (3), e0152478. doi:10.1371/journal.pone.0152478
Kumar, H., Ishtiyaq, S., Varun, M., Favas, P. J. C., Ogunkunle, C. O., and Paul, M. S. (2022). Bioremediation: plants and microbes for restoration of heavy metal contaminated soils. Bioenergy Crops 3, 37–70. doi:10.1201/9781003043522-3
Kumar, H., Sinha, S. K., Goud, V. V., and Das, S. (2019). Removal of Cr(VI) by magnetic iron oxide nanoparticles synthesized from extracellular polymeric substances of chromium resistant acid-tolerant bacterium Lysinibacillus sphaericus RTA-01. J. Environ. Health Sci. Eng. 17 (2), 1001–1016. doi:10.1007/s40201-019-00415-5
Kumar, K., and Singh, D. (2023). Toxicity and bioremediation of the lead: a critical review. Int. J. Environ. Health Res. 34, 1879–1909. doi:10.1080/09603123.2023.2165047
Kumar, S., and Saxena, S. (2019). Arbuscular mycorrhizal fungi (AMF) from heavy metal-contaminated soils: molecular approach and application in phytoremediation. Biofertilizers Sustain. Agric. Environ., 489–500. doi:10.1007/978-3-030-18933-4_22
Kumar, V., and Dwivedi, S. K. (2021). Bioremediation mechanism and potential of copper by actively growing fungus Trichoderma lixii CR700 isolated from electroplating wastewater. J. Environ. Manag. 277, 111370. doi:10.1016/j.jenvman.2020.111370
Kumar, V., Kumar, H., Vishal, V., and Lal, S. (2023). Studies on the morphology, phylogeny, and bioremediation potential of Penicillium citrinum and Paecilomyces variotii (Eurotiales) from oil-contaminated areas. Arch. Microbiol. 205 (1), 50. doi:10.1007/s00203-022-03383-x
Kurniawan, S. B., Ramli, N. N., Said, N. S. M., Alias, J., Imron, M. F., Abdullah, S. R. S., et al. (2022). Practical limitations of bioaugmentation in treating heavy metal contaminated soil and role of plant growth promoting bacteria in phytoremediation as a promising alternative approach. Heliyon 8 (4), e08995. doi:10.1016/j.heliyon.2022.e08995
Lambert, M., Leven, B. A., and Green, R. M. (2000). New methods of cleaning up heavy metal in soils and water. Environ. Sci. Technol. Briefs Citizens, 1–3.
Landa-faz, A., González-orenga, S., Boscaiu, M., Rodríguez-vázquez, R., and Vicente, O. (2021). Effect of the pesticide endosulfan and two different biostimulants on the stress responses of phaseolus leptostachyus plants grown in a saline soil. Agronomy 11 (6), 1208. doi:10.3390/agronomy11061208
Lee, D. W., Lee, H., Kwon, B. O., Khim, J. S., Yim, U. H., Kim, B. S., et al. (2018). Biosurfactant-assisted bioremediation of crude oil by indigenous bacteria isolated from Taean beach sediment. Environ. Pollut. 241, 254–264. doi:10.1016/j.envpol.2018.05.070
Lee, J., Kim, J., Ahmed, S. R., Zhou, H., Kim, J. M., and Lee, J. (2014). Plasmon-induced photoluminescence immunoassay for tuberculosis monitoring using gold-nanoparticle-decorated graphene. ACS Appl. Mater. Interfaces 6 (23), 21380–21388. doi:10.1021/am506389m
Leon-Vaz, A., Leon, R., Giraldez, I., Vega, J. M., and Vigara, J. (2021). Impact of heavy metals in the microalga Chlorella sorokiniana and assessment of its potential use in cadmium bioremediation. Aquat. Toxicol. 239, 105941. doi:10.1016/j.aquatox.2021.105941
Li, D., Zheng, X., Lin, L., An, Q., Jiao, Y., Li, Q., et al. (2022). Remediation of soils co-contaminated with cadmium and dichlorodiphenyltrichloroethanes by king grass associated with Piriformospora indica: insights into the regulation of root excretion and reshaping of rhizosphere microbial community structure. J. Hazard. Mater. 422, 126936. doi:10.1016/j.jhazmat.2021.126936
Li, Q., Zhong, H., and Cao, Y. (2020). Effective extraction and recovery of rare earth elements (REEs) in contaminated soils using a reusable biosurfactant. Chemosphere 256, 127070. doi:10.1016/j.chemosphere.2020.127070
Li, W., Chen, Y., and Wang, T. (2021). Cadmium biosorption by lactic acid bacteria Weissella viridescens ZY-6. Food control. 123, 107747. doi:10.1016/j.foodcont.2020.107747
Li, Z., He, Y., Sonne, C., Lam, S. S., Kirkham, M. B., Bolan, N., et al. (2023). A strategy for bioremediation of nuclear contaminants in the environment. Environ. Pollut. 319, 120964. doi:10.1016/j.envpol.2022.120964
Liaqat, I., Muhammad, N., Ara, C., Hanif, U., Andleeb, S., Arshad, M., et al. (2023). Bioremediation of heavy metals polluted environment and decolourization of black liquor using microbial biofilms. Mol. Biol. Rep. 50, 3985–3997. doi:10.1007/s11033-023-08334-3
Lin, J., Jiang, W., and Liu, D. (2003). Accumulation of copper by roots, hypocotyls, cotyledons and leaves of sunflower (Helianthus annuus L.). Bioresour. Technol. 86 (2), 151–155. doi:10.1016/S0960-8524(02)00152-9
Liu, H., Guo, S., Jiao, K., Hou, J., Xie, H., and Xu, H. (2015). Bioremediation of soils co-contaminated with heavy metals and 2,4,5-trichlorophenol by fruiting body of Clitocybe maxima. J. Hazard Mater 294, 121–127. doi:10.1016/j.jhazmat.2015.04.004
Liu, J., Qi, W., Li, Q., Wang, S.-G., Song, C., and Yuan, X.-z. (2020). Exogenous phosphorus-solubilizing bacteria changed the rhizosphere microbial community indirectly. 3 Biotech. 10, 164–211. doi:10.1007/s13205-020-2099-4
Liu, S., Zheng, Y., Ma, Y., Sarwar, A., Zhao, X., Luo, T., et al. (2019a). Evaluation and proteomic analysis of lead adsorption by lactic acid bacteria. Int. J. Mol. Sci. 20 (22), 5540. doi:10.3390/ijms20225540
Liu, S. H., Zeng, G. M., Niu, Q. Y., Liu, Y., Zhou, L., Jiang, L. H., et al. (2017). Bioremediation mechanisms of combined pollution of PAHs and heavy metals by bacteria and fungi: a mini review. Bioresour. Technol. 224, 25–33. doi:10.1016/j.biortech.2016.11.095
Liu, W., Zuo, Q., Zhao, C., Wang, S., Shi, Y., Liang, S., et al. (2018). Effects of Bacillus subtilis and nanohydroxyapatite on the metal accumulation and microbial diversity of rapeseed (Brassica campestris L.) for the remediation of cadmium-contaminated soil. Environ. Sci. Pollut. Res. 25 (25), 25217–25226. doi:10.1007/s11356-018-2616-8
Liu, Z., Lu, B., Xiao, H., Liu, D., Li, X., Wang, L. A., et al. (2019b). Effect of mixed solutions of heavy metal eluents on soil fertility and microorganisms. Environ. Pollut. 254, 112968. doi:10.1016/j.envpol.2019.112968
Lu, M., Jiao, S., Gao, E., Song, X., Li, Z., Hao, X., et al. (2017). Transcriptome response to heavy metals in sinorhizobium meliloti CCNWSX0020 reveals new metal resistance determinants that also promote bioremediation by Medicago lupulina in metal-contaminated soil. Appl. Environ. Microbiol. 83 (20), e01244. doi:10.1128/AEM.01244-17
Lu, P., Li, Q., Liu, H., Feng, Z., Yan, X., Hong, Q., et al. (2013). Biodegradation of chlorpyrifos and 3,5,6-trichloro-2-pyridinol by Cupriavidus sp. DT-1. Bioresour. Technol. 127, 337–342. doi:10.1016/j.biortech.2012.09.116
Luo, H., Wang, Q., Liu, Z., Wang, S., Long, A., and Yang, Y. (2020a). Potential bioremediation effects of seaweed Gracilaria lemaneiformis on heavy metals in coastal sediment from a typical mariculture zone. Chemosphere 245, 125636. doi:10.1016/j.chemosphere.2019.125636
Luo, J., Deng, J., Cui, L., Chang, P., Dai, X., Yang, C., et al. (2020b). The potential assessment of green alga Chlamydomonas reinhardtii CC-503 in the biodegradation of benz(a)anthracene and the related mechanism analysis. Chemosphere 249, 126097. doi:10.1016/j.chemosphere.2020.126097
Lwin, H. M., Zhan, W., Song, S., Jia, F., and Zhou, J. (2019). Visible-light photocatalytic degradation pathway of tetracycline hydrochloride with cubic structured ZnO/SnO2 heterojunction nanocatalyst. Chem. Phys. Lett. 736, 136806. doi:10.1016/j.cplett.2019.136806
Ma, X. K., Li, T. T., Fam, H., Charles Peterson, E., Zhao, W. W., Guo, W., et al. (2018). The influence of heavy metals on the bioremediation of polycyclic aromatic hydrocarbons in aquatic system by a bacterial-fungal consortium. Environ. Technol. 39 (16), 2128–2137. doi:10.1080/09593330.2017.1351492
Mahdavi, V., Taghadosi, F., Dashtestani, F., Bahadorikhalili, S., Farimani, M. M., Farimani, L., et al. (2021). Aminoguanidine modified magnetic graphene oxide as a robust nanoadsorbent for efficient removal and extraction of chlorpyrifos residue from water. Journal of Environmental Chemical Engineering 9 (5), 106117
Malik, S., Kishore, S., Shah, M. P., and Kumar, S. A. (2022). A comprehensive review on nanobiotechnology for bioremediation of heavy metals from wastewater. J. Basic Microbiol. 62 (3-4), 361–375. doi:10.1002/jobm.202100555
Manobala, T., Shukla, S. K., Rao, T. S., and Kumar, M. D. (2021). Kinetic modelling of the uranium biosorption by Deinococcus radiodurans biofilm. Chemosphere 269, 128722. doi:10.1016/j.chemosphere.2020.128722
Manoj, S. R., Karthik, C., Kadirvelu, K., Arulselvi, P. I., Shanmugasundaram, T., Bruno, B., et al. (2020). Understanding the molecular mechanisms for the enhanced phytoremediation of heavy metals through plant growth promoting rhizobacteria: a review. J. Environ. Manag. 254, 109779. doi:10.1016/j.jenvman.2019.109779
Manzoor, N., Ahmed, T., Noman, M., Shahid, M., Nazir, M. M., Ali, L., et al. (2021). Iron oxide nanoparticles ameliorated the cadmium and salinity stresses in wheat plants, facilitating photosynthetic pigments and restricting cadmium uptake. Sci. Total Environ. 769, 145221. doi:10.1016/j.scitotenv.2021.145221
Mao, C., Song, Y., Chen, L., Ji, J., Li, J., Yuan, X., et al. (2019). Human health risks of heavy metals in paddy rice based on transfer characteristics of heavy metals from soil to rice. Catena 175, 339–348. doi:10.1016/j.catena.2018.12.029
Mao, J., and Guan, W. (2016). Fungal degradation of polycyclic aromatic hydrocarbons (PAHs) by Scopulariopsis brevicaulis and its application in bioremediation of PAH-contaminated soil. Acta Agric. Scand. Sect. B Soil Plant Sci. 66 (5), 399–405. doi:10.1080/09064710.2015.1137629
Masotti, F., Garavaglia, B. S., Gottig, N., and Ottado, J. (2023). Bioremediation of the herbicide glyphosate in polluted soils by plant-associated microbes. Curr. Opin. Microbiol. 73, 102290. doi:10.1016/j.mib.2023.102290
Mathivanan, K., Chandirika, J. U., Mathimani, T., Rajaram, R., Annadurai, G., and Yin, H. (2021a). Production and functionality of exopolysaccharides in bacteria exposed to a toxic metal environment. Ecotoxicol. Environ. Saf. 208, 111567. doi:10.1016/j.ecoenv.2020.111567
Mathivanan, K., Chandirika, J. U., Vinothkanna, A., Yin, H., Liu, X., and Meng, D. (2021b). Bacterial adaptive strategies to cope with metal toxicity in the contaminated environment – a review. Ecotoxicol. Environ. Saf. 226, 112863. doi:10.1016/j.ecoenv.2021.112863
Mazumder, A., Bhattacharya, S., and Bhattacharjee, C. (2020). Role of nano-photocatalysis in heavy metal detoxification. Nanophotocatalysis Environ. Appl., 1–33. doi:10.1007/978-3-030-12619-3_1
McGrath, S. P., Zhao, F. J., and Lombi, E. (2001). Plant and rhizosphere processes involved in phytoremediation of metal-contaminated soils. Plant Soil 232 (1-2), 207–214. doi:10.1023/A:1010358708525
Mitra, S., Chakraborty, A. J., Tareq, A. M., Emran, T. B., Nainu, F., Khusro, A., et al. (2022). Impact of heavy metals on the environment and human health: novel therapeutic insights to counter the toxicity. J. King Saud Univ. - Sci. 34 (3), 101865. doi:10.1016/j.jksus.2022.101865
Mohammadi, A., and Veisi, P. (2018). High adsorption performance of β-cyclodextrin-functionalized multi-walled carbon nanotubes for the removal of organic dyes from water and industrial wastewater. Journal of Environmental Chemical Engineering 6 (4), 4634–4643.
Monachese, M., Burton, J. P., and Reid, G. (2012). Bioremediation and tolerance of humans to heavy metals through microbial processes: a potential role for probiotics? Appl. Environ. Microbiol. 78 (18), 6397–6404. doi:10.1128/AEM.01665-12
More, T. T., Yadav, J. S. S., Yan, S., Tyagi, R. D., and Surampalli, R. Y. (2014). Extracellular polymeric substances of bacteria and their potential environmental applications. J. Environ. Manag. 144, 1–25. doi:10.1016/j.jenvman.2014.05.010
Mustapha, M. U., and Halimoon, N. (2022). The effect of heavy metals on biodegradation of carbofuran by microbial strain enriched from agricultural areas. Afr. J. Biol. Sci. 18, 71–77. doi:10.21608/ajbs.2022.251286
Nagashetti, V., Mahadevaraju, G. K., Muralidhar, T. S., Javed, A., Trivedi, D., and Bhusal, K. P. (2013). Biosorption of heavy metals from soil by Pseudomonas aeruginosa. Int. J. Innovative Technol. Explor. Eng. 2 (6), 22–24.
Nandini, R., Amuthavallinayaki, M., Sangameswaran, R., and Arthe, R. (2023). A review on nano enhanced bioremediation of toxic contaminants in the environment. Int. J. Mod. Dev. Eng. Sci. 2 (6), 28–34.
Nath, D., Maurya, B. R., and Meena, V. S. (2017). Documentation of five potassium-and phosphorus-solubilizing bacteria for their K and P-solubilization ability from various minerals. Biocatal. Agric. Biotechnol. 10, 174–181. doi:10.1016/j.bcab.2017.03.007
Nawaz, H., Anwar-ul-Haq, M., Akhtar, J., and Arfan, M. (2021). Cadmium, chromium, nickel and nitrate accumulation in wheat (Triticum aestivum L.) using wastewater irrigation and health risks assessment. Ecotoxicol. Environ. Saf. 208, 111685. doi:10.1016/j.ecoenv.2020.111685
Nedjimi, B. (2021). Phytoremediation: a sustainable environmental technology for heavy metals decontamination. SN Appl. Sci. 3 (3), 286. doi:10.1007/s42452-021-04301-4
Neeli, S. T., Ramsurn, H., Ng, C. Y., Wang, Y., and Lu, J. (2020). Removal of Cr (VI), as (V), Cu (II), and Pb (II) using cellulose biochar supported iron nanoparticles: a kinetic and mechanistic study. J. Environ. Chem. Eng. 8 (5), 103886. doi:10.1016/j.jece.2020.103886
Noman, M., Ahmed, T., Hussain, S., Niazi, M. B. K., Shahid, M., and Song, F. (2020). Biogenic copper nanoparticles synthesized by using a copper-resistant strain Shigella flexneri SNT22 reduced the translocation of cadmium from soil to wheat plants. J. Hazard. Mater. 398, 123175. doi:10.1016/j.jhazmat.2020.123175
Oatley-Radcliffe, D. L., and Barron, A. R. (2022). “Summary of field trial results of the treatment of contaminated water using non-fouling super hydrophilic functionalized ceramic membranes,” in Sustainable Energy-Water-Environment Nexus in Deserts: Proceeding of the First International Conference on Sustainable Energy-Water-Environment Nexus in Desert Climates Cham: Springer International Publishing, 22948121–129.
O'Connell, D. W., Birkinshaw, C., and O'Dwyer, T. F. (2008). Heavy metal adsorbents prepared from the modification of cellulose: a review. Bioresour. Technol. 99 (15), 6709–6724. doi:10.1016/j.biortech.2008.01.036
Ojuederie, O. B., and Babalola, O. O. (2017). Microbial and plant-assisted bioremediation of heavy metal polluted environments: a review. Int. J. Environ. Res. Public Health 14 (12), 1504. doi:10.3390/ijerph14121504
Oladoye, P. O., Olowe, O. M., and Asemoloye, M. D. (2022). Phytoremediation technology and food security impacts of heavy metal contaminated soils: a review of literature. Chemosphere 288, 132555. doi:10.1016/j.chemosphere.2021.132555
O'Neil, R. A., Holmes, D. E., Coppi, M. V., Adams, L. A., Larrahondo, M. J., Ward, J. E., et al. (2008). Gene transcript analysis of assimilatory iron limitation in Geobacteraceae during groundwater bioremediation. Environ. Microbiol. 10 (5), 1218–1230. doi:10.1111/j.1462-2920.2007.01537.x
Ordookhani, K., Khavazi, K., Moezzi, A., and Rejali, F. (2010). Influence of PGPR and AMF on antioxidant activity, lycopene and potassium contents in tomato. Afr. J. Agric. Res. 5 (10), 1108–1116.
Pachaiappan, R., Rajendran, S., Show, P. L., Manavalan, K., and Naushad, M. (2021). Metal/metal oxide nanocomposites for bactericidal effect: a review. Chemosphere 272, 128607. doi:10.1016/j.chemosphere.2020.128607
Palanivel, T. M., Pracejus, B., and Novo, L. A. B. (2023). Bioremediation of copper using indigenous fungi Aspergillus species isolated from an abandoned copper mine soil. Chemosphere 314, 137688. doi:10.1016/j.chemosphere.2022.137688
Pande, V., Pandey, S. C., Sati, D., Bhatt, P., and Samant, M. (2022). Microbial interventions in bioremediation of heavy metal contaminants in agroecosystem. Front. Microbiol. 6 (13), 824084. doi:10.3389/fmicb.2022.824084
Pang, Y., Zeng, G. M., Tang, L., Zhang, Y., Liu, Y. Y., Lei, X. X., et al. (2011). Cr(VI) reduction by Pseudomonas aeruginosa immobilized in a polyvinyl alcohol/sodium alginate matrix containing multi-walled carbon nanotubes. Bioresour. Technol. 102 (22), 10733–10736. doi:10.1016/j.biortech.2011.08.078
Park, B., and Son, Y. (2017). Ultrasonic and mechanical soil washing processes for the removal of heavy metals from soils. Ultrason. Sonochemistry 35, 640–645. doi:10.1016/j.ultsonch.2016.02.002
Pathania, P., Bhatia, R., and Khatri, M. (2020). Cross-competence and affectivity of maize rhizosphere bacteria Bacillus sp. MT7 in tomato rhizosphere. Sci. Hortic. 272, 109480. doi:10.1016/j.scienta.2020.109480
Perera, I. C., and Hemamali, E. H. (2022). Genetically modified organisms for bioremediation: current research and advancements. Bioremediation Environ. Pollut. Emerg. Trends Strategies, 163–186. doi:10.1007/978-3-030-86169-8_7
Pinel-Cabello, M., Jauregui, R., Jroundi, F., Geffers, R., Jarek, M., Link, A., et al. (2023). Genetic mechanisms for Se(VI) reduction and synthesis of trigonal 1-D nanostructures in Stenotrophomonas bentonitica: perspectives in eco-friendly nanomaterial production and bioremediation. Sci. Total Environ. 862, 160635. doi:10.1016/j.scitotenv.2022.160635
Priyadarshanee, M., and Das, S. (2021). Biosorption and removal of toxic heavy metals by metal tolerating bacteria for bioremediation of metal contamination: a comprehensive review. J. Environ. Chem. Eng. 9 (1), 104686. doi:10.1016/j.jece.2020.104686
Punetha, A., Saraswat, S., and Rai, J. P. N. (2022). An insight on microbial degradation of benzo[a]pyrene: current status and advances in research. World J. Microbiol. Biotechnol. 38 (4), 61. doi:10.1007/s11274-022-03250-3
Rahman, Z., and Singh, V. P. (2020). Bioremediation of toxic heavy metals (THMs) contaminated sites: concepts, applications and challenges. Environ. Sci. Pollut. Res. Int. 27 (22), 27563–27581. doi:10.1007/s11356-020-08903-0
Rani, A., Souche, Y. S., and Goel, R. (2009). Comparative assessment of in situ bioremediation potential of cadmium resistant acidophilic Pseudomonas putida 62BN and alkalophilic Pseudomonas monteilli 97AN strains on soybean. Int. Biodeterior. Biodegrad. 63 (1), 62–66. doi:10.1016/j.ibiod.2008.07.002
Ravindra, K., and Mor, S. (2019). Distribution and health risk assessment of arsenic and selected heavy metals in Groundwater of Chandigarh, India. Environ. Pollut. 250, 820–830. doi:10.1016/j.envpol.2019.03.080
Reddy, K. R., and Parupudi, U. S. (1997). Removal of chromium, nickel and cadmium from clays by in-situ electrokinetic remediation. Soil Sediment Contam. 6 (4), 391–407. doi:10.1080/15320389709383574
Riaz, M., Kamran, M., Fang, Y., Wang, Q., Cao, H., Yang, G., et al. (2021). Arbuscular mycorrhizal fungi-induced mitigation of heavy metal phytotoxicity in metal contaminated soils: a critical review. J. Hazard. Mater. 402, 123919. doi:10.1016/j.jhazmat.2020.123919
Rigoletto, M., Calza, P., Gaggero, E., Malandrino, M., and Fabbri, D. (2020). Bioremediation methods for the recovery of lead-contaminated soils: a review. Appl. Sci. Switz. 10 (10), 3528. doi:10.3390/app10103528
Riseh, R. S., Vazvani, M. G., Hajabdollahi, N., and Thakur, V. K. (2022). Bioremediation of heavy metals by rhizobacteria. Appl. Biochem. Biotechnol. 195, 4689–4711. doi:10.1007/s12010-022-04177-z
Riyazuddin, R., Nisha, N., Ejaz, B., Khan, M. I. R., Kumar, M., Ramteke, P. W., et al. (2022). A comprehensive review on the heavy metal toxicity and sequestration in plants. Biomolecules 12 (1), 43. doi:10.3390/biom12010043
Rizvi, A., and Khan, M. S. (2018). Heavy metal induced oxidative damage and root morphology alterations of maize (Zea mays L.) plants and stress mitigation by metal tolerant nitrogen fixing Azotobacter chroococcum. Ecotoxicol. Environ. Saf. 157, 9–20. doi:10.1016/j.ecoenv.2018.03.063
Rizvi, A., and Saghir Khan, M. (2019). Putative role of bacterial biosorbent in metal sequestration revealed by SEM–EDX and FTIR. Indian J. Microbiol. 59 (2), 246–249. doi:10.1007/s12088-019-00780-7
Rizvi, A., Zaidi, A., Ameen, F., Ahmed, B., Alkahtani, M. D. F., and Khan, M. S. (2020). Heavy metal induced stress on wheat: phytotoxicity and microbiological management. RSC Adv. 10 (63), 38379–38403. doi:10.1039/d0ra05610c
RoyChowdhury, A., Datta, R., and Sarkar, D. (2018). “Heavy metal pollution and remediation,” in Green chemistry: an inclusive approach, 359–373.
Russo, F., Ceci, A., Pinzari, F., Siciliano, A., Guida, M., Malusà, E., et al. (2019). Bioremediation of dichlorodiphenyltrichloroethane (DDT)- contaminated agricultural soils: potential of two autochthonous saprotrophic fungal strains. Appl. Environ. Microbiol. 85 (21), e01720. doi:10.1128/AEM.01720-19
Sabourian, P., Yazdani, G., Ashraf, S. S., Frounchi, M., Mashayekhan, S., Kiani, S., et al. (2020). Effect of physico-chemical properties of nanoparticles on their intracellular uptake. Int. J. Mol. Sci. 21 (21), 8019–8020. doi:10.3390/ijms21218019
Saha, L., Tiwari, J., Bauddh, K., and Ma, Y. (2021). Recent developments in microbe–plant-based bioremediation for tackling heavy metal-polluted soils. Front. Microbiol. 12, 731723. doi:10.3389/fmicb.2021.731723
Saibu, S., Adebusoye, S. A., and Oyetibo, G. O. (2023). Soil microbiome response to 2-chlorodibenzo-p-dioxin during bioremediation of contaminated tropical soil in a microcosm-based study. J. Hazard Mater 451, 131105. doi:10.1016/j.jhazmat.2023.131105
Sakshi, , and Haritash, A. K. (2020). A comprehensive review of metabolic and genomic aspects of PAH-degradation. Archives Microbiol. 202 (8), 2033–2058. doi:10.1007/s00203-020-01929-5
Saleem, S., Rizvi, A., and Khan, M. J. I. J. o. E. S.Technology (2022). Microbiome-mediated nano-bioremediation of heavy metals: a prospective approach of soil metal detoxification, 1–24.
Sall, M. L., Diaw, A. K. D., Gningue-Sall, D., Efremova Aaron, S., and Aaron, J. J. (2020). Toxic heavy metals: impact on the environment and human health, and treatment with conducting organic polymers, a review. Environ. Sci. Pollut. Res. 27 (24), 29927–29942. doi:10.1007/s11356-020-09354-3
Sanchez-Hernandez, J. C., Narvaez, C., Cares, X. A., Sabat, P., and Naidu, R. (2023). Predicting the bioremediation potential of earthworms of different ecotypes through a multi-biomarker approach. Sci. Total Environ. 862, 160547. doi:10.1016/j.scitotenv.2022.160547
Sapre, S., Gontia-Mishra, I., and Tiwari, S. (2021). Plant growth-promoting rhizobacteria ameliorates salinity stress in pea (pisum sativum). J. Plant Growth Regul. 189, 647–656. doi:10.1007/s00344-021-10329-y
Satya, A. D. M., Cheah, W. Y., Yazdi, S. K., Cheng, Y. S., Khoo, K. S., Vo, D. N., et al. (2023). Progress on microalgae cultivation in wastewater for bioremediation and circular bioeconomy. Environ. Res. 218, 114948. doi:10.1016/j.envres.2022.114948
Sayadi, M. H., Salmani, N., Heidari, A., and Rezaei, M. R. (2018). Bio-synthesis of palladium nanoparticle using Spirulina platensis alga extract and its application as adsorbent. Surfaces Interfaces 10, 136–143. doi:10.1016/j.surfin.2018.01.002
Schirawski, J., Hagens, W., Fitzgerald, G. F., Van Sinderen, D. J. A., and microbiology, e. (2002). Molecular characterization of cadmium resistance in Streptococcus thermophilus strain 4134: an example of lateral gene transfer. Appl. Environ. Microbiol. 68 (11), 5508–5516. doi:10.1128/aem.68.11.5508-5516.2002
Sengupta, D., Datta, S., Biswas, D., Banerjee, S., and Das, S. (2021). Prospective bioremediation of toxic heavy metals in water by surfactant exopolysaccharide of Ochrobactrum pseudintermedium using cost-effective substrate. Int. Microbiol. 24 (3), 441–453. doi:10.1007/s10123-021-00182-0
Seshadri, S., Saranya, K., and Kowshik, M. (2011). Green synthesis of lead sulfide nanoparticles by the lead resistant marine yeast, Rhodosporidium diobovatum. Biotechnol. Prog. 27 (5), 1464–1469. doi:10.1002/btpr.651
Sevak, P., Pushkar, B., and Mazumdar, S. (2023). Mechanistic evaluation of chromium bioremediation in Acinetobacter junii strain b2w: a proteomic approach. J. Environ. Manage 328, 116978. doi:10.1016/j.jenvman.2022.116978
Shabaan, M., Asghar, H. N., Akhtar, M. J., Ali, Q., and Ejaz, M. (2021). Role of plant growth promoting rhizobacteria in the alleviation of lead toxicity to Pisum sativum L. Int. J. Phytoremediation 23 (8), 837–845. doi:10.1080/15226514.2020.1859988
Shabbir, Z., Sardar, A., Shabbir, A., Abbas, G., Shamshad, S., Khalid, S., et al. (2020). Copper uptake, essentiality, toxicity, detoxification and risk assessment in soil-plant environment. Chemosphere 259, 127436. doi:10.1016/j.chemosphere.2020.127436
Sharma, P., and Kumar, S. (2021). Bioremediation of heavy metals from industrial effluents by endophytes and their metabolic activity: recent advances. Bioresour. Technol. 339, 125589. doi:10.1016/j.biortech.2021.125589
Sharma, P., Pandey, A. K., Kim, S. H., Singh, S. P., Chaturvedi, P., and Varjani, S. (2021). Critical review on microbial community during in-situ bioremediation of heavy metals from industrial wastewater. Environ. Technol. Innovation 24, 101826. doi:10.1016/j.eti.2021.101826
Sher, S., and Rehman, A. (2019). Use of heavy metals resistant bacteria-a strategy for arsenic bioremediation. Appl. Microbiol. Biotechnol. 103 (15), 6007–6021. doi:10.1007/s00253-019-09933-6
Shi, T., Fang, L., Qin, H., Chen, Y., Wu, X., and Hua, R. (2019). Rapid biodegradation of the organophosphorus insecticide chlorpyrifos by Cupriavidus nantongensis X1(T). Int. J. Environ. Res. Public Health 16 (23), 4593. doi:10.3390/ijerph16234593
Sigua, G. C., Novak, J. M., Watts, D. W., Ippolito, J. A., Ducey, T. F., Johnson, M. G., et al. (2019). Phytostabilization of Zn and Cd in mine soil using corn in combination with biochars and manure-based compost. Environ. - MDPI 6 (6), 69. doi:10.3390/environments6060069
Silvianti, F., Maniar, D., Boetje, L., and Loos, K. (2020). Green pathways for the enzymatic synthesis of furan-based polyesters and polyamides. (United States: ACS Symposium Series), 3–29.
Singh, E., Osmani, R. A. M., and Banerjee, R. (2020). “Nanobioremediation: an emerging approach for a cleaner environment,” in Microbial bioremediation & biodegradation, 309–363.
Sobolev, D., and Begonia, M. F. T. (2008). Effects of heavy metal contamination upon soil microbes: lead-induced changes in general and denitrifying microbial communities as evidenced by molecular markers. Int. J. Environ. Res. Public Health 5 (5), 450–456. doi:10.3390/ijerph5050450
Soleimani, M., Hajabbasi, M. A., Afyuni, M., Mirlohi, A., Borggaard, O. K., and Holm, P. E. (2010). Effect of endophytic fungi on cadmium tolerance and bioaccumulation by Festuca arundinacea and Festuca pratensis. Int. J. Phytoremediation 12 (6), 535–549. doi:10.1080/15226510903353187
Song, B., Zeng, G., Gong, J., Liang, J., Xu, P., Liu, Z., et al. (2017). Evaluation methods for assessing effectiveness of in situ remediation of soil and sediment contaminated with organic pollutants and heavy metals. Environ. Int. 105, 43–55. doi:10.1016/j.envint.2017.05.001
Song, X., Zhang, J., Peng, C., and Li, D. (2021). Replacing nitrogen fertilizer with nitrogen-fixing cyanobacteria reduced nitrogen leaching in red soil paddy fields. Agric. Ecosyst. Environ. 312, 107320. doi:10.1016/j.agee.2021.107320
Sreedevi, P. R., Suresh, K., and Jiang, G. (2022). Bacterial bioremediation of heavy metals in wastewater: a review of processes and applications. J. Water Process Eng. 48, 102884. doi:10.1016/j.jwpe.2022.102884
Subramaniyam, V., Subashchandrabose, S. R., Thavamani, P., Megharaj, M., Chen, Z., and Naidu, R. (2015). Chlorococcum sp. MM11—a novel phyco-nanofactory for the synthesis of iron nanoparticles. J. Appl. Phycol. 27 (5), 1861–1869. doi:10.1007/s10811-014-0492-2
Sukul, P., Kumar, J., Rani, A., Abdillahi, A. M., Rakesh, R. B., and Kumar, M. H. (2021). Functioning of plant growth promoting rhizobacteria (PGPR) and their mode of actions: an overview from chemistry point of view. Plant Arch. 21, 628–634. doi:10.51470/PLANTARCHIVES.2021.v21.S1.096
Sukweenadhi, J., Kim, Y. J., Choi, E. S., Koh, S. C., Lee, S. W., Kim, Y. J., et al. (2015). Paenibacillus yonginensis DCY84T induces changes in Arabidopsis thaliana gene expression against aluminum, drought, and salt stress. Microbiol. Res. 172, 7–15. doi:10.1016/j.micres.2015.01.007
Sun, G. L., Reynolds, E. E., and Belcher, A. M. (2020). Using yeast to sustainably remediate and extract heavy metals from waste waters. Nat. Sustain. 3 (4), 303–311. doi:10.1038/s41893-020-0478-9
Sun, Y., Gao, K., Zhang, Y., and Zou, H. (2017). Remediation of persistent organic pollutant-contaminated soil using biosurfactant-enhanced electrokinetics coupled with a zero-valent iron/activated carbon permeable reactive barrier. Environ. Sci. Pollut. Res. Int. 24 (36), 28142–28151. doi:10.1007/s11356-017-0371-x
Sunanda, S., Misra, M., and Ghosh Sachan, S. (2022). Nanobioremediation of heavy metals: perspectives and challenges. J. Basic Microbiol. 62 (3-4), 428–443. doi:10.1002/jobm.202100384
Suresh, G., Balasubramanian, B., Ravichandran, N., Ramesh, B., Kamyab, H., Velmurugan, P., et al. (2021). Bioremediation of hexavalent chromium-contaminated wastewater by Bacillus thuringiensis and Staphylococcus capitis isolated from tannery sediment. Biomass Convers. Biorefinery 11 (2), 383–391. doi:10.1007/s13399-020-01259-y
Syed, K., Shale, K., Pagadala, N. S., and Tuszynski, J. (2014). Systematic identification and evolutionary analysis of catalytically versatile cytochrome P450 monooxygenase families enriched in model basidiomycete fungi. PLoS ONE 9 (1), e86683. doi:10.1371/journal.pone.0086683
Syed, Z., Sogani, M., Rajvanshi, J., and Sonu, K. (2022). Microbial biofilms for environmental bioremediation of heavy metals: a review. Appl. Biochem. Biotechnol. 195, 5693–5711. doi:10.1007/s12010-022-04276-x
Sytar, O., Ghosh, S., Malinska, H., Zivcak, M., and Brestic, M. (2021). Physiological and molecular mechanisms of metal accumulation in hyperaccumulator plants. Physiol. Plant. 173 (1), 148–166. doi:10.1111/ppl.13285
Takáčová, A., Smolinská, M., Ryba, J., Mackuľak, T., Jokrllová, J., Hronec, P., et al. (2014). Biodegradation of benzo[a]Pyrene through the use of algae. Central Eur. J. Chem. 12 (11), 1133–1143. doi:10.2478/s11532-014-0567-6
Talukdar, D., Jasrotia, T., Sharma, R., Jaglan, S., Kumar, R., Vats, R., et al. (2020). Evaluation of novel indigenous fungal consortium for enhanced bioremediation of heavy metals from contaminated sites. Environ. Technol. Innovation 20, 101050. doi:10.1016/j.eti.2020.101050
Tambat, V. S., Patel, A. K., Chen, C. W., Raj, T., Chang, J. S., Singhania, R. R., et al. (2023). A sustainable vanadium bioremediation strategy from aqueous media by two potential green microalgae. Environ. Pollut. 323, 121247. doi:10.1016/j.envpol.2023.121247
Tan, S. Y., Praveena, S. M., Abidin, E. Z., and Cheema, M. S. (2016). A review of heavy metals in indoor dust and its human health-risk implications. Rev. Environ. Health 31 (4), 447–456. doi:10.1515/reveh-2016-0026
Tauqeer, H. M., Ali, S., Rizwan, M., Ali, Q., Saeed, R., Iftikhar, U., et al. (2016). Phytoremediation of heavy metals by Alternanthera bettzickiana: growth and physiological response. Ecotoxicol. Environ. Saf. 126, 138–146. doi:10.1016/j.ecoenv.2015.12.031
Tessaro, A. P. G., de Araujo, L. G., Silva, T. T., Coelho, E., Correa, B., Rolindo, N. C., et al. (2023). Prospects for fungal bioremediation of unburied waste packages from the Goiania radiological accident. Environ. Sci. Pollut. Res. Int. 30, 41045–41059. doi:10.1007/s11356-023-25247-7
Thakur, K., Kuthiala, T., Singh, G., Arya, S. K., Iwai, C. B., Ravindran, B., et al. (2023). An alternative approach towards nitrification and bioremediation of wastewater from aquaponics using biofilm-based bioreactors: a review. Chemosphere 316, 137849. doi:10.1016/j.chemosphere.2023.137849
Thakur, M., and Pathania, D. (2019). “Environmental fate of organic pollutants and effect on human health,” in Abatement of environmental pollutants: trends and strategies, 245–262.
Thokchom, E., Thakuria, D., Kalita, M. C., Sharma, C. K., and Talukdar, N. C. (2017). Root colonization by host-specific rhizobacteria alters indigenous root endophyte and rhizosphere soil bacterial communities and promotes the growth of Mandarin orange. Eur. J. Soil Biol. 79, 48–56. doi:10.1016/j.ejsobi.2017.02.003
Tibi, F., Charfi, A., Cho, J., and Kim, J. (2020). Fabrication of polymeric membranes for membrane distillation process and application for wastewater treatment: critical review. Process Safety and Environmental Protection 141, 190–201.
Titchou, F. E., Zazou, H., Afanga, H., El Gaayda, J., Akbour, R. A., and Hamdani, M. (2021). Removal of Persistent Organic Pollutants (POPs) from water and wastewater by adsorption and electrocoagulation process. Groundw. Sustain. Dev. 13, 100575. doi:10.1016/j.gsd.2021.100575
Torabia, N., and Kardel, F. (2019). Biosorption of nickel by halobacillus sp. Kn57 isolated from the miankaleh wetland, Iran. Int. J. Aquatic Biol. 7 (5), 280–290. doi:10.22034/ijab.v7i5.704
Touliabah, H. E. S., El-Sheekh, M. M., Ismail, M. M., and El-Kassas, H. (2022). A review of microalgae-and cyanobacteria-based biodegradation of organic pollutants. Molecules 27 (3), 1141. doi:10.3390/molecules27031141
Tufail, M. A., Iltaf, J., Zaheer, T., Tariq, L., Amir, M. B., Fatima, R., et al. (2022). Recent advances in bioremediation of heavy metals and persistent organic pollutants: a review. Sci. Total Environ. 850, 157961. doi:10.1016/j.scitotenv.2022.157961
Tyagi, B., and Kumar, N. (2020). “Bioremediation: principles and applications in environmental management,” in Bioremediation for environmental sustainability: toxicity, mechanisms of contaminants degradation, detoxification and challenges, 3–28.
Ubaid ur Rahman, H., Asghar, W., Nazir, W., Sandhu, M. A., Ahmed, A., and Khalid, N. (2021). A comprehensive review on chlorpyrifos toxicity with special reference to endocrine disruption: evidence of mechanisms, exposures and mitigation strategies. Sci. Total Environ. 755, 142649. doi:10.1016/j.scitotenv.2020.142649
Ullah, A., Ma, Y., Li, J., Tahir, N., and Hussain, B. (2020). Effective amendments on cadmium, arsenic, chromium and lead contaminated paddy soil for rice safety. Agronomy 10 (3), 359. doi:10.3390/agronomy10030359
Ullah, N., Rehman, M. U., Ahmad, B., Ali, I., Younas, M., Aslam, M. S., et al. (2022). Assessment of heavy metals accumulation in agricultural soil, vegetables and associated health risks. PLoS ONE 17 (6 June), e0267719. doi:10.1371/journal.pone.0267719
Ullah, S., and Bano, A. (2015). Isolation of plant-growth-promoting rhizobacteria from rhizospheric soil of halophytes and their impact on maize (Zea mays L.) under induced soil salinity. Can. J. Microbiol. 61 (4), 307–313. doi:10.1139/cjm-2014-0668
Usman, M., Farooq, M., Wakeel, A., Nawaz, A., Cheema, S. A., Rehman, H. U., et al. (2020). Nanotechnology in agriculture: current status, challenges and future opportunities. Sci. Total Environ. 721, 137778. doi:10.1016/j.scitotenv.2020.137778
Valentim dos Santos, J., Varón-López, M., Fonsêca Sousa Soares, C. R., Lopes Leal, P., Siqueira, J. O., and de Souza Moreira, F. M. (2016). Biological attributes of rehabilitated soils contaminated with heavy metals. Environ. Sci. Pollut. Res. 23 (7), 6735–6748. doi:10.1007/s11356-015-5904-6
Valls, M., Atrian, S., De Lorenzo, V., and Fernández, L. A. (2000). Engineering a mouse metallothionein on the cell surface of Ralstonia eutropha CH34 for immobilization of heavy metals in soil. Nat. Biotechnol. 18 (6), 661–665. doi:10.1038/76516
Vanisree, C. R., Sankhla, M. S., Singh, P., Jadhav, E. B., Verma, R. K., Awasthi, K. K., et al. (2022). Heavy metal contamination of food crops: transportation via food chain. Hum. Consum. Toxic. Manag. Strateg.
Verma, N., Jujjavarapu, S. E., Mahapatra, C., and Mutra, J. K. R. (2023). Contemporary updates on bioremediation applications of graphene and its composites. Environ. Sci. Pollut. Res. Int. 30, 48854–48867. doi:10.1007/s11356-023-26225-9
Verma, N., and Sharma, R. (2017). Bioremediation of toxic heavy metals: a patent review. Recent Pat. Biotechnol. 11 (3), 171–187. doi:10.2174/1872208311666170111111631
Wan, W., Qin, Y., Wu, H., Zuo, W., He, H., Tan, J., et al. (2020). Isolation and characterization of phosphorus solubilizing bacteria with multiple phosphorus sources utilizing capability and their potential for lead immobilization in soil. Front. Microbiol. 11, 752. doi:10.3389/fmicb.2020.00752
Wang, L., Hou, D., Shen, Z., Zhu, J., Jia, X., Ok, Y. S., et al. (2020). Field trials of phytomining and phytoremediation: a critical review of influencing factors and effects of additives. Crit. Rev. Environ. Sci. Technol. 50 (24), 2724–2774. doi:10.1080/10643389.2019.1705724
Wang, P., Hu, X., He, Q., Waigi, M. G., Wang, J., and Ling, W. (2018). Using calcination remediation to stabilize heavy metals and simultaneously remove polycyclic aromatic hydrocarbons in soil. Int. J. Environ. Res. Public Health 15 (8), 1731. doi:10.3390/ijerph15081731
Wang, Q., Zhang, W. J., He, L. Y., and Sheng, X. F. (2018). Increased biomass and quality and reduced heavy metal accumulation of edible tissues of vegetables in the presence of Cd-tolerant and immobilizing Bacillus megaterium H3. Ecotoxicol. Environ. Saf. 148, 269–274. doi:10.1016/j.ecoenv.2017.10.036
Wang, X., Zhang, D., Pan, X., Lee, D. J., Al-Misned, F. A., Mortuza, M. G., et al. (2017). Aerobic and anaerobic biosynthesis of nano-selenium for remediation of mercury contaminated soil. Chemosphere 170, 266–273. doi:10.1016/j.chemosphere.2016.12.020
Wang, Z., Wang, H., Nie, Q., Ding, Y., Lei, Z., Zhang, Z., et al. (2023a). Pb(II) bioremediation using fresh algal-bacterial aerobic granular sludge and its underlying mechanisms highlighting the role of extracellular polymeric substances. J. Hazard Mater 444 (Pt B), 130452. doi:10.1016/j.jhazmat.2022.130452
Wang, Z., Zhang, J., Dai, Y., Zhang, L., Guo, J., Xu, S., et al. (2023b). Mediating effect of endocrine hormones on association between per- and polyfluoroalkyl substances exposure and birth size: findings from sheyang mini birth cohort study. Environ. Res. 115658, 115658. doi:10.1016/j.envres.2023.115658
Wei, Z., Van Le, Q., Peng, W., Yang, Y., Yang, H., Gu, H., et al. (2021). A review on phytoremediation of contaminants in air, water and soil. J. Hazard. Mater. 403, 123658. doi:10.1016/j.jhazmat.2020.123658
Wickramasinghe, W., Girija, D., Gopal, K. S., and Kesevan, S. (2021). Multi-phasic nitrogen fixing plant growth promoting rhizobacteria as biofertilizer for rice cultivation. Res. J. Agric. Sci. 12, 399–404.
Wu, X., Huang, P., Dong, C., and Deng, X. (2021). Nickel bioaccumulation by a marine bacterium Brevibacterium sp. (X6) isolated from Shenzhen Bay, China. Mar. Pollut. Bull. 170, 112656. doi:10.1016/j.marpolbul.2021.112656
Wu, Z., Yu, F., Sun, X., Wu, S., Li, X., Liu, T., et al. (2018). Long term effects of Lespedeza bicolor revegetation on soil bacterial communities in Dexing copper mine tailings in Jiangxi Province, China. Appl. Soil Ecol. 125, 192–201. doi:10.1016/j.apsoil.2018.01.011
Wyszkowska, J., Borowik, A., Kucharski, M., and Kucharski, J. (2013). Effect of cadmium, copper and zinc on plants, soil microorganisms and soil enzymes. J. Elem. 18 (4), 769–796. doi:10.5601/jelem.2013.18.4.455
Xia, X., Lin, S., Zhao, J., Zhang, W., Lin, K., Lu, Q., et al. (2018). Toxic responses of microorganisms to nickel exposure in farmland soil in the presence of earthworm (Eisenia fetida). Chemosphere 192, 43–50. doi:10.1016/j.chemosphere.2017.10.146
Xiang, Y., Xing, Z., Liu, J., Qin, W., and Huang, X. (2020). Recent advances in the biodegradation of polychlorinated biphenyls. World J. Microbiol. Biotechnol. 36 (10), 145. doi:10.1007/s11274-020-02922-2
Xie, Y., Fan, J., Zhu, W., Amombo, E., Lou, Y., Chen, L., et al. (2016). Effect of heavy metals pollution on soil microbial diversity and bermudagrass genetic variation. Front. Plant Sci. 7 (MAY2016), 755. doi:10.3389/fpls.2016.00755
Xu, Y., Wang, S., Li, L., Sahu, S. K., Petersen, M., Liu, X., et al. (2019). Molecular evidence for origin, diversification and ancient gene duplication of plant subtilases (SBTs). Sci. Rep. 9 (1), 12485. doi:10.1038/s41598-019-48664-6
Yadav, K. K., Singh, J. K., Gupta, N., and Kumar, V. (2017). A review of nanobioremediation technologies for environmental cleanup: a novel biological approach. J. Mater. Environ. Sci. 8 (2), 740–757.
Yan, F. F., Wu, C., Cheng, Y. Y., He, Y. R., Li, W. W., and Yu, H. Q. (2013). Carbon nanotubes promote Cr(VI) reduction by alginate-immobilized Shewanella oneidensis MR-1. Biochem. Eng. J. 77, 183–189. doi:10.1016/j.bej.2013.06.009
Yang, J., Cao, J., Xing, G., and Yuan, H. (2015). Lipid production combined with biosorption and bioaccumulation of cadmium, copper, manganese and zinc by oleaginous microalgae Chlorella minutissima UTEX2341. Bioresour. Technol. 175, 537–544. doi:10.1016/j.biortech.2014.10.124
Yang, Q., Guo, Y., Xiang, Y., Chen, L., Liu, G., Liu, Y., et al. (2023). Toward efficient bioremediation of methylmercury in sediment using merB overexpressed Escherichia coli. Water Res. 229, 119502. doi:10.1016/j.watres.2022.119502
Yang, X., Liu, L., Tan, W., Liu, C., Dang, Z., and Qiu, G. (2020). Remediation of heavy metal contaminated soils by organic acid extraction and electrochemical adsorption. Environ. Pollut. 264, 114745. doi:10.1016/j.envpol.2020.114745
Yang, Y., Zhou, X., Tie, B., Peng, L., Li, H., Wang, K., et al. (2017). Comparison of three types of oil crop rotation systems for effective use and remediation of heavy metal contaminated agricultural soil. Chemosphere 188, 148–156. doi:10.1016/j.chemosphere.2017.08.140
Yang, Y.-C., Huang, W.-S., Hu, S.-M., Huang, C.-W., Chiu, C.-H., and Chen, H.-Y. J. P. (2020). Synergistic and regulatable bioremediation capsules fabrication based on vapor-phased encapsulation of bacillus bacteria and its regulator by poly-p-Xylylene. Polym. (Basel). 13 (1), 41. doi:10.3390/polym13010041
Yanitch, A., Kadri, H., Frenette-Dussault, C., Joly, S., Pitre, F. E., and Labrecque, M. (2020). A four-year phytoremediation trial to decontaminate soil polluted by wood preservatives: phytoextraction of arsenic, chromium, copper, dioxins and furans. Int. J. Phytoremediation 22, 1505–1514. doi:10.1080/15226514.2020.1785387
Yetunde Mutiat, F.-B., Gbolahan, B., and Olu, O. J. B. J. (2018). A comparative study of the wild and mutated heavy metal resistant Klebsiella variicola generated for cadmium bioremediation. Bioremediat. J. 22 (1-2), 28–42. doi:10.1080/10889868.2018.1445695
Yu, Y., Shi, K., Li, X., Luo, X., Wang, M., Li, L., et al. (2022). Reducing cadmium in rice using metallothionein surface-engineered bacteria WH16-1-MT. Environ. Res. 203, 111801. doi:10.1016/j.envres.2021.111801
Zafar-ul-Hye, M., Tahzeeb-ul-Hassan, M., Abid, M., Fahad, S., Brtnicky, M., Dokulilova, T., et al. (2020). Potential role of compost mixed biochar with rhizobacteria in mitigating lead toxicity in spinach. Sci. Rep. 10 (1), 12159. doi:10.1038/s41598-020-69183-9
Zanganeh, F., Heidari, A., Sepehr, A., and Rohani, A. (2022). Bioaugmentation and bioaugmentation–assisted phytoremediation of heavy metal contaminated soil by a synergistic effect of cyanobacteria inoculation, biochar, and purslane (Portulaca oleracea L.). Environ. Sci. Pollut. Res. 29 (4), 6040–6059. doi:10.1007/s11356-021-16061-0
Zaynab, M., Al-Yahyai, R., Ameen, A., Sharif, Y., Ali, L., Fatima, M., et al. (2022). Health and environmental effects of heavy metals. J. King Saud Univ. - Sci. 34 (1), 101653. doi:10.1016/j.jksus.2021.101653
Zhai, X., Li, Z., Huang, B., Luo, N., Huang, M., Zhang, Q., et al. (2018). Remediation of multiple heavy metal-contaminated soil through the combination of soil washing and in situ immobilization. Sci. Total Environ. 635, 92–99. doi:10.1016/j.scitotenv.2018.04.119
Zhan, F., Li, B., Jiang, M., Li, T., He, Y., Li, Y., et al. (2019). Effects of arbuscular mycorrhizal fungi on the growth and heavy metal accumulation of bermudagrass [Cynodon dactylon (L.) Pers.] grown in a lead–zinc mine wasteland. Int. J. Phytoremediation 21 (9), 849–856. doi:10.1080/15226514.2019.1577353
Zhang, J., Wang, X., Zhang, L. X., and Zhao, F. J. (2021). Reducing cadmium bioavailability and accumulation in vegetable by an alkalizing bacterial strain. Sci. Total Environ. 758, 143596. doi:10.1016/j.scitotenv.2020.143596
Zhang, L., Liu, W., Liu, S., Zhang, P., Ye, C., and Liang, H. (2020). Revegetation of a barren rare earth mine using native plant species in reciprocal plantation: effect of phytoremediation on soil microbiological communities. Environ. Sci. Pollut. Res. 27 (2), 2107–2119. doi:10.1007/s11356-019-06645-2
Zhang, W., Zhang, H., Xu, R., Qin, H., Liu, H., and Zhao, K. (2023). Heavy metal bioremediation using microbially induced carbonate precipitation: key factors and enhancement strategies. Front. Microbiol. 14, 1116970. doi:10.3389/fmicb.2023.1116970
Zhao, X., Zeng, X., Qin, Y., Li, X., Zhu, T., and Tang, X. (2018). An experimental and theoretical study of the adsorption removal of toluene and chlorobenzene on coconut shell derived carbon. Chemosphere 206, 285–292. doi:10.1016/j.chemosphere.2018.04.126
Zhou, H., Gao, X., Wang, S., Zhang, Y., Coulon, F., and Cai, C. (2023a). Enhanced bioremediation of aged polycyclic aromatic hydrocarbons in soil using immobilized microbial consortia combined with strengthening remediation strategies. Int. J. Environ. Res. Public Health 20 (3), 1766. doi:10.3390/ijerph20031766
Zhou, X., Zhang, S., Wang, R., An, Z., Sun, F., Shen, C., et al. (2023b). A novel strategy for enhancing bioremediation of polychlorinated biphenyl-contaminated soil with resuscitation promoting factor and resuscitated strain. J. Hazard Mater 447, 130781. doi:10.1016/j.jhazmat.2023.130781
Zhu, J., Zhao, Y. A. N., and Ruan, H. (2019). Comparative study on the biodegradation of chlorpyrifos-methyl by bacillus megaterium CM-Z19 and pseudomonas syringae CM-Z6. An. Acad. Bras. Ciencias 91 (3), e20180694. doi:10.1590/0001-3765201920180694
Zhu, M., Yin, H., Yuan, Y., Qi, X., Liu, H., Wei, X., et al. (2022). Promotion of the biodegradation of phenanthrene adsorbed on microplastics by the functional bacterial consortium QY1 in the presence of humic acid: bioavailability and toxicity evaluation. Environ. Pollut. 307, 119591. doi:10.1016/j.envpol.2022.119591
Zhu, N., Zhang, B., and Yu, Q. (2020). Genetic engineering-facilitated coassembly of synthetic bacterial cells and magnetic nanoparticles for efficient heavy metal removal. ACS Appl. Mater. Interfaces 12 (20), 22948–22957. doi:10.1021/acsami.0c04512
Zhuo, R., and Fan, F. (2021). A comprehensive insight into the application of white rot fungi and their lignocellulolytic enzymes in the removal of organic pollutants. Sci. Total Environ. 778, 146132. doi:10.1016/j.scitotenv.2021.146132
Keywords: heavy metals, nano-bioremediation, PGPR, phytoremediation, pollution
Citation: Karnwal A, Martolia S, Dohroo A, Al-Tawaha ARMS and Malik T (2024) Exploring bioremediation strategies for heavy metals and POPs pollution: the role of microbes, plants, and nanotechnology. Front. Environ. Sci. 12:1397850. doi: 10.3389/fenvs.2024.1397850
Received: 08 March 2024; Accepted: 17 July 2024;
Published: 31 July 2024.
Edited by:
Khandaker Rayhan Mahbub, Primary Industries and Resources South Australia, AustraliaReviewed by:
Neha Pandey, Pandit Ravishankar Shukla University, IndiaPadmanabh Dwivedi, Banaras Hindu University, India
Copyright © 2024 Karnwal, Martolia, Dohroo, Al-Tawaha and Malik. This is an open-access article distributed under the terms of the Creative Commons Attribution License (CC BY). The use, distribution or reproduction in other forums is permitted, provided the original author(s) and the copyright owner(s) are credited and that the original publication in this journal is cited, in accordance with accepted academic practice. No use, distribution or reproduction is permitted which does not comply with these terms.
*Correspondence: Arun Karnwal, YXJ1bmthcm53YWxAZ21haWwuY29t; Tabarak Malik, bWFsaWtpdHJjQGdtYWlsLmNvbQ==