- 1Key Laboratory of Shale Gas and Geoengineering, Institute of Geology and Geophysics, Chinese Academy of Sciences, Beijing, China
- 2University of Chinese Academy of Sciences, Beijing, China
- 3Innovation Academy for Earth Science, Chinese Academy of Sciences, Beijing, China
- 4Guangzhou Marine Geological Survey, China Geological Survey, Guangzhou, China
- 5National Engineering Research Center of Gas Hydrate Exploration and Development, Guangzhou, China
- 6Center of Oil & Natural Gas Resource Exploration, China Geological Survey, Beijing, China
- 7School of Energy Resources, China University of Geosciences, Beijing, China
Natural gas hydrates as sustainable energy resources are inherently affected by mineral surfaces and confined spaces in reservoirs. However, the habits of hydrates in geological sediments are still an open question. In this work, we systemically studied the process of hydrate formation and dissociation in sediments from the Shenhu area of the South China Sea to examine the evolution of hydrate saturation and permeability in sediments and their relationship. Characterization of samples indicates that sediments of the Shenhu area are mainly composed of clay and fine sand grains and provide a large number of nanopores for hydrate accumulation. For in situ observations enabled by low-field nuclear magnetic resonance methods, the formation of hydrates shows a different kinetic behavior with an induction time compared to hydrate dissociation. Estimated by variations of hydrate saturation (%) over time, the rate of hydrate formation is around 12%/min, while the dissociation rate increases to 3%/min with the higher temperature. With the presence of hydrates, pore space and thus permeability of sediments decreased obviously by one and three orders of magnitude when the hydrate saturation is 20 and 45%, respectively. Compared to models with the assumption of grain-coating and pore-filling hydrates, the tendency of permeability evolution from NMR measurements is between fitted lines from models. It highlights that the existing models considering a single pattern of hydrate growth cannot precisely describe the relationship between permeability and hydrate saturation. Hybrid hydrate habits coexist in sediments resulting from heterogeneous pore structures and thus complex gas–water distributions.
Introduction
Gas hydrates are ice-like compounds composed of hydrogen-bound water molecules and low molecular weight gases that are stable at high pressure and low temperature. Natural gas hydrates (NGHs) are primarily distributed in permafrost and sediments and have attracted widespread attention as promising clean and sustainable energy resources (Sloan, 2003; Letcher, 2020). In particular, the Shenhu area, located in the center of the northern continental slope of the South China Sea (SCS), has become a key target area for marine gas hydrate production with abundant NGH resources (Li et al., 2019).
The Shenhu area in the Pearl River Mouth Basin has sea knolls, submarine canyons, and erosion toughs, and obviously, seabed topography fluctuates with a water depth of 1000∼1700 m (Wang et al., 2021). Previous studies indicate that the sea bottom of the Shenhu area has favorable conditions for hydrate formation with a temperature of 2∼4°C, a pressure larger than 10 MPa, and the abundant thermogenic gas or biogenic gas for hydrate growth (Su et al., 2016; Liang et al., 2017; Li et al., 2019; Zhang et al., 2019). Moreover, geological bodies including slope fans, slumping blocks, and contour current sedimentation systems are conducive to the accumulation of hydrates. However, a comprehensive understanding of the reservoir characteristics and their impact on hydrate habits in this area is still an open question.
It is increasingly evident that properties of porous media play a critical role in the formation, dissociation, and occurrence of gas hydrates under natural conditions. Compared with homogeneous nucleation processes, hydrate formation can be promoted by the addition of porous media with regard to the lower activation energy, more nucleation sites, and the reduced potential barrier of nucleation (Prasad, 2015; Ghaedi et al., 2016; Andres-Garcia et al., 2019). Previous studies have paid attention to the particle size, distribution, composition of porous media, and their impacts on hydrate habits. In particular, the particle size is a key factor affecting mass transfer performance, hydrate phase equilibrium conditions, and thus the induction time of hydrate formation in geomaterials (Qin et al., 2021). Some scholars believed that the enhancement of heat and mass transfer in porous media with a large particle size facilitates the system to achieve supersaturation and nucleation, which results in a shorter induction time of hydrate (Kumar Saw et al., 2015; Liu et al., 2015). However, others believed that the reduction of induction time can be observed in systems with a smaller particle size because of the larger nucleation area, the greater variation in capillary pressure, and the stronger nucleation (Liu et al., 2015; Siangsai et al., 2015; Heeschen et al., 2016). To date, there is no uniform boundary between small and large particle size, and no consistent conclusion on the effect of the particle size on hydrate nucleation and growth (Qin et al., 2021). In marine sediments, different particle sizes and their ratio result in complex pore structures, which may complicate the processes of hydrate formation and dissociation. Therefore, further experimental investigation in terms of coupled factors regarding pore structural properties on hydrate habits is highly needed.
With the hydrate formation or dissociation, permeability in hydrate-bearing sediments varies subjected to pore structure evolution and has a profound impact on heat and mass transfer and the subsequent dynamics of hydrates (Wang, 2019; Li et al., 2020; Song et al., 2020). Previous studies have demonstrated that the evolution of permeability is highly controlled by sediment features and the saturation and pore habits of hydrate crystals (Ren et al., 2020; Wang et al., 2021; Lu et al., 2021a; 2021b). There are a lot of experimental results about hydrate–saturation-dependent permeability in sediments, but the obvious discrepancy is observed as a result of differences in sample types, measurement methods, and experimental conditions (Kuang et al., 2020; Shen et al., 2020; Sun et al., 2021; Wen et al., 2021). Because of the absence of comparable experimental data, theoretical permeability models are developed to estimate the hydrate saturation and permeability relationship in sediments (Kleinberg, 2003; Mahabadi et al., 2016; Kossel et al., 2018). Most of the models are derived using simplified pore space, i.e., straight or tortuous capillary tubes, with the assumption of pore-filling or grain-coating hydrates in pores (Wang et al., 2021; Lei et al., 2022a). Apart from classic models, the hybrid model, modified Corey model, and cubic model are developed to address the hydrate-saturation-dependent permeability (Delli and Grozic, 2014; Lei et al., 2022a). Theoretical models usually include one or multiple uncertain parameters, which can be fitted by a variety of experimental results under different gas–water conditions. Various gas–water supply patterns complicate hydrate pore habits and limit the application of models for permeability prediction (Yin et al., 2018). In particular, gas hydrates mainly cement grain contacts under excess gas conditions, while tend to be as sediment frame components under excess water conditions (Delli and Grozic, 2014). Permeability usually decreases with increasing hydrate saturation; however, the permeability can vary several orders of magnitude regarding different sediment types and conditions even with identical hydrate saturation (Liang et al., 2011; Kang et al., 2016). A mechanistic understanding of permeability in hydrate-bearing sediments with regard to complex pore structure, gas–water conditions, and thus dynamic hydrate pore habits is still lacking.
In this work, we focus on the dynamic properties of hydrates during the process of formation and dissociation in sediments of the Shenhu area to examine the hydrate pore habits in the complex porous system. The mineral composition, particle size, and pore size distribution of sediment samples were analyzed to reveal pore structure features. The in situ observations of hydrate dynamics were enabled by the low-field nuclear magnetic resonance (LF-NMR) method, which provides a fast and nondestructive way of detecting hydrogen-bearing fluids and has been widely used to measure the water saturation and permeability in hydrate-bearing sediments (Ji et al., 2019; Kuang et al., 2020; Wen et al., 2021). A comparison of permeability results from experiments and theoretical models was performed to examine the dynamic evolution of hydrate saturation and pore morphology in sediments of the Shenhu area. Further insights on favorable conditions for hydrate formation and model applicability are expected to provide.
Materials and Methods
Samples
In this work, five representative sediment samples (i.e., W04-1 to W04-5) were acquired from the gas hydrate-bearing sediments in the Shenhu area (Figure 1). These samples were depressurized and stored after physical property measurements. For experiments on hydrate formation and dissociation, samples were initially evacuated and then saturated with distilled water at 12 MPa for 48 h.
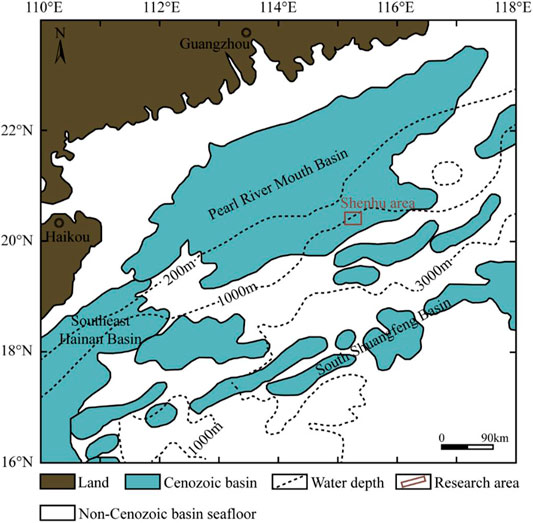
FIGURE 1. Geological map of the Shenhu area and location of sites drilled. (Modified after Li et al., 2019; Wang et al, 2021).
Pore Structure Analysis of Samples
The characterization of mineral composition, particle size, and pore size was performed in this work. Mineralogical data were analyzed using the X-ray diffraction (XRD) method. The grain size analysis of sediment samples for the nano- or micrograins was performed using the Zetasizer Nano ZS90 particle size analyzer and HELOS-OASIS particle size analyzer, respectively. The ASAP 2460 instrument was used for N2 adsorption measurements to determine pore size distributions of sediments.
In Situ Measurements of Gas Hydrates
Figure 2 shows a schematic of the LF-NMR monitoring apparatus, which was used to perform the in situ hydrate formation and dissociation experiments. The experimental apparatus system mainly comprises an LF-NMR analysis system, a core holder, a gas booster, a confining pressure tracking pump, a refrigeration cycle system, and the gas-liquid separator. The LF-NMR system is a Mini MR60 spectrometer with a magnetic field strength of 0.5 T and resonance frequency of 23 MHz. The confining pressure tracking pump is used to maintain certain confining pressure for CO2 injection. The refrigeration cycle system contains a circulation pump for cooling the fluorocarbon oil to control the experimental temperature.
In experiments, all the sediment samples were enclosed with heat-shrink tubing as a core with a length of 3.9 cm and a diameter of 2.5 cm. They were saturated with distilled water in the core holder, and then the CO2 was injected into the core holder with a constant flux until the water was displaced to specific content. The outlet valve of the core holder was then closed, and the CO2 supplement was enabled by a high-pressure pump to maintain the pore pressure at 4 MPa. During the process, some gases dissolved in water across gas–water interfaces. In situ NMR measurements started with decreasing temperature. Temperature controlled by the refrigeration cycle system decreased at a speed of 0.01°C/min from room temperature to 4°C and then kept stable until the end of hydrate formation. Then, the temperature increased at a speed of 0.02°C/min to 15°C for the hydrate dissociation. Gas permeability of the core was measured before and after the experiment. NMR measurements were performed using the Carr–Purcell–Meiboom–Gill (CPMG) pulse sequence during the experiment. Parameters of the CPMG pulse sequence included the echo number of 18,000, echo spacing of 0.15 ms, and waiting time of 1,000 ms.
Calculation of Hydrate Saturation and Permeability
The cumulative value of NMR signal intensity can quantify the water content and volume fraction in porous media, and the transverse relaxation time (T2) of water, proportional to the surface-to-volume ratio (S/V), can be used to evaluate the pore structure (Ji et al., 2019). For CO2 hydrate (CO2·5.75 H2O) formation and dissociation, the volume of CO2 hydrate (Vh) can be calculated by water reduction as follows:
where
The evolution of permeability subject to hydrate saturation is estimated by experimental data and theoretical models. The SDR method is usually used to calculate permeability from NMR data. It is based on the measured T2 distribution being a pseudo-pore size distribution during hydrate formation or dissociation. Eqn. 2 is described as follows:
where K is the permeability, Φ is the porosity (%), and T2gm is the logarithmic mean of T2 distribution (mD). The parameter C in the model is estimated by NMR measurements with known porosity and permeability of samples.
Theoretical permeability models differ from each other by using various assumptions of hydrate pore habits. The parallel capillary model, Kozeny grain model, and Masuda model are used in this paper.
1) Parallel capillary model (Kleinberg, 2003).
This model describing porous media as a bundle of straight, parallel cylindrical capillaries was developed by Kleinberg (2003). In this model, hydrate forms in the center of cylindrical pores, uniformly coating the walls of capillary. With the increase in hydrate saturation, an annular flow path is left for fluid. For the hydrate coating capillary walls model, the permeability can be calculated using Eqn. 3:
where K0 is the permeability before hydrate formation and Sh is the hydrate saturation. If hydrate occurs in the center of the capillary model, Eqn. 4 is used:
2) Kozeny grain model (Spangenberg, 2001).
Spangberg (2001) assumed porous media as Kozeny grain models and hydrate distribution patterns of both coating the grains and occupying pore centers can be calculated. For the former pattern, the permeability is evaluated as shown in Eqn. 5:
where the saturation exponent n is 1.5 for 0 <
3) Masuda model.
Masuda model (Masuda et al., 1997) is commonly applied to interpret the relationship between permeability and hydrate saturation as Eqn. 7:
where N with regard to pore structure is the permeability decreasing index and changes from 2 to 15 (Kumar et al., 2010).
Pore Structure Characteristics of Sediments
Mineral Composition Analysis
The XRD analysis of sediment samples shows that the predominant minerals are quartz (30.9%∼37.0 wt. %), carbonate minerals (22.8%∼30.8 wt. %), and mica (13.6∼31.6 wt. %) and are followed by clay minerals. The lower contents of clay minerals were determined in this work compared to previous studies (Li et al., 2019; Wang et al., 2021), which reported the weight percent of clay minerals high to 40% for samples from the SCS.
Grain Size Analysis
The results of the grain size analysis show similar properties of particle size distributions for five sediment samples. The frequency curves of all the samples are unimodal (Figure 3) and negatively skewed, with peak values varying over a broad range of 20∼40 um (Figure 3B). In particular, the median grain size of W04-1, W04-2, W04-3, W04-4, and W04-5 is 5.47, 18.57, 17.09, 11.84, and 17.48 um, respectively. It indicates that the sediments from the SCS mainly comprise clay (< 4 μm) and fine sand grains (4∼64 μm). Particle size distributions of nanosized grains also highlight the grains of samples are very fine. The frequency curves are also unimodal with a range of 396∼1,106 nm, and peak values of curves vary from 600 to 800 nm (Figure 3A).
Pore Size Analysis
The N2 adsorption–desorption isotherms of five samples display similar patterns (Figure 4A). The adsorption isotherms increase slightly at a relative pressure (p/p0) <0.45, followed by a sharp increase when p/p0 is close to 1.0. The hysteresis loops of adsorption–desorption isotherms are indistinctive, which indicates the pore system of sediment samples is composed of cylindrical, wedge-shaped, or slit pores.
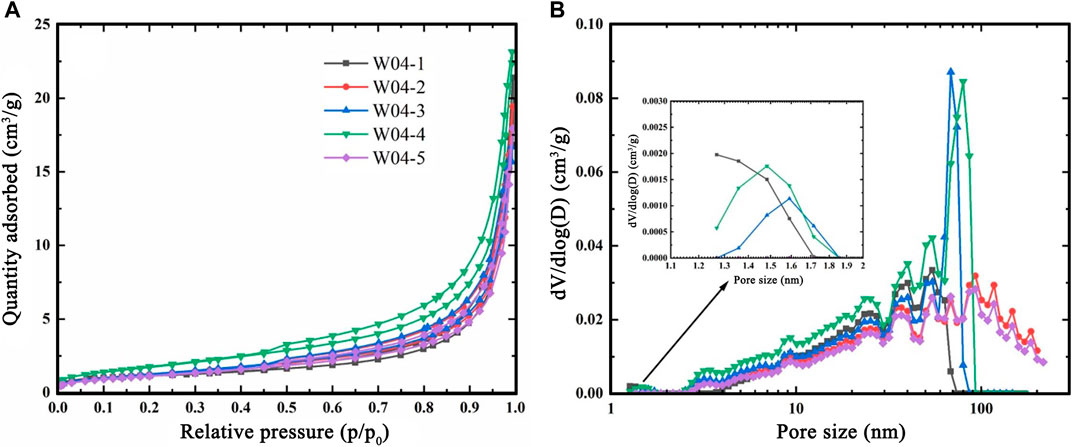
FIGURE 4. Adsorption and desorption isotherms (A) and pore size distributions (B) of sediment samples from N2 adsorption measurements.
Figure 4B shows pore size distributions of W04-1 to W04-5. For W04-1, W04-3, and W04-4, results of pore size with the bimodal distribution mainly range from 1.3 to 80 nm. In particular, the first peak value is 1.27, 1.59, and 1.48 nm, and the second peak value is 54.42, 68.50, and 79.87 nm for W04-1, W04-3, and W04-4, respectively. The pore size curves are unimodal with a peak value of 93.13 nm for W04-2 and W04-5. Based on pore size analysis, a large number of nanopores in these sediments can provide considerable space for hydrate accumulation.
Hydrate Dynamics Analysis and Permeability Evolution
Results of NMR Measurements
Figure 5 shows the procedure of the experiments with an increase and then a decrease in the temperature. The whole process can be divided into several periods in terms of the variation of hydrate saturation. For the period A-B, an induction time for hydrate formation is observed with the limited variation of hydrate. During period B-C, the pressure and temperature conditions are favorable for hydrate formation, and hydrate saturation increased rapidly up to 50%. The rate of hydrate formation is around 12%/min, estimated by variations of hydrate saturation (%) over time. The hydrate saturation is almost consistent within the period C-D resulting from the consumption of water and the limited contact between water and gas. After the D point, the temperature increases gradually and results in the dissociation of hydrates. During period D-E, the rate of dissociation gradually increases to 3%/min with the higher temperature. It is because the experimental condition is far away from the phase equilibrium condition, leading to a larger driving force for hydrate dissociation. At the end of the experiments, all the CO2 hydrates dissociate, and the hydrate saturation decreases to 0.
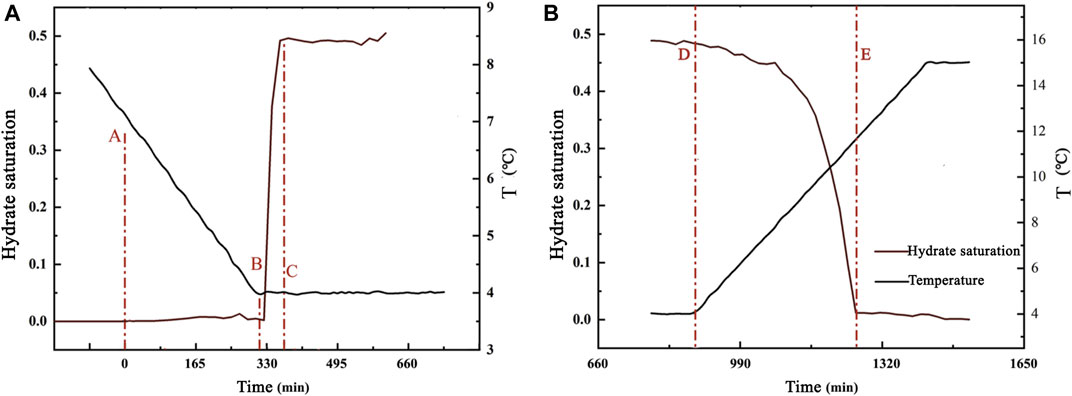
FIGURE 5. Variations in hydrate saturation during the process of gas hydrate formation (A) and dissociation (B).
Evolution of Pore Structure With Hydrate Dynamics
T2 distribution spectra of NMR provide insights into the pore size distribution of sediments and the change of pore space subjected to the hydrate formation or dissociation. As shown in Figure 6A, there are two distinct parts for the initial signal of sediment samples, i.e., Part 1 (0.2∼2 ms) and Part 2 (10∼300 ms), representing the complex pore structure with mixed grains. At the early stage of hydrate formation, there is an obvious decrease in Part 2, whereas there is a slight increase in the peak of Part 1. It indicates that the pore space of Part 2 is occupied by the formation of hydrate and leads to a faster relaxation time of the NMR signal. The peak area of Part 2 decreases 40% from 275 to 350 min with a preferential formation of hydrate in larger pores. Some scholars believed that the enhanced performance of heat and mass transfer makes it easier for large pore spaces to achieve nucleation (Qin et al., 2021). With the continuous increase of hydrate saturation, Part 2 became vanished, while Part 1 was still with a low peak at the end of hydrate formation. The phenomenon shows the movement of fluid is quite limited with the presence of hydrates, and thus the local hydrate formation is restrained due to the lack of water or gas.
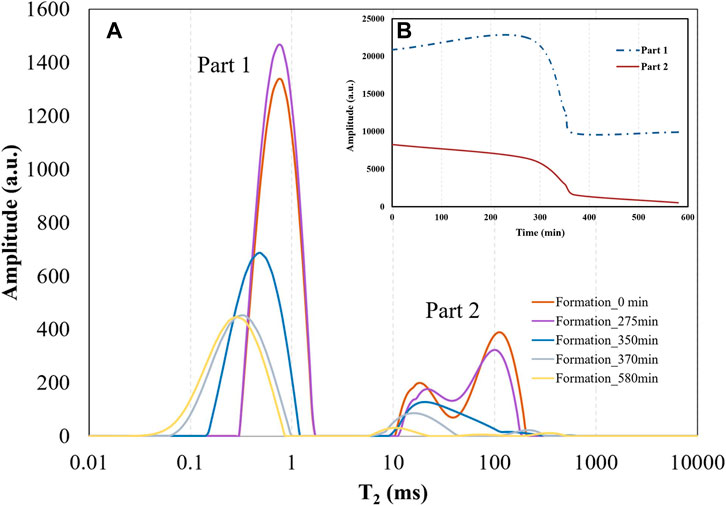
FIGURE 6. T2 distribution spectra of NMR (A) and cumulative signal of NMR (B) during gas hydrate formation.
An opposite tendency of NMR signal variation can be observed in the process of hydrate dissociation (Figure 7). The value and width of peaks increase with the hydrate dissociation. Compared to the process of formation, the increase of Part 2 is slightly later than that of Part 1. The rate of hydrate dissociation gradually increases in both Part 1 and Part 2 over time confirmed by the cumulative intensity of the NMR signal. A noticeable difference is shown between the T2 distribution spectra of the initial situation and the end of dissociation. Similar behaviors have been observed by Lei et al. (2022b). They analyzed microscopic pore characteristics and flowability of five samples from the Shenhu area, showing better connectivity in hydrate-bearing sediment samples after hydrate dissociation compared to samples from the underlying layer. It demonstrates that pore structure may be altered after the hydrate dynamics in both pore size and pore quantity.
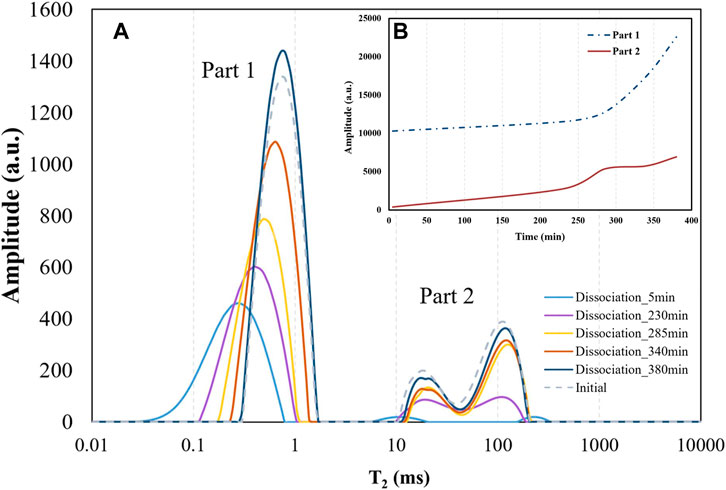
FIGURE 7. T2 distribution spectra of NMR (A) and cumulative signal of NMR (B) during gas hydrate dissociation.
Evolution of Permeability With Hydrate Dynamics
Hydrate saturation and permeability in the porous medium were determined by NMR measurements, and the tendency of permeability evolution subjected to the hydrate formation or dissociation is shown in Figure 8. With the increase of hydrate saturation, a remarkable decrease in permeability can be observed. It indicates that the permeability of porous media obviously decreases with occupying gas hydrates in pore spaces, and the evolution of permeability differs in terms of the hydrate quantity and distribution. In particular, an order of magnitude lower is in permeability with the hydrate saturation of 32%, while three orders of magnitude are lower with the hydrate saturation of 50% compared to the initial permeability.
By comparing permeability results obtained from experimental data and theory models, different tendencies of permeability evolution are shown in Figure 8. Generally, a sharper change of permeability is determined by experimental data at the early stage, while a gentler change at the middle stage is compared to other models. In particular, NMR results show a good agreement with the parallel capillary model (pore-filling) when the hydrate saturation is smaller than 0.1, indicating hydrates prefer to form in the pore central space. Compared to other patterns of hydrate distribution, e.g., a thin film of hydrate coating grain walls, the changes in the permeability are expected to be larger if hydrates occupy flow paths. When the hydrate saturation is larger than 0.3, permeability evolution can be especially captured by the Masuda model (n = 12). Some studies demonstrated that grain-coating hydrates appear to be more common than pore-filling ones with a low porosity and permeability (Lei et al., 2022a). Moreover, a drastic reduction in permeability may occur if formed hydrates plug the pore throats in addition to pore bodies, especially when a high hydrate saturation is in porous media. It is clear that the tendency of permeability evolution is differentiated induced by various patterns of hydrate growth in pores and is more complicated when hybrid patterns exist. In this work, most experimental permeability data points fall between fitted lines from different theoretical permeability models, i.e., the pore wall coating and pore center occupying models. It highlights that hybrid pore habits of hydrates affect the evolution of permeability in hydrate-bearing sediments of the Shenhu area, and a preponderant pattern of hydrate growth changes spatially and temporally.
During the process of hydrate formation and dissociation, pore structure characteristics of sediments influence the hydrate saturation and distribution, as well as gas–water conditions. Previous studies pointed out that various gas–water flow patterns result in excess-gas, excess-water, or dissolved-gas conditions for hydrate formation in sediments and have a profound impact on hydrate growth (Wang et al., 2021). In the excess-gas condition, a limited amount of water mainly cements hydrophilic grain surfaces or contacts, which results in grain-coating hydrates with gas diffusing into water layers. But when the ratio of gas to water is low, the formation of hydrate starts close to trapped gas bubbles or dissolved-gas regions, and gradually exhibits a pore-filling morphology. In this experiment, excess-gas, excess-water, and dissolved-gas conditions coexist in sediments, and gas–water ratios and distributions vary with hydrate dynamics (illustrated in Figure 8). Hydrates formed at gas–water interfaces can continuously grow to coat grains or occupy pore central spaces subjected to the specific gas–water condition. In this regard, a theoretical model assuming a single pattern of hydrate growth is expected to fail to predict the hydrate–saturation-dependent permeability in a geosystem in terms of heterogeneous pore structures and gas–water conditions.
Conclusions
Properties of porous media play a critical role in the formation, dissociation, and occurrence of gas hydrates under natural conditions. In a complex geological media, heterogeneous pore structure will largely complicate the scientific nature of hydrate dynamics during the process of formation and dissociation. This work performed in situ observations of pore structure evolution with regard to the changes in hydrate saturation by using LF-NMR measurements. The sediments from the Shenhu area of the SCS with the main components of quartz, calcite, and mica minerals, are composed of clay and fine grains. Nanopores with a range from several to tens of nanometers contribute to the pore space for hydrate growth and accumulation.
In such porous media, gas hydrates gradually form after an induction time; however, they immediately dissociate with an increasing temperature. Estimated by variations of hydrate saturation (%) over time, the rate of hydrate formation is around 12%/min, while the highest rate of dissociation is 3%/min. Because of the occupation of pore space with the hydrate growth, the permeability of sediments decreases obviously with an increase in hydrate saturation. However, the relationship between permeability and saturation is quite complex and cannot be described by theoretical models with a simple assumption of hydrate pore habits. When the saturation is from 20 to 45%, one to three orders of magnitude lower in permeability is determined by experiments, respectively. The tendency of permeability evolution compared to predicted outcomes from permeability models indicates coexistence of both pore-filling and grain-coating hydrate pore morphology in sediments of the Shenhu area. Moreover, heterogeneous pore structure complicates gas–water conditions in sediments, which leads to spatial and temporal variations in hydrate pore habits. For the improved understanding and predictive capability of hydrate–saturation-dependent permeability, coupled effects of porous media properties and evolution conditions have to be addressed in hydrate-bearing sediments.
Data Availability Statement
The original contributions presented in the study are included in the article/Supplementary Material, further inquiries can be directed to the corresponding author.
Author Contributions
QZ, YD, and HZ contributed to the conception and design of the study. LX organized the database. XQ performed the statistical analysis. QZ and HZ wrote the first draft of the manuscript. CL, SL, CM, and HB wrote sections of the manuscript. All authors contributed to manuscript revision, read, and approved the submitted version.
Funding
This research was supported by the Key Research Program of the Institute of Geology & Geophysics, CAS (no. IGGCAS-201903), the National Natural Science Foundation of China (No. 51991365), Guangdong Major Project of Basic and Applied Basic Research (No. 2020B0301030003), and China Geological Survey Project (No. DD20211350).
Conflict of Interest
The authors declare that the research was conducted in the absence of any commercial or financial relationships that could be construed as a potential conflict of interest.
Publisher’s Note
All claims expressed in this article are solely those of the authors and do not necessarily represent those of their affiliated organizations or those of the publisher, the editors, and the reviewers. Any product that may be evaluated in this article, or claim that may be made by its manufacturer, is not guaranteed or endorsed by the publisher.
Acknowledgments
The authors are grateful to the Editor and two reviewers for their critical and constructive reviews.
References
Andres-Garcia, E., Dikhtiarenko, A., Fauth, F., Silvestre-Albero, J., Ramos-Fernández, E. V., Gascon, J., et al. (2019). Methane Hydrates: Nucleation in Microporous Materials. Chem. Eng. J. 360, 569–576. doi:10.1016/j.cej.2018.11.216
Delli, M. L., and Grozic, J. L. H. (2014). Experimental Determination of Permeability of Porous media in the Presence of Gas Hydrates. J. Pet. Sci. Eng. 120, 1–9. doi:10.1016/j.petrol.2014.05.011
Ghaedi, H., Ayoub, M., Bhat, A. H., Mahmood, S. M., Akbari, S., and Murshid, G. (2016). The Effects of Salt, Particle and Pore Size on the Process of Carbon Dioxide Hydrate Formation: A Critical Review. AIP Conf. Proc. 1787, 060001. doi:10.1063/1.4968128
Heeschen, K. U., Schicks, J. M., and Oeltzschner, G. (2016). The Promoting Effect of Natural Sand on Methane Hydrate Formation: Grain Sizes and mineral Composition. Fuel 181, 139–147. doi:10.1016/j.fuel.2016.04.017
Ji, Y., Hou, J., Cui, G., Lu, N., Zhao, E., Liu, Y., et al. (2019). Experimental Study on Methane Hydrate Formation in a Partially Saturated sandstone Using Low-Field NMR Technique. Fuel 251, 82–90. doi:10.1016/j.fuel.2019.04.021
Kang, D. H., Yun, T. S., Kim, K. Y., and Jang, J. (2016). Effect of Hydrate Nucleation Mechanisms and Capillarity on Permeability Reduction in Granular Media. Geophysical Research Letters 43 (17), 9018–9025.
Kleinberg, R. L. (2003). Deep Sea NMR: Methane Hydrates Growth Habit in Porous media and its Relationship to Hydraulic Permeability, deposit Accumulation, and Submarine Slope Stability[J]. J. Geophys. Res. Solid Earth 108 (B10), 2508. doi:10.1029/2003jb002389
Kossel, E., Deusner, C., Bigalke, N., and Haeckel, M. (2018). The Dependence of Water Permeability in Quartz Sand on Gas Hydrate Saturation in the Pore Space. J. Geophys. Res. Solid Earth 123 (2), 1235–1251. doi:10.1002/2017jb014630
Kuang, Y., Zhang, L., Song, Y., Yang, L., and Zhao, J. (2020). Quantitative Determination of Pore‐structure Change and Permeability Estimation under Hydrate Phase Transition by NMR. AIChE J. 66 (4), e16859. doi:10.1002/aic.16859
Kumar, A., Maini, B., Bishnoi, P. R., Clarke, M., Zatsepina, O., and Srinivasan, S. (2010). Experimental Determination of Permeability in the Presence of Hydrates and its Effect on the Dissociation Characteristics of Gas Hydrates in Porous Media[J]. J. Pet. Sci. Eng. 70 (1/2), 114–122. doi:10.1016/j.petrol.2009.10.005
Kumar Saw, V., Udayabhanu, G., Mandal, A., and Laik, S. (2015). Methane Hydrate Formation and Dissociation in the Presence of Silica Sand and Bentonite Clay. Oil Gas Sci. Technol. - Rev. IFP Energies Nouvelles 70, 1087–1099. doi:10.2516/ogst/2013200
Lei, X., Yao, Y., Luo, W., and Wen, Z. (2022a). Permeability Change in Hydrate Bearing Sediments as a Function of Hydrate Saturation: A Theoretical and Experimental Study. J. Pet. Sci. Eng. 208, 109449. doi:10.1016/j.petrol.2021.109449
Lei, X., Yao, Y., Qin, X., Lu, C., Luo, W., Wen, Z., et al. (2022b). Pore Structure Changes Induced by Hydrate Dissociation: An Example of the Unconsolidated Clayey-Silty Hydrate Bearing Sediment Reservoir in the South China Sea. Mar. Geology. 443, 106689. doi:10.1016/j.margeo.2021.106689
Letcher, M. T. (Editor) (2020). Future Energy: Improved, Sustainable and Clean Options for Our planet[M] (Amsterdam, Netherlands: Elsevier), 111–131.
Liang, H., Song, Y., Chen, Y., and Liu, Y. (2011). The Measurement of Permeability of Porous Media With Methane Hydrate. Petroleum Science and Technology, 2011 29, 79–87. doi:10.1016/j.margeo.2021.106689
Liang, J. Q., Wei, J. G., Bigalke, N., Roberts, J., Schultheiss, P., Holland, M., et al. (2017). “Laboratory Quantification of Geomechanical Properties of Hydrate-Bearing Sediments in the Shenhu Area of the South China Sea at In-Situ Conditions,” in Proceedings of the 9th International Conference on Gas Hydrates, Denver, 30.
Li, J., Lu, J. a., Kang, D., Ning, F., Lu, H., Kuang, Z., et al. (2019). Lithological Characteristics and Hydrocarbon Gas Sources of Gas Hydrate-Bearing Sediments in the Shenhu Area, South China Sea: Implications from the W01B and W02B Sites. Mar. Geology. 408, 36–47. doi:10.1016/j.margeo.2018.10.013
Li, Z.-d., Tian, X., Li, Z., Xu, J.-z., Zhang, H.-x., and Wang, D.-j. (2020). Experimental Study on Growth Characteristics of Pore-Scale Methane Hydrate. Energ. Rep. 6, 933–943. doi:10.1016/j.egyr.2020.04.017
Liu, W., Wang, S., Yang, M., Song, Y., Wang, S., and Zhao, J. (2015). Investigation of the Induction Time for THF Hydrate Formation in Porous media. J. Nat. Gas Sci. Eng. 24, 357–364. doi:10.1016/j.jngse.2015.03.030
Lu, C., Qin, X., Mao, W., Ma, C., Geng, L., Yu, L., et al. (2021b). Experimental Study on the Propagation Characteristics of Hydraulic Fracture in Clayey-Silt Sediments. Geofluids 2021, 6698649. doi:10.1155/2021/6698649
Lu, C., Qin, X., Yu, L., Geng, L., Mao, W., Bian, H., et al. (2021a). The Characteristics of Gas-Water Two-phase Radial Flow in Clay-Silt Sediment and Effects on Hydrate Production. Geofluids 2021 (3), 1–14. doi:10.1155/2021/6623802
Masuda, Y. S., Naganawa, S., Ando, S., and Sato, K. (1997). “Numerical Calculation of Gas Production Performance From Reservoirs Containing Natural Gas Hydrates; Paper SPE 38291,” in Proceedings, Western Regional Meeting, June, 25–27.
Prasad, P. S. R. (2015). Methane Hydrate Formation and Dissociation in the Presence of Hollow Silica. J. Chem. Eng. Data 60, 304–310. doi:10.1021/je500597r
Qin, Y., Pan, Z., Liu, Z., Shang, L., and Shang, L. (2021). Influence of the Particle Size of Porous Media on the Formation of Natural Gas Hydrate: A Review[J]. Energy & Fuels 35 (15), 11640. doi:10.1021/acs.energyfuels.1c00936
Ren, X., Guo, Z., Ning, F., and Ma, S. (2020). Permeability of Hydrate-Bearing Sediments. Earth-Science Rev. 202, 103100. doi:10.1016/j.earscirev.2020.103100
Shen, P., Li, G., Li, B., and Li, X. (2020). Coupling Effect of Porosity and Hydrate Saturation on the Permeability of Methane Hydrate-Bearing Sediments. Fuel 269, 117425. doi:10.1016/j.fuel.2020.117425
Siangsai, A., Rangsunvigit, P., Kitiyanan, B., Kulprathipanja, S., and Linga, P. (2015). Investigation on the Roles of Activated Carbon Particle Sizes on Methane Hydrate Formation and Dissociation . Chemical Engineering Science 126, 383–389.
Sloan, E. D. (2003). Fundamental Principles and Applications of Natural Gas Hydrates. Nature 426 (6964), 353–359. doi:10.1038/nature02135
Song, G., Li, Y., and Sum, A. K. (2020). Characterization of the Coupling between Gas Hydrate Formation and Multiphase Flow Conditions. J. Nat. Gas Sci. Eng. 83, 103567. doi:10.1016/j.jngse.2020.103567
Spangenberg, E. (2001). Modeling of the Influence of Gas Hydrate Content on the Electrical Properties of Porous Sediments. J. Geophys. Res. 106 (B4), 6535–6548. doi:10.1029/2000jb900434
Su, M., Yang, R., Wang, H., Sha, Z., Liang, J., Wu, N., et al. (2016). Gas Hydrates Distribution in the Shenhu Area, Northern South China Sea: Comparisons Between the Eight Drilling Sites With Gas-Hydrate Petroleum System. Geol. Acta. 14 (2), 79–100.
Sun, J., Dong, H., Arif, M., Yu, L., Zhang, Y., Golsanami, N., et al. (2021). Influence of Pore Structural Properties on Gas Hydrate Saturation and Permeability: a Coupled Pore-Scale Modelling and X-ray Computed Tomography Method. J. Nat. Gas Sci. Eng. 88, 103805. doi:10.1016/j.jngse.2021.103805
Wang, P. (2019). Hydrate Formation Characteristic under Gas Migration in Porous medium[D]. Dalian, China: Dalian University of Technology.
Wang, Q., Chen, X., Zhang, L., Wang, Z., Wang, D., and Dai, S. (2021). An Analytical Model for the Permeability in Hydrate‐bearing Sediments Considering the Dynamic Evolution of Hydrate Saturation and Pore Morphology. Geophys. Res. Lett. 48 (8), e2021GL093397. doi:10.1029/2021gl093397
Wen, Z., Yao, Y., Luo, W., and Lei, X. (2021). Memory Effect of CO2-hydrate Formation in Porous media. Fuel 299, 120922. doi:10.1016/j.fuel.2021.120922
Yin, Z., Moridis, G., Chong, Z. R., Tan, H. K., and Linga, P. (2018). Numerical Analysis of Experimental Studies of Methane Hydrate Dissociation Induced by Depressurization in a sandy Porous Medium. Appl. Energ. 230, 444–459. doi:10.1016/j.apenergy.2018.08.115
Keywords: gas hydrate, sediment, formation and dissociation, nuclear magnetic resonance, pore habit
Citation: Zhang Q, Qin X, Zhang H, Dong Y, Lu C, Li S, Xiao L, Ma C and Bian H (2022) Experimental Insights Into the In Situ Formation and Dissociation of Gas Hydrate in Sediments of Shenhu, South China Sea. Front. Earth Sci. 10:882701. doi: 10.3389/feart.2022.882701
Received: 24 February 2022; Accepted: 04 April 2022;
Published: 11 May 2022.
Edited by:
Zhifeng Wan, Sun Yat-sen University, ChinaReviewed by:
Chao Fu, China National Offshore Oil Corporation (China), ChinaYanbin Yao, China University of Geosciences, China
Copyright © 2022 Zhang, Qin, Zhang, Dong, Lu, Li, Xiao, Ma and Bian. This is an open-access article distributed under the terms of the Creative Commons Attribution License (CC BY). The use, distribution or reproduction in other forums is permitted, provided the original author(s) and the copyright owner(s) are credited and that the original publication in this journal is cited, in accordance with accepted academic practice. No use, distribution or reproduction is permitted which does not comply with these terms.
*Correspondence: Yanhui Dong, ZG9uZ3loQG1haWwuaWdnY2FzLmFjLmNu