- 1Department of Surgery, New York Presbyterian Hospital-Cornell Medical Center Weill-Cornell Medical College, New York, NY, United States
- 2Department of Biology, University of Alabama at Birmingham, Birmingham, AL, United States
- 3Department of Neurology, Rutgers New Jersey Medical School, Newark, NJ, United States
- 4Department of Medicine, Guam Regional Medical Center, Dededo, Guam
Since its inception, cellular plasticity has undergone many iterations. Today we define it as the ability of mature, terminally differentiated cells to change their identity, meaning lineage change of the cells by transdifferentiation, dedifferentiation and reprogramming. This process does not involve a single DNA sequence change or a mutation. We now know that the behavior of a cell is profoundly affected by the surrounding environment. There is a perpetual pressure placed on the genetic expression of the cells. The external environment and specifically the microenvironment of the cells greatly influences the genotype. There is a never-ending dynamic interplay between the genotype and the phenotype. Incremental phenotypic adjustments are continuously occurring to yield improved cell survival. These changes are beneficial to the cells at a given moment. As the environmental condition declines, then more extensive phenotypic transformation (via transdifferentiation and dedifferentiation) can follow. When the cellular environment further deteriorates, cellular plasticity can trigger a pathologic sequence that eventually leads to cancers/diseases. These modifications are all part of an adaptive process that enhances the survival of the cells. They can offer short term advantages, but they can also lead to diseases. Oxygen level plays a pivotal role in the development of chronic diseases. Cellular response to hypoxia is mediated through hypoxia inducible factor (HIF). HIF is an oxygen sensor that is closely involved in the pathophysiologic adaptation to hypoxia. Our hypothesis centers on hypoxia as the major stressor initiating cellular plasticity and restoring normoxia is an essential step in the healing process. This theory could be tested using chronic pathological processes in animal models whereby achieving an adequate cellular oxygen level could improve or halt both plastic change and diseases.
Introduction
The human body is a fine-tuned machine aimed at deliberate precision. Biological responses of the body to stimuli are carefully measured for an explicit purpose, but this same design can precipitate a disease process. Our body is an Evolutionary construct that is a product of a fluid environment. Survival of each of the 37 trillion cells hinges on homeostasis which operates in a tight physiologic range to maintain a stable internal environment. When the cells are damaged, homeostasis is disturbed and proceeded by inflammation and repair (Kotas and Medzhitov, 2015; Modell et al., 2015). As the environmental condition is perturbed, the equilibrium is disrupted but reset to optimize the survival of the cells.
In response to injury, cellular plasticity plays an integral role in regenerating and restoring homeostasis (Galliot and Ghila, 2010). The timing and the nature of the physiologic insult, and how the body counteracts a specific injury, determines the fate of recovery or disease (Furman et al., 2019). On the surface, the most common diseases such as hypertension, heart disease, stroke, diabetes and cancer seem to stem from different etiologies. But if we examine diseases at a cellular level then we can better decipher their common origin. Furthermore, diseases manifest in a spectrum, shifting from one end to the other based on the signals from the environment. This process is precisely calibrated by the malleability of the cells. Cellular plasticity offers a paradigm shift in the way we look at diseases.
Cellular environment
Observable physical characteristics of a cell is the phenotype. The genotype or the genetic material of a cell gives rise to the phenotype. The interaction between genotype and the environment produces a discrete phenotype. Environmental factors such as diet, temperature, oxygen levels, humidity, light cycles, and the presence of mutagens can all impact an organism’s gene expressions. which ultimately affects the phenotype.
There’s a bidirectional communication between the organisms and the surrounding habitat. There are three distinct environments that can impact the phenotype: micro, macro, and the external environment. Microenvironment is a small subset of the macroenvironment and the macroenvironment is a further subset of the external environment.
External environment is simply the world we inhabit. There are many factors like the weather including the sun, the wind, the temperature, the atmospheric pressure, the humidity, and the vegetation, the elevation, and much more that each organism on earth confronts every day. But even the environmental pollution, the food we eat, the air we breathe can also affect the phenotype. The external world shapes the phenotype by exerting pressure on the macroenvironment which then transmits the message to the microenvironment.
Macroenvironment encompasses all the contiguous structure surrounding the cells including the tissues, the organs, the muscles, the bones, the blood vessels etc., all of which make up the entire body. The phenotype is deeply swayed by the body. Any fluctuation in the biological parameters of the body—like the blood pressure, the blood vessel physiology, the blood flow, various hormones, or factors such as nutrients, toxins, pathogens, and even stress—can reconfigure the macroenvironment.
Microenvironment is the smallest of the environmental unit and what immediately abuts the cells. Due to its close proximity, the microenvironment has the greatest direct impact on the phenotype. Major components of the microenvironment include other neighboring cells, extracellular matrix, interstitial fluid containing nutrients, toxins, hormones, immune cells, various factors, waste products and oxygen—of which oxygen level is recognized to be the most influential (Maltepe and Saugstad, 2009; Samanta et al., 2017; al-Ani et al., 2018).
Phenotypic plasticity offers flexibility to differentiated cells in navigating uncertain environments. Although plasticity is employed occasionally to facilitate cellular function, it is reserved mostly as a survival tool. When the phenotype encounters environmental stress, it can frequently modify the expression of the genotype, or intermittently alter the identity, or sporadically lead to a mutation—and can give rise to a brand new phenotype (Cuypers et al., 2017).
The role of oxygen
About 3 billion years ago, gaseous oxygen began to accumulate in the earth’s atmosphere due to cyanobacteria. Incorporating the sun, the water, and carbon dioxide, photosynthesis produced carbohydrates and oxygen. Six to nine-hundred million years later, the Great Oxidation Event transformed earth’s biosphere into an oxygen-rich environment. Oxygen is an efficient electron acceptor in the mitochondrial electron transport chain. Utilizing aerobic metabolism, metazoan life flourished by harnessing enormous amounts of energy. Coupling of evolution with oxygen accelerated the complexity of the organisms on earth. Oxygen abundance produced more diverse cell types, but these cells also became extremely dependent on oxygen (Hedges et al., 2004).
The importance of oxygen for the cells starts early in cells’ development. Oxygen is critical in promoting the differentiation process in the stem cells, while hypoxia is key in maintaining embryonic stem cell pluripotency (Lin et al., 2008). Even in fully differentiated cells, oxygen continues to exert a fundamental role in selecting a phenotype. Oxygen is so vital to the very survival of the cells that even a modest drop in oxygen level could have dire consequences (Grayson et al., 2006).
Injury to the cells prompts the immune system activation, unleashing inflammation. If insults continue, then chronic inflammation sets in, triggering a cascade of molecular and cellular events. Chronic inflammation leads to damaged blood vessels, especially to the microvasculature. The blood flow is interrupted, and the cells become deprived of oxygen and nutrients (injury-inflammation cascade; Granger and Kubes, 1994). When cellular oxygen level diminishes, hypoxia inducible factor is dissociated from Von Hippel–Lindau tumor suppressor (VHL). In a well-oxygenated state, HIF-1a is bound to VHL and become susceptible to proteasomal degradation by ubiquitin ligase (Semenza, 2001).
Under Hypoxic conditions, HIF is activated which is a transcription factor that regulates over 100 genes, binds to the DNA, and alters oxygen homeostasis and cellular metabolism (Liu et al., 2012; Choudhry and Harris, 2018). Oxidative phosphorylation is shut down, and anaerobic glycolytic pathway is chosen. Using glycolysis, the cells are able to generate an adequate amount of energy despite low oxygen levels. Nutrient uptake is enhanced through the activation of glucose transporter (GLUT) to increase glucose metabolism (Mamun et al., 2020). In addition, angiogenesis is initiated through vascular endothelial growth factor (VEGF) and many new blood vessels form (Semenza, 2012). Both isoforms of hypoxia inducible factor, HIF-1a and HIF-2a, play a critical role in the development and the maintenance of functioning circulatory system (Hashimoto and Shibasaki, 2015).
Oxygen homeostasis also involves reactive oxygen species (ROS). ROS are a byproduct of normal cellular metabolism. A balance between production and scavenging of ROS are essential to cellular proliferation and survival. Some ROS are needed for signaling molecules, but too much (oxidative stress) is detrimental to the cells (Thannickal and Fanburg, 2000). ROS play a regulatory role in the cellular response to hypoxia. Although hyperoxia can increase ROS production, hypoxia is the most prevalent cause under physiologic condition. As ROS level rise, HIF is then activated and the cells switch to glycolysis, limiting the buildup of ROS (Mullarky and Cantley, 2015).
When the cells experience prolonged hypoxia they mobilize deeper adaptive response. Along with HIF stabilization, the cells undergo phenotypic change. At first, they may proliferate through hyperplasia (both physiological and pathological), making many identical copies to combat hypoxia. Notably, it is through metaplasia that the cells are able to endure long standing unfavorable conditions. When the cells transdifferentiate into other distinct phenotypes, they acquire survival advantages (Jessen et al., 2015; Kumar and Choi, 2015). Once hypoxia is ameliorated, cessation of plastic change follows, and the cells may even revert to their original phenotype. However, if hypoxia is sustained, cells may regress to a stem cell-like, dedifferentiated state that can pave the way into malignancy (Wang et al., 2017). This equilibrium is both dynamic and fluid with oxygen as the principal driving force in cellular plasticity.
Cardiovascular disease
The most common cardiovascular diseases include hypertension, heart disease, peripheral vascular disease, stroke and heart attacks. Atherosclerosis is the predominant risk factor in the development of cardiovascular disease. The pathophysiology of atherosclerosis continues to evolve. It is no longer viewed as simply a fatty plaque buildup in the arterial walls that eventually narrows the lumen. We now know that chronic inflammation plays a major role in the formation of plaques. Plaques only occur in the thicker-walled arteries and not in thin-walled veins. Lower pressure arteries possess thinner walls and are far less likely to develop plaques (Vaudo et al., 2000; Feng et al., 2012).
Blood vessel wall is made up of three distinct layers. The intima is the innermost layer that contains the endothelium; the media is largely composed of vascular smooth muscle cells (VSMs); the adventitia is the outer lining. Higher pressure arteries have much thicker walls, and the thickening is due to the expansion of the media containing VSMs. As the blood pressure rises, the arterial wall further thickens (again, mostly in the VSMs). Thicker walled arteries have higher demand for oxygen (Laurent, 1995).
Arterial wall is perfused with oxygen by direct diffusion from the lumen and through the vasa vasorum. Vasa vasorum is an intricate network of vascular highways that penetrate the wall from the adventitia. Dual blood supply is needed to properly perfuse the entire wall. Vascular smooth muscle cells which make up the lion share of the arterial wall reside in the middle layer—the media is the watershed area that is most vulnerable to hypoxic injury (Milutinović et al., 2020).
Damage to the endothelium or a problem in the vasa vasorum will block oxygen delivery to the VSMs via injury-inflammation cascade (Mulligan-Kehoe and Simons, 2014; Sun et al., 2019). When oxygen delivery to the VSMs is compromised, HIF is activated. At first, VSMs cope by turning on glycolysis while inducing angiogenesis (pathologic angiogenesis; Camaré et al., 2017). With further insult, VSMs respond with hyperplasia, followed by phenotypic changes. Vascular smooth muscle cells possess a wide range of plasticity. Full phenotypic spectrum is on display as the VSMs are transformed into a macrophage-like foam cell, adipose-like cell, collagen producing cell, and osteochondrogenic cell (calcium forming). Switching of VSMs from a contractile to a synthetic phenotype strengthens the vascular wall and prevents rupture (Speer et al., 2009; Allahverdian et al., 2018; Lambert and Jørgensen, 2021). As inflammation subsides and oxygen level is restored to the VSMs, metaplasia ends, and the plaque recedes. However, if hypoxia persists, calcification of the artery that leads to the hardening of the blood vessel wall progresses (Balogh et al., 2019). When the blood vessel wall is injured, the healing process is orchestrated by the versatile vascular smooth muscle cells—plaque is the end result (Sorokin et al., 2020).
Metabolic disease
Our ancestors were hunter-gatherer who lived in an environment with nutritional scarcity. The metabolic system developed under fasting and feasting states where starvation was much more prevalent. Consequently, our bodies are better adept at handling starvation than overabundance (de Fano et al., 2022).
Metabolic diseases involve disturbances in making energy from ingested food. There are many different inherited disorders with enzymatic defects that alter the metabolism of the cells. However, the most common type of acquired metabolic disease, obesity and diabetes, both stem from excessive intake of nutrients and metabolic overload. In obesity, remarkable plastic changes in the adipose tissues lead to cardio-metabolic disorder. Although adipose tissue plasticity is an evolving topic that warrants further investigation, it is well known that adipose tissue expansion can result in lipotoxicity and in diabetes (de Fano et al., 2022).
The pathogenesis of diabetes can be traced to the impairment in the metabolic activities of the pancreas. The pancreas is essential in converting the food we eat into energy—this gland is composed of exocrine and endocrine cells. The exocrine portion is made up of the acinar and the ductal cells, and the endocrine encompasses the alpha, the beta, and the delta cells. Majority of the endocrine pancreas are the beta cells that produce insulin, but glucagon, somatostatin, and polypeptide are also made. These hormones are the main regulators of nutrient metabolism and glucose homeostasis.
Beta cells are tasked with maintaining glucose homeostasis by sensing blood sugar and linking it with energy metabolism. Exposure to high glucose stresses the beta cells as insulin demand rises. This challenge is met mostly by releasing more insulin, and to a lesser extent from the beta cell proliferation. However, as insulin resistance sets in, the beta cells enter a compensatory phase with further increase in secretion of insulin followed by the creation of new beta cells (Migliorini et al., 2014; Honzawa and Fujimoto, 2021). The cellular origin of these beta cells can be traced to other islets and also to the extra-islet cells. Nascent beta cells are derived from the transdifferentiation of the alpha, the delta, the acinar, the ductal and even from the cells of other organs. Phenotypic transformation into beta cells is responsible for hyperinsulinemia, but it may be reversible when the glucose level is normalized (Mezza et al., 2019). When confronted with metabolic adversity, our bodies employ extraordinary measures to preserve insulin production. Eventually, overstimulation of the beta cells results in the secretory disruption, followed by the failure of the compensatory mechanism (Cerf, 2013).
Plastic changes transform some beta cells back into the alpha and other pancreatic cells, however, many go onto dedifferentiate. These phenotypic adjustments are the preliminary segue into the development of diabetes (Talchai et al., 2012).
Concomitantly, hyperglycemia induces inflammation-hypoxia cascade (due to nutrient overload and increased inflammatory mediators) (Sato et al., 2014; Gunton, 2020). The pancreatic beta cells are highly metabolic and require ample supply of oxygen. Vascular architecture of the islet cells is structured to receive over 20% of the pancreatic blood flow. However, blood vessels surrounding the beta cells were found to be abnormal in the early stages of diabetes, thus diminishing the blood flow (Burganova et al., 2021). Hypoxia causes damage to the islet cells that translates into abnormal insulin secretion and beta cell dysfunction. Although apoptosis can occasionally occur, stress drives the beta cells to a dedifferentiated phenotype, which accounts for the majority of the functional loss. Just as oxygen is needed for the beta cell differentiation in early development (Heinis et al., 2010), hypoxia now drives the dedifferentiation process. Retreating to a dedifferentiated phenotype may be an adaptive response to escape death from hypoxia (Bensellam et al., 2018; Khin et al., 2021). In this progenitor-like state, the beta cells retain some identity but lose all their function. Most likely, hypoxia is a dominant factor in the demise of the beta cells, leading to a gradual beta cell breakdown and burnout, resulting in insulin deficient diabetes (Gerber and Rutter, 2017).
The pancreas exhibits an incredible array of plasticity. Cellular plasticity is critical for the beta cells to survive when the environment becomes hostile. This phenotypic flexibility that affords survival advantage in the pancreas is also taking place in the liver, the lungs, the cervix, the breasts, the skin, and in the gastrointestinal system (Tata and Rajagopal, 2016; Yuan et al., 2019).
Gastrointestinal disease
Gastrointestinal cells are one of the fastest renewing cells in the body. In response to injury, plasticity allows the cells in the esophagus, the stomach, the small and the large intestine, a pathway to adapt. The etiology of the most common gastrointestinal diseases arise from infectious, inflammatory, and chemo-induced injury—they often trigger plastic changes that can continue into malignancy. Additionally, hypoxia and HIF play a major role in the development of many gastrointestinal diseases (Goldenring and Mills, 2022).
Of the GI disorders, Barrett’s esophagus has been extensively studied and yet it is poorly understood. We have known for many years that the lining in the distal esophagus sustains injury due to acid reflux. To better understand Barrett’s esophagus, science needs to catch up to the mechanistic process of the disease. According to conventional wisdom, the transformation in the lining of the esophagus was simply a reactive response to acid burn injury. At the core of Barrett’s, chronic exposure to gastric acid causes damage to the lining in the distal esophagus, followed by a cytokine-mediated inflammatory response and wound healing (Souza, 2017). Fully differentiated cells that line this area then undergo cellular change that defy logic. Hypoxia inducible factor is activated as the squamous epithelium of the distal esophagus is replaced by the columnar cells. The cellular origin of Barrett’s is still being debated, but there’s no doubt about the elaborate plastic change (resembling gastrointestinal lining) that takes place (Que et al., 2019). This is part of an adaptive response to combat the toxic environment (especially hypoxia). Metaplasia and reprogramming of the cells are imperative to survive. If the reflux continues, then the columnar phenotype continues to thrive and may proceed to dysplasia. Theoretically, if the reflux is treated or reversed early, then the progression of the disease potentially could be halted. However, most Barrett’s metaplasia are pre-malignant, and the majority are not reversible (Martinucci et al., 2016).
Hypoxia inducible factor is elevated early and plays a prominent role in advancing the disease towards malignancy (Griffiths et al., 2007; Ling et al., 2009). With prolongation of Barrett’s, the axis can be tilted all the way to the dedifferentiation end that supports cancer (Helm et al., 2005).
Cancer
What if we looked at cancer from an entirely different lens. Let us think of cancer as a continuum of cellular plasticity rather than an isolated event prompted by a random mutation. The cells throughout the body share a similar fate when confronted with injury, inflammation, and hypoxia. They have the innate ability to adjust to the harsher surrounding. By using phenotypic modulation, the cells are better equipped to meet the environmental challenges. The single most important element for cellular microenvironment is oxygen. Hypoxia is a noxious stimulus that could profoundly alter the behavior of the cells. Since oxygen regulates the microenvironment of the cells, and hypoxia is the most pertinent physiological stressor, when oxygen level falls, the cells produce considerably less energy and accumulate substantially more toxic metabolic waste (Solaini et al., 2010). To survive, the cells mobilize HIF which then binds to the DNA and redirects cellular function. HIF protects our cells when oxygen is in short supply. Reprogrammed cells respond initially by switching to glycolysis and turning on angiogenesis, and frequently by replicating (Chen et al., 2019). If further challenged, they (cells) also incorporate metaplasia to remake their phenotype. When the cells are pushed to the brink and the oxygen supply dramatically dwindles, the cells shift to the far end of the phenotypic spectrum and a dedifferentiated phenotype emerges with stem cell-like features. Morphogenesis of cancer is a hierarchical process, transitioning from benign to malignant, primarily driven by the cellular environment. Through severe epigenetic disturbances, increasing alteration in gene expression leading to genetic instability, may be the impetus for the malignant transformation (adaptive mutation; Yamada et al., 2014; Carvalho, 2020).
Cellular plasticity operates in a dynamic equilibrium—equilibrium that continuously readjusts, selecting the optimal phenotype depending on the environmental condition. Plasticity provides the transcriptional rewiring to proliferate (hyperplasia), produce a different phenotype (metaplasia), become an abnormal cell (dysplasia), or trigger a mutation (neoplasia), giving birth to cancer (Yuan et al., 2019; Figure 1).
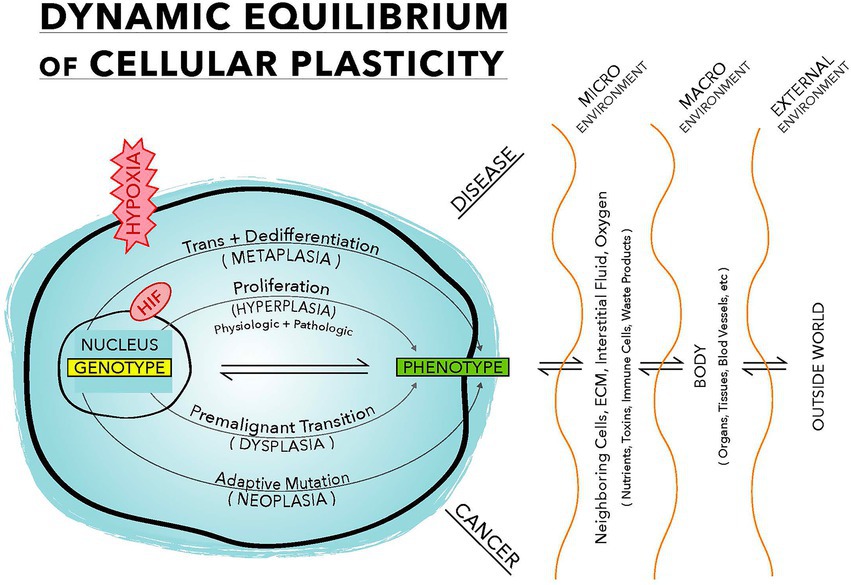
Figure 1. This illustration demonstrates a dynamic equilibrium between the genotype and the phenotype. Oxygen plays a pivotal role in selecting a phenotype. Pathophysiologic stress, mainly hypoxia, drives this equilibrium. Physiologic response involves only the proliferation arm, while pathological response involves all four arms. Upper half of this equilibrium leads to disease formation and the lower half is the malignant transformation.
Pancreatic cancer is one of the deadliest diseases, and pancreatic ductal adenocarcinoma (PDAC) is the most common malignancy in the pancreas. As with other cancers, chronic inflammation is a major precursor to PDAC. Injury-inflammation cascade leads to hypoxia. HIF is activated early and is intricately involved in the propagation of the disease (Li et al., 2019; Jung et al., 2020). When faced with chronic hypoxia, acinar cells undergo trans- and dedifferentiation. Although acinar to ductal metaplasia (ADM) is a very common and a reversible process, it is also the first step into malignancy (Storz, 2017; Grimont et al., 2022). ADM may be an adaptive mechanism to mitigate injury from hypoxic insults.
Hypoxia in the cells exist long before malignancy. When oxygen level remains persistently low, cells are faced with apoptosis. HIF which is present in all mammalian cells become active, VEGF is expressed, and angiogenesis follows to preserve the viability of the cells. Angiogenesis is a physiologic response to hypoxia. However, in the presence of chronically inflamed or diseased state (due to disrupted blood flow), angiogenesis yields dysfunctional blood vessels (pathologic angiogenesis). Despite the formation of extensive network of new blood vessels, the oxygen level in the cells does not improve (Felmeden et al., 2003). Under such hypoxic setting, select cells may be provoked and undergo plastic changes (and trigger an adaptive mutation) resulting in cancer. Neoplasia represents the ultimate adaptive pathway where the cancer phenotype is the fittest for this environment—consequently, once cells transition into malignancy, they are unable to return to their original phenotype. Born from a low oxygen milieu, cancer cells gain survival advantages as the tumor microenvironment remains hypoxic (Emami Nejad et al., 2021). They utilize the Warburg effect (which refers to the preference for glycolysis even in the presence of oxygen) to make energy, provided there are ample amounts of substrate (DeBerardinis and Chandel, 2020). Furthermore, cancer cells can thrive, exploiting cellular plasticity, evolving into a more evasive phenotype (Fanelli et al., 2020). Neoplastic transformation may actually be part of an evolutionary apparatus adopted by our cells to adapt in the harshest of conditions, especially hypoxia (Hypoxic Adaptation Theory of cancer or HAT).
Diseases as an evolutionary process
In Darwin’s, The Origin of Species, all creatures on earth share a common ancestor. Similarly, diseases we experience have a common origin. On a macro level, when the organisms are met with a hostile environment, they survive by adapting. Adaptation can occur immediately or gradually depending on the circumstances. Whether adapting through different shaped beaks of finches on Galapagos or the coloration of lizards living around the Pisgah Lava Flow, it is all in the name of survival, and evolution is fluid and evident at all times (Corl et al., 2018).
On a micro level, the cells also survive by adapting. They are being persistently pressured in an ever-changing environment. The cells are confronted with survival choices, where they must adapt or perish. Cellular plasticity not only plays a central role in adaptation, but emerging evidence suggesting that these cellular changes can also be individually heritable through a genetic or an epigenetic route (Buss, 1987; Katzir et al., 2012; Wund, 2012; Baugh and Day, 2020). Inheritance of acquired characteristics has enjoyed a recent resurgence (Liu, 2007). Cellular plasticity is the mechanism utilized by the cells to survive—evolution is occurring through cellular plasticity and cellular plasticity is evolution in motion. Both of these processes are taking place continuously.
Evolution is on full display when we examine malaria, a mosquito-borne parasitic disease that contributes to high rates of childhood mortality worldwide and especially in Africa. Untreated Plasmodium infection can lead to devastating hypoxic injuries in the various organs throughout the body (Park et al., 2019). Extraordinary evolutionary pressure has given way to a multitude of red blood cell pathologies including sickle cell, beta thalassemia, glucose-6-phosphate dehydrogenase deficiency, and other red blood cell defects (Kwiatkowski, 2005). These phenotypic polymorphisms have afforded some protection against malaria, but they have also resulted in red blood cell related disorders. Although red blood cell polymorphism in malaria has taken thousands of years of evolution, most common diseases we encounter today are occurring in a much shorter timeline. Diseases such as hypertension, heart diseases, stroke, diabetes, and cancer are all taking place within a human lifespan.
Our bodies and our cells are under constant assault, and survival is contingent on the plastic nature of the cells. Plasticity (both developmental and cellular) ensures that the most fit phenotype will be chosen for a specific environment (Fusco and Minelli, 2010). When the cells experience extreme pressures (like prolonged hypoxia), they adapt by acquiring a different identity. Cellular plasticity provides the vehicle for this adaptive response. The more robust phenotype arises with features that can endure tougher conditions. However, the newly modified phenotype undergoes morphological changes that initiate the disease process. Evolutionary trade-off has translated into enhanced survival in the short term, but it has also led to disease in the long run (Hypoxic Adaptation Theory of Disease).
The oxygen fix
Transporting oxygen to all the cells in a complex multicellular organism is a difficult endeavor. The development of a sophisticated circulatory system allowed efficient delivery of oxygen to every single cell in the body. The hemoglobin molecule on the red blood cell is the conduit for the transfer of oxygen from the air to the cells. Red blood cells travel through the blood vessels to the tiniest capillaries where an orderly gas exchange of oxygen for carbon dioxide occurs. Proficient oxygen delivery systems using hemoglobin must be equipped with a functioning vascular network and an intact capillary membrane interface, otherwise cells will suffer hypoxia.
Cellular response to hypoxia encompasses both physiological and pathological processes. Physiologic response is mandatory for embryonic and fetal development, growth, wound healing, endometrial proliferation during ovulation, and skeletal muscle adaptation to exercise; accompanied by the formation of many new, functional blood vessels (physiologic angiogenesis; Tahergorabi and Khazaei, 2012). Pathologic response leads to disease. Chronic diseases start with damage to the cells which sets off an injury-inflammation cascade. This wreaks havoc on the vascular tree (especially to the microvasculature) resulting in cellular hypoxia (Levy et al., 2008). HIF is activated, which then binds to the DNA and ignites a series of adaptive events. Cells switch to glycolysis and continue energy production. VEGF is expressed, followed by angiogenesis; the new blood vessels that form in this setting are abnormal, fragile and leaky, therefore, the cells stay oxygen deficient (pathologic angiogenesis; Ramakrishnan et al., 2014; Hashimoto and Shibasaki, 2015; Aguilar-Cazares et al., 2019).
In cardiovascular diseases, the underlying pathology is localized to the blood vessel walls. Damage to the endothelium and the vasa vasorum compromises the blood flow to the arterial wall. HIF is markedly elevated as hypoxia ensues, and impaired angiogenesis emanating from the vasa vasorum is seen throughout the pathogenesis of atherosclerosis (Sedding et al., 2018). Phenotypic changes witnessed during plaque formation are propagated by HIF. Hypoxia drives atherosclerosis, including the rupture of the most vulnerable part of the plaque (Gao et al., 2012; de Vries and Quax, 2016).
We observe a similar sequence of events occurring in chronic eye diseases such as age-related macular degeneration (AMD) and diabetic retinopathy (DR), where the retina suffers from hypoxia. HIF is activated and despite extensive angiogenesis, the retinal cells remain ischemic, followed by a paradoxical elevation in ROS (mitochondrial ROS are central mediators of hypoxic signaling) (Hamanaka and Chandel, 2009). Although AMD and DR occur from different etiologies, the quality of the new blood vessels are structurally defective, and the oxygenation does not improve. Adaptive remodeling and the reprogramming that follow are needed for survival (Sene et al., 2015; Cheng et al., 2017).
Other major chronic diseases such as cancer share a familiar pattern of pathologic angiogenesis. Architectural integrity of the blood vessels exhibit a disorderly, porous, and tortuous quality (Nagy et al., 2009). Although there is rampant angiogenesis, the framework of the new blood vessels is in complete disarray, and the tumor microenvironment remains hypoxic. Cancer cells enlist the Warburg effect and recruit glucose transporters to produce an ample amount of energy. They are well-adapted to flourish in a low oxygen environment, evading both the immune system and anti-cancer treatment, shielded by these aberrant blood vessels (Nishida et al., 2006; Liu et al., 2021; Rodriguez et al., 2021).
HIF is a transcriptional messenger that identifies the hypoxic regions. The underlying problem is hypoxia and not the activation of HIF, not the elevation of VEGF, and certainly not angiogenesis. To avert a pathologic course, a good blood flow and a stable oxygen source is imperative (Fraisl et al., 2009; Chen et al., 2020).
A novel way of oxygen delivery without hemoglobin has materialized in the form of oxygen microbubbles (OMBs). Oxygen microbubbles or nano-bubbles can carry oxygen without being bound to the hemoglobin. OMBs can be synthesized by mixing lipid solution and bubbling in oxygen. Microbubbles will form with phospholipid shells and many oxygen trapped inside (Kheir et al., 2012; Thomson et al., 2014). When injected into the blood vessel, they can travel safely throughout the body. As OMBs reach the vicinity of the cells, oxygen molecules can diffuse using the concentration gradient, improving cellular oxygen level. Hypoxia can be reversed and subsequently, HIF will be downregulated, and pathologic angiogenesis interrupted. Furthermore, these OMBs have been shown to normalize the structure of dysfunctional blood vessels that are often found in pathologic settings (Khan et al., 2018; Ho et al., 2019). Chronic diseases suffer from damaged blood vessels (especially to the capillaries) and cellular hypoxia, forcing cells to adopt a new phenotype. Once the circulatory competency is reestablished, hemoglobin bound oxygen is able to reach the cells. By replenishing the oxygen supply, the equilibrium shifts, cellular plasticity can be halted or reversed, and the phenotype reinstated.
Conclusion and future direction
We arrived at this concept utilizing data from the most recent literature but applying a different set of theoretical models. Based on the evidence of these disease processes discussed above, our theories could be tested in hypoxic cells of animal models both in vivo and in vitro by examining cellular metaplasia, gene expression, progression of diseases, tumorigenesis and so on. OMBs could be tested in animal and in human studies. If established, the therapeutic benefit of oxygen delivery could offer an alternative or adjuvant treatment for disease and health.
Future research could focus on disease process in the central nervous system (CNS). The CNS operates under lower oxygen tension, and due to the blood brain barrier, plastic changes are more complex. Neurodegenerative diseases undergo cellular changes in response to injury and drop in oxygen level. The mechanism for the most common neurodegenerative disease like Alzheimer’s disease remains elusive. Perhaps our model may help elucidate the disease processes.
Author contributions
EK conception of the hypothesis and theories, drafted the first version of the manuscript, reviewed all the citation, editing, and responsible for the manuscript. JF literature research and review. XM editing the manuscript, verifying references and citations, organizing and formatting for submission. All authors contributed to the article and approved the submitted version.
Conflict of interest
The authors declare that the research was conducted in the absence of any commercial or financial relationships that could be construed as a potential conflict of interest.
Publisher’s note
All claims expressed in this article are solely those of the authors and do not necessarily represent those of their affiliated organizations, or those of the publisher, the editors and the reviewers. Any product that may be evaluated in this article, or claim that may be made by its manufacturer, is not guaranteed or endorsed by the publisher.
References
Aguilar-Cazares, D., Chavez-Dominguez, R., Carlos-Reyes, A., Lopez-Camarillo, C., Hernadez de la Cruz, O. N., and Lopez-Gonzalez, J. S. (2019). Contribution of angiogenesis to inflammation and cancer. Front. Oncol. 9:1399. doi: 10.3389/fonc.2019.01399
al-Ani, A., Toms, D., Kondro, D., Thundathil, J., Yu, Y., and Ungrin, M. (2018). Oxygenation in cell culture: critical parameters for reproducibility are routinely not reported. PLoS One 13:e0204269. doi: 10.1371/journal.pone.0204269
Allahverdian, S., Chaabane, C., Boukais, K., Francis, G. A., and Bochaton-Piallat, M. L. (2018). Smooth muscle cell fate and plasticity in atherosclerosis. Cardiovasc. Res. 114, 540–550. doi: 10.1093/cvr/cvy022
Balogh, E., Tóth, A., Méhes, G., Trencsényi, G., Paragh, G., and Jeney, V. (2019). Hypoxia triggers Osteochondrogenic differentiation of vascular smooth muscle cells in an HIF-1 (hypoxia-inducible factor 1)-dependent and reactive oxygen species-dependent manner. Arterioscler. Thromb. Vasc. Biol. 39, 1088–1099. doi: 10.1161/ATVBAHA.119.312509
Baugh, L. R., and Day, T. (2020). Nongenetic inheritance and multigenerational plasticity in the nematode C. elegans. eLife 9:e58498. doi: 10.7554/eLife.58498
Bensellam, M., Jonas, J. C., and Laybutt, D. R. (2018). Mechanisms of β-cell dedifferentiation in diabetes: recent findings and future research directions. J. Endocrinol. 236, R109–r143. doi: 10.1530/JOE-17-0516
Burganova, G., Bridges, C., Thorn, P., and Landsman, L. (2021). The role of vascular cells in pancreatic Beta-cell function. Front. Endocrinol. 12:667170. doi: 10.3389/fendo.2021.667170
Buss, L. W. (1987). The evolution of individuality Princeton, NJ: Princeton University Press. xv, 201.
Camaré, C., Pucelle, M., Nègre-Salvayre, A., and Salvayre, R. (2017). Angiogenesis in the atherosclerotic plaque. Redox Biol. 12, 18–34. doi: 10.1016/j.redox.2017.01.007
Carvalho, J. (2020). Cell reversal from a differentiated to a stem-like state at cancer initiation. Front. Oncol. :10. doi: 10.3389/fonc.2020.00541
Cerf, M. E. (2013). Beta cell dysfunction and insulin resistance. Front. Endocrinol. (Lausanne) 4:37.
Chen, P.-S., Chiu, W. T., Hsu, P. L., Lin, S. C., Peng, I. C., Wang, C. Y., et al. (2020). Pathophysiological implications of hypoxia in human diseases. J. Biomed. Sci. 27:63. doi: 10.1186/s12929-020-00658-7
Chen, Y., Xu, H., Shi, Q., Gu, M., Wan, X., Chen, Q., et al. (2019). Hypoxia-inducible factor 1α (HIF-1α) mediates the epithelial-mesenchymal transition in benign prostatic hyperplasia. Int. J. Clin. Exp. Pathol. 12, 295–304.
Cheng, L., Yu, H., Yan, N., Lai, K., and Xiang, M. (2017). Hypoxia-inducible factor-1α target genes contribute to retinal neuroprotection. Front. Cell. Neurosci. 11:20. doi: 10.3389/fncel.2017.00020
Choudhry, H., and Harris, A. L. (2018). Advances in hypoxia-inducible factor biology. Cell Metab. 27, 281–298. doi: 10.1016/j.cmet.2017.10.005
Corl, A., Bi, K., Luke, C., Challa, A. S., Stern, A. J., Sinervo, B., et al. (2018). The genetic basis of adaptation following plastic changes in coloration in a novel environment. Curr. Biol. 28, 2970–2977.e7. doi: 10.1016/j.cub.2018.06.075
Cuypers, T. D., Rutten, J. P., and Hogeweg, P. (2017). Evolution of evolvability and phenotypic plasticity in virtual cells. BMC Evol. Biol. 17:60. doi: 10.1186/s12862-017-0918-y
de Fano, M., Bartolini, D., Tortoioli, C., Vermigli, C., Malara, M., Galli, F., et al. (2022). Adipose tissue plasticity in response to pathophysiological cues: a connecting link between obesity and its associated comorbidities. Int. J. Mol. Sci. 23:5511. doi: 10.3390/ijms23105511
de Vries, M. R., and Quax, P. H. (2016). Plaque angiogenesis and its relation to inflammation and atherosclerotic plaque destabilization. Curr. Opin. Lipidol. 27, 499–506. doi: 10.1097/MOL.0000000000000339
DeBerardinis, R. J., and Chandel, N. S. (2020). We need to talk about the Warburg effect. Nat. Metab. 2, 127–129. doi: 10.1038/s42255-020-0172-2
Emami Nejad, A., Najafgholian, S., Rostami, A., Sistani, A., Shojaeifar, S., Esparvarinha, M., et al. (2021). The role of hypoxia in the tumor microenvironment and development of cancer stem cell: a novel approach to developing treatment. Cancer Cell Int. 21:62. doi: 10.1186/s12935-020-01719-5
Fanelli, G. N., Naccarato, A. G., and Scatena, C. (2020). Recent advances in cancer plasticity: cellular mechanisms, surveillance strategies, and therapeutic optimization. Front. Oncol. 10:10. doi: 10.3389/fonc.2020.00569
Felmeden, D. C., Blann, A. D., and Lip, G. Y. (2003). Angiogenesis: basic pathophysiology and implications for disease. Eur. Heart J. 24, 586–603. doi: 10.1016/S0195-668X(02)00635-8
Feng, Y., Shen, Y., and Zhang, H. (2012). Molecular mechanism of remodeling of autologous artery graft interposed to vein in rabbit. Anat Rec. (Hoboken) 295, 432–437. doi: 10.1002/ar.21536
Fraisl, P., Mazzone, M., Schmidt, T., and Carmeliet, P. (2009). Regulation of angiogenesis by oxygen and metabolism. Dev. Cell 16, 167–179. doi: 10.1016/j.devcel.2009.01.003
Furman, D., Campisi, J., Verdin, E., Carrera-Bastos, P., Targ, S., Franceschi, C., et al. (2019). Chronic inflammation in the etiology of disease across the life span. Nat. Med. 25, 1822–1832. doi: 10.1038/s41591-019-0675-0
Fusco, G., and Minelli, A. (2010). Phenotypic plasticity in development and evolution: facts and concepts. Philos. Trans. R. Soc. B: Biol. Sci. 365, 547–556. doi: 10.1098/rstb.2009.0267
Galliot, B., and Ghila, L. (2010). Cell plasticity in homeostasis and regeneration. Mol. Reprod. Dev. 77, 837–855. doi: 10.1002/mrd.21206
Gao, L., Chen, Q., Zhou, X., and Fan, L. (2012). The role of hypoxia-inducible factor 1 in atherosclerosis. J. Clin. Pathol. 65, 872–876. doi: 10.1136/jclinpath-2012-200828
Gerber, P. A., and Rutter, G. A. (2017). The role of oxidative stress and hypoxia in pancreatic Beta-cell dysfunction in diabetes mellitus. Antioxid. Redox Signal. 26, 501–518. doi: 10.1089/ars.2016.6755
Goldenring, J. R., and Mills, J. C. (2022). Cellular plasticity, reprogramming, and regeneration: metaplasia in the stomach and beyond. Gastroenterology 162, 415–430. doi: 10.1053/j.gastro.2021.10.036
Granger, D. N., and Kubes, P. (1994). The microcirculation and inflammation: modulation of leukocyte-endothelial cell adhesion. J. Leukoc. Biol. 55, 662–675. doi: 10.1002/jlb.55.5.662
Grayson, W. L., Zhao, F., Izadpanah, R., Bunnell, B., and Ma, T. (2006). Effects of hypoxia on human mesenchymal stem cell expansion and plasticity in 3D constructs. J. Cell. Physiol. 207, 331–339. doi: 10.1002/jcp.20571
Griffiths, E. A., Pritchard, S. A., McGrath, S. M., Valentine, H. R., Price, P. M., Welch, I. M., et al. (2007). Increasing expression of hypoxia-inducible proteins in the Barrett's metaplasia-dysplasia-adenocarcinoma sequence. Br. J. Cancer 96, 1377–1383. doi: 10.1038/sj.bjc.6603744
Grimont, A., Leach, S. D., and Chandwani, R. (2022). Uncertain beginnings: acinar and ductal cell plasticity in the development of pancreatic cancer. Cell. Mol. Gastroenterol. Hepatol. 13, 369–382. doi: 10.1016/j.jcmgh.2021.07.014
Gunton, J. E. (2020). Hypoxia-inducible factors and diabetes. J. Clin. Invest. 130, 5063–5073. doi: 10.1172/JCI137556
Hamanaka, R. B., and Chandel, N. S. (2009). Reactive Oxygen Species regulate Hypoxic signaling. Curr. Opin. Cell Biol. 21, 894–899.
Hashimoto, T., and Shibasaki, F. (2015). Hypoxia-inducible factor as an angiogenic master switch. Front. Pediatr. 3:33. doi: 10.3389/fped.2015.00033
Hedges, S. B., Blair, J. E., Venturi, M. L., and Shoe, J. L. (2004). A molecular timescale of eukaryote evolution and the rise of complex multicellular life. BMC Evol. Biol. 4:2. doi: 10.1186/1471-2148-4-2
Heinis, M., Simon, M. T., Ilc, K., Mazure, N. M., Pouysségur, J., Scharfmann, R., et al. (2010). Oxygen tension regulates pancreatic beta-cell differentiation through hypoxia-inducible factor 1alpha. Diabetes 59, 662–669. doi: 10.2337/db09-0891
Helm, J., Enkemann, S. A., Coppola, D., Barthel, J. S., Kelley, S. T., and Yeatman, T. J. (2005). Dedifferentiation precedes invasion in the progression from Barrett's metaplasia to esophageal adenocarcinoma. Clin. Cancer Res. 11, 2478–2485. doi: 10.1158/1078-0432.CCR-04-1280
Ho, Y. J., Chu, S. W., Liao, E. C., Fan, C. H., Chan, H. L., Wei, K. C., et al. (2019). Normalization of tumor vasculature by oxygen microbubbles with ultrasound. Theranostics 9, 7370–7383. doi: 10.7150/thno.37750
Honzawa, N., and Fujimoto, K. (2021). The plasticity of pancreatic β-cells. Meta 11:4. doi: 10.3390/metabo11040218
Jessen, K. R., Mirsky, R., and Arthur-Farraj, P. (2015). The role of cell plasticity in tissue repair: adaptive cellular reprogramming. Dev. Cell 34, 613–620. doi: 10.1016/j.devcel.2015.09.005
John, R. M., and Rougeulle, C. (2018). Developmental epigenetics: phenotype and the flexible epigenome. Front. Cell Dev. Biol. 6:6. doi: 10.3389/fcell.2018.00130
Jung, J. H., Sosnowska, D., Weaver, J., Parson, H. K., Casellini, C. M., and Vinik, A. I. (2020). Expression of hypoxia-inducible factors in different stages of pancreatic tumor progression. Reports 3:30. doi: 10.3390/reports3040030
Katzir, Y., Stolovicki, E., Stern, S., and Braun, E. (2012). Cellular plasticity enables adaptation to unforeseen cell-cycle rewiring challenges. PLoS One 7:e45184. doi: 10.1371/journal.pone.0045184
Khan, M. S., Hwang, J., Seo, Y., Shin, K., Lee, K., Park, C., et al. (2018). Engineering oxygen nanobubbles for the effective reversal of hypoxia. Artif Cells Nanomed. Biotechnol. 46, S318–s327. doi: 10.1080/21691401.2018.1492420
Kheir, J. N., Scharp, L. A., Borden, M. A., Swanson, E. J., Loxley, A., Reese, J. H., et al. (2012). Oxygen gas-filled microparticles provide intravenous oxygen delivery. Sci. Transl. Med. 4:140ra88. doi: 10.1126/scitranslmed.3003679
Khin, P. P., Lee, J. H., and Jun, H. S. (2021). A brief review of the mechanisms of β-cell dedifferentiation in type 2 diabetes. Nutrients 13:1593. doi: 10.3390/nu13051593
Kotas, M. E., and Medzhitov, R. (2015). Homeostasis, inflammation, and disease susceptibility. Cells 160, 816–827. doi: 10.1016/j.cell.2015.02.010
Kumar, H., and Choi, D. K. (2015). Hypoxia inducible factor pathway and physiological adaptation: a cell survival pathway? Mediat. Inflamm. 2015:584758. doi: 10.1155/2015/584758
Kwiatkowski, D. P. (2005). How malaria has affected the human genome and what human genetics can teach us about malaria. Am. J. Hum. Genet. 77, 171–192. doi: 10.1086/432519
Lambert, J., and Jørgensen, H. F. (2021). Vascular smooth muscle cell phenotypic switching and plaque stability: a role for CHI3L1. Cardiovasc. Res. 117, 2691–2693. doi: 10.1093/cvr/cvab099
Laurent, S. (1995). Arterial wall hypertrophy and stiffness in essential hypertensive patients. Hypertension 26, 355–362. doi: 10.1161/01.HYP.26.2.355
Levy, B. I., Schiffrin, E. L., Mourad, J. J., Agostini, D., Vicaut, E., Safar, M. E., et al. (2008). Impaired tissue perfusion. Circulation 118, 968–976. doi: 10.1161/CIRCULATIONAHA.107.763730
Li, J. T., Wang, Y. P., Yin, M., and Lei, Q. Y. (2019). Metabolism remodeling in pancreatic ductal adenocarcinoma. Cell Stress 3, 361–368. doi: 10.15698/cst2019.12.205
Lin, Q., Kim, Y., Alarcon, R. M., and Yun, Z. (2008). Oxygen and cell fate decisions. Gene Regul Syst Bio 2, 43–51. doi: 10.4137/GRSB.S434
Ling, F. C., Khochfar, J., Baldus, S. E., Brabender, J., Drebber, U., Bollschweiler, E., et al. (2009). HIF-1alpha protein expression is associated with the environmental inflammatory reaction in Barrett's metaplasia. Dis. Esophagus 22, 694–699. doi: 10.1111/j.1442-2050.2009.00957.x
Liu, Y. (2007). Like father like son. A fresh review of the inheritance of acquired characteristics. EMBO Rep. 8, 798–803. doi: 10.1038/sj.embor.7401060
Liu, C., Jin, Y., and Fan, Z. (2021). The mechanism of Warburg effect-induced Chemoresistance in cancer. Front. Oncol. 11:11. doi: 10.3389/fonc.2021.698023
Liu, W., Shen, S. M., Zhao, X. Y., and Chen, G. Q. (2012). Targeted genes and interacting proteins of hypoxia inducible factor-1. Int. J. Biochem. Mol. Biol. 3, 165–178.
Maltepe, E., and Saugstad, O. D. (2009). Oxygen in health and disease: regulation of oxygen homeostasis-clinical implications. Pediatr. Res. 65, 261–268. doi: 10.1203/PDR.0b013e31818fc83f
Mamun, A. A., Hayashi, H., Yamamura, A., Nayeem, M. J., and Sato, M. (2020). Hypoxia induces the translocation of glucose transporter 1 to the plasma membrane in vascular endothelial cells. J. Physiol. Sci. 70:44. doi: 10.1186/s12576-020-00773-y
Martinucci, I., de Bortoli, N., Russo, S., Bertani, L., Furnari, M., Mokrowiecka, A., et al. (2016). Barrett's esophagus in 2016: from pathophysiology to treatment. World J. Gastrointest. Pharmacol. Ther. 7, 190–206. doi: 10.4292/wjgpt.v7.i2.190
Mezza, T., Cinti, F., Cefalo, C. M. A., Pontecorvi, A., Kulkarni, R. N., and Giaccari, A. (2019). β-Cell fate in human insulin resistance and type 2 diabetes: a perspective on islet plasticity. Diabetes 68, 1121–1129. doi: 10.2337/db18-0856
Migliorini, A., Bader, E., and Lickert, H. (2014). Islet cell plasticity and regeneration. Mol. Metab 3, 268–274. doi: 10.1016/j.molmet.2014.01.010
Milutinović, A., Šuput, D., and Zorc-Pleskovič, R. (2020). Pathogenesis of atherosclerosis in the tunica intima, media, and adventitia of coronary arteries: an updated review. Bosn. J. Basic Med. Sci. 20, 21–30. doi: 10.17305/bjbms.2019.4320
Modell, H., Cliff, W., Michael, J., McFarland, J., Wenderoth, M. P., and Wright, A. (2015). A physiologist's view of homeostasis. Adv. Physiol. Educ. 39, 259–266. doi: 10.1152/advan.00107.2015
Mullarky, E., and Cantley, L. C. (2015). “Diverting glycolysis to combat oxidative stress” in Innovative medicine: basic research and development. eds. K. Nakao, N. Minato, and S. Uemoto (Tokyo: Springer), 3–23.
Mulligan-Kehoe, M. J., and Simons, M. (2014). Vasa vasorum in normal and diseased arteries. Circulation 129, 2557–2566. doi: 10.1161/CIRCULATIONAHA.113.007189
Nagy, J. A., Chang, S. H., Dvorak, A. M., and Dvorak, H. F. (2009). Why are tumour blood vessels abnormal and why is it important to know? Br. J. Cancer 100, 865–869. doi: 10.1038/sj.bjc.6604929
Nishida, N., Yano, H., Nishida, T., Kamura, T., and Kojiro, M. (2006). Angiogenesis in cancer. Vasc. Health Risk Manag. 2, 213–219. doi: 10.2147/vhrm.2006.2.3.213
Orgogozo, V., Morizot, B., and Martin, A. (2015). The differential view of genotype–phenotype relationships. Front. Genet. 6:179. doi: 10.3389/fgene.2015.00179
Park, M. K., Ko, E. J., Jeon, K. Y., Kim, H., Jo, J. O., Baek, K. W., et al. (2019). Induction of angiogenesis by malarial infection through hypoxia dependent manner. Korean J. Parasitol. 57, 117–125. doi: 10.3347/kjp.2019.57.2.117
Que, J., Garman, K. S., Souza, R. F., and Spechler, S. J. (2019). Pathogenesis and cells of origin of Barrett's esophagus. Gastroenterology 157, 349–364.e1. doi: 10.1053/j.gastro.2019.03.072
Ramakrishnan, S., Anand, V., and Roy, S. (2014). Vascular endothelial growth factor signaling in hypoxia and inflammation. J. Neuroimmune Pharmacol. 9, 142–160. doi: 10.1007/s11481-014-9531-7
Rodriguez, D., Watts, D., Gaete, D., Sormendi, S., and Wielockx, B. (2021). Hypoxia pathway proteins and their impact on the blood vasculature. Int. J. Mol. Sci. 22:9191. doi: 10.3390/ijms22179191
Samanta, D., Prabhakar, N. R., and Semenza, G. L. (2017). Systems biology of oxygen homeostasis. Wiley Interdiscip. Rev. Syst. Biol. Med. 9. doi: 10.1002/wsbm.1382
Sato, Y., Inoue, M., Yoshizawa, T., and Yamagata, K. (2014). Moderate hypoxia induces β-cell dysfunction with HIF-1–independent gene expression changes. PLoS One 9:e114868. doi: 10.1371/journal.pone.0114868
Sedding, D. G., Boyle, E. C., Demandt, J. A. F., Sluimer, J. C., Dutzmann, J., Haverich, A., et al. (2018). Vasa Vasorum angiogenesis: key player in the initiation and progression of atherosclerosis and potential target for the treatment of cardiovascular disease. Front. Immunol. 9:706. doi: 10.3389/fimmu.2018.00706
Semenza, G. L. (2001). HIF-1 and mechanisms of hypoxia sensing. Curr. Opin. Cell Biol. 13, 167–171. doi: 10.1016/S0955-0674(00)00194-0
Semenza, G. L. (2012). Hypoxia-inducible factors in physiology and medicine. Cells 148, 399–408. doi: 10.1016/j.cell.2012.01.021
Sene, A., Chin-Yee, D., and Apte, R. S. (2015). Seeing through VEGF: innate and adaptive immunity in pathological angiogenesis in the eye. Trends Mol. Med. 21, 43–51. doi: 10.1016/j.molmed.2014.10.005
Solaini, G., Baracca, A., Lenaz, G., and Sgarbi, G. (2010). Hypoxia and mitochondrial oxidative metabolism. Biochim. Biophys. Acta 1797, 1171–1177. doi: 10.1016/j.bbabio.2010.02.011
Sorokin, V., Vickneson, K., Kofidis, T., Woo, C. C., Lin, X. Y., Foo, R., et al. (2020). Role of vascular smooth muscle cell plasticity and interactions in Vessel Wall inflammation. Front. Immunol. 11:599415. doi: 10.3389/fimmu.2020.599415
Souza, R. F. (2017). Reflux esophagitis and its role in the pathogenesis of Barrett's metaplasia. J. Gastroenterol. 52, 767–776. doi: 10.1007/s00535-017-1342-1
Speer, M. Y., Yang, H. Y., Brabb, T., Leaf, E., Look, A., Lin, W. L., et al. (2009). Smooth muscle cells give rise to osteochondrogenic precursors and chondrocytes in calcifying arteries. Circ. Res. 104, 733–741. doi: 10.1161/CIRCRESAHA.108.183053
Storz, P. (2017). Acinar cell plasticity and development of pancreatic ductal adenocarcinoma. Nat. Rev. Gastroenterol. Hepatol. 14, 296–304. doi: 10.1038/nrgastro.2017.12
Sun, H. J., Wu, Z. Y., Nie, X. W., and Bian, J. S. (2019). Role of endothelial dysfunction in cardiovascular diseases: the link between inflammation and hydrogen sulfide. Front. Pharmacol. 10:1568. doi: 10.3389/fphar.2019.01568
Tahergorabi, Z., and Khazaei, M. (2012). A review on angiogenesis and its assays. Iran. J. Basic Med. Sci. 15, 1110–1126.
Talchai, C., Xuan, S., Lin, H. V., Sussel, L., and Accili, D. (2012). Pancreatic β cell dedifferentiation as a mechanism of diabetic β cell failure. Cells 150, 1223–1234. doi: 10.1016/j.cell.2012.07.029
Tata, P. R., and Rajagopal, J. (2016). Cellular plasticity: 1712 to the present day. Curr. Opin. Cell Biol. 43, 46–54. doi: 10.1016/j.ceb.2016.07.005
Thannickal, V. J., and Fanburg, B. L. (2000). Reactive oxygen species in cell signaling. Am. J. Physiol. Lung Cell. Mol. Physiol. 279, L1005–L1028. doi: 10.1152/ajplung.2000.279.6.L1005
Thomson, L. M., Polizzotti, B. D., FX, M. G., and Kheir, J. N. (2014). Manufacture of concentrated, lipid-based oxygen microbubble emulsions by high shear homogenization and serial concentration. J. Vis. Exp. 87:51467. doi: 10.3791/51467
Vaudo, G., Schillaci, G., Evangelista, F., Pasqualini, L., Verdecchia, P., and Mannarino, E. (2000). Arterial wall thickening at different sites and its association with left ventricular hypertrophy in newly diagnosed essential hypertension. Am. J. Hypertens. 13, 324–331. doi: 10.1016/S0895-7061(99)00229-0
Wang, P., Wan, W. W., Xiong, S. L., Feng, H., and Wu, N. (2017). Cancer stem-like cells can be induced through dedifferentiation under hypoxic conditions in glioma, hepatoma and lung cancer. Cell Death Discov 3:16105. doi: 10.1038/cddiscovery.2016.105
Wund, M. A. (2012). Assessing the impacts of phenotypic plasticity on evolution. Integr. Comp. Biol. 52, 5–15. doi: 10.1093/icb/ics050
Yamada, Y., Haga, H., and Yamada, Y. (2014). Concise review: dedifferentiation meets cancer development: proof of concept for epigenetic cancer. Stem Cells Transl. Med. 3, 1182–1187. doi: 10.5966/sctm.2014-0090
Keywords: cellular plasticity, HIF, oxygen, trans-differentiation, dedifferentiation, reprogramming, genotypes, phenotypes
Citation: Kwan EK, Flowers J and Ming X (2023) Dynamic equilibrium of cellular plasticity: The origin of diseases. Front. Ecol. Evol. 11:1077902. doi: 10.3389/fevo.2023.1077902
Edited by:
Michael Schubert, UMR7009 Laboratoire de Biologie du Développement de Villefranche sur Mer, FranceReviewed by:
Neil W. Blackstone, Northern Illinois University, United StatesNathalie Oulhen, Brown University, United States
Copyright © 2023 Kwan, Flowers and Ming. This is an open-access article distributed under the terms of the Creative Commons Attribution License (CC BY). The use, distribution or reproduction in other forums is permitted, provided the original author(s) and the copyright owner(s) are credited and that the original publication in this journal is cited, in accordance with accepted academic practice. No use, distribution or reproduction is permitted which does not comply with these terms.
*Correspondence: Edmund K. Kwan, edmundkwanmd@yahoo.com