- 1Center for Space Plasma Physics, Space Science Institute, Boulder, CO, United States
- 2Los Alamos National Laboratory, Theoretical Division, Los Alamos, NM, United States
Planned active space experiments and ideas for future active space experiments are reviewed. Three active experiments being readied are DSX (Demonstration and Space eXperiments), SMART (Space Measurement of Rocket-released Turbulence), and BeamPIE (Beam Plasma Interaction Experiment). Ideas for future experiments include relativistic-electron-beam experiments for magnetic-field-line tracing, relativistic-electron-beam experiments to probe the middle atmosphere, plasma-wave launching using superparamagnetic-nanoparticle amplification of magnetic fields, the heavy-ion mass loading of collisionless magnetic-field-line reconnection, the use of electrostatically charged tethers to pitch-angle scatter radiation-belt particles, cold plasma releases to modify magnetospheric plasma physics, and neutral-gas releases to enhance neutral-particle imaging of the magnetosphere. Technologies that are being developed to enable future space active experiments are reviewed: this includes the development of compact relativistic accelerators, superparamagnetic particle amplified antennae, CubeSats, and a new understanding of how to control dynamic spacecraft charging. New capabilities to use laboratory facilities to design space active experiments as well as new computer-simulation capabilities to design and understand space active experiments are reviewed.
1. Introduction
Space active experiments are experiments that deliberately perturb the space environment in ways that can yield new information about the environment. They offer unique ways to gather scientific information, to study the interaction between space platforms and the space environment, and to perform space engineering. Active experiments can be used to study ionospheric physics, magnetosphere-ionosphere coupling, cometary physics, and magnetospheric plasma waves. Importantly, some experiments can only be performed in space. Space-based plasma-physics and plasma-astrophysics experiments can uniquely address the physics of large-scale plasmas, long-range coupling, and truly collisionless physical processes. In general, particle distribution functions can be obtained with more accuracy and less perturbation in space experiments than in laboratory plasma experiments. Besides scientific exploration, active experiments also support national security. For instance, a motivation of future space engineering comes in the design of active experiments for radiation-belt remediation, whereby an enhanced radiation belt environment is rapidly weakened by means of an external forcing. For scientific and engineering experiments in space, there will be needs for other space experiments to gain understanding of the interaction of those scientific and space-engineering platforms with the space environment.
There has been a rich history of active experiments in space (c.f. Grandal, 1982; Winckler, 1992; Raitt, 1995; Unan and Rietveld, 1995; James et al., 1998; Haerendel, 2018; Pongratz, 2018; Prech et al., 2018; Mishin, 2019; Winske et al., 2019 for reviews). These past experiments have involved electron and ion beams, plasma releases, chemical releases, tethers, antennae, and nuclear detonations. They span several decades, starting from high-altitude nuclear detonations in the late fifties to the plasma and chemical release experiments of the mid-nineties. In more recent years, the active-experiments program has changed, focusing on ground-based modification of the ionosphere by intense electromagnetic waves from facilities like HAARP (High frequency Active Auroral Research Program) and Arecibo.
At the “Active Experiments in Space: Past, Present, and Future” workshop in September 2017 in Santa Fe, New Mexico (Delzanno and Borovsky, 2018), several planned and proposed space active-experiment missions were discussed: these and other future missions are described in sections 2 and 3 of this report (Note that, in this paper, we only focus only on space-based active experiments: We do not review ground-based ionospheric modification experiments, but we acknowledge that these experiments are and will remain a very important component of the overall active-experiments program.). Among the advantages that future space active experiments will have over past active experiments are (1) better diagnostics, (2) newer technologies, and (3) better planning via modern computer simulations. These aspects are discussed in section 4, while conclusions are drawn in section 5. Following the “mandate” from the Santa Fe workshop, the goal of this paper is to demonstrate the importance and uniqueness of space active experiments and to generate increased enthusiasm toward an area that, fostered by many new innovations, can tremendously improve our understanding of the near-Earth environment.
At the Santa Fe workshop, there was also an overwhelming call to pass the knowledge and capabilities of active space experiments on from the older generation to newer scientists.
2. Planned Experiments
Three interesting active experiments (DSX, BeamPIE and SMART) are planned in the next few years and their objectives are briefly reviewed here. Note that all three experiments have a common objective to investigate wave-generation processes in space and this fits into the broader picture of how artificially-injected electromagnetic waves could be used for radiation-belt remediation (e.g., Inan et al., 2003; Dupont, 2004) or for communication.
2.1. The DSX Dipole Antenna
The Demonstration and Science eXperiments (DSX) of the Air Force (Scherbarth et al., 2009) is currently scheduled for launch by the summer of 2019 aboard the Space-X Falcon Heavy. With an orbit of 6000 × 12000 km, 42 degrees inclination, it will explore the Medium Earth Orbit (MEO) environment and particularly the slot region of the electron radiation belts. DSX carries an 80-m long dipole antenna, which will be the largest, unmanned, self-supporting structure ever deployed in space, and a comprehensive suite of space environment sensors. Its primary science objective is to study Very Low Frequency (VLF) wave transmission in MEO, including the injected VLF power by antennae in space and the interaction of VLF waves with the local particles of the environment. In this regard, DSX will work in conjunction with the VLF and Particle Mapper (VPM) nanosat mission in Low Earth Orbit (LEO), which will act as a far-field probe for DSX. Conjunctions with other spacecraft and ground stations will also be pursued. The secondary science objectives are (1) to map the local MEO radiation and plasma environment and (2) collect data to understand environmental effects and the degradation of selected spacecraft electronics and materials.
2.2. The Beam-PIE Cerenkov Wave Emission
The Beam Plasma Interaction Experiment (Beam-PIE) is a suborbital rocket experiment funded by NASA and led by Los Alamos National Laboratory. Its launch is planned for the spring/summer of 2020 from Poker Flat, Alaska. Beam-PIE is a mother-daughter system (see Figure 1), where the mother rocket will carry a new, compact electron accelerator technology driven by high-electron-mobility transistors. The accelerator is pulsed, designed to provide tens mA of current and energies up to 54 keV. The daughter system hosts a wave receiver and particle instrument to characterize the local environment, at a distance of 1–5 km from the mother rocket. The primary objectives of BeamPIE are two. The first is to demonstrate and increase the technology readiness level of the new electron accelerator technology for space applications. The second is to study wave generation from pulsed electron beams and quantify the generation efficiency of whistler waves relative to extraordinary-mode type waves. If waves of sufficient amplitude can be generated, a secondary science objective will be the investigation of wave-particle interaction physics and the changes to the local particle populations, possibly induced by the beam-generated waves.
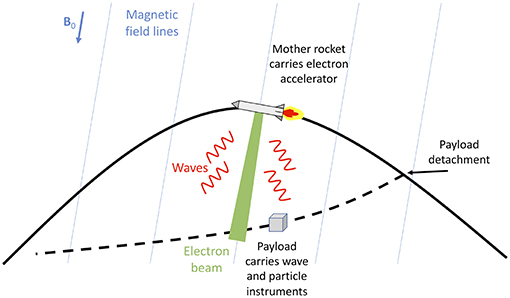
Figure 1. A depiction of the BeamPIE electron beam (green), launching plasma waves (red), and a secondary rocket payload (cube) diagnosing the plasma-wave emission.
2.3. The SMART Barium Shape Charge Experiment
The Space Measurement of Rocket-released Turbulence (SMART) is a sounding-rocket experiment concept developed by the Naval Research Laboratory (Ganguli et al., 2015). At an altitude of ~700 km, a shaped-charge explosion will release 1.5 kg of barium atoms at high velocity (~10 km/s) across the Earth's magnetic field. In ~30 s, the barium atoms photo-ionize and create an ion ring distribution in velocity space that is unstable to electrostatic lower-hybrid waves and develops broadband lower-hybrid turbulence. SMART targets a regime of parameters where the linear damping rates are smaller than the non-linear scattering rates, implying that lower-hybrid waves can be converted into whistler or magnetosonic waves (and secondary lower hybrid waves), before significant dissipation and local plasma heating occurs. Furthermore, the electromagnetic whistler waves can propagate out of the ionospheric source region into the magnetosphere and never return to it. Estimates of the net energy extracted from the initial ring distribution (~5–10%) translate into whistler wave amplitudes of the order of 200 pT (Ganguli et al., 2015), which are easily detectable from magnetospheric spacecraft. The SMART rocket will carry the barium release module and an instrumented payload that will characterize the local turbulent source region. Operating in conjunction with magnetospheric spacecraft like THEMIS (Time History of Events and Macroscale Interactions during Substorms) to detect the SMART-induced waves, the SMART science objective is to unravel the physics of lower-hybrid turbulence in magnetized plasmas. An estimated launch date for SMART is the middle of 2021 (G. Ganguli, 2019, private communication).
3. Potential Future Experiments
At the “Active Experiments in Space: Past, Present, and Future” workshop in Santa Fe (Delzanno and Borovsky, 2018), several concepts for future space active experiments were presented, and during audience-participation discussions, the attendees highlighted the need to design active experiments to investigate (1) magnetic-reconnection onset, (2) the triggering of substorms by active experiments, (3) the mass loading of ongoing collisionless reconnection, (4) critical-ionization-velocity physics, (5) Alfvén-wave transits from one hemisphere to the other, (6) conjugate traveling-ionospheric-disturbance phenomena, and (7) magnetosphere-ionosphere coupling. There were also discussions of the pros and cons of repeating previous active space experiments with newer experimental designs and with more-powerful modern diagnostics. Calls were made by the attendees for active space experiments to address issues beyond plasma physics and the space environment: the need for experiments addressing problems in planetary physics, astrophysics, and extreme environments were suggested. Some of that workshop discussion has been incorporated into subsections 3.1–3.7 into section 4. Some of these are experiments that address large-scale issues of magnetospheric physics, such as magnetosphere-ionosphere connectivity, triggering atmospheric discharges, triggering substorms, and producing pitch-angle scattering of magnetospheric particles into the atmosphere.
3.1. Electron Beams and Magnetic-Field-Line Tracing
The goal of this project is to accurately connect magnetospheric spacecraft measurements to ionospheric phenomena. Much of the connection between ionospheric physical processes and magnetospheric physical processes is not known. This is particularly true for the aurora and the magnetospheric processes that cause the aurora (Swift, 1978; Borovsky, 1993; Haerendel, 2011). Without understanding which physical processes act in the magnetosphere, one cannot assess the impact of auroral occurrence on the dynamics of the magnetosphere. The magnetospheric processes are unknown because the space-physics community has not been able to unambiguously connect spacecraft measurements in the magnetosphere to specific auroral forms. Magnetic field models can be used to connect large-scale regions of the magnetosphere to large-scale regions of the ionosphere (e.g., Feldstein and Galperin, 1985; Elphinstone et al., 1991; Galperin and Feldstein, 1996) but the magnetic-field models fail for the detailed mapping that is needed for auroral physics (Weiss et al., 1997; Ober et al., 2000; Shevchenko et al., 2010; Nishimura et al., 2011). The holy grail of auroral research is the low-latitude auroral arc, where one school of thought has the arcs in the ionosphere magnetically mapping out into the dipolar region of the magnetosphere (McIlwain, 1975; Meng et al., 1979; Mauk and Meng, 1991; Pulkkinen et al., 1991; Lu et al., 2000; Motoba et al., 2015), while another school has them mapping into the stretched magneto tail (Birn et al., 2004, 2012; Sergeev et al., 2012a; Hseih and Otto, 2014). One active experiment methodology, proposed to overcome the problem of connecting magnetosphere measurements with ionospheric phenomena, is the use of an electron accelerator on a spacecraft making measurements in the magnetosphere (Borovsky et al., 1998; Delzanno et al., 2016). This is depicted in Figure 2. Firing the electron beam into the atmospheric loss cone and optically imaging the atmospheric beam spot using ground-based cameras can unambiguously connect critical magnetospheric measurements of plasma, flows, fields, and waves to the various auroral forms. (This spacecraft-deployed electron beam is called out in the NRC Decadal Survey (National Research Council, 2012) as a needed emerging technology for space physics.) 1 kW of beam power into the upper atmosphere will produce 3 W of optical emission in the 3914-Å band of N+2N+2 (Dalgarno et al., 1965; Marshall et al., 2014). To get 1 kW of beam power, 25 mA of beam current at 40 keV is needed or 1 mA of beam current at 1 MeV is needed; firing the beam for 1 s would remove 0.025 C or 0.001 C of negative charge from the spacecraft, respectively. Spacecraft charging in the tenuous collisionless magnetospheric plasma is a potential problem. The development of compact, efficient relativistic-electron accelerators (cf. section 4.1) greatly reduces the spacecraft-charging problem by reducing the beam current. Using a plasma contactor (e.g., Olsen, 1985; Comfort et al., 1998) on the spacecraft, simulation analysis (Delzanno et al., 2015a,b; Lucco Castello et al., 2018) finds that the mechanism of ion emission from the surface of a kilometer-sized plasma-contactor plume will be able to balance the 1-mA electron-beam current and keep spacecraft charging to a low level. Magnetic-field measurements onboard the spacecraft are used to point the accelerator beam into the atmospheric loss cone. Increasing the beam energy could further reduce the beam current, which would further reduce the risk of spacecraft charging. However, for beams with energies above 1 MeV, beam pointing becomes a challenging issue, since the atmospheric loss cone shifts away from the 0°-pitch-angle direction owing to finite-gyroradius effects (Mozer, 1966; Il'ina et al., 1993; Porazik et al., 2014). The present design for the 1-MeV compact accelerator (Lewellen et al., 2019) yields an electron beam with an angular divergence of <0.05°, including the beam's electrostatic expansion after exiting the accelerator (The space charge of the 1-MeV 1-mA beam is very low.). Such a beam easily fits inside an atmospheric loss cone that is >1°. One unfortunate fact is that the electron beam produces optical emission in the exact same airglow wavelength bands as does the electron aurora, making it difficult for the ground-based cameras to identify the spacecraft beam spot in the presence of active aurora: using a time-coded on-and-off beam sequence and looking for the blinking beam spot greatly improves the detection. There is also the possibility of detecting the beam spot via ground-based radar (Izhovkina et al., 1980; Uspensky et al., 1980; Zhulin et al., 1980; Marshall et al., 2014, 2018) and of using the relativistic beam to do ionospheric and atmospheric experiments diagnosed by the radar.
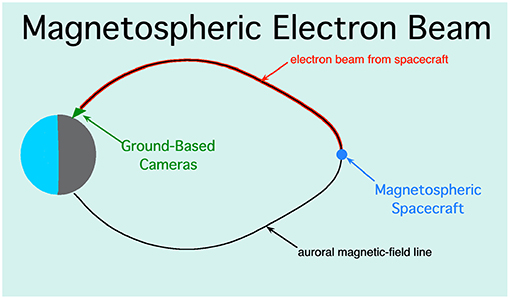
Figure 2. A sketch of a spacecraft in the magnetosphere (blue), firing an electron beam (red) along the Earth's magnetic-field line to create an optical spot in the upper atmosphere to locate the magnetic footpoint of the spacecraft in the context of optical aurora as viewed by ground-based cameras (green).
3.2. Relativistic Electron Beams Into the Middle Atmosphere
Ionospheric and atmospheric experiments could be performed with a relativistic-electron beam fired downward from the magnetosphere, or fired from a low-altitude spacecraft, a rocket (e.g., Nunz, 1990; O'Shea et al., 1991), or even from a balloon if the beam energy is high enough (See depiction in Figure 3). Electrons with energies of a few MeV range out at about 40–50 km altitude (Marshall et al., 2014), where the atmospheric number density and collision density is about the same as in a 1-Torr vacuum chamber. Ionization and recombination/attachment experiments have been suggested by Banks et al. (1988, 1990), Neubert et al. (1996), and by Neubert and Gilchrist (2004); these experiments could be diagnosed by ground-based radar (cf. Figure 3). Issues that could be investigated include the decay of electrical conductivity, electron-attachment rates, and the transport of negative and positive ions in the atmospheric electric field (Borovsky, 2017). The stimulation of atmospheric-electricity discharges by the electrical-conductivity paths, provided by relativistic-electron-beam ionization columns above thunderstorms, have been suggested by Banks et al. (1988, 1990), Neubert et al. (1990), Neubert and Gilchrist (2004), and Marshall et al. (2018) with the discharge current flowing between the top of a thunderstorm and the ionosphere. The energy deposition of a 1 kW beam is about 50 times the energy deposition of a naturally occurring relativistic-electron microburst (Lorentzen et al., 2001; Borovsky, 2017). These triggered thunderstorm discharges could be diagnosed by ground-based optics or by ground-based electric (Thomas et al., 2004; Sonnenfeld and Hager, 2013), magnetic (Whitley et al., 2011), or electromagnetic (Rhodes et al., 1994; Qin et al., 2012) measurements. The observation of upward accelerated energetic particles from the triggered discharges (e.g., Lehtinen et al., 2000, 2001) has also been suggested by Neubert and Gilchrist (2004); such observations can be made from the spacecraft or rocket that carries the relativistic-electron accelerator. Atmospheric chemistry modification by relativistic electron beams has also been explored (Neubert et al., 1990; Marshall et al., 2018), with the suggestion of diagnostic via ground-based spectroscopy; the chemistry of NOx, HOx, and ozone production in the middle atmosphere by energetic electron precipitation is of particular interest for the information it can supply about the interaction of the Earth's radiation belt with the Earth's climate system (Rodger et al., 2010; Andersson et al., 2012; Verronen et al., 2013).
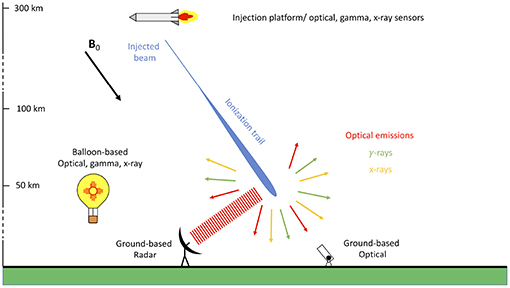
Figure 3. A depiction of a relativistic-electron beam fired downward along the Earth's magnetic field into the middle atmosphere, being diagnosed by ground-based radar, ground-based optical, and balloon-based x-rays and gamma rays [After Figure 1 of Marshall et al. (2014)].
3.3. Modifying Magnetic Reconnection With Heavy Ions
Gaining an understanding of the factors that control the onset of collisionless reconnection and the factors that control reconnection rates is of great importance to magnetospheric physics and solar-coronal physics. Using an artificial plasma cloud to modify collisionless reconnection (to initiate the onset of reconnection or to mass load and reduce ongoing reconnection) is a possibility. The onset of collisionless reconnection is an outstanding science issue that would improve the prediction of substorm occurrence (McPherron et al., 1973; Sergeev et al., 2012b) and of solar-flares occurrence (Priest, 1986; Li et al., 2017). It has been speculated both that the introduction of heavy ions to a plasma will make it (a) easier for the plasma to reach conditions for the onset of field-line reconnection (Baker et al., 1982, 1985, 1989) or (b) harder for it to reach the onset of reconnection (Liu et al., 2013; Liang et al., 2016). The onset of reconnection in collisionless plasmas is usually thought to be the caused by the thinning of a current sheet to a thickness below ion-inertial-length or ion-gyroradius spatial scales (Hesse and Birn, 2000; Liu et al., 2014). It has been variously speculated that introducing heavy ions (1) alters tearing modes that thin the current sheet, or (2) changes the ratio of current sheet thickness to gyroradii, or (3) mass loads current sheets. More simulation work with modern kinetic simulation codes (e.g., Karimabadi et al., 2011; Pritchett, 2013; Birn and Hesse, 2014) is needed to verify these conjectures. The mass loading of ongoing reconnection is an important concept for the reduction of solar-wind/magnetosphere coupling via magnetospheric feedback (Borovsky et al., 2013; Walsh et al., 2014). A cloud consisting of 1 kg of barium ions (e.g., Bryant et al., 1985), with a diameter of 1,000 km, has a mass density of about 1,000 AMU/cm3, which is about 20 times higher than the mass density of the magnetosheath plasma at the dayside reconnection site. This barium mass density is sufficient to effectively turn off dayside reconnection within the cloud, if the barium cloud could be released close enough to the dayside reconnection site. Figure 4 depicts the fact that getting the cloud (#1, purple) over the reconnection diffusion region (red) is helped by the fact that the barium ions will be carried into the reconnection line by the Mach-0.1 inflow of ambient plasma into the line. If the barium ions at the dayside magnetosphere could be optically imaged, the reconnection rate could be gauged by the speed of the barium ions carried in the reconnection outflow. Targeting reconnection away from the nose of the magnetosphere may allow ground-based imaging of the barium cloud via cameras located beyond the solar terminator. Since the location of the reconnection X-line may be difficult to predict, experiments on the mass loading of the reconnection outflow fan (which can extend across the entire dayside magnetopause) with barium releases, may be easier to implement. This is depicted as cloud #2 in Figure 4. Getting barium into the reconnection fan is again aided by the Mach-0.1 inflow of ambient plasma into the fan. Comparison of Earth's reconnection regimes (with and without heavy ions) with reconnection observations by MAVEN at Mars with O+ and O2+ ions (e.g., Harada et al., 2015) and by Juno at Jupiter with S+ ions could be useful for preparing and planning heavy-ion active experiments as described above.
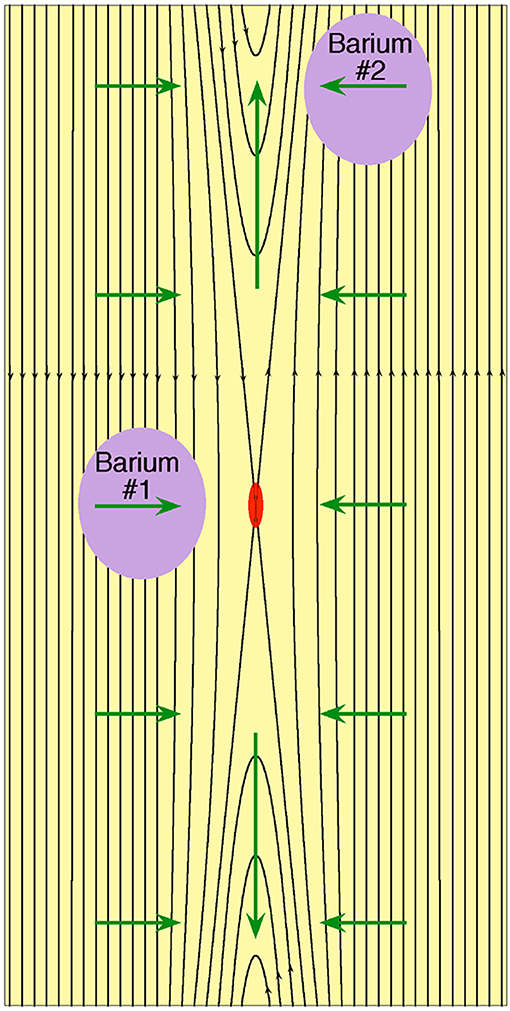
Figure 4. Magnetic-field-line reconnection on the dayside of the Earth is depicted with the solar-wind plasma to the left and the magnetospheric plasma to the right. The reconnection diffusion region is marked in red. There is a flow (green arrows) everywhere into the vertical plane of the current sheet that feeds plasma into the reconnection site or into the reconnection-outflow fan. Two barium clouds are depicted: Cloud #1 is being drawn into the reconnection diffusion region and cloud #2 is being drawn into the reconnection outflow fan.
3.4. Plasma-Wave Launching With Rotating-Magnet Antenna
Efficient ways to launch plasma waves into the magnetosphere are of interest for future technologies, such as radiation-belt remediation (Inan et al., 2003; Dupont, 2004). A space experiment has been suggested (Dennis Papadopoulos, private communication 2018) for the launching of whistler, EMIC (electromagnetic ion-cyclotron), and Alfvén waves, from a low-Earth-orbit spacecraft or a rocket using a superparamagnetic-nanoparticle-amplified rotating magnetic antenna. A rotating magnetic field can by created with an orthogonal pair of magnetic coils driven by sinusoidal currents with a 90° phase difference between the two coils. At the center of the orthogonal-coil pair, a vacuum vessel containing ~1 kg of superparamagnetic nanoparticles (Raikher et al., 2004) would act to amplify the strength of the rotating magnetic field by a factor of about 100, greatly amplifying the efficiency of the coils to launch whistler waves, EMIC waves, or Alfvén waves, depending on the frequency applied to the coils. Alfvén waves are important for understanding magnetosphere-ionosphere coupling (Goertz and Boswell, 1979) and whistler and EMIC waves are important for coupling the evolution of the radiation belt to the evolution of other magnetospheric plasmas (Borovsky and Valdivia, 2018). Without the superparamagetic nanoparticles, the two-coil rotating-magnetic-field concept has been successfully tested in the laboratory for the launching of Alfvén waves (Gigliotti et al., 2009; Karavaev et al., 2011) and whistler waves (Karavaev et al., 2010). As discussed in section 4, this proposed active experiment is being enabled by the technology development of superparamagnetic nanoparticles. A similar active space experiment has been suggested by Karavaev (2010) and de Sonria-Santacruz et al. (2014), using a mechanically rotating superconducting magnetic coil.
3.5. Space Tether Experiments
Tethers are a powerful technology tool that can be used to facilitate space experiments (Johnson et al., 2017; Huang et al., 2018): enabling multipoint measurements, launching whistler and Alfvén waves, acting as an antenna, and providing propulsion. Past active experiments using tethers (Lorenzini and Sanmartin, 2004; Cartmell and McKenzie, 2008) involved examining the dynamics and electrodynamics of tethers, the electrodynamic interaction between tethers and the space plasma environment, and the emission of plasma waves. More space experiments are needed to further understand the interactions of electrodynamic tethers with the plasma environment (e.g., Choiniere et al., 2001; Siguier et al., 2013; Janeski et al., 2015) and to explore wave launching by tethers (Estes, 1988; Luttgen and Neubauer, 1994; Sanchez-Arriaga and Sanmartin, 2010). One suggested active experiment is to use a kV-charged tether to electrostatically pitch-angle scatter radiation-belt particles into the atmospheric loss cone as the particles pass through the tether's sheath (Hoyt and Minor, 2005; Huboda de Badyn et al., 2016), although estimated time scales for remediation appear too long (~1 yr). Another interesting active experiment involving a tape tether used to explore the upper atmosphere has been suggested by Sanmartin (Sanmartin et al., 2006; Sanmartin, 2010): ambient ions would be accelerated into a long, negatively biased tape producing secondary electrons which are then accelerated off the tape to excite an artificial aurora in the upper atmosphere.
3.6. Cold-Plasma Releases
The idea of using cold-plasma releases in the magnetosphere, to trigger instabilities that stimulate electron and/or ion precipitation and produce artificial auroras, has been suggested since the seventies (Brice, 1970; Brice and Lucas, 1971; Cuperman and Landau, 1974). In the magnetosphere, EMIC waves are driven by hot-ion temperature anisotropies associated with magnetospheric convection and charge exchange, and whistler-mode chorus waves are driven by hot-electron temperature anisotropies associated with substorm injections. The addition of cold ions to the magnetosphere by a plasma release will change the growth rates and saturation amplitudes of EMIC waves (Fu et al., 2016; Gary et al., 2016). Whereas, the addition of cold electrons to the magnetosphere by a plasma release will change the growth rates and saturation amplitudes for whistler waves (Cuperman et al., 1973; Cuperman and Sternlieb, 1975; Gary et al., 2012). The cold ions and electrons also change the energetic-particle resonance conditions for EMIC waves and whistler waves, respectively (Summers et al., 1998). Provided that certain conditions on the anisotropy of the distribution function are met, a plasma injection can allow more particles to precipitate in concert with the development of the instability and the generation of electromagnetic waves. A likely location in the magnetosphere for such a cold-plasma experiment is in the nightside of the dipolar region, where there can be anisotropic hot populations to drive waves, and where ordinarily, there is an absence of cold ions and electrons owing to magnetospheric convection bringing plasma in from the magnetotail.
Magnetospheric barium and lithium release experiments were performed in the Active Magnetospheric Particle Tracer Explorer (AMPTE) (Krimigis et al., 1982) and the Combined Release and Radiation Effects Satellite (CRRES) programs, with several scientific goals including substorm triggering and stimulation of particle precipitation. In particular, three lithium releases (G-5, G-6 and G-7), by CRESS at ~33,000 km, did not show enhanced aurora that would be a sign of enhanced wave-particle interactions (Bernhardt, 1992). Two barium releases (G-8 and G-10) showed increased auroral activity within 5 min from the release, although the definitive association with the release was uncertain (Bernhardt, 1992). Similarly, a magnetotail barium release by AMPTE, during the development of a substorm, showed the barium cloud moving antisunward and was interpreted with the formation of a reconnection plasmoid (Baker et al., 1989).
Given the importance of substorms and wave-particle-interaction physics for magnetospheric dynamics, cold-plasma release active experiments should be pursued in the future with modern technology to test relevant theories of magnetosphere-ionosphere coupling.
3.7. Hydrogen-Gas Releases for Enhancing Energetic-Neutral-Atom Imaging of the Magnetosphere
Information (densities and temperatures) about the global distribution of hot plasma in the Earth's magnetosphere is obtained by imaging the energetic neutral atoms that are produced when energetic plasma ions charge exchange with the Earth's neutral-hydrogen geocorona (e.g., Roelof et al., 1985; Gruntman, 1997). One difficulty with the neutral-atom-imaging technique is that the measured fluxes of neutral atoms are line-of-sight integrated through the entire magnetosphere. Scime and Keesee (2018) propose a method to focus the neutral-atom measurements on a single point in space by releasing neutral hydrogen gas at that point in space to greatly enhance the number of charge exchange collisions, and hence greatly enhance the flux of energetic neutral atoms originating from the release site. This would provide higher spatial resolution measurements of the magnetospheric hot plasmas of the magnetosphere at the same time as global images are being obtained.
4. Critical Technical Advances
For the future of space active experiments, several technical advances are being made that will facilitate new and improved experiments. Further, there is presently improved laboratory and computer simulation support capabilities for the design of future space experiments.
4.1. Advances in Electron Accelerators
For future electron-beam experiments in the magnetosphere, the research and development advances of compact relativistic-electron accelerators has been crucial. Accelerators that have relatively high efficiency (bus power to beam power) are in development (Lewellen et al., 2019): this increased efficiency saves battery weight on the spacecraft and reduces battery recharging time from solar panels, the latter enabling more beam time. The critical thermal issue of heat removal from the accelerator has been reduced by the development of a method for re-tuning the frequency fed to the linear accelerator, as the accelerator changes temperature and mechanically expands. Designs for the remote operation of fault-tolerant linear accelerators are in development.
4.2. Superparamagnetic Nanoparticles
As discussed in section 4, advances in the development of superparamagnetic nanoparticles for amplifying AC magnetic fields is making the design of more-powerful space-based wave antennas possible.
4.3. CubeSats
The development and availability of low-cost CubeSats has increased access to low-Earth orbit for experiments (Bahcivan et al., 2012; Poghosyan and Golkar, 2017) and diagnostics (Blum et al., 2013; Fish et al., 2014). Active-space-experiment diagnostics with constellations of CubeSats (Glumb et al., 2016; Deng et al., 2017) is a new possibility.
4.4. Controlling Spacecraft Charging
As discussed in section 3, the advancement in our understanding of methods to ameliorate spacecraft charging in electron-beam experiments is allowing for lower-risk experiments to be designed. A significant advance has been made by the interpretation of plasma contactors in the collisionless magnetosphere, working as ion emitters rather than electron collectors (Delzanno et al., 2015a,b; Lucco Castello et al., 2018). This work was guided by new plasma-simulation capabilities (see section 4.6).
4.5. Laboratory Support for Developing Space Experiments
Laboratory experiments are becoming increasingly important for our understanding of plasma and space physics and in support of (active or inactive) space experiments, as reinforced in a recent review (Howes, 2018) that coined the term “laboratory space physics.” Often driven by similar advances in diagnostics and technology, laboratory experiments complement space experiments by allowing a more controlled environment that can be diagnosed much more extensively. On the other hand, laboratory experiments operate with plasma densities, temperatures and, more importantly, collisionality that can be very different from those of the space environment, thus allowing scaled experiments where only ratios of relevant quantities controlling the physics of interest can be kept in the same range. Laboratory experiments are ideally suited to isolate particular physics aspects of more complex problems, while their size limitation makes it difficult to explore things like long-range coupling.
In the US, there are several facilities with a history of significant contributions to space physics and the interested reader is referred to Howes (2018) and references therein for a summary. See also Koepke (2008). Here, we only focus on the connection between laboratory and active experiments and highlight relevant experiments.
The Basic Plasma Science Facility (BAPSF) at the University of California Los Angeles is a national user facility that hosts the LArge Plasma Device (LAPD), a 19-m long, 75-cm diameter cylindrical plasma column (Gekelman et al., 2016). LAPD operates with typical densities of 1012 cm−3 and electron temperatures of few eV (with lower values in the afterglow plasma). The high reproducibility of the experiments, combined with extensive diagnostics, make detailed three-dimensional characterization of the plasma an important feature of LAPD. To guide the design and interpretation of planned electron-beam experiments in space, electron-beam experiments are being performed on LAPD. While earlier experiments used a low-energy (3 keV) electron beam to explore the excitation of chirped whistler waves (Van Compernolle et al., 2015; An et al., 2016), a 1-MeV linac (Jenkins et al., 2018) is being installed on LAPD. The new experiments will study relativistic-beam stability and the generation of plasma waves, with application to solar radio bursts as well as to electron-beam active experiments for radiation-belt remediation. LAPD experiments involving a laser-generated plasma and its explosive dynamics across a magnetic field are investigating processes associated with the formation of a diamagnetic cavity and collisionless shocks (Niemann et al., 2013, 2014; Winske et al., 2019), and are relevant to early nuclear detonation experiments in space.
The Space Physics Simulation Chamber at the Naval Research Laboratory, shown in Figure 5, also allows for studies across different parameter regimes targeting ionospheric and magnetospheric conditions. Examples include the role of shear-driven ion-cyclotron waves in ion heating and initiation of ionospheric outflows (Amatucci et al., 1998), electron-ion hybrid instabilities important for the plasma sheet boundary layer (Amatucci et al., 2003), and the generation of electromagnetic ion cyclotron waves through shear flows (Tejero et al., 2011). More recent experiments have focused on non-linear scattering processes, successfully demonstrating the conversion of electrostatic lower-hybrid waves to electromagnetic whistler waves above an amplitude threshold (Tejero et al., 2015). This is a key aspect of the non-linear weak-turbulence physics that the SMART barium-release experiment aims to demonstrate (cf. section 2.3).
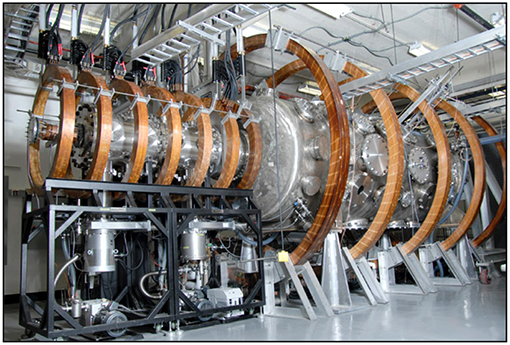
Figure 5. The 7.6-m long Space Physics Simulation Chamber at the Naval Research Laboratory in Washington DC (Photo courtesy of Erik Tejero).
The 6m × 9m Large Vacuum Test Facility (LVTF) and the 2m × 0.6m Cathode Test Facility (CTF) at the University of Michigan's Plasmadynamics and Electric Propulsion Laboratory (PEPL) (Gallimore et al., 1996; Gilchrist et al., 2002) have been used for experimental validation of spacecraft charging mitigation induced by high-power electron beams. LVTF is capable of reaching 10−7 (10−8) Torr and is the biggest vacuum chamber in the US. In the LVTF experiments, an isolated hollow-cathode represents the spacecraft. The hollow cathode emits a high-density charge-neutral plasma (known as the plasma contactor), while the emission of the spacecraft electron beam is mimicked through a separate power supply operated in constant-current mode. Several Langmuir probes, emissive probes and a retarding potential analyzer provide measurements of key quantities, identified by the space-experiment modeling work (Delzanno et al., 2015a,b; Lucco Castello et al., 2018). Remarkable agreement between theory and experiments has been obtained (Miars et al., 2018), thus validating the ion-emission model for spacecraft-charging mitigation for the operation of electron-beam experiments in the low-density magnetosphere (cf. section 4.4).
A Community-Coordinated Modeling-Challenge Facility that uses laboratory facilities at West Virginia University, combined with high-performance-computing modeling from interested parties, is also being proposed to study spacecraft-environment interactions (Koepke and Marchand, 2017).
4.6. Simulation Support for Designing Space Experiments
Another major advance in support of the design and planning of (active or inactive) space experiments comes from numerical simulations. This is the result of both the increased power and availability of modern high-performance computers, and also of the recent advances in development of new numerical algorithms to tackle the multiscale nature of plasmas. The major challenge comes from the large spatial and temporal scale separation typical of magnetized, collisionless plasmas. This occurs already at the microscopic/kinetic level, due to the mass difference between electrons and ions, but quickly becomes overwhelming when one compares microscopic scales with system scales.
Recent advances in the development of kinetic Vlasov-Maxwell solvers include the implicit particle-in-cell (PIC) method (where implicit refers to the temporal discretization of the method) (Chen et al., 2011; Markidis and Lapenta, 2011) and the use of discontinuous-Galerkin discretization techniques (Juno et al., 2018). Moreover, electrostatic PIC methods that employ some form of non-uniform mesh (either conforming or through adaptive mesh refinement, structured, or unstructured) are commonly used to study dynamic spacecraft-environment interactions (Mandell et al., 2006; Roussel et al., 2008; Marchand, 2012; Delzanno et al., 2013; Meierbachtol et al., 2017).
In terms of global codes for large-scale dynamics, hybrid (kinetic ions and fluid electrons) codes, running on high-performance computing platforms, are now routinely applied to study the dynamic of the Earth's magnetosphere (Karimabadi et al., 2014; Lin et al., 2017; Palmroth et al., 2018). Furthermore, methods for “fluid-kinetic coupling” are also being developed for large-scale simulations that include microscopic physics. One approach is based on a regional kinetic code locally embedded in a large-scale fluid-like simulation (which is typically from a magnetohydrodynamic code) (Sugiyama and Kusano, 2007; Kolobov and Arslanbekov, 2012; Daldorff et al., 2014; Tóth et al., 2016; Ho et al., 2018). This approach has been successfully applied to study flux-transfer events and Earth's dayside reconnection (Chen et al., 2017). Other approaches are based on higher-order fluid moments with suitable closures (Wang et al., 2015). A new method that encompasses both techniques described above has been developed in the SpectralPlasmaSolver (SPS) code (Delzanno, 2015; Vencels et al., 2016). It is based on a spectral expansion of the plasma distribution function in Hermite functions, such that the low-order terms of the expansion are akin to a fluid description of the plasma, while kinetic physics is retained by adding (possibly locally in the simulation domain) more terms to the expansion. As such, fluid-kinetic coupling is an intrinsic feature of SPS, but the method is not constrained to a fixed number of moments and the transition between fluid and kinetic regimes can be handled as smoothly as necessary. SPS has been successfully applied to the turbulent cascade in the solar wind (Roytershteyn and Delzanno, 2018; Roytershteyn et al., 2019). Global space weather models, such as the SHIELDS (Space Hazards Induced near Earth by Large Dynamic Storms) framework (Jordanova et al., 2018), are now beginning to incorporate some of these innovations (which also include data-assimilation techniques to assimilate available observational data) and will be very important in the future to put spacecraft observations into better context, particularly for geomagnetically active times.
Finally, besides some of the more technical innovations highlighted above, we mention the Community Coordinated Modeling Center (CCMC, https://ccmc.gsfc.nasa.gov/index.php) (Bellaire, 2006; Rastatter et al., 2012), which hosts a large number of heliospheric, magnetospheric, and ionospheric simulation codes and models, and offers free “runs on request” using the computational resources of the center. CCMC's goal is to provide access to modern space science simulations for the international research community.
5. Concluding Remarks on the Future
There are still many open questions that need to be answered by future active experiments. Three examples from three research fields are given to highlight the breadth of future active experiments. For plasma astrophysics: (1) Under what conditions does the critical-ionization-velocity effect operate? For space physics: (2) What is the magnetic-field connectivity between ionospheric regions and processes and magnetospheric regions and processes? For space engineering: (3) What is the most effective way to generate various types of plasma waves from a space platform? There are also technology capabilities that need to be developed via space experiments: e.g., (i) radiation-belt remediation and (ii) power transmission between Earth and space. And there are also new, modern technologies (in a broad sense that encompasses also diagnostics, laboratory experiments and computer simulations), perhaps best exemplified by the fact that a Tesla automobile is currently traveling in deep-space orbit (Chang, 2018), that justify new and more ambitious active experiments.
In addition, active experiments that are not necessarily associated with plasma or space physics will also be extremely important. An example is the Stratospheric Controlled Perturbation Experiment (SCoPEx, https://projects.iq.harvard.edu/keutschgroup/scopex) experiment, which plans to release aerosols in the stratosphere as a possible way to reduce or eliminate ozone loss and mitigate global warming.
Active experiments have a rich history of important contributions to the field of space physics. As the spiral of knowledge advances, revisiting active experiments holds a key to finally closing fundamental questions.
Some of these grand-challenge problems can only be addressed successfully with a broad cross-disciplinary team at the intersection between theory, modeling, observations, experiments (in laboratory and, ultimately, in space) and, importantly, technology. It is, however, extremely hard to develop and maintain these large collaborations until suitable opportunities open up. One potential remedy and recommendation would be to reinvigorate and expand the active space-based experiments program, which flourished in the 1970s and 1980s to test basic scientific ideas and new technologies in space, but it has reduced its footprint in recent decades (Delzanno and Borovsky, 2018).
For the field of space active experiments, the future looks busy.
Author Contributions
JB and GD shared equally in the planning and outlining of the manuscript and in the researching and writing of the manuscript.
Funding
Work at the Space Science Institute was supported by NASA Heliophysics LWS TRT program via grants NNX16AB75G and NNX14AN90G, by the NSF GEM Program via award AGS-1502947, by the NSF Solar-Terrestrial Program via grant AGS-1261659, and by the NASA Heliophysics Guest Investigator Program via grant NNX14AC15G. Work at Los Alamos National Laboratory was supported the Laboratory Directed Research and Development program (LDRD), under the auspices of the National Nuclear Security Administration of the U.S. Department of Energy by Los Alamos National Laboratory, operated by Los Alamos National Security LLC under contract DE-AC52-06NA25396.
Conflict of Interest Statement
The authors declare that the research was conducted in the absence of any commercial or financial relationships that could be construed as a potential conflict of interest.
Acknowledgments
The authors thank Guru Ganguli, Brian Gilchrist, Bob Marshall, Dennis Papadopoulos, Vadim Roytershteyn, Ennio Sanchez, Erik Tejero, and Kateryna Yakymenko for helpful conversations and in particular they thank Gerhard Haerendel.
References
Amatucci, W. E., Ganguli, G., Walker, D. N., Gatling, G., Balkey, M., and McCullock, T. (2003). Laboratory investigation of boundary layer processes due to strong spatial inhomogeneity. Phys. Plasmas 10:1963. doi: 10.1063/1.1562631
Amatucci, W. E., Walker, D. N., Ganguli, G., Duncan, D., Antoniades, J. A., Bowles, J. H., et al. (1998). Velocity-shear-driven ion-cyclotron waves and associated transverse ion heating. J. Geophys. Res. 103:11711. doi: 10.1029/98JA00659
An, X., Van Compernolle, B., Bortnik, J., Thorne, R. M, Chen, L., and Li, W. (2016). Resonant excitation of whistler waves by a helical electron beam. Geophys. Res. Lett. 43:2413. doi: 10.1002/2015GL067126
Andersson, M. E., Verronen, P. T., Wang, S., Rodger, C. J., Clilverd, M. A., and Carson, B. R. (2012). Precipitating radiation belt electrons and enhancements of mesospheric hydroxyl during 2004-2009. J. Geophys. Res. 117, 1–13. doi: 10.1029/2011JD017246
Bahcivan, H., Cutler, J. W., Bennett, M., Kempke, B., Springmann, J. C., Buonocore, J., et al. (2012). First measurements of radar coherence scatter by the radio aurora explorer cubeSat. Geophys. Res. Lett. 39, 1–5. doi: 10.1029/2012GL052249
Baker, D. N., Fritz, T. A., and Bernhardt, P. A. (1989). A small-scale plasmoid formed during the May 13, 1985, AMPTE magnetotail barium release. J. Geophys. Res. 94:17084. doi: 10.1029/JA094iA12p17084
Baker, D. N., Fritz, T. A., Lennartsson, W., Wilken, B., Kroehl, H. W., and Birn, J. (1985). The role of heavy ionospheric ions in the localization of substorm disturbances on March 22, 1979: CDAW 6. J. Geophys. Res. 90, 1273–1281. doi: 10.1029/JA090iA02p01273
Baker, D. N., Hones, E. W., Young, D. T., and Birn, J. (1982). The possible role of ionospheric oxygen in the initiation and development of plasma sheet instabilities. Geophys. Res. Lett. 9, 1337–1340. doi: 10.1029/GL009i012p01337
Banks, P. M., Fraser-Smith, A. C., Gilchirst, B. E., Harker, K. J., Storey, L. R. O., and Willianmson, P. R. (1988). New Concepts in Ionospheric Modification. Air Force Geophysical Laboratory report AFGL-TR-88–0133.
Banks, P. M., Fraser-Smith, A. C., and Gilchrist, B. E. (1990). “Ionospheric modification using relativistic electron beams,” in AGARD Conference Proceedings (Loughton: Specialised Printing Services Limited), 485.
Bellaire, P. J. (2006). Community Coordinated Modeling Center 2005 Workshop report, Space Weath. 4:S02004.
Bernhardt, P. A. (1992). Probing the magnetosphere using chemical releases from the combined release and radiation effects satellite. Phys. Fluids B. 4:2249. doi: 10.1063/1.860193
Birn, J., and Hesse, M. (2014). Forced reconnection in the near magnetotail: onset and energy conversion in PIC and MHD simulations. J. Geophys. Res. 119, 290–309. doi: 10.1002/2013JA019354
Birn, J., Schindler, K., and Hesse, M. (2004). Thin electron current sheets and their relation to auroral potentials. J. Geophys. Res. 109:A02217. doi: 10.1029/2003JA010303
Birn, J., Schindler, K., and Hesse, M. (2012). Magnetotail aurora connection: the role of thin current sheets. Geophys Monog Ser. 197:337. doi: 10.1029/2011GM001182
Blum, L. W., Schiller, Q., Li, X., Millan, R., Halford, A., and Woodger, L. (2013). New conjunctive CubeSat and balloon measurements to quantify rapid energetic electron precipitation. Geophys. Res. Lett. 40, 5833–5837. doi: 10.1002/2013GL058546
Borovsky, J. (1993). E. Auroral-arc thicknesses as predicted by various theories. J. Geophys. Res. 98:6101. doi: 10.1029/92JA02242
Borovsky, J. (2017). E. Electrical conductivity channels in the atmosphere produced by relativistic-electron microbursts from the magnetosphere. J. Atmos Solar-Terr Phys. 155:22. doi: 10.1016/j.jastp.2017.01.004
Borovsky, J. E., Denton, M. H., Denton, R. E., Jordanova, V. K., and Krall, J. (2013). Estimating the effects of ionospheric plasma on solar-wind/magnetosphere coupling via mass loading of dayside reconnection: ion-plasma-sheet oxygen, plasmaspheric drainage plumes, and the plasma cloak. J. Geophys. Res. 118:5695. doi: 10.1002/jgra.50527
Borovsky, J. E., Greenwald, R. A., Hallinan, T. J., Horwitz, J. L., Kelley, M. C., Klumpar, D. M., et al. (1998). The magnetosphere-ionosphere facility: a satellite cluster in geosynchronous orbit connected to ground-based observatories. Eos Trans. Am. Geophys. Union 79:F744.
Borovsky, J. E., and Valdivia, J. A. (2018). The Earth's magnetosphere: a systems science overview and assessment. Surv. Geophys. 39, 817–859. doi: 10.1007/s10712-018-9487-x
Brice, N. M. (1970). Artificial enhancement of energetic particle precipitation through cold plasma injection: a technique for seeding substorms? J. Geophys. Res. 75:4890. doi: 10.1029/JA075i025p04890
Brice, N. M., and Lucas, C. (1971). Influence of magnetospheric convection and polar wind loss of electrons from the outer radiation belt. J. Geophys. Res. 76:900. doi: 10.1029/JA076i004p00900
Bryant, D. A., Krimigis, S. M., and Haerendel, G. (1985). Outline of the active magnetospheric particle tracers explorers (AMPTE) mission. IEEE Trans. Geosci. Remote Sensing 23, 177–181. doi: 10.1109/TGRS.1985.289511
Cartmell, M. P., and McKenzie, D. J. (2008). A review of space tether research. Prog. Aerospace Sci. 44, 1–21. doi: 10.1016/j.paerosci.2007.08.002
Chang, L. (2018). Falcon Heavy in a roar of thunder, carries Space-X's ambition into orbit. New York Times.
Chen, G., Chacon, L., and Barnes, D. C. (2011). An energy- and charge-conserving, implicit, electrostatic particle-in-cell algorithm. J. Comput. Phys. 230, 7018–7036. doi: 10.1016/j.jcp.2011.05.031
Chen, Y., Tóth, G., Cassak, P., Jia, X., Gombosi, T. I., Slavin, J. A., et al. (2017). Global three-dimensional simulation of Earth's dayside reconnection using a two-way coupled magnetohydrodynamics with embedded particle-in-cell model: initial results. J. Geophys. Res. 122, 10318–10335. doi: 10.1002/2017JA024186
Choiniere, E., Gilchrist, B. E., and Bilen, S. G. (2001). “Enhancement of electrodynamic tether electron current colletion using radio frequency power,” in AIP Conference Proceeding (Melville; New York, NY). 473–478. doi: 10.1063/1.1357964
Comfort, R. H., Moore, T. E., Craven, P. D., Pollock, C. F., Mozer, F. S., and Williamson, W. S. (1998). Spacecraft potential control by the Plasma Source Instrument on the POLAR satellite. J. Spacecraft Rockets 35, 845–849. doi: 10.2514/2.7586
Cuperman, S., and Landau, R. W. (1974). On the enhancement of the whistler mode instability in the magnetosphere by cold plasma injection. J. Geophys. Res. 79, 128–134. doi: 10.1029/JA079i001p00128
Cuperman, S., Salu, Y., Bernstein, W., and Williams, D. J. (1973). Computer simulation of cold plasma effects on the whistler instability for geostationary orbit plasma parameters. J. Geophys. Res. 78, 7372–7387. doi: 10.1029/JA078i031p07372
Cuperman, S., and Sternlieb, A. (1975). Computer simulation of cold plasma effects on the whistler instability for geostationary orbit plasma parameters 2. The case of enhancement of the maximum rate of growth. J. Geophys. Res. 80, 1357–1359. doi: 10.1029/JA080i010p01357
Daldorff, L. K. S., Tóth, G., Gombosi, T. I., Lapenta, G., Amaya, J., Markidis, S., et al. (2014). Two-way coupling of a global Hall magnetohydrodynamics model with a local implicit particle-in-cell model. J. Comput. Phys. 268, 236–254. doi: 10.1016/j.jcp.2014.03.009
Dalgarno, A., Latimer, I. D., and McConkey, J. W. (1965). Corpuscular bombardment and N2+ radiation. Planet Space Sci. 13:1008. doi: 10.1016/0032-0633(65)90160-1
de Sonria-Santacruz, M., Martinez-Sanchez, M., and Shprits, Y. Y. (2014). Controlled precipitation of energetic Van Allen belt protons by electromagnetic ion cyclotron (EMIC) waves. Space Weath 12, 354–367. doi: 10.1002/2014SW001047
Delzanno, G. L. (2015). Multi-dimensional, fully-implicit, spectral method for the Vlasov-Maxwell equations with exact conservation laws in discrete form. J. Comput. Phys. 301, 338–356. doi: 10.1016/j.jcp.2015.07.028
Delzanno, G. L., and Borovsky, J. E. (2018). Assessing the future of space-based experiments. Eos. 4:100. doi: 10.1029/2018EO100867
Delzanno, G. L., Borovsky, J. E., Thomsen, M. F., Gilchrist, B. E., and Sanchez, E. (2016). Can an electron gun solve the outstanding problem of magnetosphere-ionosphere connectivity? J. Geophys. Res. 121:6769. doi: 10.1002/2016JA022728
Delzanno, G. L., Borovsky, J. E., Thomsen, M. F., and Moulton, J. D. (2015a). Beam experiments in the magnetosphere with plasma contactors: the electron collection and ion emission routes, J. Geophys. Res. 120:3588. doi: 10.1002/2014JA020683
Delzanno, G. L., Borovsky, J. E., Thomsen, M. F., Moulton, J. D., and MacDonald, E. A. (2015b). Beam experiments in the magnetosphere: how do we get the charge off the spacecraft? J. Geophys. Res. 120:3647. doi: 10.1002/2014JA020608
Delzanno, G. L., Camporeale, E., Moulton, J. D., Borovsky, J. E., MacDonald, E. A., and Thomsen, M. F. (2013). CPIC: a curvilinear particle-in-cell code for plasma-material interaction studies. IEEE Trans. Plasma Sci. 41:3577. doi: 10.1109/TPS.2013.2290060
Deng, S.-C., Meng, T., and Jin, Z.-H. (2017). Nonlinear programming control using differential aerodynamic drag for CubeSat formation flying. Front. Informat. Tech. Electron Eng. 18, 867–881. doi: 10.1631/FITEE.1500493
Dupont, D. G. (2004). Nuclear explosions in orbit. Sci. Am, 290:100–107. doi: 10.1038/scientificamerican0604-100
Elphinstone, R. D., Hearn, D., Murphree, J. S., and Cogger, L. L. (1991). Mapping using the Tsyganenko Long magnetospheric model and its relationship to Viking auroral images. J. Geophys. Res. 96:1467. doi: 10.1029/90JA01625
Estes, R. D. (1988). Alfvén waves from an electrodynamic tethered satellite system. J. Geophys Res. 93, 945–956. doi: 10.1029/JA093iA02p00945
Feldstein, Y. I., and Galperin, Y. I. (1985). The auroral luminosity structure in the high-latitude upper atmosphere: its dynamics and relationship to the large-scale structure of the Earth's magnetosphere. Rev. Geophys. 23:217. doi: 10.1029/RG023i003p00217
Fish, C. S., Swensen, C. M., Crowly, G., Barjatya, A., Neilsen, T., Gunther, J., et al. (2014). Design, development, implementation, and on-orbit performance of the Dynamic Ionosphere CubeSat Experiment mission. Space Sci. Rev. 181, 61–120. doi: 10.1007/s11214-014-0034-x
Fu, X., Cowee, M. M., Jordanova, V. K., Gary, S. P., Reeves, G. D., and Winske, D. (2016). Predicting electromagnetic ion cyclotron wave amplitude from unstable ring current plasma conditions. J. Geophys. Res. 121, 10954–10965. doi: 10.1002/2016JA023303
Gallimore, A. C., Kim, S.-W., Foster, J. E., King, L. B., and Gulczinski, S. (1996). Near- and far-field plume studies of a one-kilowatt arcjet. J. Propulsion Power. 12, 105–111. doi: 10.2514/3.23997
Galperin, Y. I., and Feldstein, Y. I. (1996). Mapping the precipitation regions to the plasma sheet. Geomag. Geoelectr. J. 48:857.
Ganguli, G., Crabtree, C., Mithaiwala, M., Rudakov, L., and Scales, W. (2015). Evolution of lower hybrid turbulence in the ionosphere. Phys. Plasmas. 22:112904. doi: 10.1063/1.4936281
Gary, S. P., Fu, X., Cowee, M. M., Winske, D., and Liu, K. (2016). Scalings for the Alfvén-cyclotron instability: linear dispersion theory and hybrid particle-in-cell simulations. J. Geophys. Res. 122, 464–474. doi: 10.1002/2016JA023425
Gary, S. P., Liu, K., Denton, R. E., and Wu, S. (2012). Whistler anisotropy instability with a cold electron component: linear theory. J. Geophys. Res. 117:A07203. doi: 10.1029/2012JA017631
Gekelman, W., Pribyl, P., Lucky, Z., Drandell, M., Leneman, D., Maggs, J., et al. (2016). The upgraded large plasma device, a machine for studying frontier basic plasma physics. Rev. Sci. Instrum. 87:025105. doi: 10.1063/1.4941079
Gigliotti, A., Gekelman, W., Pribyl, P., Vincena, S., Karavaev, A., Shao, X., et al. (2009). Generation of polarized shear Alfvén waves by a rotating magnetic field source. Phys. Plasmas. 16, 1–8. doi: 10.1063/1.3224030
Gilchrist, B. E., Bilen, S. G., Choiniere, E., Gallimore, A. C., and Smith, T. B. (2002). Analysis of chamber simulations of long collecting probes in high-speed dense plasmas. IEEE Trans. Plasma Sci. 30, 2023–2034. doi: 10.1109/TPS.2002.807538
Glumb, R., Lapsley, M., Luce, S., Dery, J.-P., Scott, D., and Nielsen, T. (2016). HyperCube: A hyperspectral CubeSat constellation for measurements of 3D winds. Proc SPIE. 9978, 1–11. doi: 10.1117/12.2237796
Goertz, C. K., and Boswell, R. W. (1979). Magnetosphere-ionosphere coupling. J. Geophys. Res. 84, 7239–7246. doi: 10.1029/JA084iA12p07239
Grandal, B Eds. (1982). Aritficial Particle Beams in Space Plasma Studies. New York, NY: Plenum Press. doi: 10.1007/978-1-4684-4223-6
Gruntman, M. (1997). Energetic neutral atom imaging of space plasmas. Rev. Sci. Instrum. 68, 3617–3656. doi: 10.1063/1.1148389
Haerendel, G. (2011). Six auroral generators: a review. J. Geophys. Res. 116:A00K05. doi: 10.1029/2010JA016425
Haerendel, G. (2018). Experiments with plasmas artificially injected into near-Earth space. Front. Astron. Space Phys. 22 doi: 10.3389/fspas.2019.00029
Harada, Y., Haleka, J. S., McFadden, J. P., Mitchell, D. L., Mazelle, C., Connerney, J. E. P., et al. (2015). Magnetic reconnection in the near-Mars magnetotail: MAVEN obervations. Geophys. Res. Lett. 42, 8838–8845. doi: 10.1002/2015GL065004
Hesse, M., and Birn, J. (2000). Magnetic reconnection: three-dimensional aspects and onset in the magnetotail. IEEE Trans. Plasma Sci. 28, 1887–1900. doi: 10.1109/27.902217
Ho, A., Datta, I. A. M., and Shumlak, U. (2018). Physics-based-adaptive plasma model for high-fidelity numerical simulations. Front. Phys. 6:105. doi: 10.3389/fphy.2018.00105
Howes, G. (2018). Laboratory space physics: investigating the physics of space plasmas in the laboratory. Phys. Plasmas 25:055501. doi: 10.1063/1.5025421
Hoyt, R. P., and Minor, B. M. (2005). “Remediation of radiation belts using electrostatic tether structures, in 2005 IEEE Aerospace Conference (New York, NY: IEEE), 583–594. doi: 10.1109/AERO.2005.1559348
Hseih, M.-S., and Otto, A. (2014). The influence of magnetic flux depletion on the magnetotail and auroral morphology during the substorm growth phase. J. Geophys. Res. 119:3430. doi: 10.1002/2013JA019459
Huang, P., Zhang, F., Chun, L., Meng, Z., Zhang, Y., Liu, Z., et al. (2018). A review of space tether in new applications. Nonlinear Dyn. 94, 1–19. doi: 10.1007/s11071-018-4389-5
Huboda de Badyn, M., Marchand, R., and Sydora, R. D. (2016). Using orbital tethers to remediate geomagnetic radiation belts. J. Geophys. Res. 121, 1114–1123. doi: 10.1002/2015JA021715
Il'ina, A. N., Il'in, V. D., Kuznetsov, S. N., Yushkov, B. Y., Amirkhanov, I. V., and Il'in, I. V. (1993). Model of nonadiabatic charged-particle motion in the field of a magnetic dipole. JETP 77, 246–252.
Inan, U. S., Bell, T. F., Bortnik, J., and Albert, J. M. (2003). Controlled precipitation of radiation belt electrons. J. Geophys. Res. 108:1186. doi: 10.1029/2002JA009580
Izhovkina, N. I., JKosik, J. C., Pyatsi, A. K., Reme, H., Saint-Marc, A., Sverdlov, J. L., et al. (1980). Comparison between experimental and theoretical conjugate points locations in the Araks experiments. Ann. Geophys. 36:319.
James, H. G., Raitt, W. J., and Sulzer, M. P. (1998). Preface active experiments in space physics. Adv. Space Res. 24, 979–980.
Janeski, J. A., Hall, C. D., and Scales, W. A. (2015). Effects of local plasma environment on dynamics of electrodynamic tether systems. J. Spacecraft Rockets 52, 496–505. doi: 10.2514/1.A32684
Jenkins, M., Burt, G., Praveen Kumar, A. V., Saveliev, Y., Corlett, P., Hartnett, T., et al. (2018). A prototype 1 MeV X-band linac for aviation cargo inspection. Phys. Rev. Accel. Beams. 22:020101. doi: 10.1103/PhysRevAccelBeams.22.020101
Johnson, L., Bilen, S. G., Gilchrist, B. E., and Habash Krause, L. (2017). Tethers in space. Acta Astronautica. 138:502. doi: 10.1016/j.actaastro.2017.07.022
Jordanova, V. K., Delzanno, G. L., Henderson, M. G., Godinez, H. C., Jeffery, C. A., Lawrence, E. C., et al. (2018). Specification of the near-Earth space environment with SHIELDS. J. Atmos. Solar-Terr. Phys. 177, 148–159. doi: 10.1016/j.jastp.2017.11.006
Juno, J., Hakim, A., TenBarge, J., Shi, E., and Dorland, W. (2018). Discontinuous galerkin algorithms for fully kinetic plasmas. J. Comput. Phys. 353, 110–147. doi: 10.1016/j.jcp.2017.10.009
Karavaev, A. V. (2010). Waves in Plasmas Generated by a Rotating Magnetic Field and Implications to Radiation Belts. Ph.D. Thesis, Department of Physics and Astronomy, University of Maryland, Maryland.
Karavaev, A. V., Gumerov, N. A., Papdopoulos, K., Shao, X., Sharma, A. S., Gekelman, W., et al. (2010). Generation of whistler waves by a rotating magnetic field source. Phys. Plasmas 17:012102. doi: 10.1063/1.3274916
Karavaev, A. V., Gumerov, N. A., Papdopoulos, K., Shao, X., Sharma, A. S., Gekelman, W., et al. (2011). Generation of shear Alfvén waves by a rotating magnetic field source: three-dimensional simulations. Phys. Plasmas. 18:032113. doi: 10.1063/1.3562118
Karimabadi, H., Roytershteyn, V., Mouikis, C. G., Kistler, L. M., and Daughton, W. (2011). Flushing effect in reconnection: Effects of minority species of oxygen ions. Planet Space Sci. 59, 526–536. doi: 10.1016/j.pss.2010.07.014
Karimabadi, H., Roytershteyn, V., Vu, H. V., Omelchenko, Y. A., Scudder, J., Daughton, W., et al. (2014). The link between shocks, turbulence, and magnetic reconnection in collisionless plasmas. Phys. Plasmas. 21:062308. doi: 10.1063/1.4882875
Koepke, M. E. (2008). Interrelated laboratory and space plasma experiments. Rev. Geophys. 46:RG3001. doi: 10.1029/2005RG000168
Koepke, M. E., and Marchand, R. (2017). “Modeling-challenge paradigm using design of experiments (DOE) for spacecraft immersed in nonstationary, between-regimes, flowing plasma, in Active Experiments in Space: Past, Present and Future Workshop, ed N. M. Sante Fe. Available online at: https://cnls.lanl.gov/External/aefy17/15_Koepke.pdf
Kolobov, V., and Arslanbekov, R. (2012). Towards adaptive kinetic-fluid simulations of weakly ionized plasmas. J. Comput. Phys. 231, 839–869. doi: 10.1016/j.jcp.2011.05.036
Krimigis, S. M., Haerendel, G., McEntire, R. W., Paschmann, G., and Bryant, D. A. (1982). The active magnetospheric particle tracer explorers (AMPTE) program. Eos Trans. AGU 63:843. doi: 10.1029/EO063i045p00843
Lehtinen, N. G., Inan, U. S., and Bell, T. S. (2000). Trapped energetic electron curtains produced by thunderstorm driven relativistic runaway electrons. Geophys. Res. Lett. 27, 1095–1098. doi: 10.1029/1999GL010765
Lehtinen, N. G., Inan, U. S., and Bell, T. S. (2001). Effects of thunderstorm-driven runaway electrons in the conjugate hemisphere: purple sprites, ionization enhancements, and gamma rays. J. Geophys. Res. 106, 28841–28856. doi: 10.1029/2000JA000160
Lewellen, J. W., Buechler, C. B., Carlsten, B. E., Dale, G. E., Holloway, M. A., Patrick, D., et al. (2019). Space-borne electron accelerators. Front. Phys.
Li, Y., Sun, X., Ding, M. D., Qiu, J., and Priest, E. R. (2017). Imaging observations of magnetic reconnection in a solar eruptive flare. Astrophys. J. 835, 1–8. doi: 10.3847/1538-4357/835/2/190
Liang, H., Ashour-Abdalla, M., Lapenta, G., and Walker, R. J. (2016). Oxygen impacts on depolarization fronts and reconnection rate. J. Geophys. Res. 121, 1148–1166. doi: 10.1002/2015JA021747
Lin, Y., Wing, S., Johnsen, J. R., Wang, X. Y., Perez, J. D., and Cheng, L. (2017). Formation and transport of entropy stuctures in the magnetotail simulated with a 3-D global hybrid code. Geophys. Res. Lett. 44, 5892–5899. doi: 10.1002/2017GL073957
Liu, Y., Kistler, L. M., Mouikis, C. G., Klecker, B., and Dandouras, I. (2013). Heavy ion effects on substorm loading and unloading in the Earth's magnetotail. J. Geophys. Res. 118, 2101–2112. doi: 10.1002/jgra.50240
Liu, Y.-H., Birn, J., Daughton, W., Hesse, M., and Schindler, K. (2014). Onset of reconnection in the near magnetotail: PIC simulations. J. Geophys. Res. 119, 9773–9789. doi: 10.1002/2014JA020492
Lorentzen, K. R., Looper, M. D., and Blake, J. B. (2001). Relativistic electron microbursts during the GEM storms. Geophys. Res. Lett. 28:2573. doi: 10.1029/2001GL012926
Lorenzini, E., and Sanmartin, J. (2004). Tethers in space. Sci. Am. 291, 50–57. doi: 10.1038/scientificamerican0804-50
Lu, G., Brittnacher, M., Parks, G., and Lummerzheim, D. (2000). On the magnetospheric source regions of substorm-related field-aligned currents and auroral precipitation. J. Geophys. Res. 105:18483. doi: 10.1029/1999JA000365
Lucco Castello, F., Delzanno, G. L., Borovsky, J. E., Miars, G., Leon, O., and Gilchrist, B. E. (2018). Spacecraft-charging mitigation of a high-power electron beam emitted by a magnetospheric spacecraft: simple theoretical model for the transient of the spacecraft potential. J. Geophys. Res. 123:6424. doi: 10.1029/2017JA024926
Luttgen, A., and Neubauer, F. M. (1994). Generation of plasma waves by a tethered satellite system with satellites elongated in the direction of flight for arbitrary oblique geometry. J. Geophys. Res. 99, 23349–23358. doi: 10.1029/94JA01905
Mandell, M. J., Davis, V. A., Cooke, D. L., Wheelock, A. T., and Roth, C. J. (2006). Nascap-2k spacecraft charging code overview. IEEE Trans. Plasma Sci. 34, 2084–2093. doi: 10.1109/TPS.2006.881934
Marchand, R. (2012). PTetra, a tool to simulate low orbit satellite-plasma interaction. IEEE Trans. Plasma Sci. 40, 217–229. doi: 10.1109/TPS.2011.2172638
Markidis, S., and Lapenta, G. (2011). The energy conserving particle-in-cell method. J. Comput. Phys. 230, 7037–7052. doi: 10.1016/j.jcp.2011.05.033
Marshall, R. A., Nicolls, M., Sanchez, E., Lehtinen, N. G., and Neilson, J. (2014). Diagnostics of an artificial relativistic electron beam interacting with the atmosphere. J. Geophys. Res. 119, 8560–8577. doi: 10.1002/2014JA020427
Marshall, R. A., Wei, W., Kero, A., Kabirzadeh, R., and Sanchez, E. (2018). Atmospheric effects of a relativistic electron beam injected from above: chemistry, electrodynamics, and radio scattering. Front. Astron. Space Sci. 6:6. doi: 10.3389/fspas.2019.00006
Mauk, B. H., and Meng, C.-I. (1991). “The aurora and middle magnetospheric processes, in Auroral Physics, eds Meng, C-.I., Rycroft, M. J., and Frank, L. A (Cambridge, Cambridge Press), 223.
McIlwain, C. E. (1975). “Auroral electron beams near the magnetic equator,” in Physics of the Hot Plasma in the Magnetosphere, eds Hultqvist, B., and Stenflo, L (New York, NY: Plenum), 91. doi: 10.1007/978-1-4613-4437-7_5
McPherron, R. L., Russell, C. T., and Aubry, M. P. (1973). Satellite studies of magnetospheric substorms on August 15, 1968 9. Phenomenological model for substorms. J. Geophys. Res. 78:3131. doi: 10.1029/JA078i016p03131
Meierbachtol, C. S., Svyatskiy, D., Delzanno, G. L., Vernon, L. J., and Moulton, J. D. (2017). An electrostatic particle-in-cell code on multi-block structured meshes. J. Comp. Phys. 350, 796–823. doi: 10.1016/j.jcp.2017.09.016
Meng, C.-I., Mauk, B., and McIlwain, C. E. (1979). Lectron precipitation of evening diffuse aurora and its conjugate electron fluxes near the magnetospheric equator. J. Geophys. Res. 84:2545. doi: 10.1029/JA084iA06p02545
Miars, G., Delzanno, G. L., Gilchrist, B. E., Leon, O., and Lucco Castello, F. (2018). “Laboratory experiments enabling electron beam use in tenuous space plasmas,” in 2018 Fall Meeting (Washington, DC: AGU).
Mishin, E. M. (2019). Artifical aurora experiments and application to natural aurora. Front. Phys. 6:14. doi: 10.3389/fspas.2019.00014
Motoba, T., Ohtani, S., Anderson, B. J., Korth, H., Mitchell, D., Lanzerotti, L. J., et al. (2015). On the formation and origin of substorm growth phase/onset auroral arcs inferred from conjugate space-ground observations. J. Geophys. Res. 120:8707. doi: 10.1002/2015JA021676
Mozer, F. S. (1966). Proton trajectories in the radiation belts. J. Geophys. Res. 71, 2701–2708. doi: 10.1029/JZ071i011p02701
National Research Council (2012). “Magnetosphere-to-ionosphere field-line tracing technology,” in Solar and Space Physics: A Science for a Technological Society (Washington, DC: National Academies Press), 333,
Neubert, T., Banks, P. M., Gilchrist, B. E., Fraser-Smith, A. C., Williamson, P. R., Raitt, W. J., et al. (1990). The interation of an artificial electron beam with the Earth's upper atmosphere: effects on spacecraft charging and the near-plasma environment. J. Geophys. Res. 95, 12209–12217. doi: 10.1029/JA095iA08p12209
Neubert, T., Gilchrist, B., Wilderman, S., Habash, L., and Wang, H. J. (1996). Relativistic electron beam propagation in the earth's atmosphere: modeling results. Geophys. Res. Lett. 23, 1009–1012. doi: 10.1029/96GL00247
Neubert, T., and Gilchrist, B. E. (2004). Relativistic electron beam injection from spacecraft: performance and applications. Adv. Space Sci. 34, 2409–2412. doi: 10.1016/j.asr.2003.08.081
Niemann, C., Gekelman, W., Constantin, C. G., Everson, E. T., Schaeffer, D. B., Bondarenko, A. S., et al. (2014). Observation of collisionless shocks in a large current-free laboratory plasma. Geophys. Res. Lett. 41, 7413–7418. doi: 10.1002/2014GL061820
Niemann, C., Gekelman, W., Constantin, C. G., Everson, E. T., Schaeffer, D. B., Clark, S. E., et al. (2013). Dynamics of exploding plasmas in a large magnetized plasma. Phys. Plasmas 20:012108. doi: 10.1063/1.4773911
Nishimura, Y., Bortnik, J., Li, W., Thorne, R. M., Lyons, L. R., Angelopulos, V., et al. (2011). Estimation of magnetic field mapping accuracy using the pulsating aurora-chorus connection. Geophys Res Lett. 38:L14110. doi: 10.1029/2011GL048281
Nunz, G. J. (1990). Beam Experiments Aboard a Rocket (BEAR) project final report. Vol. 1 Project Summary (Los Alamos, NM: Los Alamos National Laboratory report LA-11737-MS).
Ober, D. M., Maynard, N. C., Burke, W. J., Moen, J., Egeland, A., Sandhold, P. E., et al. (2000). Mapping prenoon auroral structures to the magnetosphere. J. Geophys. Res. 105, 27519–27530. doi: 10.1029/2000JA000009
Olsen, R. C. (1985). Experiments in charge control at geosynchronous orbit-ATS-5 and ATS-6. J. Spacecraft. 22, 254–264. doi: 10.2514/3.25742
O'Shea, P. G., Butler, T. A., Lynch, M. T., McKenna, K. F., Pongratz, M. B., and Zaugg, T. J. (1991). “A linear accelerator in space – the beam experiment aboard rocket, in Proceedings of the 1990 Linear Accelerator Conference (Los Alamos, NM: Los Alamos National Laboratory), 739–742.
Palmroth, M., Ganse, U., Pfau-Kempf, Y., Battarbee, M., Turc, L., Brito, T., et al. (2018). Vlasov methods in space physics and astrophysics. Liv. Rev. Comput. Astrophys. 4:1. doi: 10.1007/s41115-018-0003-2
Poghosyan, A., and Golkar, A. (2017). CubeSat evolution: Analyzing cubesat capabilities for conducting science missions. Prog. Aerospace Sci. 88, 59–83. doi: 10.1016/j.paerosci.2016.11.002
Pongratz, M. (2018). History of Los Alamos participation in active experiments in space. Front. Phys. 6:144. doi: 10.3389/fphy.2018.00144
Porazik, P., Johnson, J. R., Kaganovich, I., and Sanchez, E. (2014). Modification of the loss cone for energetic particles. Geophys. Res. Lett. 41:8107. doi: 10.1002/2014GL061869
Prech, L., Ruzhin, Y. Y., Dokukin, V. S., Nemecek, Z., and Saftankova, J. (2018). Overview of APEX Project results. Front. Astron. Space Sci. 5:46. doi: 10.3389/fspas.2018.00046
Priest, E. R. (1986). Magnetohydrodynamic theories of solar flares. Solar Phys. 104, 1–18. doi: 10.1007/BF00159941
Pritchett, P. L. (2013). The onset of magnetic reconnection in three dimensions. Phys. Plasmas. 20, 1–4. doi: 10.1063/1.4817961
Pulkkinen, T. I., Koskinen, H. E. J., and Pellinen, R. J. (1991). Mapping of auroral arcs during substorm growth phase. J. Geophys. Res. 96:21087. doi: 10.1029/91JA01960
Qin, J., Celestin, S., and Pasko, V. P. (2012). Low frequency electromagnetic radiation from sprite streamers. Geophys. Res. Lett. 39:L22803, 1–5. doi: 10.1029/2012GL053991
Raikher, Y. L., Stepanov, V. I., and Perzynski, R. (2004). Dynamic hysteresis of a superparamagnetic nanoparticle. Phys. B. 343, 262–266. doi: 10.1016/j.physb.2003.08.105
Raitt, W. J. (1995). Stimulating our piece of the universe: active experiments in space. Rev. Geophys. 33, 559–564. doi: 10.1029/95RG00102
Rastatter, L., Kuznetsova, M. M., Sibeck, D. G., and Berrios, D. H. (2012). Scientific visualization to study flux transfer events at the Community Coordinated Modeling Center. Adv. Space Res. 49:1623. doi: 10.1016/j.asr.2011.12.034
Rhodes, C. T., Shao, X. M., Krehbiel, P. R., Thomas, R. J., and Hayenga, C. O. (1994). Observations of lightning phenomena using radio interferometry. J. Geophys. Res. 99, 13059–13082. doi: 10.1029/94JD00318
Rodger, C. K., Clilverd, M. A., Seppala, A., Thomson, N. R., Gamble, R. J., Parrot, M., et al. (2010). Radiation belt electron precipitation due to geomagnetic storms: Significance to middle atmosphere ozone chemistry. J. Geophys. Res. 115:A11320. doi: 10.1029/2010JA015599
Roelof, E. C., Mitchell, D. G., and Williams, D. J. (1985). Energetic neutral atoms (E ~ 50 keV) from the ring current: IMP 7/8 and ISEE 1. J. Geophys. Res. 90, 10991–11008. doi: 10.1029/JA090iA11p10991
Roussel, J. F., Rogier, F., Dufour, G., Mateo-Velez, J. C., Forest, J., Hilgers, A., et al. (2008). SPIS open-source code: methods, capabilities, achievements, and prospects. IEEE Trans. Plasma Sci. 36, 2360–2368. doi: 10.1109/TPS.2008.2002327
Roytershteyn, V., Boldyrev, S., Delzanno, G. L. K., Chen, C., Grošelj, D., Loureiro, N. F., et al. (2019). Inertial kinetic Alfvén turbulence. Astrophys. J, 870, 1–9. doi: 10.3847/1538-4357/aaf288
Roytershteyn, V., and Delzanno, G. L. (2018). Spectral approach to plasma kinetic simulations based on hermite decomposition in the velocity space. Front. Astron. Space Sci. 5:27. doi: 10.3389/fspas.2018.00027
Sanchez-Arriaga, G., and Sanmartin, J. R. (2010). Magnetic pumping of whistler waves by tether current modulation. J. Geophys. Res. 115:A03311. doi: 10.1029/2009JA014478
Sanmartin, J. R. (2010). A review of electrodynamic tethers for science applications. Plasma Sour. Sci. Technol. 19:034022. doi: 10.1088/0963-0252/19/3/034022
Sanmartin, J. R., Charro, M., Pelaez, J., Tinao, I., Elaskar, S., Hilgers, A., et al. (2006). Floating bare tether as an upper atmosphere probe. J. Geophys Res. 111:A11310. doi: 10.1029/2006JA011624
Scherbarth, M., Smith, D., Adler, A., Stuart, J., and Ginet, G. (2009). AFRL's Demonstration and Science Experiments (DSX) mission. Proc. SPIE. 7438:74380B–1. doi: 10.1117/12.824898
Scime, E. E., and Keesee, A. M. (2018). Enhancing energetic neutral atom imaging. Front. Astron. Space Sci. 6:9. doi: 10.3389/fspas.2019.00009
Sergeev, V., Nishimura, Y., Kubyshkina, M., Angelopoulos, V., Nakamura, R., and Singer, H. (2012a). Magnetospheric location of the equatorial pregreakup arc. J. Geophys. Res. 117:A01212. doi: 10.1029/2011JA017154
Sergeev, V. A., Angelopouls, V., and Nakamura, R. (2012b). Recent advances in understanding substorm dynamics. Geophys. Res. Lett. 39:L05101. doi: 10.1029/2012GL050859
Shevchenko, I. G., Sergeev, V., Kubyshkina, M., Angelopoulos, V., Glassmeier, K. H., and Singer, H. J. (2010). Estimation of magnetosphere- ionosphere mapping accuracy using isotropy boundary and THEMIS observations. J. Geophys. Res. 115:A11206. doi: 10.1029/2010JA015354
Siguier, J.-M., Sarrailh, P., Roussel, J.-F., Inguimbert, V., Murat, G., and Sanmartin, J. (2013). Drifting plasma collection by a positive biased tether wire in LEO-like plasma conditions: current measurements and plasma diagnostic. IEEE Trans. Plasma Sci. 41, 3380–3386. doi: 10.1109/TPS.2013.2257871
Sonnenfeld, R. G., and Hager, W. W. (2013). Electric field reversal in sprite electric field signature. Monthy Weath Rev. 141, 1731–1735. doi: 10.1175/MWR-D-12-00220.1
Sugiyama, T., and Kusano, K. (2007). Multi-scale plasma simulation by the interlocking of magnetohydrodynamic model and particle-in-cell kinetic model. J. Comp. Phys. 227, 1340–1352. doi: 10.1016/j.jcp.2007.09.011
Summers, D., Thorne, R. M., and Xiao, F. (1998). Relativistic theory of wave-particle resonant diffusion wit application to electron acceleration in the magnetosphere. J. Geophys. Res. 103, 20487–20500. doi: 10.1029/98JA01740
Swift, D. W. (1978). Mechanisms for the discrete aurora – a review. Space Sci. Rev. 22:35. doi: 10.1007/BF00215813
Tejero, E. M., Amatucci, W. E., Ganguli, G., Cothran, C. D., Crabtree, C., and Thomas, E. (2011). Spontaneous electromagnetic emission from a strongly localized plasma flow. Phys. Rev. Lett. 106:185001. doi: 10.1103/PhysRevLett.106.185001
Tejero, E. M., Crabtree, C., Blackwell, D. D., Amatucci, W. E., Mithaiwala, M., Ganguli, G., et al. (2015). Nonlinear generation of electromagnetic waves through induced scattering by thermal plasma. Sci. Rep. 5:17852. doi: 10.1038/srep17852
Thomas, J. N., Holzworth, R. H., and Chin, J. (2004). A new high-Voltage electric field instrument for studying sprites. IEEE Trans. Geosci. Remote Sens. 42, 1399–1404. doi: 10.1109/TGRS.2004.826806
Tóth, G., Jia, X., Markidis, S., Peng, I. B., Chen, Y., Daldorff, L. K. S., et al. (2016). Extended magnetohydrodynamics with embedded particle-in-cell simulation of Ganymede's magnetosphere. J. Geophys. Res. 121, 1273–1293. doi: 10.1002/2015JA021997
Unan, I., and Rietveld, M. T. (1995). Preface active experiments in space physics. Adv Space Res. 15:1.
Uspensky, M. V., Timopheev, E. E., and Sverdlov, Y. L. (1980). “Araks” Doppler radar measurements of the ionospheric effects of artificial electron beam in the North hemisphere. Ann. Geophys. 36, 303–312.
Van Compernolle, B., An, X., Bortnik, J., Thorne, R. M., Pribyl, P., and Gekelman, W. (2015). Excitation of chirping whistler waves in a laboratory plasma. Phys. Rev. Lett. 114:245002. doi: 10.1103/PhysRevLett.114.245002
Vencels, J., Delzanno, G. L., Manzini, G., Markidis, S., Peng, I. B., and Roytershteyn, V. (2016). SpectralPlasmaSolver: a spectral code for multiscale simulations of collisionless, magnetized plasmas. J. Phys. 719:012022. doi: 10.1088/1742-6596/719/1/012022
Verronen, P. T., Andersson, M. E., Rodger, C. F., Clilverd, M. A., Wang, S., and Turunen, E. (2013). Comparison of modeled and observed effects of radiation belt electron precipitation on mesospheric hydroxyl and ozone. J. Geophys. Res. 118, 11419–11428. doi : 10.1002/jgrd.50845
Walsh, B. M., Foster, J. C., Erickson, P. J., and Sibeck, D. G. (2014). Simultaneous ground- and space-based observations of the plasmaspheric plume and reconnection. Science 343:1122. doi: 10.1126/science.1247212
Wang, L., Hakim, A. H., Bhattacharjee, A., and Germaschewski, K. (2015). Comparison of multi-fluid moment models with particle-in-cell simulations of collisionless magnetic reconnection. Phys. Plasmas 22:012108. doi: 10.1063/1.4906063
Weiss, L. A., Thomsen, M. F., Reeves, G. D., and McComas, D. J. (1997). An examination of the Tsyganenko (T89A) field model using a database of two-satellite magnetic conjunctions. J. Geophys. Res. 102:4911. doi: 10.1029/96JA02876
Whitley, T., Fullenkrug, M., Rycroft, M., Bennett, A., Wyatt, F., Elliott, D., et al. (2011). Worldwide extremely low frequency magnetic field sensor network for sprite studies. Radio Sci. 46, 1–9. doi: 10.1029/2010RS004523
Winckler, J. R. (1992). Controlled experiments in the Earth's magnetosphere with artificial electron beams. Rev. Mod. Phys. 64, 859–871. doi: 10.1103/RevModPhys.64.859
Winske, D., Huba, J. D., Niemann, C., and Le, A. (2019). Recalling and updating research on diamagnetic cavities: experiments, theory and simulations. Front. Astron. Space Sci. 5:51. doi: 10.3389/fspas.2018.00051
Keywords: active space experiments, plasma physics, magnetospheres, ionosphere, laboratory astrophysics, space physics
Citation: Borovsky JE and Delzanno GL (2019) Active Experiments in Space: The Future. Front. Astron. Space Sci. 6:31. doi: 10.3389/fspas.2019.00031
Received: 01 December 2018; Accepted: 08 April 2019;
Published: 09 May 2019.
Edited by:
Rudolf von Steiger, University of Bern, SwitzerlandReviewed by:
Anton Artemyev, Space Research Institute (RAS), RussiaGeorgios Balasis, National Observatory of Athens, Greece
Copyright © 2019 Borovsky and Delzanno. This is an open-access article distributed under the terms of the Creative Commons Attribution License (CC BY). The use, distribution or reproduction in other forums is permitted, provided the original author(s) and the copyright owner(s) are credited and that the original publication in this journal is cited, in accordance with accepted academic practice. No use, distribution or reproduction is permitted which does not comply with these terms.
*Correspondence: Joseph E. Borovsky, jborovsky@spacescience.org