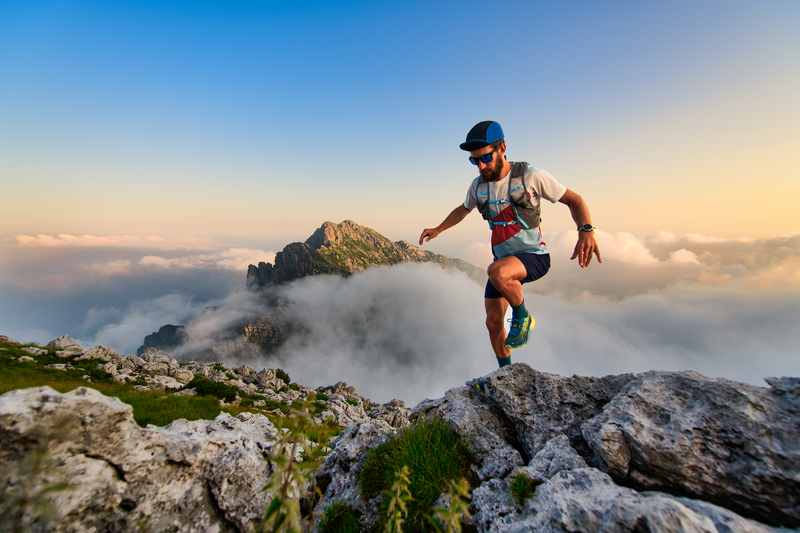
95% of researchers rate our articles as excellent or good
Learn more about the work of our research integrity team to safeguard the quality of each article we publish.
Find out more
HYPOTHESIS AND THEORY article
Front. Sustain. Food Syst. , 26 March 2025
Sec. Nutrition and Sustainable Diets
Volume 9 - 2025 | https://doi.org/10.3389/fsufs.2025.1564425
This article is part of the Research Topic Innovative and Sustainable Management of Organic Food and Beverage Wastes View all articles
With a growing global population, ways to counterbalance the demand for meat products with effective food security and waste management demand innovative and scalable solutions. Concurrently, the alarming incidence of end-stage organ failure, limited availability of transplantable organs, and directives to reduce reliance on animal testing underscore the need for clinically viable and sustainable alternatives. Our approach introduces a hypothesis-driven, renewable tissue engineering strategy that creates low-cost keratoplasty models derived entirely from agri-food waste. Specifically, we hypothesize that abundant meat by-products, such as eyes and bladders, provide practically unlimited and readily available supplies of corneal tissues and urine-derived stem cells (USCs) that can be repurposed into cost-effective, clinically relevant solutions. Traditional approaches often rely on cadaveric tissues, invasive cell sourcing, or expensive commercial stem cell lines, which require complex and resource-intensive processes, including high-end bioreactor systems and manufacturing environments. These requirements often limit the widespread adoption and technological progress needed to increase the global supply of keratografts. Our proposed strategy leverages a combination of post-mortem corneal and bladder harvesting, which in turn facilitates tissue decellularization, non-invasive USC sourcing, stem cell differentiation, and compartment-specific recellularization approaches to help overcome barriers associated with traditional cell seeding and generate keratoplasty models derived entirely from this type of waste. Overall, our perspective suggests a way to devise a transformative and resource-efficient approach to tissue engineering, specifically geared toward improving keratoplasty outcomes while offering broader applications for the regeneration of other bodily tissues/organs and biotechnological innovation.
Agri-food systems rely on various extraction, storage, and distribution processes to support human consumption and waste management. With the growing emphasis on sustainability, these practices are being revised to ensure food security through better-integrated supply chains focused on biovalorization and circular bioeconomy principles. Recent studies highlight the potential of sustainable integrated food chains to transform organic waste and biomass into valuable products, including biomaterials (Ashokkumar et al., 2022; Khan et al., 2023; Tarafdar et al., 2021; Limeneh et al., 2022; Shibru et al., 2024).
The use of natural components for tissue and organ engineering is a growing focus in regenerative medicine. One critical application is addressing the global need for keratoplasty. An estimated 12.7 million people worldwide require corneal transplants, yet only one donor cornea is available for every 70 patients (Romano et al., 2024). The cornea, a thin, transparent tissue, plays essential roles in light propagation, protection, immunological defense, and sensory perception. While treatments like eye drops, implants, and medications can address mild corneal damage, severe cases often necessitate transplantation.
Advances in regenerative medicine have enabled the development of corneal substitutes using bovine, porcine, ovine, and cadaveric tissues through techniques such as additive manufacturing (Jia et al., 2023), decellularization/recellularization (Nara et al., 2016; Polisetti et al., 2021), stem cell-based techniques (El Zarif et al., 2020), and xenografting (Ali et al., 2024a). However, these methods often rely on invasive cell sourcing, expensive stem cell lines, and complex manufacturing processes, limiting scalability and accessibility.
Urine-derived stem cells (USCs) have emerged as a promising, non-invasive, and multipotent alternative for regenerative applications. These adult stem cells exhibit high proliferative capacity and can be directly differentiated into multiple cell types, including epithelial, fibroblast, and endothelial cells (Liu et al., 2018; Yin et al., 2024; Sun et al., 2024; Sridhar, 2018). Unlike mesenchymal stem cells (MSCs) or fibroblasts, USCs are easier to reprogram into induced pluripotent stem cells (iPSCs), i.e., urine-derived iPScs (u-iPSCs), maintaining their genetic characteristics and offering a versatile platform for tissue engineering (Yin et al., 2024; Yu et al., 2024; Jing et al., 2019; Bento et al., 2020). Building on our prior efforts to develop scalable platforms for decellularized corneal scaffolds using slaughterhouse waste (Ali et al., 2024b; Pantic et al., 2023; Wang et al., 2023b), our hypothesis centers on devising clinically relevant pathways that can be used to establish bioengineered keratografts exclusively using agri-food waste from major meat sources. As shown in Figures 1–3, our approach combines simplified decellularization, bespoke recellularization, and advanced stem cell technologies, offering cost-effective solutions. These results provide a scalable and ethical framework for developing corneal model tissues for tissue engineering research. While this approach can inform future clinical strategies, the primary goal is to generate model tissues that can support biomaterial validation, structural and functional studies, and ophthalmic drug testing rather than direct transplantation. Future studies would be required to evaluate immunogenicity and clinical feasibility.
Figure 1. The figure depicts our proposed strategy to establish bioengineered keratografts exclusively using agri-food waste from major meat sources, including organ extraction, USC isolation, expansion, and corneal decellularization.
Figure 2. An illustration of the proposed bespoke approach designed to overcome conventional cell seeding challenges, including suboptimal cell adhesion, migration, penetration, retention, and uniformity. The proposed method combines topical and injection-based seeding techniques with region-specific scaffold modifiers to enhance critical epithelial, stromal, and endothelial recellularization parameters.
Figure 3. Corneal xenografts generated from discarded slaughterhouse ocular tissues. (A) An image of a freshly slaughtered ovine carcass, (B) A whole ovine eye extracted from the slaughtered animal, (C) Dissection of the corneal tissue from the whole eye to collect the intact cornea, (D) Histological section of a native cornea showing intact cellular structure, and (E) Histological section of a decellularized corneal scaffold demonstrating the removal of cellular components while retaining the extracellular matrix structure.
We hypothesize that a sustainable keratoplasty model can be developed by repurposing agri-food waste, such as bovine, porcine, or ovine corneas, into decellularized extracellular matrix (dECM) scaffolds and reseeding them with urine-derived stem cells (USCs). This approach leverages the regenerative potential of USCs to differentiate into corneal-specific lineages (epithelial, stromal, and endothelial cells) and the structural integrity of dECM to support functional grafts. By addressing the critical shortage of donor tissues, this model also aligns with circular bioeconomic practices, reducing environmental waste and promoting medical innovation.
• To examine how non-invasively sourced, multipotent USCs can be used to generate the three major cellular lineages within the cornea (endothelial, keratocytes, and endothelial cells) via direct differentiation or pluripotent iPSC-based reprogramming;
• To devise a unique corneal recellularization approach tailored to each major cellular compartment; and
• To qualitatively and quantitatively evaluate the keratograft morphology and functionality post-recellularization.
It is essential first to establish agreements with local abattoirs to ensure target organs, in this case, intact bladders and eyes, are collected effectively and ethically. This process will support quality control for downstream applications, such as stem cell isolation and tissue engineering. Specifically, researchers are required to obtain the relevant approvals from their institutional animal care and use committee (IACUC), internal review board (IRB), and animal ethics committee to collect and utilize animal byproducts to ensure compliance with municipal regulatory bodies, their home institutions, and international scientific guidelines (e.g., OECD and ARRIVE guidelines). After such agreements have been established, coordination and slaughterhouse employee training sessions are required to collect freshly harvested organs properly. It is also important to define areas designated for collection and initial processing to minimize contamination and uphold food safety standards. This systematic process is depicted in Figure 1.
Stem cell collection and viability can be assessed through a series of processes outlined as follows:
• Pre-sterilized equipment. The following surgical tools, containers, and solutions should be allocated: forceps, scalpels, scissors, syringes, needles, gloves, and storage bags containing phosphate-buffered saline enriched with antibiotics.
• Personal protective equipment (PPE) and sanitization. Research personnel should also be equipped with protective eye wear and disposable surgical caps, gowns, and shoe covers, and the designated area should be thoroughly sanitized using 70% ethanol.
• Animal selection, bowel evisceration, and enucleation. With the aid of abattoir staff, viable animals will be identified during the initial phase of meat processing. During this phase, animals are generally slaughtered via swift, deep incisions that sever jugular veins, carotid arteries, and windpipe for rapid death and blood drainage. After which, their hides will be removed, midline incisions performed, and abdominal cavities opened for bladder isolation and dissection by first gently separating this organ from its surrounding tissues, ligating the ureters proximal to bladder entry points to prevent urine leakage, and severing the urethra at its base. Likewise, intraocular muscles surrounding the eyeballs will be sectioned, and the eyeball can be gently advanced from its socket to limit compression. Finally, the optic nerve can then be severed at the base of the eye, and the organ carefully removed from the orbit.
• Post-harvesting preservation and transportation. Connective tissues will be dissected, and the organs will be thoroughly rinsed with the antibiotic-supplemented buffer to remove residual blood and other bodily fluids. The organs will be then inspected for physical damage and contamination and discarded if either event occurs. Properly extracted eyes and bladders are sealed in the containers with the sterile buffer and placed on ice in coolers for transportation.
• Urine extraction. Upon arrival at the sterile research facility, the bladders and eyes can again be rinsed with antibiotic-enriched saline. 16-20 Fr Foley catheter connected to urine collection bags can be inserted through the bladder wall to aspirate the urine slowly. The extracted urine can then be transferred to sterile chambers containing the antibiotic-supplemented buffer.
• Corneal extraction. The cornea can be extracted from the eyeballs by making an initial incision in the sclera, advancing this cut circumferential along the limbus, and placing this tissue in culture dishes containing the antibiotic-supplemented buffer.
USC collection and viability, as well as u-iPSCs reprogramming, can be assessed through the following series of processes:
• USC isolation (Zhou et al., 2022). The urine samples can be transferred to 50 ml sterile canonical tubes where they will be subjected to repeated centrifugation (400 × g for 10 min at room temperature, supernatant aspiration, and pellet resuspension in the antibiotic-supplemented buffer to ensure removal of debris and contaminants. Under normal conditions, the following cell types can be found in the urine: squamous, transitional, and renal tubular epithelial cells; white blood cells; macrophages; red blood cells; urine-derived stem cells; urothelial and renal progenitor cells; caudate cells; fungi; and male/female reproductive cells (Sullivan et al., 2010). Generally, USCs are adherent in culture, and only a few progenitor cells adhere and proliferate, and the remaining non-adherent cell types can be removed during media passages. Moreover, sorting for USCs is achieved by selecting adherent, spindle-shaped colonies and confirming their identity using stem cell-specific markers outlined below.
• USC expansion (Kim et al., 2020). Further centrifugation can be performed to produce a new pellet that will be resuspended in a primary medium containing Dulbecco's Modified Eagle's Medium (DMEM)/Ham's Nutrient Mixture F12 consisting of 10% FBS, 1% penicillin/streptomycin and 0.5 ml urine renal epithelial growth medium supplemented with or without Y-27632 on gelatin-coated culture plates. Thereafter, expansion can be supported using USCs, a proliferation medium consisting of 50% DMEM (high glucose consisting of 10% FBS, 1% penicillin/streptomycin), and 50% renal epithelial growth medium (REGM) under standard culture conditions (37°C, 5% CO2) for 7–10 days with medium changes every 2–3 days to maintain nutrient supply and remove non-adherent, non-target cells.
• USC multipotency verification (Sun et al., 2024; Bento et al., 2020; Pavathuparambil Abdul Manaph et al., 2018; Sato et al., 2019). Established methods to evaluate cellular potency rely on common microscopic analyses and immunoassays. Brightfield, phase contrast, and or fluorescent microscopy can be used to evaluate the presence of adherent clones with phenotypical structures, while clusters of differentiation (CD) techniques can be used to evaluate surface marker phenotypes via the expression of CD73, CD90, CD105, and CD133, as well as the absence of CD45, CD31, and CD34, which are common MSC markers within expanded USCs. USCs often also express pluripotent stem cell makers, including POU5FI, Oct 3/4, c-Myc, SSEA 1/4, and Klf 4.
• USC reprogramming to u-iPSCs (Yin et al., 2024; Baghbaderani et al., 2016). USC reprogramming can be efficiently and cost-effectively achieved within a period of 14 days via the viral and non-viral introduction of Yamanaka transcription factors (Oct 4, Sox 2, Klf 4, and c-Myc). After which, pluripotency can be verified via the presence of surface antigens such as SSEA3/-4, Tra-1-60, and Tra-1-81.
• Formation of epithelial, keratocytes, and endothelial lineages (Zhou et al., 2022). Table 1 summarizes a series of approaches that can be used to differentiate USCs into corneal epithelial, keratocyte, and endothelial lineages that vary in complexity and benefit.
Table 1. An overview of techniques required to generate corneal cell lineages using non-evasively collected USCs.
Previously established protocols rely on immersion-based techniques using chemical (ionic or non-ionic detergents, enzymes, acids/bases, alcohols) and/or physical (free-thaw cycles, agitation, electroporation, osmotic shock, and sonication). For this high-throughout process, we can utilize protocols that define optimized mechanical agitation of the multiple corneal sections in low concentration (1–4%) environmentally friendly, plant-based detergents on laboratory shakers set at 300–800 rpm over a period of 1 week to generate dECM scaffolds for research applications (Pantic et al., 2023; Wang et al., 2023b), supplemented with dextran treatment (5%−20%) to regulate swelling (Polisetti et al., 2021). Following this, the dECM can be processed into hydrogels within an additional week through solubilization, neutralization, gelation, and cross-linking processes (Shibru et al., 2024; Zeng et al., 2022; Zhang et al., 2023). Subsequent characterizations will include (Polisetti et al., 2021; Ali et al., 2024b; Pantic et al., 2023; Wang et al., 2023b; Dong et al., 2019; Hamedi et al., 2024):
• Biochemical assessments. DNA quantifications to confirm effective decellularization;
• Biomechanical measurements. Rheological analyses to evaluate the mechanical properties of the gel;
• Biocompatibility tests. Cell viability, adhesion, proliferation, and differentiation studies to examine scaffold suitability; and
• Structural analyses. Hematoxylin-eosin (H&E), toluidine blue, and Masson's trichrome assess the structural integrity of the dECM; and
• Optical transparency. Light transmittance will be evaluated within the visible range (380–700 nm).
Topical cell seeding remains the most widely adopted method for corneal recellularization. Despite its simplicity, it has several drawbacks, like suboptimal cell adhesion, migration, penetration, retention, and uniformity. We propose a bespoke recellularization approach to address these challenges and introduce the concept of hydrodynamic injections of suspensions containing cells and adhesion factors. This process provides rapid delivery (0.5 ml) within 5 s (Corridon, 2023; Corridon et al., 2013) to support uniform distribution throughout thicker tissue sections (Suda et al., 2023). The primary goal of this model is to develop corneal scaffolds for tissue engineering research rather than for direct transplantation. It leverages the benefits of topical- and injection-based seeding with region-specific scaffold modifiers to enhance these critical parameters. This systematic strategy, illustrated in Figure 2, is outlined as follows:
• Sterilization. Gamma irradiation and ethylene oxide are preferred choices, but their substantial costs limit their applications. Alternatively, we propose an established, low-cost, albeit less effective combination of immersion in 70% ethanol or 0.1–0.3% peracetic acid for 30 min and UV irradiation for 30 min.
• Epithelial, stromal, and endothelial adhesion proteins. Reseeding the scaffold with suspensions of various cell lineages along with their region-specific molecules to support cellular adhesion, uniformity, penetration, retention, and migration can be an effective strategy to enhance recellularization and facilitate integration, retention and uniform distribution (apart from the stroma, in which keratocyte density is relatively low and varies significantly across anterior and posterior layers, as well as central and peripheral regions), as presented below.
• Epithelial reseeding
° Scaffold pre-treatment (Hosseinikhah et al., 2024). Coat the dECM scaffold with a solution containing laminin and fibronectin to promote cellular adhesion. Incorporate RGD peptides into the coating solution to support integrin-mediated adhesion;
° Scaffold injection and cell seeding (Guindolet et al., 2021; Sotozono et al., 1995). Hydrodynamically inject a cocktail of USC-derived epithelial cells (>2,000 cells/mm2), keratinocyte and epidermal growth factors, along with retinoic acid, into the scaffold to support migration, uniformity, and proliferation; and
° Incubation. Create an air-liquid interface to mimic physiological conditions by exposing the injected side to air and keeping the stromal/endothelial region in contact with the culture medium.
• Stromal reseeding
° Scaffold pre-treatment. Coat the dECM scaffold with hyaluronic acid and fibronectin to enhance cellular adhesion and retention. Incorporate RGD peptides into the scaffold to facilitate cell attachment and penetration;
° Scaffold injection and cell seeding (Zheng et al., 2016; Patricelli et al., 2023). Inject a solution under hydrodynamic conditions containing pre-differentiated keratocytes (> 1,000 cells/mm2), TGF-β (5 ng/ml), and ascorbic acid (100 μg/ml) into the scaffold to promote ECM synthesis and keratocyte migration; and
° Incubation. Maintain the air-liquid interface conditions.
• Endothelial reseeding
° Scaffold pre-treatment. Coat the posterior surface of the dECM scaffold with collagen IV and fibronectin to mimic the native Descemet's membrane and enhance cellular adhesion;
° Scaffold Injection (Zheng et al., 2016; Eyre et al., 2021; Wimmer et al., 2012; Lv et al., 2023). Again, under hydrodynamic conditions, inject a cocktail containing VEGF (40–50 ng/ml), FGF2 (40–50 ng/ml), and the rho kinase inhibitor, Y-27632 (10–20 μM), and USC-derived endothelial cells (<500 cells/mm2); and
° Incubation. With the established air-liquid interface, rotate the culture chamber (low rotation speeds: 1–10 rpm) to improve even cellular distribution and attachment.
Thereafter, processes geared toward validation and verification outlined in Table 1, as well as biochemical, biomechanical, biocompatibility, morphological, and optical assessments, can be employed to evaluate the recellularization strategy.
In order to assess the validity of the hypothesis, it is necessary to evaluate the stages of the tissue engineering strategy. The first involves post-mortem urine and corneal tissue collection, during which standard colorimetric (pale yellow), pH (5.0–8.0), creatinine (0.4–1.2 g/dl), and microbial urinalyses (Ct >35 or no Ct value (NA) are considered as negative and indicate the absence of a given pathogen), can confirm the quality of the urine samples. Common agri-food pathogens can include bacterial (salmonella), parasitic (toxoplasma), fungal (candida), viral (hepatitis), and prion proteins.
Likewise, previously outlined biochemical (residual DNA < 50 ng/mg), biomechanical (G″ < G′), and structural analyses (nuclei absent and ECM component retention rate >70–80%) with their respective thresholds can be applied to the examine native corneal tissues along with optical transparency measurements (transmittance levels >85% within the visible range), biocompatibility (cell viability, proliferation, and differentiation rates >70–80%), and microbial testing. This information can be supplemented with animal demographics, health, welfare, and microbial data supplied by the abattoir to support experimental reproducibility and keratograft development.
The second stage involves isolating and transforming USCs into u-iPSCs, as well as epithelial, fibroblast, and endothelial cells. Each target cell type's identification, quantification, and function can be confirmed using additional assays that evaluate cell viability and homogeneity via their endocytic (high rate of low-weight molecular vesicular internalization), colony-forming/proliferative (≥10 CFUs), adhesive (>75% adhesion efficiency), and migratory (appreciable displacement within a 24-h period) capacities.
The remaining two stages of keratoplasty development rely on the production of dECM scaffolds and hydrogels and compartment-specific dECM recellularization. These structures provide natural microenvironments for corneal cells and the retention of essential epithelial and endothelial (collagen type IV, laminin, and fibronectin) and stromal (collagen type I, GAGs, and proteoglycans) ECM components (Espana and Birk, 2020; Wang et al., 2023a), which facilitate the major barrier and transmission functions of the cornea. Other simpler and more cost-effective means can be employed to evaluate optical clarity, using laser pointer beam scattering and smartphone light intensity mobile applications.
In this article, we propose an approach that combines decellularization, stem cells, and bespoke recellularization technologies to economically generate vast quantities of keratoplasty models solely from discarded animal eyes and bladders. Such progress may ultimately lead to innovations that can increase the global supply of keratografts. Simultaneously, this approach offers a scalable solution to counterbalance the demand for meat products with new waste management strategies.
Corneal diseases and injuries are one of the leading causes of blindness worldwide, and several barriers limit keratoprosthesis development, including adequate access to corneal tissues, high economic costs, limited technological scalability, and ethical and regulatory issues. Recent advances in ophthalmic tissue engineering have highlighted the dECM as a highly suitable substrate for keratoprostheses with various advantages over conventional allografts. Compared to synthetic or other biofabricated scaffolds, the dCEM best mimics the endogenous tissue environment, preserving essential extracellular components such as collagen types I and IV, laminins, fibronectin, and GAGs, which are essential for scaffold function and long-term cellular integration (Taylor et al., 2018). The interaction between these elements of the ECM matrix with the surrounding cells plays a significant role in tissue differentiation, migration, adhesion, and proliferation (Yue, 2014; Corridon et al., 2006).
Previous studies have demonstrated that ECM retention is crucial for maintaining the bioactivity of acellular scaffolds and ensuring their effectiveness as biomaterials for ophthalmic applications (Brown et al., 2010; Naba et al., 2017; Spang and Christman, 2018; Ott et al., 2008). For instance, results from Dai et al., have shown that this environment supports cellular viability for periods exceeding 3 months (Dai et al., 2024), and can be an ideal dynamic environment for directly differentiated USCs or those reprogrammed into u-iPSCs to regenerate functional and structurally intact corneal epithelial, stromal, and endothelial compartments for keratografts that can provide superior optical properties post-transplantation while serving as test beds for enhancements. Nevertheless, one of the challenges in dECM-based tissue engineering is the loss of soluble matrix proteins, including glycoproteins and proteoglycans, which play key roles in cell adhesion and bioactivity (Harris et al., 2018). To mitigate this, studies by Lynch et al. have outlined incorporating dextran treatment (5%−20%) into the decellularization process to reduce the risk of excessive swelling and preserve ECM hydration and integrity (Lynch et al., 2016).
In so doing, we have established a framework to develop keratoplasty models derived entirely from agri-food waste. As this research outlines, bioartificial keratoprostheses can offer enhanced customizability for broader clinically relevant applications. The applications we propose will also help reduce the reliance on donor tissues, as well as the number of live animal studies needed to advance these processes. According to Bron et al. (Brown et al., 2010), acellular bladder matrices, which have been widely studied as ECM-based scaffolds, offer insights into the importance of matrix composition in cell adhesion and tissue remodeling. Similarly, the proteomic characterization of ECM-derived scaffolds, as highlighted in previous work by Naba et al. (2017), reinforces the importance of biochemical analysis in ensuring scaffold integrity. While full proteomic profiling was not conducted in this study, our results confirm the retention of key ECM proteins post-decellularization, supporting scaffold biocompatibility and mechanical stability.
Although a full life-cycle analysis (LCA) is beyond the scope of this study, our recent work on integrated environmental and health economic assessments of xeno-keratoplasty provides evidence of the environmental and economic impact of tissue-engineered alternatives (Ali and Corridon, 2024). This study compares native corneal use and biofabricated scaffolds, providing a structured framework for assessing the sustainability of xeno-keratoplasty models. Additionally, Table 2 provides a technoeconomic analysis (TEA), which evaluates material sourcing, energy use, and scalability constraints in biomaterial preparation. Future studies will further explore LCA metrics to quantify resource efficiency across different biomanufacturing processes. This approach can undoubtedly lower production costs and support research in wider regions. This work seeks to develop a novel sustainable tissue engineering strategy built on the use of USC-based differentiation, which is significantly more cost-effective than commercial stem cell lines, as outlined in our estimated comparison with conventional approaches that use commercially available stem cell lines. This affordability makes it an attractive alternative for large-scale or resource-limited research applications, particularly when leveraging agri-food waste for sustainable tissue engineering and further highlighting the benefits of a direct differentiation approach.
Table 2. Comparison of associated costs and production timelines for the various stages of the tissue engineering strategy for the hypothesized and conventional approaches.
Needless to say, this approach has its limitations. First, intra- and inter-species variations should be controlled to limit their effect on scientific reproducibility by standardizing protocols, processing samples in batches, and incorporating sample randomizations and cross-species validations. Second, Even though the dECM corneal scaffold mimics the natural eye, its integrity, and thus functionality, can be compromised during decellularization and recellularization. Non-uniform cellular adhesion, proliferation, differentiation, and reduced intrinsic interactions in the post-transplantation environment remain challenges. Studies on ECM degradation kinetics in other tissue models, including vascular and bladder scaffolds, indicate that biochemical optimization of decellularization techniques can help mitigate these issues (Ott et al., 2008). Third, urine is a sterile body fluid, but there is still a risk of contamination during the collection process. This risk is particularly relevant when translating our experimental models to non-invasive collection from patients, regardless of age and gender, as contamination can arise from intrinsic animal/patient infections or environmental antigen invasion (Yu et al., 2023). Furthermore, direct collection can also be compromised in the case of anuria. Fourth, there is also the potential for immunogenic and tumorigenic risks. While USCs display telomerase activity and are generally non-tumorigenic (Zhang et al., 2014), this property may be lost after reprogramming into u-iPSCs, making direct differentiation preferable. Finally, beyond existing microscopy and genetic analyses, which can help understand and overcome these limitations, machine learning assessments are also being explored to advance technological development at a faster and more reliable rate. These computational approaches can provide high-throughput evaluations to refine scaffold production, optimize decellularization/recellularization efficiencies, and improve biomaterial standardization in tissue engineering research (Pantic et al., 2023; Jeznach et al., 2024).
If validated, this hypothesis can have broader applications for other organ systems, demonstrating its scalability and contribution to circular bioeconomy practices. Key regulatory considerations, particularly concerning the use of agri-food waste for medical applications, must be addressed to ensure compliance and safety. Additional collaborations with biobanks and stakeholders within the agri-food supply chain will be essential for scalable tissue sourcing and potential clinical translation.
Our sustainable tissue engineering strategy repurposes slaughterhouse waste tissues, such as bladders and whole eyes, to generate dECM scaffolds and USCs, providing ethical and resource-efficient foundations for innovation. This hypothesis paper proposes direct USC differentiation and USC reprogramming into u-iPSC and compartment-specific recellularization techniques to reseed the acellular corneal epithelium, stroma, and endothelium, which are crucial steps for keratograft development. This hypothesis is supported by previously devised high-throughput scaffolding methods that repurposed bovine, ovine, and porcine ocular tissues. Evidence is also from established protocols and adapted techniques to support stem cell allocation, expansion, differentiation, and hydrodynamic-based recellularization. Rigorous preclinical testing via long-term in vitro and in vivo studies is required to evaluate the hypothesis. As outlined, the main objective of this model is to create corneal scaffolds for tissue engineering research rather than for direct transplantation. These studies will assess procedural consistency and effectiveness, and can be used to gauge the feasibility of our approach and devise a pathway to address critical shortages in the global supply of viable keratoprostheses. Integrating advanced tissue engineering with waste-derived materials offers a transformative pathway for the treatment of end-stage ophthalmic disorders using corneal dECMs and non-invasively sourced stem cells. If validated, this model can potentially transform the keratoplasty landscape by providing affordable, scalable, and sustainable solutions. By redefining the use of agri-food waste in tissue engineering, our strategy offers a pathway to meet the rising global demand for keratoprostheses. It can also set a precedent for future regenerative medicine and bioengineering applications that can be applied to other tissues and organs.
The original contributions presented in the study are included in the article/supplementary material, further inquiries can be directed to the corresponding author.
The animal study was reviewed and approved by the Animal Research Oversight Committee at Khalifa University of Science and Technology.
PC: Conceptualization, Data curation, Formal analysis, Funding acquisition, Investigation, Methodology, Project administration, Resources, Software, Supervision, Validation, Visualization, Writing – original draft, Writing – review & editing. AM: Conceptualization, Writing – original draft. ZH: Conceptualization, Writing – original draft. JP: Conceptualization, Writing – original draft. SV: Conceptualization, Writing – original draft. IP: Conceptualization, Writing – original draft.
The author(s) declare that financial support was received for the research and/or publication of this article. This project was supported by funds granted to PC from Khalifa University of Science and Technology, grant numbers ESIG-2023-005 (8474000476) and KU-9622 (8474000655), Center for Biotechnology, Health Engineering Innovation Group and College of Medicine and Health Sciences. The project was also funded by support granted to PC from the Abu Dhabi Automated Slaughterhouse, Municipality of the City of Abu Dhabi. IP and JP acknowledge support from the Ministry of Education and Science of the Republic of Serbia, grant no. 451-03-66/2024-03/200110.
We recognize their staff, particularly Dr. Anas Baroudi and Dr. Hassan Mohamed Hassanein, for their aid in examining slaughterhouse practices relevant to this study and the long-term support of this research initiative.
The authors declare that the research was conducted in the absence of any commercial or financial relationships that could be construed as a potential conflict of interest.
The author(s) declared that they were an editorial board member of Frontiers, at the time of submission. This had no impact on the peer review process and the final decision.
The author(s) declare that Gen AI was used in the creation of this manuscript. During the preparation of this work the author(s) used ChatGPT 4.0 to reduce spelling and grammar errors. After using this tool/service, the author(s) reviewed and edited the content as needed and take(s) full responsibility for the content of the publication.
All claims expressed in this article are solely those of the authors and do not necessarily represent those of their affiliated organizations, or those of the publisher, the editors and the reviewers. Any product that may be evaluated in this article, or claim that may be made by its manufacturer, is not guaranteed or endorsed by the publisher.
Ali, A., Kemter, E., and Wolf, E. (2024a). Advances in organ and tissue xenotransplantation. Ann. Rev. Animal Biosci. 12, 369–390. doi: 10.1146/annurev-animal-021122-102606
Ali, M., and Corridon, P. R. (2024). Integrated environmental and health economic assessments of novel xeno-keratografts addressing a growing public health crisis. Sci. Rep. 14:25600. doi: 10.1038/s41598-024-77783-y
Ali, Z. M., Wang, X., Shibru, M. G., Alhosani, M., Alfadhli, N., Alnuaimi, A., et al. (2024b). A sustainable approach to derive sheep corneal scaffolds from stored slaughterhouse waste. Regen Med. 19, 303–315. doi: 10.1080/17460751.2024.2357499
Ashokkumar, V., Flora, G., Venkatkarthick, R., SenthilKannan, K., Kuppam, C., Mary Stephy, G., et al. (2022). Advanced technologies on the sustainable approaches for conversion of organic waste to valuable bioproducts: Emerging circular bioeconomy perspective. Fuel. 324:124313. doi: 10.1016/j.fuel.2022.124313
Baghbaderani, B. A., Syama, A., Sivapatham, R., Pei, Y., Mukherjee, O., Fellner, T., et al. (2016). Detailed characterization of human induced pluripotent stem cells manufactured for therapeutic applications. Stem Cell Rev. Rep. 12, 394–420. doi: 10.1007/s12015-016-9662-8
Bento, G., Shafigullina, A. K., Rizvanov, A. A., Sardão, V. A., Macedo, M. P., Oliveira, P. J., et al. (2020). Urine-derived stem cells: applications in regenerative and predictive medicine. Cells. 9:573. doi: 10.3390/cells9030573
Brown, B. N., Barnes, C. A., Kasick, R. T., Michel, R., Gilbert, T. W., Beer-Stolz, D., et al. (2010). Surface characterization of extracellular matrix scaffolds. Biomaterials. 31, 428–37. doi: 10.1016/j.biomaterials.2009.09.061
Corridon, P., Ascázubi, R., Krest, C., and Wilke, I. (2006). “Time-domain terahertz spectroscopy of artificial skin,” in Proceedings of SPIE - The International Society for Optical Engineering, Vol. 6080 (San Jose, CA: SPIE).
Corridon, P. R. (2023). Enhancing the expression of a key mitochondrial enzyme at the inception of ischemia-reperfusion injury can boost recovery and halt the progression of acute kidney injury. Front. Physiol. 14:1024238. doi: 10.3389/fphys.2023.1024238
Corridon, P. R., Rhodes, G. J., Leonard, E. C., Basile, D. P., and Gattone, V. H. (2013). 2nd, Bacallao RL, et al. A method to facilitate and monitor expression of exogenous genes in the rat kidney using plasmid and viral vectors. Am. J. Physiol. Renal Physiol. 304, F1217–F1229. doi: 10.1152/ajprenal.00070.2013
Dai, W., Gong, X., Wang, C., Liu, P., Shi, W., Cheng, J., et al. (2024). Injectable decellularized extracellular matrix hydrogel with cell-adaptable supramolecular network enhances cartilage regeneration by regulating inflammation and facilitating chondrogenesis. Chem. Eng. J. 498:155138. doi: 10.1016/j.cej.2024.155138
Dong, M., Zhao, L., Wang, F., Hu, X., Li, H., Liu, T., et al. (2019). Rapid porcine corneal decellularization through the use of sodium N-lauroyl glutamate and supernuclease. J. Tissue Eng. 10:2041731419875876. doi: 10.1177/2041731419875876
El Zarif, M., Del Barrio, J. A., Arnalich-Montiel, F., De Miguel, M. P., Makdissy, N., and Alió, J. L. (2020). Corneal stroma regeneration: new approach for the treatment of cornea disease. Asia Pac. J. Ophthalmol. 9, 571–579 doi: 10.1097/APO.0000000000000337
Espana, E. M., and Birk, D. E. (2020). Composition, structure and function of the corneal stroma. Exp. Eye Res. 198:108137. doi: 10.1016/j.exer.2020.108137
Eyre, K., Samper, E., Haverich, A., Hilfiker, A., and Andrée, B. (2021). Re-endothelialization of non-detergent decellularized porcine vessels. Artif. Organs. 45, E53–E64. doi: 10.1111/aor.13836
Guindolet, D., Crouzet, E., He, Z., Herbepin, P., Perrache, C., Garcin, T., et al. (2021). Epithelial regeneration in human corneas preserved in an active storage machine. Transl. Vis. Sci. Technol. 10:31. doi: 10.1167/tvst.10.2.31
Hamedi, E., Boroumand, S., Sigaroodi, F., Rahmani, M., Hosseinzadeh, S., Khani, M-. M., et al. (2024). Incorporation of GelMA/PEGDA into the decellularized cornea as a potential hybrid scaffold for in situ repairing of deep anterior corneal defects. J. Polym. Environ. 32, 4763–4776. doi: 10.1007/s10924-024-03284-8
Harris, G. M., Raitman, I., and Schwarzbauer, J. E. (2018). Cell-derived decellularized extracellular matrices. Methods Cell. Biol. 143, 97–114. doi: 10.1016/bs.mcb.2017.08.007
Hosseinikhah, S. M., Vahdat-Lasemi, F., Farhoudi, L., Gupta, G., Kesharwani, P., Sahebkar, A., et al. (2024). RGD-decorated nanoparticles: therapeutic potential beyond cancer. J. Drug Deliv. Sci. Technol. 98:105924. doi: 10.1016/j.jddst.2024.105924
Jeznach, O., Tabakoglu, S., Zaszczyńska, A., and Sajkiewicz, P. (2024). Review on machine learning application in tissue engineering: What has been done so far? Application areas, challenges, and perspectives. J. Mat. Sci. 59, 21222–21250. doi: 10.1007/s10853-024-10449-2
Jia, S., Bu, Y., Lau, D-. S. A., Lin, Z., Sun, T., Lu, W. W., et al. (2023). Advances in 3D bioprinting technology for functional corneal reconstruction and regeneration. Front. Bioeng. Biotechnol. 10:1065460. doi: 10.3389/fbioe.2022.1065460
Jing, Y., Zhou, Y., Wang, C., Liu, J., Guo, Y., Mao, S., et al. (2019). Establishment of a non-integrate iPS cell line CSUASOi002-A, from urine-derived cells of a female patient with macular corneal dystrophy carrying compound heterozygous CHST6 mutations. Stem Cell Res. 41:101598. doi: 10.1016/j.scr.2019.101598
Khan, R. L., Khraibi, A. A., Dumee, L. F., and Corridon, P. R. (2023). From waste to wealth: repurposing slaughterhouse waste for xenotransplantation. Front. Bioeng. Biotechnol. 11:1091554. doi: 10.3389/fbioe.2023.1091554
Kim, K., Gil, M., Dayem, A. A., Choi, S., Kang, G. H., Yang, G. M., et al. (2020). Improved Isolation and Culture of Urine-Derived Stem Cells (USCs) and Enhanced Production of Immune Cells from the USC-Derived Induced Pluripotent Stem Cells. J. Clin. Med. 9:827 doi: 10.3390/jcm9030827
Limeneh, D. Y., Tesfaye, T., Ayele, M., Husien, N. M., Ferede, E., Haile, A., et al. (2022). A comprehensive review on utilization of slaughterhouse by-product: current status and prospect. Sustainability. 14:6469. doi: 10.3390/su14116469
Liu, G., Wu, R., Yang, B., Deng, C., Lu, X., Walker, S. J., et al. (2018). Human Urine-Derived Stem Cell Differentiation to Endothelial Cells with Barrier Function and Nitric Oxide Production. Stem Cells Transl Med. 7, 686–98. doi: 10.1002/sctm.18-0040
Lv, C., Huang, Y., Yan, R., and Gao, Y. (2023). Vascular endothelial growth factor induces the migration of human airway smooth muscle cells by activating the RhoA/ROCK pathway. BMC Pulm. Med. 23:505. doi: 10.1186/s12890-023-02803-y
Lynch, A. P., Wilson, S. L., and Ahearne, M. (2016). Dextran preserves native corneal structure during decellularization. Tissue Eng. Part C Methods. 22, 561–572. doi: 10.1089/ten.tec.2016.0017
Naba, A., Pearce, O. M. T., Del Rosario, A., Ma, D., Ding, H., Rajeeve, V., et al. (2017). Characterization of the extracellular matrix of normal and diseased tissues using proteomics. J. Proteome. Res. 16, 3083–3091. doi: 10.1021/acs.jproteome.7b00191
Nara, S., Chameettachal, S., Midha, S., Murab, S., and Ghosh, S. (2016). Preservation of biomacromolecular composition and ultrastructure of a decellularized cornea using a perfusion bioreactor. RSC Adv. 6, 2225–2240. doi: 10.1039/C5RA20745B
Ott, H. C., Matthiesen, T. S., Goh, S. K., Black, L. D., Kren, S. M., Netoff, T. I., et al. (2008). Perfusion-decellularized matrix: using nature's platform to engineer a bioartificial heart. Nat. Med. 14, 213–221. doi: 10.1038/nm1684
Pantic, I. V., Cumic, J., Valjarevic, S., Shakeel, A., Wang, X., Vurivi, H., et al. (2023). Computational approaches for evaluating morphological changes in the corneal stroma associated with decellularization. Front. Bioeng. Biotechnol. 11:1105377. doi: 10.3389/fbioe.2023.1105377
Patricelli, C., Lehmann, P., Oxford, J. T., and Pu, X. (2023). Doxorubicin-induced modulation of TGF-β signaling cascade in mouse fibroblasts: insights into cardiotoxicity mechanisms. Sci. Rep. 13:18944. doi: 10.1038/s41598-023-46216-7
Pavathuparambil Abdul Manaph, N., Al-Hawwas, M., Bobrovskaya, L., Coates, P. T., and Zhou, X-. F. (2018). Urine-derived cells for human cell therapy. Stem Cell Res. Ther. 9:189. doi: 10.1186/s13287-018-0932-z
Polisetti, N., Schmid, A., Schlötzer-Schrehardt, U., Maier, P., Lang, S. J., Steinberg, T., et al. (2021). A decellularized human corneal scaffold for anterior corneal surface reconstruction. Sci Rep. 11:2992. doi: 10.1038/s41598-021-82678-3
Romano, V., Passaro, M. L., Ruzza, A., Parekh, M., Airaldi, M., Levis, H. J., et al. (2024). Quality assurance in corneal transplants: donor cornea assessment and oversight. Surv. Ophthalmol. 69, 465–482. doi: 10.1016/j.survophthal.2023.12.002
Sato, M., Takizawa, H., Nakamura, A., Turner, B. J., Shabanpoor, F., Aoki, Y., et al. (2019). Application of urine-derived stem cells to cellular modeling in neuromuscular and neurodegenerative diseases. Front. Mol. Neurosci. 12:297. doi: 10.3389/fnmol.2019.00297
Shibru, M. G., Ali, Z. M., Almansoori, A. S., Paunovic, J., Pantic, I. V., Corridon, P. R., et al. (2024). Slaughterhouse waste: a unique and sustainable source for dECM-based bioinks. Regen Med. 19, 113–118 doi: 10.2217/rme-2023-0194
Sotozono, C., Inatomi, T., Nakamura, M., and Kinoshita, S. (1995). Keratinocyte growth factor accelerates corneal epithelial wound healing in vivo. Invest. Ophthalmol. Vis. Sci. 36, 1524–1529.
Spang, M. T., and Christman, K. L. (2018). Extracellular matrix hydrogel therapies: in vivo applications and development. Acta Biomater. 68, 1–14. doi: 10.1016/j.actbio.2017.12.019
Sridhar, M. S. (2018). Anatomy of cornea and ocular surface. Indian J Ophthalmol. 66, 190–194. doi: 10.4103/ijo.IJO_646_17
Suda, T., Yokoo, T., Kanefuji, T., Kamimura, K., Zhang, G., Liu, D., et al. (2023). Hydrodynamic delivery: characteristics, applications, and technological advances. Pharmaceutics. 15:1111 doi: 10.3390/pharmaceutics15041111
Sullivan, P. S., Chan, J. B., Levin, M. R., and Rao, J. (2010). Urine cytology and adjunct markers for detection and surveillance of bladder cancer. Am. J. Transl. Res. 2, 412–440.
Sun, Y., Zhao, H., Yang, S., Wang, G., Zhu, L., Sun, C., et al. (2024). Urine-derived stem cells: promising advancements and applications in regenerative medicine and beyond. Heliyon. 10:e27306.doi: 10.1016/j.heliyon.2024.e27306
Tarafdar, A., Gaur, V. K., Rawat, N., Wankhade, P. R., Gaur, G. K., Awasthi, M. K., et al. (2021). Advances in biomaterial production from animal derived waste. Bioengineered. 12, 8247–8258. doi: 10.1080/21655979.2021.1982321
Taylor, D. A., Sampaio, L. C., Ferdous, Z., Gobin, A. S., and Taite, L. J. (2018). Decellularized matrices in regenerative medicine. Acta Biomaterialia. 74, 74–89. doi: 10.1016/j.actbio.2018.04.044
Wang, X., Elbahrawi, R. T., Abdukadir, A. M., Ali, Z. M., Chan, V., Corridon, P. R. A., et al. (2023a). proposed model of xeno-keratoplasty using 3D printing and decellularization. Front. Pharmacol. 14:1193606. doi: 10.3389/fphar.2023.1193606
Wang, X., Shakeel, A., Salih, A. E., Vurivi, H., Daoud, S., Desidery, L., et al. (2023b). A scalable corneal xenograft platform: simultaneous opportunities for tissue engineering and circular economic sustainability by repurposing slaughterhouse waste. Front. Bioeng. Biotechnol. 11:1133122. doi: 10.3389/fbioe.2023.1133122
Wimmer, R., Cseh, B., Maier, B., Scherrer, K., and Baccarini, M. (2012). Angiogenic sprouting requires the fine tuning of endothelial cell cohesion by the Raf-1/Rok-α complex. Dev. Cell. 22, 158–171. doi: 10.1016/j.devcel.2011.11.012
Yin, X., Li, Q., McNutt, P. M., and Zhang, Y. (2022). Urine-derived stem cells for epithelial tissues reconstruction and wound healing. Pharmaceutics. 14:1669. doi: 10.3390/pharmaceutics14081669
Yin, X., Li, Q., Shu, Y., Wang, H., Thomas, B., Maxwell, J. T., et al. (2024). Exploiting urine-derived induced pluripotent stem cells for advancing precision medicine in cell therapy, disease modeling, and drug testing. J Biomed Sci. 31, 47. doi: 10.1186/s12929-024-01035-4
Yu, P., Bosholm, C. C., Zhu, H., Duan, Z., Atala, A., Zhang, Y., et al. (2024). Beyond waste: understanding urineand#x2019; s potential in precision medicine. Trends Biotechnol. 42, 953–969. doi: 10.1016/j.tibtech.2024.01.009
Yu, P., Opara, E. C., and Zhang, Y. (2023). Power of pee: Urine-derived stem cells in urological disorders. UroPrecision. 1, 38–44. doi: 10.1002/uro2.16
Yue, B. (2014). Biology of the extracellular matrix: an overview. J Glaucoma. 23, S20–3. doi: 10.1097/IJG.0000000000000108
Zeng, J., Huang, L., Xiong, H., Li, Q., Wu, C., Huang, Y., et al. (2022). Injectable decellularized cartilage matrix hydrogel encapsulating urine-derived stem cells for immunomodulatory and cartilage defect regeneration. NPJ Regener. Med. 7:75. doi: 10.1038/s41536-022-00269-w
Zhang, D., Wei, G., Li, P., Zhou, X., and Zhang, Y. (2014). Urine-derived stem cells: a novel and versatile progenitor source for cell-based therapy and regenerative medicine. Genes. Dis. 1, 8–17. doi: 10.1016/j.gendis.2014.07.001
Zhang, M., Yang, F., Han, D., Zhang, S. Y., Dong, Y., Li, X., et al. (2023). 3D bioprinting of corneal decellularized extracellular matrix: GelMA composite hydrogel for corneal stroma engineering. Int. J. Bioprint. 9:774. doi: 10.18063/ijb.774
Zheng, T., Le, Q., Hong, J., and Xu, J. (2016). Comparison of human corneal cell density by age and corneal location: an in vivo confocal microscopy study. BMC Ophthalmol. 16:109. doi: 10.1186/s12886-016-0290-5
Keywords: sustainable tissue engineering, agri-food waste, urine-derived stem cells (USCs), keratoplasty, decellularized extracellular matrix (dECM)
Citation: Corridon PR, Mobin A, Hashem Z, Paunovic J, Valjarevic S and Pantic IV (2025) Sustainable keratoplasty models using agri-food waste: a hypothesis for transforming biowaste into biomaterials for tissue engineering research. Front. Sustain. Food Syst. 9:1564425. doi: 10.3389/fsufs.2025.1564425
Received: 21 January 2025; Accepted: 10 March 2025;
Published: 26 March 2025.
Edited by:
Gheorghe Adrian Martau, University of Agricultural Sciences and Veterinary Medicine of Cluj-Napoca, RomaniaReviewed by:
Luke Roger Perreault, Boston College, United StatesCopyright © 2025 Corridon, Mobin, Hashem, Paunovic, Valjarevic and Pantic. This is an open-access article distributed under the terms of the Creative Commons Attribution License (CC BY). The use, distribution or reproduction in other forums is permitted, provided the original author(s) and the copyright owner(s) are credited and that the original publication in this journal is cited, in accordance with accepted academic practice. No use, distribution or reproduction is permitted which does not comply with these terms.
*Correspondence: Peter R. Corridon, cGV0ZXIuY29ycmlkb25Aa3UuYWMuYWU=
Disclaimer: All claims expressed in this article are solely those of the authors and do not necessarily represent those of their affiliated organizations, or those of the publisher, the editors and the reviewers. Any product that may be evaluated in this article or claim that may be made by its manufacturer is not guaranteed or endorsed by the publisher.
Research integrity at Frontiers
Learn more about the work of our research integrity team to safeguard the quality of each article we publish.