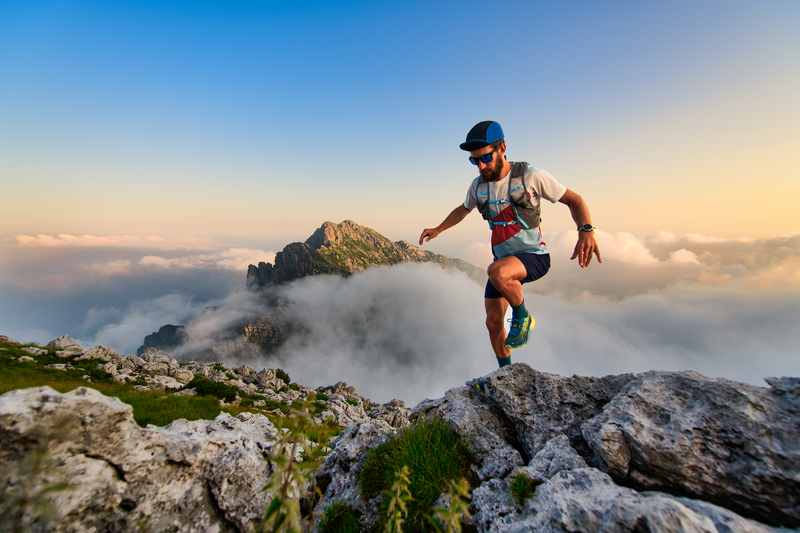
95% of researchers rate our articles as excellent or good
Learn more about the work of our research integrity team to safeguard the quality of each article we publish.
Find out more
REVIEW article
Front. Sustain. Food Syst. , 26 March 2025
Sec. Crop Biology and Sustainability
Volume 9 - 2025 | https://doi.org/10.3389/fsufs.2025.1549048
This article is part of the Research Topic Innovative Solutions For Next-Generation Fertilizers View all 13 articles
The increase in demand for food production due to the ever-increasing human population across the world requires that food production should grow exponentially. For agricultural food production to meet the needs of human requirements and demands there is a need for sustainable practices that will ensure production and availability of food without affecting soil health, soil biota and soil fertility. Over the years, many plant growth promoting bacteria (PGPB) strains have been identified and reported to provide a number of benefits to plants, including enhanced nutrient uptake, growth, and development as well as increased resistance to biotic and abiotic stress. However only a small number of them, are sold today, mostly due to the formulations’ inability to support bacterial survival both during and after application in agroecosystems. PGPB strains that present these difficult constraints can be employed in the production of cell-free supernatants (CFSs), which are broth cultures that have undergone various mechanical and physical procedures to eliminate cells. The available literature suggests that CFS may be a reliable source of secondary metabolites for sustainable agriculture. This review therefore discusses cell free supernatant of various soil microorganisms that have been used in crop production and offered pertinent information about CFS for upcoming studies on CFSs as bio stimulant and biocontrol agents in sustainable agriculture. The significance, sources, applications, mechanisms of action of CFS and benefits of studies on CFS agricultural applications—both as a bio fertilizer and a biocontrol agent were studied.
Conventional agriculture employed to increase agricultural production and meet the food demand of the ever-increasing human population heavily relies on excessive use of agrochemicals such as fertilizers, herbicides and pesticides (Li et al., 2022). However, this agricultural system, although increasing production, has proven unsustainable in the long run. Further nutrient input for instance, can result in a decline in soil fertility (Kopittke et al., 2019). The excessive and inappropriate use of agrochemicals pollute soil, air and water through nutrient leaching, causing a chemical imbalance across multiple ecosystems (Chandini et al., 2019). These chemical fertilizers and pesticides can also lead to soil acidification, greenhouse gas emissions, negative impacts on human health and harm to non-target organisms resulting in a loss of biodiversity (Li et al., 2020). Chemical fertilizers have considerable deleterious effects on soil, plants, humans and environmental sustainability increasing the costs and reducing profitability (Alori et al., 2019).
Therefore, there is a need for a more eco-friendly and sustainable methods that may be practiced without health hazards. The use of microbial inoculants holds great promise to improve crop yield without negative environmental and health risks (Alori et al., 2019). However, under harsh environmental condition, the impact of microbial inoculant diminishes (Naamala et al., 2023).
Cell-free supernatant is a liquid fraction obtained from microorganism cultures after centrifugation. It contains various plant growth-promoting compounds, enzymes and secondary metabolites such as antibiotics (Naamala et al., 2023). Microbial cell-free supernatant (CFS) has been reported to be associated with the strong proliferation of resident beneficial soil microbes that activate beneficial soil microorganisms; therefore, it is a safe, efficient and environmentally friendly approach to minimize shortfalls related to the technology of microbial inoculation (Morcillo et al., 2020).
The use of cell-free supernatant in sustainable crop production has garnered attention for its potential to promote plant growth, increase yield, and reduce the negative impact of chemical fertilizers and pesticides on the environment. The compounds are less likely to experience diminished effects under harsh environmental conditions. Additionally, they are generally required in low concentrations and are easier to store compared to live microbial cells (Naamala et al., 2022).
Cell-free supernatants can be extracted via several methods depending on the purpose of use of the supernatant. These methods include centrifugation and filtration that are appropriate for general applications (Subramanian et al., 2021; Zhou et al., 2022), solvent extraction and precipitation are useful for isolating specific metabolites (Gudiña et al., 2015; Santoyo et al., 2021), and Lyophilization method which is preferred for long-term storage (Sornsenee et al., 2021).
Extracted CFS has been stored by several researchers using various techniques. The following methods have reported. Refrigeration (4°C) Storage (Koohestani et al., 2018). Freezing (−20°C to −80°C) (Gunal Köroglu et al., 2024). Lyophilization (Freeze-Drying) Storage Technique: Šuchová et al. (2022) Spray Drying (Gullifa et al., 2023). Storage in Preservative Solutions (Hamad et al., 2022). Encapsulation Technique (Bassani et al., 2019).
Several researchers have reported the use of CFS in medicine and food. For example, Arrioja-Bretón et al. (2020), investigated the antimicrobial activity and storage stability of CFS from lactic acid bacteria and its applications with fresh beef. Mani-López et al. (2022) studied CFS production, composition, and the antimicrobial activity of CFS from LAB in vitro, on foods, and in active packaging. Mao et al. (2023) explored the impact of CFS of lactic acid bacteria on Staphylococcus aureus biofilm and its metabolites.
In agriculture, Wang et al. (2018) examined the application and mechanism of Bacillus subtilis in biocontrol of plant disease. Khamsuk et al. (2024) investigated the anti-microbial activities of CFS of plant growth promoting bacteria from rhizospheric soil of rice plant. Li et al. (2023), studied if the application of Bacillus subtilis promotes growth and quality of cucumber. Pellegrini et al. (2020) reviewed the application of CFS from plant growth-promoting bacteria in sustainable agriculture.
However, there is limited information on the comprehensive application of microbial CFS (from both bacteria and fungi) in agriculture and environmental remediation. This research aims to discuss the application of microbial CFS to various soil microorganisms for crop growth and yield improvement, crop protection, and crop response to abiotic stressors including the remediation of polluted soils. It emphasizes the significance of CFS in sustainable crop production. Sources of CFS, mechanisms of action, challenges and limitations were thoroughly explained.
Cell-Free Supernatants are significantly important in sustainable crop production as follows:
Cell-free supernatant contains various secondary metabolites, including organic acids, enzymes, and siderophores, which can enhance soil health. These compounds can stimulate the growth of beneficial microorganisms such as mycorrhizal fungi, rhizobia, and other nitrogen-fixing bacteria, ultimately improving soil fertility (Pellegrini et al., 2020). Microbial CFS supports the proliferation of resident beneficial soil microbes (Morcillo et al., 2022).
Cell-free supernatant contains various plant growth-promoting compounds, including indole-3-acetic acid (IAA), gibberellins, cytokinins, and auxins. These compounds can enhance plant growth by promoting cell division, elongation, and differentiation (Naamala et al., 2023). Additionally, cell-free supernatant can improve nutrient uptake by plants by increasing the solubility and availability of essential minerals such as nitrogen, phosphorus, and potassium (Islam et al., 2016). Bacillus subtilis and Bacillus licheniformis can enhance plant growth and biomass production through the synthesis of various phytohormones, nitrogen fixation, phosphate solubilization, and ammonium ion production (Khan et al., 2018). Moreover, microbial CFS can suppress the growth of plant pathogens, thereby reducing the need for chemical pesticides (Islam et al., 2016). Plants treated with CFS exhibited a higher fruit yield than control plants (Imran et al., 2023).
Cell free supernatants as shown in Figure 1 are obtained from bacteria, fungi, plant tissue, and animal tissue cultures. Bacterial cultures are the most common source of cell-free supernatant. Bacteria produce various secondary metabolites such as antibiotics, enzymes, and plant growth-promoting compounds, which are present in the cell-free supernatant (Kumar et al., 2020).
Fungal cultures are another source of cell-free supernatants. Fungi produce various secondary metabolites such as mycotoxins, antibiotics, and enzymes, which are present in the cell-free supernatant (Dozolme and Moukha, 2020; Nasrollahzadeh et al., 2022). These compounds are secreted by these microorganisms into the surrounding environment. Plant tissue cultures are also a potential source of cell-free supernatants. Plant cells produce various secondary metabolites such as phenolics, flavonoids, and alkaloids, which are present in the cell-free supernatant (Yadav and Yadav, 2023). These compounds are secreted by plant cells into the surrounding environment to defend against herbivores or pathogens.
Animal cell cultures are another potential source of cell-free supernatant. Animal cells produce various secondary metabolites such as cytokines, growth factors, and antibodies, which can be found in the cell-free supernatant (Feder-Mengus et al., 2008; Bourebaba et al., 2022). Figure 1 illustrates the various sources of cell free supernatant for agricultural purposes.
To ensure only extracellular metabolites are obtained without microbial cell contamination, the extraction method of culturing the desired microorganism in the appropriate growth medium is critical. The type of bioactive compounds, desired purity, and intended application of CFS determine the choice of extraction method.
The first step is to culture the desired microorganism in the appropriate growth medium. The cells in culture can then be removed or separated using various methods.
For general applications, centrifugation and filtration are sufficient, while solvent extraction and precipitation are useful for isolating specific metabolites. For long-term storage, Lyophilization will be more appropriate.
i. Centrifugation: The culture is centrifuged at 5,000–10,000 rpm for 10–20 min to pellet microbial cells (Majeedras et al., 2018). Differences in density allow centrifugation to separate cells from the liquid culture. The supernatant is then carefully collected without disturbing the pellet (Subramanian et al., 2021). This method is simple, cost-effective and fast. However, some microbial cells may remain in the supernatant, requiring additional filtration.
ii. Filtration: This process involves using a membrane filter with an appropriate pore size to eliminate microbial cells and debris. Following centrifugation, the supernatant is filtered through 0.22 μm or 0.45 μm membrane filters to eliminate any remaining cells (Zhou et al., 2022). Sterile filtration guarantees the absence of live microorganisms, ensuring complete removal of microbial cells. This method is ideal for heat-sensitive compounds (Rashid et al., 2021). However some bioactive molecules may adhere to the filter membrane, and highly viscous cultures can lead to filter clogging.
iii. Dialysis and ultrafiltration: This method allow for the removal of small molecules or concentrated-bioactive compounds using semipermeable membranes. The CFS is placed in a dialysis bag with a molecular weight cutoff (MWCO) to eliminate salts and low-molecular-weight impurities. Ultrafiltration membranes, with MWCOs (such as 3 kDa, 10 kDa, or 30 kDa) can selectively concentrate larger bioactive molecules (Khan et al., 2020). This process effectively eliminates unwanted small molecules while retaining bioactive compounds, and ultrafiltration enables the fractionation of compounds based on molecular weight. However this technique can be time-consuming and may result in the loss of some metabolites during dialysis.
iv. Solvent extraction: This method involves using organic solvents to extract bioactive compounds based on polarity differences. The CFS is combined with an organic solvent like ethyl acetate, methanol, or chloroform at a 1:1 ratio. The mixture is then shaken and allowed to separate into two phases. The organic phase, which contains bioactive metabolites, is collected and evaporated under reduced pressure (Singh et al., 2016). The technique is effective for extracting non-polar bioactive compounds such as antibiotics and secondary metabolites. It can be utilized for selective extraction of various classes of metabolites. However, it’s important to note that organic solvents may degrade heat-sensitive compounds, and some solvents can be toxic and should be handled with caution.
v. Precipitation (ammonium sulfate or ethanol precipitation): In this method, salts or alcohol are used to precipitate proteinaceous bioactive molecules by altering their solubility. Ammonium sulfate is added to the CFS to achieve 40–80% saturation, leading to protein precipitation (Gudiña et al., 2015). The precipitate is collected by centrifugation and dissolved in buffer for further analysis. Ethanol or acetone can also be used to precipitate extracellular proteins and peptides (Santoyo et al., 2021). This method is suitable for purifying enzymes and antimicrobial peptides as well as concentrating bioactive molecules. However, it requires optimization of precipitation conditions and some bioactive molecules may be lost in the process.
vi. Lyophilization (freeze-drying) is a process where water is removed from supernatants through sublimation under vacuum while preserving bioactive compounds. The CFS is frozen at −80°C or in liquid nitrogen before being subjected to a vacuum in a lyophilizer to remove ice through sublimation. The resulting dry powder can be stored for future use, maintaining bioactivity for long-term storage and being suitable for thermostable compounds. However, this method can be expensive and time-consuming, and there is a risk of losing some volatile compounds.
Cell-free supernatant has various potential applications in agriculture, and environmental remediation. In agriculture, it can be used as a natural substitute for chemical fertilizers and pesticides, because it contains plant growth-promoting compounds and can suppress the spread of plant diseases (Kaewchomphunuch et al., 2022).
Several microbial CFS have been reported to enhance plant growth. This technology is desirable amidst the threat of climate change because it is sustainable and environmentally friendly. CFS obtained from Lactobacillus helveticus EL2006H enhanced the growth of corn, soybean and potato (Naamala et al., 2023). Azospirillum brasilense CFS increased the number of root branches and nodules in soybean (Rondina et al., 2020). According to Posada et al. (2016), CFS of Bacillus subtilis EA-CB0575 significantly increased the dry weight of banana plants. The inclusion of CFS from Bradyrhizobium diazoefficiens strain USDA 110 and Rhizobium tropici strain CIAT 889 increased root diameter by up to 1.6%, root length by 28.5%, root volume by 19.7%, root surface area by 17.8%, number of nodules by 29%, nodule dry weight by 27.2%, root dry weight by 13.5%, and shoot dry weight by 3.8% resulting in a yield increase of by 485 kg ha−1 (Moretti et al., 2020). The soil biostimulant products CFS obtained from Lactobacillus rhamnosus are made up of lactic acid, peptides, and free amino acids modified by microbial biodiversity, favoring bacterial genera recognized as growth plant promoters (Caballero et al., 2020). CFSs of Bacillus sp. strains (U35, U47, U48, U49, and U50) increased all the growth traits of corn (Yaghoubian et al., 2022).
CFS containing lipopeptides from Bacillus subtilis have shown potent inhibitory activity against plant pathogenic fungus Fusarium oxysporum even at very low concentration. They inhibited spore germination by up to 26% and mycelium growth by up to 49% compared to the control (Mihalache et al., 2018). In environmental remediation, cell-free supernatant can be used to degrade pollutants or promote the growth of beneficial microorganisms (Xu et al., 2021). Soil biostimulant products such as (CFS obtained from Lactobacillus rhamnosus) consist of lactic acid, peptides, free amino acids, and protein hydrolysates, exhibiting biocontrol activity against some phytopathogenic microorganisms (Caballero et al., 2020). When applied to potato tubers, the filtrated supernatant of Streptomyces TN258 24 h before significantly decreased pathogen penetration (Pythium ultimum) by 62% and reduced the percentage of weight loss by 59.43% (Sellem et al., 2017). CFS from Bacillus subtilis GLB191 containing cyclic lipopeptides fengycin and surfactin is highly active against downy mildew in grapevines through the induction of defense gene expression and callose production (Li et al., 2019).
Globally, abiotic stresses such as drought, salinity, extreme pH, and heavy metals pose a significant threat to crop productivity. Improving crop tolerance to abiotic stress with microbial CFS is a sustainable strategy to meet the increasing demand for food. Cell-free supernatant may also mitigate abiotic stressors including heavy metal toxicity, salinity, and drought (Ibitoye and Kolawole, 2022). This can occur through various mechanisms such as osmotic adjustment, ion homeostasis, and antioxidant defense. For example, some bacteria can produce exopolysaccharides that protect plants from drought stress by increasing water retention in soil and improving soil structure. CFS produced from Acinetobacter calcoaceticus AC06 and Bacillus amyloliquefaciens BA01 contains biostimulants that induce osmotic tolerance and metabolic changes in groundnuts under drought stress (Eswaran et al., 2024).
CFS can also be used for bioremediation, which is the process of using microorganisms to degrade or remove environmental contaminants (Pacwa-Płociniczak et al., 2011). Many bacteria in the rhizosphere have the ability to degrade pollutants, such as heavy metals, organic compounds, and pesticides (Alori and Fawole, 2017). These bacteria can be cultured to produce CFS containing enzymes and other molecules that can degrade pollutants. Some examples of bacteria associated with the remediation of environmental pollutants include Pseudomonas fluorescens F113, P. cepacia ATCC 29351 (Alori and Babalola, 2018), and Rhodopseudomonas palustris (Sravya and Sangeetha, 2022). Additionally, CFS also stimulate the growth of indigenous microorganisms, enhancing the natural attenuation of contaminants. Table 1 shows some microorganisms whose CFS has been used to remediate polluted soil. The CFS of these soil microorganisms consists of various types of biosurfactants, glycolipids, exopolymeric substances, and siderophores, which have the potential to reduce and remove soil pollutants ranging from hydrocarbons to heavy metals. The percentage of pollutants removed ranged from 24 to 93%.
Cell-free supernatant exerts various effects including pest and disease control, plant-growth-promoting, bioremediation of polluted soil and defense against abiotic stressors (such as acidity, and salinity) through various mechanisms. Detailed examples of these mechanisms are provided below.
Cell-free supernatant contains various antibiotics that can inhibit the growth of bacterial and fungal pathogens. These antibiotics can act by inhibiting protein synthesis, cell wall synthesis, DNA replication, or cell membrane integrity of the target microorganism (Borges et al., 2021). The mechanism of action depends on the specific antibiotic present in the cell-free supernatant. The growth inhibition of Rhizopus oryzae by CFS from Trichoderma spp. could be due to the secretion of harmful extra-cellular compounds like antibiotics such as gliotoxin, and glyoviridin (Alka and Prajapati, 2017).
Many antibiotics exert their antimicrobial activity by inhibiting the synthesis of cell wall components such as peptidoglycan or chitin. This can lead to the weakening or rupture of the cell wall, causing the death of the microorganism (Borges et al., 2021). Additionally, many antibiotics can inhibit the synthesis of bacterial proteins by binding to bacterial ribosomes and preventing the formation of peptide bonds between amino acids. This can lead to the disruption of essential cellular processes such as DNA replication, transcription, and translation, ultimately causing the death of the microorganism (Sarathy et al., 2019). Moreover, cell-free supernatant may also modulate plant-microbe interactions, leading to improved plant health and yield (Santoyo et al., 2021).
Some bacteria can produce siderophores, which bind iron and make it unavailable to pathogenic fungi (Zhang et al., 2023). The antifungal activity of Lactobacillus spp. is associated with the synthesis of organic acids, fatty acids, esters of fatty acids, hydrogen peroxide, bacteriocins, and other secondary metabolites (Perczak et al., 2018). The antifungal activity of CFS containing lipopeptides produced by Bacillus subtilis is related to the inhibition of spore germination and the irreversible damage of the hyphae cell wall of plant pathogenic fungi Fusarium oxysporum (Mihalache et al., 2018). Microbial CFS activates plants and protects them against harmful bacteria and fungi (Pellegrini et al., 2020). The bioactive compounds in CFS inhibit fungal and bacterial pathogens by disrupting cell membranes (Kumar S. et al., 2021). The presence of amino acids, vitamins, and other nutrients in the supernatant can also contribute to its efficacy in disease resistance (Kumar S. et al., 2020).
CFS from Trichoderma simmonsii, Aspergillus westerdijkiae, and Bacillus sp. prevents dieback disease on apple rootstocks both when applied and in combination. This suggest additive or synergistic effects between the two biocontrol agents (M’henni et al., 2022).
Lactobacillus plantarum CFS can prevent spore development of pathogenic fungi Fusarium oxysporum for up to 6 days by producing extracellular hydrolytic enzymes (Di Rico et al., 2025). Some CFS contain chitinases or glucanases, which degrade the cell walls of fungi (Veliz et al., 2017). The growth inhibition of Rhizopus oryzae could be due to the secretion of cell wall-degrading enzymes such as glucanases, endochitinases, and chitinases (Alka and Prajapati, 2017). The production of hydrolytic enzymes (such as chitinase, glucanase, protease, and cellulase; and antibiotics such as 2,4-diacetyl phloroglucinol, amphisin, oomycin A, hydrogen cyanide, phenazine, pyoluteorin, pyrrolnitrin, cyclic lipopeptides, oligomycin A, zwittermicin A, kanosamine, and xanthobaccin) by beneficial microbes is an important mechanism against phytopathogens for sustainable plant disease management. These enzymes break down the cell wall of fungal pathogens, leading to cell death (Jadhav et al., 2017).
Cell-free supernatant contains various compounds that can interfere with quorum sensing (QS), a mechanism of communication among bacteria that regulates gene expression in response to cell population density and virulence. This mechanism is relied upon by many pathogenic bacteria to control virulence, biofilm formation, and antibiotic resistance. These compounds n CFS can either block the synthesis of quorum sensing molecules or inhibit their binding to receptors, thus disrupting the quorum sensing network (Escobar-Muciño et al., 2022). The bioactive compounds in microbial CFS inhibit fungal and bacterial pathogens by interfering with quorum sensing and inducing systemic resistance in plants (Kumar S. et al., 2021). Mechanisms of Quorum Sensing Inhibition by Microbial CFS include the following:
a. Enzymatic degradation of QS signals
Enzymes such as lactonases, acylases, and oxidoreductases found in microbial CFS produced by certain microbes can breakdown or alter quorum sensing signals (autoinducers). For example, AHL-lactonases produced by Bacillus and Pseudomonas species can breakdown acyl-homoserine lactones (AHLs), which are important QS signals in Gram-negative bacteria (Uroz et al., 2009). Some Pseudomonas species can also produce enzymes that can breakdown N-acyl homoserine lactones (AHLs), a QS signaling molecule, effectively disrupting QS communication, and thereby reducing the virulence of plant pathogens (Dong et al., 2000).
b. Disruption of biofilm formation
Some microbial CFS disrupts biofilms by interfering with QS pathways. Biofilms are structured bacterial communities that contribute to antimicrobial resistance. For example CFS from Bacillus subtilis, produces surfactin, this inhibits Pseudomonas aeruginosa biofilm formation. This alters cell adhesion and signaling (Bodini et al., 2007).
c. Competitive binding to QS receptors
Certain metabolites in CFS can prevent native autoinducers from activating virulence pathways mimic QS signals by competitively binding to bacterial QS receptors. For example, phenolic compounds in CFS from Lactobacillus spp. interfere with QS-controlled biofilm formation in Pseudomonas aeruginosa (Rasamiravaka et al., 2015).
d. Downregulation of QS gene expression
CFS from Streptomyces spp. have been reported to down regulate luxI/R genes in Chromobacterium violaceum. This disruption affects QS-regulated pigment production and biofilm formation ultimately reducing virulence and biofilm formation (Lade et al., 2014).
Cell-free supernatant can induce systemic resistance in plants, which is a heightened defense response to subsequent pathogen infections (Sivojiene et al., 2021). This can enhance plant resistance to a wide range of pathogens, leading to improved crop health and yield. It is a mechanism by which plants can boost their resistance to a broad range of plant pathogens by activating plant defense pathways, resulting in the accumulation of phytohormones and other defense-related compounds.
For example, treatment with cell-free supernatant of Trichoderma asperellum SKT-1 induces systemic resistance against cucumber mosaic virus (CMV) by regulating the expression of various pathogen-related genes, enhancing the defense mechanism against CMV infection (Elsharkawy et al., 2013). Cell free supernatant may contain plant signal molecules such as lipo-chitooligosaccharides (LCO), chitooligosaccharides (CO), chitinous compounds, flavonoids, jasmonic acid, linoleic acid, linolenic acid, and karrikins (Bywater Ekegard and Fitzsimmons, 2020).
Certain compounds derived from CFS can trigger induced systemic resistance (ISR) and systemic acquired resistance (SAR) in plants, making them more resilient against biotic and abiotic stresses (Köhl et al., 2019). This preemptive defense mechanism enhances plant immunity, reducing disease incidence and improving overall crop health. ISR requires jasmonic acid (JA), ethylene (ET) signaling pathways, and nonexpressor of pathogenesis-related protein 1 (NPR1) (Pieterse and Van Loon, 2007). The two major branches of the JA signaling pathways are controlled by the transcription factor MYC2 and the ethylene response factor (ERF). The ERF branch of the JA pathway is associated with enhanced resistance to necrotrophic pathogens that is regulated by members of the APETALA2/ethylene response factor (AP2/ERF) family, including the JA-responsive marker gene plant defensin1.2 (PDF1.2) (Lorenzo et al., 2003). JA also mediates resistance against herbivores (HIR). After leaf wounding, an increase in the JA derivative Jasmonoyl-isoleucine (JA-Ile) is perceived by a complex consisting of the protein Coronatine insensitive1 (COI1) and Jasmonate ZIM-domain (JAZ) protein. When the hormone is perceived, JAZ repressors are degraded by the proteasome releasing MYC2 and allowing the activation of JA responses such as the accumulation of vegetative storage protein2 (VSP2) (Pieterse et al., 2014). It has been demonstrated that volatiles derived from the linoleic pathway increase sensitivity to methyl jasmonate and induce several defense-related genes, such as chalcone synthase (CHS), allene oxide synthase (AOS), hydroperoxide lyase (HPL), and lipoxygenase 2 (LOX2) (Hirao et al., 2012).
Table 1 summarizes the application and implication of CFS used as biocontrol agents against pests and diseases. It shows that CFS has been studied on various crops including cereal crops like maize, legumes such as soybeans, Lotus japonicas, and chickpeas; vegetables like pepper, tomato, and potato, tuber crops like cassava, and ornamental crops. CFS has been produced from both bacteria, such as the genus Streptomyces, Bacillus, etc., and fungi, such as the genus Penicillium, Trichoderma, etc. Components of such CFS include harzianic acid, linoleic acid, hydroxymethylfurfural, gliotoxin, glyoviridin, siderophores, lipopeptides, enzymes such as glucanases, endochitinases, chitinases, etc.
Table 2 summarizes the use and application of CFS for plant growth promotion. It shows that CFS as biofertilizers has been studied on cereal crops such as maize, legumes including soybeans, Lotus japonicas, and chickpeas; vegetables such as pepper, tomato, and potato; tuber crops like cassava; and ornamental crops. CFS has been produced from both bacteria such as genus Lactobacillus, Bacillus etc. and fungi such as genus Penicillium, Trichoderma etc. Mechanisms of action of CFS as a biofertilizer are as follows:
Cell-free supernatant contains various plant growth-promoting compounds such as auxins, cytokinins, and gibberellins. These compounds can stimulate plant growth and development by promoting cell division, elongation, and differentiation (Naamala et al., 2023). They can also enhance nutrient uptake, water retention, and stress tolerance of plants, thus improving their productivity and quality (Kumar S. et al., 2021). For example, some bacteria can produce indole acetic acid (IAA), which promotes root growth and lateral root formation. CFS contains phytohormones that can promote the expansion and maturation of plants, including gibberellins, cytokinins, and auxins. Phytohormones regulate numerous processes, such as cell division, elongation, and differentiation, leading to improved shoot and root growth, nutrient uptake, and stress tolerance (El Sabagh et al., 2022).
Cell-free supernatants that promote plant growth may contain biostimulants that enhance metabolic or physiological processes such as respiration, photosynthesis, nucleic acid uptake, ion uptake, and nutrient delivery. Examples of biostimulants found in CFS include humic acids, fulvic acids, myo-inositol, and glycine (Bywater Ekegard and Fitzsimmons, 2020). Additionally, organic acids and siderophores in CFS improve nutrient solubilization and uptake, ultimately enhancing plant nutrition and yield (Patel and Saraf, 2017).
According to Glick (2020), plant growth-promoting substances like auxins, gibberellins, and cytokinin-like compounds, which can stimulate root elongation, seed germination, and overall plant vigor, are often present in microbial CFS. Cell-free supernatant can also enhance nutrient uptake by plants by increasing the solubility and availability of essential minerals such as nitrogen, phosphorus, and potassium (Yuan et al., 2020). The presence of amino acids, vitamins, and other nutrients in the supernatant can also contribute to its efficacy in promoting plant growth (Kumar S. et al., 2020).
CFS can also contain exopolysaccharides (EPS) or biofilms, which can increase soil aggregation and water holding capacity. This can improve soil aeration, water infiltration, and nutrient retention, leading to improved plant growth and yield (Ibitoye and Kolawole, 2022).
Cell-free supernatant contains various enzymes such as lipases, amylases, and proteases. These catalysts can hydrolyze different macromolecules like proteins, lipids, and carbohydrates, releasing nutrients that can be utilized by microorganisms or plants (Cruz-Casas et al., 2021).
Cell-free supernatant may also regulate gene expression in plants, leading to improved plant growth and yield (Kumar S. et al., 2021). For example, some bacteria can produce small RNA molecules that can target specific plant genes and regulate their expression. This can affect various plant processes such as photosynthesis, nutrient uptake, and stress response, leading to improved plant performance.
Table 3 shows some microorganisms that mitigate the effects of abiotic stresses such as drought, salinity, and heavy metals on crops and their components. The active components in these CFS include growth hormones, antioxidants, proteins, amino acids, vitamins, and osmolytes.
Microbial cell-free supernatants (CFS) have emerged as a promising alternative to traditional agricultural inputs due to their bioactive properties and hence exhibit the following advantages:
By acting as biofertilizers and biostimulants, CFS can reduce the reliance on synthetic fertilizers and pesticides. This contributes to sustainable agriculture by minimizing chemical residues in the soil and preventing environmental pollution (Chowdhury et al., 2015). Furthermore, CFS-based treatments are often compatible with organic farming practices. The incorporation of natural resistance inducers in pest management programs of woody crops, alone or in combination with classical methods, could be a reliable method for reducing the amount of chemical residues in the environment. Cell-free supernatant can reduce the use of agrochemicals by promoting natural plant growth and suppressing the growth of plant pathogens (Yuan et al., 2020).
Unlike synthetic agrochemicals that may disrupt soil microbial communities, CFS typically supports beneficial rhizosphere interactions. The absence of live microbial cells reduces competition and interference with native soil microbiota while still promoting beneficial interactions, such as increased phosphate solubilization and nitrogen fixation (Garg et al., 2020). CFS contains antimicrobial compounds such as lipopeptides, enzymes, and volatile organic compounds that effectively suppress plant pathogens without harming beneficial microbes (Olanrewaju et al., 2019). This makes CFS an eco-friendly alternative to synthetic pesticides.
The compounds present in them can promote the growth of beneficial microorganisms such as mycorrhizal fungi, rhizobia, and other nitrogen-fixing bacteria, which can enhance soil fertility (Kumar S. et al., 2020).
Compared to live microbial inoculants, CFS offers a more stable and easily scalable solution for agricultural applications. It eliminates concerns regarding microbial survival, colonization, and environmental adaptability (Patel and Saraf, 2017). Additionally, CFS formulations can be stored and transported more efficiently than live microbial cultures, making them more practical for commercial use.
CFS can be easily applied to plants through foliar sprays or soil drenches, making them convenient for farmers to use.
CFS can be stored and transported more easily than live microbial cultures, making them more practical for large-scale applications.
To maintain the biological activity and stability of the bioactive compounds contained in CFS, proper storage techniques are essential. The choice of storage method depends on the nature of the bioactive compounds, the intended application, and the duration of storage. The following are some storage technique options that can be explored in the storage of CFS.
Refrigeration at 4°C is a commonly used method for short-term storage (up to a few weeks). This method slows down microbial contamination and enzymatic degradation and is hence effective at preserving heat-sensitive compounds. CFS containing organic acids, bacteriocins, and quorum sensing inhibitors can remain stable for 1–2 weeks under refrigeration (Koohestani et al., 2018).
Freezing is a widely used method to store CFS containing proteins, enzymes, and other temperature-sensitive metabolites. Standard freezing −20°C is suitable for antimicrobial peptides, biosurfactants, and quorum sensing inhibitors (Simpson, 2010). However, repeated freeze–thaw cycles can lead to protein degradation. Ultra-low freezing at −80°C preserves heat-sensitive biomolecules like bacteriocins and enzymes without significant loss of activity (Simpson, 2010).
Lyophilization also known as freeze-drying removes water from CFS while preserving bioactive compounds. CFS is frozen at −80°C and then subjected to vacuum sublimation to remove ice without affecting bioactivity (Ge et al., 2024). This process prevents degradation of heat-sensitive compounds and extends their shelf life to months or years at room temperature (Kristensen et al., 2020). Lyophilized powder is stored in airtight vials at room temperature or 4°C. Šuchová et al. (2022) used the lyophilization storage technique for bacteriocins, biosurfactants, plant growth-promoting compounds, and quorum sensing inhibitors. Lyophilized bacteriocins from Lactobacillus spp. retain activity for over 12 months at 4°C (Cheikhyoussef et al., 2009).
This method is suitable and cost-effective for industrial-scale storage. CFS is atomized into fine droplets and rapidly dried using heated air (80–150°C) (Huang et al., 2017). It can also be encapsulated with maltodextrin or gum arabic to enhance stability (Barcelos et al., 2014). It can be used for probiotic metabolites, biosurfactants, and microbial fertilizers (Gullifa et al., 2023). Biosurfactants from Bacillus spp. stored via spray drying remain stable for over a year at room temperature (Marchant and Banat, 2012).
Preservatives such as glycerol (10–30%) will protect proteins and bacteriocins during freezing (Corral et al., 2014). Ethyl alcohol (10–50%) was used for organic solvent-stable metabolites such as quorum sensing inhibitors (Hamad et al., 2022).
To protect CFS bioactive compounds from environmental degradation, they can be microencapsulated with polymers (such as alginate and chitosan) (Bassani et al., 2019). Gunal Köroglu et al. (2024) discussed encapsulation of hydrophobic compounds in yeast cells for use in food industries.
Several limitations hinder the widespread adoption of microbial cell-free supernatants (CFS) in sustainable crop production despite their promising applications.
i. Variability in composition: CFS is a complex mixture of different compounds, and its composition can vary significantly depending on the microbial strain, growth conditions, and extraction methods used. This variability makes it difficult to standardize CFS formulations for large-scale agricultural applications and ensure consistent performance (Compant et al., 2005; Olanrewaju et al., 2019). Standardization and optimization remain critical issues for ensuring reproducibility in field applications.
ii. Inadequate understanding of the mode of action: The incomplete full understanding of the mode of action makes it difficult to optimize CFS formulations for specific applications and to predict their efficacy in different environmental conditions (Santos et al., 2017).
iii. Compatibility with other inputs: The compatibility of CFS with other agricultural inputs, such as fertilizers and pesticides, is not well understood. CFS may enhance the efficacy of other inputs, while in other cases, it may have an antagonistic effect, reducing the efficacy of other inputs (Kudjordjie et al., 2019).
iv. Concentration and dosage: The concentration and dosage of CFS required to achieve optimal effects are not well understood. Some studies have reported that lower concentrations of CFS can be more effective than higher concentrations, while others have reported the opposite (Santos et al., 2017).
v. Shelf-life and storage: The shelf life and storage conditions of CFS can also be a limitation, as the bioactive compounds such as antimicrobial peptides, enzymes, and secondary metabolites in CFS can degrade over time or under inappropriate storage conditions due to factors such as temperature, pH, and UV radiation (Garg et al., 2020). This degradation can reduce the efficacy of CFS and make it difficult to transport and use CFS in remote areas (Borges et al., 2021). Furthermore, compared to chemical agro-inputs, the shelf life of microbial CFS-based formulations is shorter, necessitating frequent application or the use of stabilizing agents (Chowdhury et al., 2015).
vi. Potential phytotoxicity and environmental concerns: Although CFS is generally considered environmentally friendly, the potential environmental impact of widespread CFS application needs to be carefully assessed. Some microbial metabolites in CFS, when applied in high concentrations, may exhibit phytotoxic effects, which could result in growth inhibition or stress responses in certain crops (Kumar S. et al., 2021). Furthermore, the long-term impact of repeated CFS applications on soil microbial communities and ecosystem balance is not fully understood, warranting further investigation.
vii. Economic and scalability challenges: The cost of producing CFS at an industrial scale may not be competitive with conventional fertilizers and pesticides which can be cost-prohibitive for some farmers unless optimized biotechnological approaches are adopted (Patel and Saraf, 2017). Additionally, regulatory hurdles in different countries may delay commercialization and adoption. It is crucial to develop cost-effective production and delivery methods for the widespread adoption of CFS technology.
viii. Limited field efficacy: Although CFS has demonstrated strong efficacy in controlled environments and laboratory settings, its performance in open-field conditions remains unpredictable. Factors such as soil microbiome interactions, climatic variations, and plant genotype can influence the bioactivity of CFS, potentially reducing its effectiveness compared to synthetic agrochemicals (Köhl et al., 2019).
Cell-free supernatant has emerged as a promising tool for sustainable crop production. Sources of microbial CFS used in crop production include bacteria and fungi. Its applications include plant growth promotion, biocontrol of plant diseases, and mitigation of the effects of abiotic stresses, including heavy metal remediation. By harnessing the power of CFS, we can reduce our reliance on synthetic chemicals and promote a more sustainable and environmentally friendly approach to crop production.
EA: Conceptualization, Writing – original draft, Writing – review & editing, Supervision. AO: Writing – original draft. AI: Writing – original draft.
The author(s) declare that no financial support was received for the research and/or publication of this article.
Authors would like to acknowledge Landmark University for providing enabling environment for this research.
The authors declare that the research was conducted in the absence of any commercial or financial relationships that could be construed as a potential conflict of interest.
The authors declare that no Gen AI was used in the creation of this manuscript.
All claims expressed in this article are solely those of the authors and do not necessarily represent those of their affiliated organizations, or those of the publisher, the editors and the reviewers. Any product that may be evaluated in this article, or claim that may be made by its manufacturer, is not guaranteed or endorsed by the publisher.
Abbasi, S., Safaie, N., Sadeghi, A., and Shamsbakhsh, M. (2019). Streptomyces Strains Induce Resistance to Fusarium oxysporum f. sp. lycopersici Race 3 in Tomato Through Different Molecular Mechanisms. Frontiers in microbiology 10:1505. doi: 10.3389/fmicb.2019.01505
Ali, A., Haider, M. S., Ashfaq, M., and Hanif, S. (2014). Effect of culture filtrates of Trichoderma spp. on seed germination and seedling growth in chickpea–an in-vitro study. Pak. J. Phytopathol. 26, 01–05. Available at: https://www.pjp.pakps.com/index.php/PJP/article/view/55
Alka, R. K., and Prajapati, B. K. (2017). Effect of Trichoderma spp. and its culture filtrate antagonists on growth and management of rhizopus rot of tomato fruit in vitro and in vivo. J. Pharmacogn. Phytochem. 6, 394–398. Available at: https://www.phytojournal.com/archives/2017/vol6issue4/PartF/6-3-204-831.pdf
Alori, E. T., and Babalola, O. O. (2018). Microbial inoculants for improving crop quality and human health in Africa. Front. Microbiol. 9:02213. doi: 10.3389/fmicb.2018.02213
Alori, E. T., Babalola, O. O., and Prigent-Combaret, C. (2019). Impacts of microbial inoculants on the growth and yield of maize plant. Open Agricult. J. 13, 1–8. doi: 10.2174/1874331501913010001
Alori, E. T., and Fawole, O. B. (2017). “Microbial inoculants-assisted phytoremediation for sustainable soil management” in Phytoremediation: Management of Environmental Contaminants. eds. A. A. Ansari, S. S. Gill R, G. G. R. Lanza, and L. Newman, vol. 5 (Switzerland: Springer International Publishing), 3–17.
Araújo, L. S. S., Silva, S. Q., and Teixeira, M. C. (2021). Developing a biosurfactant to attenuate arsenic contamination in mining tailings. Heliyon 7:e06093. doi: 10.1016/j.heliyon.2021.e06093
Arrioja-Bretón, D., Mani-López, E., Palou, E., and López-Malo, A. (2020). Antimicrobial activity and storage stability of cell-free supernatants from lactic acid bacteria and their applications with fresh beef. Food Control 115:107286. doi: 10.1016/j.foodcont.2020.107286
Baroja-Fernández, E., Almagro, G., Sánchez-López, Á. M., Bahaji, A., Gámez-Arcas, S., De Diego, N., et al. (2021). Enhanced yield of pepper plants promoted by soil application of volatiles from cell-free fungal culture filtrates is associated with activation of the beneficial soil microbiota. Front. Plant Sci. 12:752653. doi: 10.3389/fpls.2021.752653
Barcelos, G. S., Dias, L. C., Fernandes, P. L., Fernandes Rde, C., Borges, A. C., Kalks, K. H., et al. (2014). Spray drying as a strategy for biosurfactant recovery, concentration and storage. Springerplus. 24, 3–49. doi: 10.1186/2193-1801-3-49
Bassani, J. C., Queiroz Santos, V. A., Barbosa-Dekker, A. M., Dekker, R. F. H., Da-Cunha, M. A. A., and Pereira, E. A. (2019). Microbial cell encapsulation as a strategy for the maintenance of stock cultures. LWT. 102, 411–417. doi: 10.1016/j.lwt.2018.12.058
Biktasheva, L., Gordeev, A., Usova, A., Kirichenko, A., Kuryntseva, P., and Selivanovskaya, S. (2024). Bioremediation of oil-contaminated soils using biosurfactants produced by Bacteria of the genus Nocardiopsis sp. Microbiol. Res. 15, 2575–2592. doi: 10.3390/microbiolres15040171
Bodini, S. F., Manfredini, S., Eppis, F., Valentini, F., Santori, F., and Maestri, A. (2007). Quorum sensing inhibition activity of Bacillus sp. natural isolates and their plant growth-promoting effect. J. Biotechnol. 132, 44–48. doi: 10.1016/j.jbiotec.2007.08.024
Borges, A., Ferreira, C., Saavedra, M. J., and Simões, M. (2021). Antibacterial activity and mechanisms of action of natural compounds isolated from lichens: a review. Molecules 26:347. doi: 10.3390/molecules190914496
Bourebaba, Y., Marycz, K., Mularczyk, M., and Bourebaba, L. (2022). Postbiotics as potential new therapeutic agents for metabolic disorders management. Biomed. Pharmacother. 153:113138. doi: 10.1016/j.biopha.2022.113138
Buensanteai, N., Sompong, M., Thamnu, K., Athinuwat, D., Brauman, A., and Plassard, C. (2013). The plant growth promoting bacterium Bacillus sp. CaSUT007 produces phytohormone and extracellular proteins for enhanced growth of cassava. Afr. J. Microbiol. Res. 7, 4949–4954. doi: 10.5897/AJMR12.1839
Buensanteai, N., Yuen, G. Y., and Prathuangwong, S. (2008). The biocontrol bacterium Bacillus amyloliquefaciens KPS46 produces auxin, surfactin and extracellular proteins for enhanced growth of soybean plant. Thai J. Agricult. Sci. 41, 101–116. Available at: https://www.thaiscience.info/journals/Article/TJAS/10469537.pdf
Bywater Ekegard, M., and Fitzsimmons, A. (2020). Supernatant composition of microbial culture for agricultural use. United States Patent website. Available online at: https://patents.google.com/patent/US10588320B2/en (Accessed February 9, 2025).
Caballero, P., Rodríguez-Morgado, B., Macías, S., Tejada, M., and Parrado, J. (2020). Obtaining plant and soil biostimulants by waste whey fermentation. Waste Biomass Valorizat. 11, 3281–3292. doi: 10.1007/s12649-019-00660-7
Campestre, M. P., Castagno, L. N., Estrella, M. J., and Ruiz, O. A. (2016). Lotus japonicus plants of the Gifu B-129 ecotype subjected to alkaline stress improve their Fe2+ bio-availability through inoculation with Pantoea eucalypti M91. J. Plant Physiol. 192, 47–55. doi: 10.1016/j.jplph.2016.01.001
Chandini, R. K., Kumar, R., and Om, P. (2019). The impact of chemical fertilizers on our environment and ecosystem. In: Research trends in environmental sciences, 2, pp. 71–86. Available online at: https://www.researchgate.net/publication/331132826.
Cheikhyoussef, A., Pogori, N., and Chen, H. (2009). Lyophilization of lactic acid bacteria-derived bacteriocins for prolonged shelf-life. Biotechnol. Lett. 31, 695–700. doi: 10.1007/s10529-009-9923-8
Chowdhury, S. P., Hartmann, A., Gao, X., and Borriss, R. (2015). Biocontrol mechanism by root-associated Bacillus species with a focus on secondary metabolites. Microb. Ecol. 69, 459–470. doi: 10.1007/s00248-014-0533-3
Compant, S., Duffy, B., Nowak, J., Clément, C., and Barka, E. A. (2005). Use of plant growth-promoting bacteria for biocontrol of plant diseases: principles, mechanisms of action, and future prospects. Appl. Environ. Microbiol. 71, 4951–4959. doi: 10.1128/AEM.71.9.4951-4959.2005
Corral, S., Salvador, A., Belloch, C., and Flores, M. (2014). Effect of fat and salt reduction on the sensory quality of slow fermented sausages inoculated with Debaryomyces hansenii yeast. Food Control 45, 1–7. doi: 10.1016/j.foodcont.2014.04.013
Cruz-Casas, D. E., Aguilar, C. N., Ascacio-Valdés, J. A., Rodríguez-Herrera, R., Chávez-González, M. L., and Flores-Gallegos, A. C. (2021). Enzymatic hydrolysis and microbial fermentation: the most favorable biotechnological methods for the release of bioactive peptides. Food Chem. Mol. Sci. 3:100047. doi: 10.1016/j.fochms.2021.100047
Debnath, B., Majumdar, M., Bhowmik, M., Bhowmik, K. L., Debnath, A., and Roy, D. N. (2020). The effective adsorption of tetracycline onto zirconia nanoparticles synthesized by novel microbial green technology. J. Environ. Manag. 261:110235. doi: 10.1016/j.jenvman.2020.110235
Di Rico, F., Vuolo, F., and Puglisi, E. (2025). Evaluating the role of viable cells, heat-killed cells or cell-free supernatants in bacterial biocontrol of fungi: a comparison between lactic acid bacteria and Pseudomonas. Microorganisms 13:105. doi: 10.3390/microorganisms13010105
Dong, Y.-H., Zhang, Q., Xu, J.-L., Li, X.-Z., and Zhang, L.-H. (2000). Quenching quorum sensing signal molecule by N-acyl homoserine lactonases. FEMS Microbiol. Lett. 184, 81–88.
Dozolme, P. M. A., and Moukha, S. M. (2020). The in vitro production potentialities of secondary toxic metabolites by the fungal factory Fusarium verticillioides is, fortunately, largely underestimated in fields: pioneering study on Fumonisins. Front. Microbiol. 11:562754. doi: 10.3389/fmicb.2020.562754
El Sabagh, A., Islam, M. S., Hossain, A., Iqbal, M. A., Mubeen, M., Waleed, M., et al. (2022). Phytohormones as growth regulators during abiotic stress tolerance in plants. Front. Agron. 4:765068. doi: 10.3389/fagro.2022.765068
Elsharkawy, M. M., Shimizu, M., Takahashi, H., Ozaki, K., and Hyakumachi, M. (2013). Induction of systemic resistance against cucumber mosaic virus in Arabidopsis thaliana by Trichoderma asperellum SKT-1. Plant Pathol. J. 29, 193–200. doi: 10.5423/PPJ.SI.07.2012.0117
Escobar-Muciño, E., Arenas-Hernández, M. M. P., and Luna-Guevara, M. L. (2022). Mechanisms of inhibition of quorum sensing as an alternative for the control of E. coli and Salmonella. Microorganisms 10:884. doi: 10.3390/microorganisms10050884
Eswaran, S. U. D., Sundaram, L., Perveen, K., Bukhari, N. A., and Sayyed, R. Z. (2024). Osmolyte-producing microbial biostimulants regulate the growth of Arachis hypogaea L. under drought stress. BMC Microbiol. 24:165. doi: 10.1186/s12866-024-03320-6
Feder-Mengus, C., Ghosh, S., Reschner, A., Martin, I., and Spagnoli, G. C. (2008). New dimensions in tumor immunology: what does 3D culture reveal? Trends Mol. Med. 14, 333–340. doi: 10.1016/j.molmed.2008.06.001.18614399
Franzetti, A., Gandolfi, I., Bestetti, G., Smyth, T. J., and Banat, I. M. (2010). Production and applications of trehalose lipid biosurfactants. Eur. J. Lipid Sci. Tech. 112, 617–627. doi: 10.1002/ejlt.200900162
Garg, A., Gupta, D., and Sharma, S. (2020). Stability and shelf life of microbial biopesticides: challenges and advancements. Biol. Control 143:104209. doi: 10.1016/j.biocontrol.2020.104209
Gautam, S., Sharma, R., Chauhan, A., Shirkot, C. K., and Kaushal, R. (2020). Biocontrol activities of rhizobacteria associated with apple, apricot and kiwi rhizosphere against bacterial canker caused by Clavibacter michiganensis. Indian Phytopathol. 73, 45–56. doi: 10.1007/s42360-019-00172-3
Ge, S., Han, J., Sun, Q., Ye, Z., Zhou, Q., Li, P., et al. (2024). Optimization of cryoprotectants for improving the freeze-dried survival rate of potential probiotic Lactococcus lactis ZFM559 and evaluation of its storage stability. LWT 198:116052. doi: 10.1016/j.lwt.2024.116052
Ghazy, N., and El-Nahrawy, S. (2021). Siderophore production by Bacillus subtilis MF497446 and Pseudomonas koreensis MG209738 and their efficacy in controlling Cephalosporium maydis in maize plant. Arch. Microbiol. 203, 1195–1209. doi: 10.1007/s00203-020-02113-5
Glick, B. R. (2020). “Introduction to Plant Growth-Promoting Bacteria” in Beneficial Plant-Bacterial Interactions (Cham: Springer), 1–37. doi: 10.1007/978-3-030-44368-9_1
Gudiña, E. J., Teixeira, J. A., and Rodrigues, L. R. (2015). Biosurfactant production by Bacillus strains isolated from dairy industries. Biochem. Eng. J. 101, 108–118. doi: 10.1016/j.bej.2015.05.006
Gullifa, G., Risoluti, R., Mazzoni, C., Barone, L., Papa, E., Battistini, A., et al. (2023). Microencapsulation by a spray drying approach to produce innovative probiotics-based products extending the shelf-life in non-refrigerated conditions. Molecules 28:860. doi: 10.3390/molecules28020860
Gunal Köroglu, D., Bilgin, A., Karabulut, G., Saricaoglu, B., and Capanoglu, E. (2024). Encapsulation of hydrophobic compounds in yeast cells: methods, characterization, and applications:252–74. doi: 10.37349/eff.2024.00037
Hamad, S., et al. (2022). Ethanol stabilization of quorum sensing inhibitors from Pseudomonas spp. Microb. Biotechnol. 15, 874–885. doi: 10.1111/1751-7915.14005
Hirao, T., Okazawa, A., Harada, K., Kobayashi, A., Muranaka, T., and Hirata, K. (2012). Green leaf volatiles enhance methyl jasmonate response in Arabidopsis. J. Biosci. Bioeng. 114, 540–545. doi: 10.1016/j.jbiosc.2012.06.010
Huang, S., Vignolles, M.-L., Chen, X. D., Le Loir, Y., Jan, G., Schuck, P., et al. (2017). Spray drying of probiotics and other food-grade bacteria: a review. Trends Food Sci. Technol. 63, 1–17. doi: 10.1016/j.tifs.2017.02.007
Ibitoye, D. O., and Kolawole, A. O. (2022). Farmers’ appraisal on okra [Abelmoschus esculentus (L.)] production and phenotypic characterization: a synergistic approach for improvement. Front. Plant Sci. 13, 1–9. doi: 10.3389/fpls.2022.787577
Idris, E. E., Bochow, H., Ross, H., and Borriss, R. (2004). Use of Bacillus subtilis as biocontrol agent. VI. Phytohormonelike action of culture filtrates prepared from plant growth-promoting Bacillus amyloliquefaciens FZB24, FZB42, FZB45 and Bacillus subtilis FZB37/Nutzung von Bacillus subtilis als Mittel für den biologischen Pflanzenschutz. VI. Phytohormonartige Wirkung von Kulturfiltraten von pflanzenwachstumsfördernden Bacillus amyloliquefaciens FZB24, FZB42, FZB45 und Bacillus subtilis FZB37. J. Plant Dis. Protect. 111, 583–597. Available at: https://www.jstor.org/stable/43215615
Imran, M., Abo-Elyousr, K. A. M., Mousa, M. A. A., and Saad, M. M. (2023). Use of Trichoderma culture filtrates as a sustainable approach to mitigate early blight disease of tomato and their influence on plant biomarkers and antioxidants production. Front. Plant Sci. 14:1192818. doi: 10.3389/fpls.2023.1192818
Islam, S., Akanda, A. M., Prova, A., Islam, M. T., and Hossain, M. M. (2016). Isolation and Identification of Plant Growth Promoting Rhizobacteria from Cucumber Rhizosphere and Their Effect on Plant Growth Promotion and Disease Suppression. Frontiers in microbiology 6:1360. doi: 10.3389/fmicb.2015.01360
Ismail, H. Y., Isa, T., Ngoshe, I. Y., and Gajere, H. M. (2018). Biosurfactant production by Bacteria isolated from hydrocarbon impacted soil. Bioremed. Sci. Technol. Res. 6, 1–4. doi: 10.54987/bstr.v6i2.430
Jadhav, H. P., Shaikh, S. S., and Sayyed, R. Z. (2017). “Role of hydrolytic enzymes of rhizoflora in biocontrol of fungal phytopathogens: an overview” in Rhizotrophs: Plant growth promotion to bioremediation, microorganisms for sustainability. ed. S. Mehnaz, vol. 2, 183–203.
Ji, C., Nguyen, L. N., Hou, J., Hai, F. I., and Chen, V. (2017). Direct immobilization of laccase on titania nanoparticles from crude enzyme extracts of P. ostreatus culture for micro-pollutant degradation. Sep. Purif. Technol. 178, 215–223. doi: 10.1016/j.seppur.2017.01.043
Kaewchomphunuch, T., Charoenpichitnunt, T., Thongbaiyai, V., Ngamwongsatit, N., and Kaeoket, K. (2022). Cell-free culture supernatants of Lactobacillus spp. and Pediococcus spp. inhibit growth of pathogenic Escherichia coli isolated from pigs in Thailand. BMC Vet. Res. 18, 60–13. doi: 10.1186/s12917-022-03140-8
Kantar, C., Demiray, H., and Dogan, N. M. (2010). Role of microbial exopolymeric substances on chromium sorption and transport in heterogeneous subsurface soil. II. Binding of Cr(III) in EPS/soil system. Chemosphere 82, 1489–1495. doi: 10.1016/j.chemosphere.2011.01.009
Khamsuk, K., Dell, B., Pathom-Aree, W., Pathaichindachote, W., Suphrom, N., Nakaew, N., et al. (2024). Screening Plant Growth-Promoting Bacteria with Antimicrobial Properties for Upland Rice. Journal of microbiology and biotechnology 34, 1029–1039. doi: 10.4014/jmb.2402.02008
Khan, W., Costa, C., Souleimanov, A., Prithiviraj, B., and Smith, D. L. (2011). Response of Arabidopsis thaliana roots to lipo-chitooligosaccharide from Bradyrhizobium japonicum and other chitin-like compounds. Plant Growth Regul. 63, 243–249. doi: 10.1007/s10725-010-9521-6
Khan, N., Martínez-Hidalgo, P., Ice, T. A., Maymon, M., Humm, E. A., Nejat, N., et al. (2018). Antifungal activity of Bacillus species against Fusarium and analysis of the potential mechanisms used in biocontrol. Front. Microbiol. 9:2363. doi: 10.3389/fmicb.2018.02363
Khan, N., Maymon, M., Hirsch, A. M., and Dasgupta, P. R. (2020). Isolation and characterization of plant growth-promoting rhizobacteria (PGPR) from agricultural fields. Appl. Soil Ecol. 150:103457. doi: 10.1016/j.apsoil.2019.103457
Köhl, J., Kolnaar, R., and Ravensberg, W. J. (2019). Mode of action of microbial biological control agents against plant diseases: relevance beyond efficacy. Front. Plant Sci. 10:845. doi: 10.3389/fpls.2019.00845
Koohestani, M., Moradi, M., Tajik, H., and Badali, A. (2018). Effects of cell-free supernatant of Lactobacillus acidophilus LA5 and Lactobacillus casei 431 against planktonic form and biofilm of Staphylococcus aureus. Vet. Res. Forum Fall 9, 301–306. doi: 10.30466/vrf.2018.33086
Kopittke, P. M., Menzies, N. W., Wang, P., McKenna, B. A., and Lombi, E. (2019). Soil and the intensification of agriculture for global food security. Environ. Int. 132:105078. doi: 10.1016/j.envint.2019.105078
Kristensen, M. F., Leonhardt, D., Neland, M. L. B., and Schlafer, S. (2020). A 3D printed microfluidic flow-cell for microscopy analysis of in situ-grown biofilms. J. Microbiol. Methods 172:105876. doi: 10.1016/j.mimet.2020.105876
Kudjordjie, E. N., Sapkota, R., Steffensen, S. K., Fomsgaard, I. S., and Nicolaisen, M. (2019). Maize synthesized benzoxazinoids affect the host associated microbiome. Microbiome 7:151. doi: 10.1186/s40168-019-0765-8
Kumar, S., Mishra, A., Sharma, S., and Vishwakarma, R. K. (2020). Plant growth promoting rhizobacteria: diversity and applications in agriculture. Environ. Sustain. 3, 15–27. doi: 10.1007/s42398-019-00113-3
Kumar, S., Patel, J. S., and Meena, R. S. (2021). Biostimulants in sustainable agriculture: advances and future outlook. Agronomy 11:199. doi: 10.3390/agronomy11020199
Lade, H., Paul, D., Kweon, J. H., and Yang, Y. H. (2014). Quorum sensing regulation and inhibition of virulence gene expression in Chromobacterium violaceum, an opportunistic human pathogen. Sci. Rep. 4:6839. doi: 10.1038/srep06839
Li, Y., Héloir, M., Zhang, X., Geissler, M., Trouvelot, S., Jacquens, L., et al. (2019). Surfactin and fengycin contribute to the protection of a Bacillus subtilis strain against grape downy mildew by both direct effect and defence stimulation. Mol. Plant Pathol. 20, 1037–1050. doi: 10.1111/mpp.12809
Li, P., Su, R., Yin, R., Lai, D., Wang, M., Liu, Y., et al. (2020). Detoxification of mycotoxins through biotransformation. Toxins 12, 1–37. doi: 10.3390/toxins12020121
Li, J., Wang, J., Liu, H., Macdonald, C. A., and Singh, B. K. (2022). Application of microbial inoculants significantly enhances crop productivity: a meta-analysis of studies from 2010 to 2020. J. Sustain. Agric. Environ. 1, 216–225. doi: 10.1002/sae2.12028
Li, B., Zhao, L., Liu, D., Zhang, Y., Wang, W., Miao, Y., et al. (2023). Bacillus subtilis Promotes Cucumber Growth and Quality under Higher Nutrient Solution by Altering the Rhizospheric Microbial Community. Plants (Basel, Switzerland) 12:298. doi: 10.3390/plants12020298
Lorenzo, O., Piqueras, R., Sánchez-Serrano, J. J., and Solano, R. (2003). Ethylene response factor1 integrates signals from ethylene and jasmonate pathways in plant defense. Plant Cell Online 15, 165–178. doi: 10.1105/tpc.007468
Mahanty, S., Chatterjee, S., Ghosh, S., Tudu, P., Gaine, T., Bakshi, M., et al. (2020). Synergistic approach towards the sustainable management of heavy metals in wastewater using mycosynthesized iron oxide nanoparticles: biofabrication, adsorptive dynamics and chemometric modeling study. J. Water Proces Eng. 37:101426. doi: 10.1016/j.jwpe.2020.101426
Mahmoud, Y. A. G., El-Halmouch, Y. H., Nasr, E. E., Al-Sodany, Y. M., El-Nogoumy, B. A., Ali, S. S., et al. (2024). Exploring the potential of biosurfactants produced by fungi found in soil contaminated with petrochemical wastes. Sci. Rep. 14:25733. doi: 10.1038/s41598-024-75865-5
Majeedras, H., Gill, B., and Bhanot, R. (2018). A comparative study on microbial supernatant extraction methods. Microb. Biotechnol. J. 5, 34–42. doi: 10.1007/s12355-018-0093-1
Mani-López, E., Arrioja-Bretón, D., and López-Malo, A. (2022). The impacts of antimicrobial and antifungal activity of cell-free supernatants from lactic acid bacteria in vitro and foods. Compr. Rev. Food Sci. Food Saf. 21, 604–641. doi: 10.1111/1541-4337.12872
Mao, Y., Wang, Y., Luo, X., Chen, X., and Wang, G. (2023). Impact of cell-free supernatant of lactic acid bacteria on Staphylococcus aureus biofilm and its metabolites. Front. Vet. Sci. 10:1184989. doi: 10.3389/fvets.2023.1184989
Marchant, R., and Banat, I. M. (2012). Biosurfactants: a sustainable replacement for chemical surfactants? Biotechnol. Lett. 34, 1597–1605. doi: 10.1007/s10529-012-0956-x
M’henni, Y. B., Salem, I. B., Souli, M., Tounsi, S., Debieu, D., Fillinger, S., et al. (2022). Biocontrol and growth promotion potential of combined application of Trichoderma simmonsii and aspergillus westerdijkiae against apple tree dieback disease. PhytoFrontiers 2, 268–279. doi: 10.1094/PHYTOFR-01-22-0005-R
Mihalache, G., Balaes, T., Gostin, I., Stefan, M., Coutte, F., and Krier, F. (2018). Lipopeptides produced by Bacillus subtilis as new biocontrol products against fusariosis in ornamental plants. Environ. Sci. Pollut. Res. 25, 29784–29793. doi: 10.1007/s11356-017-9162-7
Monjezi, N., Yaghoubian, I., and Smith, D. L. (2023). Cell-free supernatant of Devosia sp. (strain SL43) mitigates the adverse effects of salt stress on soybean (Glycine max L.) seed vigor index. Front. Plant Sci. 14:1071346. doi: 10.3389/fpls.2023.1071346
Morcillo, R. J., Singh, S. K., He, D., An, G., Vílchez, J. I., Tang, K., et al. (2020). Rhizobacterium-derived diacetyl modulates plant immunity in a phosphate-dependent manner. EMBO J. 39:e102602. doi: 10.15252/EMBJ.2019102602
Morcillo, R. J. L., Baroja-Fernández, E., López-Serrano, L., Leal-López, J., Muñoz, F. J., Bahaji, A., et al. (2022). Cell-free microbial culture filtrates as candidate biostimulants to enhance plant growth and yield and activate soil- and plant-associated beneficial microbiota. Front. Plant Sci. 13:1040515. doi: 10.3389/fpls.2022.1040515
Morel, M. A., Cagide, C., Minteguiaga, M. A., Dardanelli, M. S., and Castro-Sowinski, S. (2015). The pattern of secreted molecules during the co-inoculation of alfalfa plants with Sinorhizobium meliloti and Delftia sp. strain JD2: an interaction that improves plant yield. Mol. Plant-Microbe Interact. 28, 134–142. doi: 10.1094/MPMI-08-14-0229-R
Moretti, L. G., Crusciol, C. A. C., Kuramae, E. E., Bossolani, J. W., Moreira, A., Costa, N. R., et al. (2020). Effects of growth-promoting bacteria on soybean root activity, plant development, and yield. Agron. J. 112, 418–428. doi: 10.1002/agj2.20010
Naamala, J., Msimbira, L. A., Antar, M., Subramanian, S., and Smith, D. L. (2022). Cell-free supernatant obtained from a salt tolerant Bacillus amyloliquefaciens strain enhances germination and radicle length under NaCl stressed and optimal conditions. Front. Sustain. Food Syst. 6:788939. doi: 10.3389/fsufs.2022.788939
Naamala, J., Msimbira, L. A., Subramanian, S., and Smith, D. L. (2023). Lactobacillus helveticus EL2006H cell-free supernatant enhances growth variables in Zea mays (maize), Glycine max L. Merill (soybean) and Solanum tuberosum (potato) exposed to NaCl stress. Front. Microbiol. 13, 1–12. doi: 10.3389/fmicb.2022.1075633
Nair, A., Juwarkar, A. A., and Singh, S. K. (2007). Production and characterization of siderophores and its application in arsenic removal from contaminated soil. Water Air Soil Pollut. 180, 199–212. doi: 10.1007/s11270-006-9263-2
Nasrollahzadeh, A., Mokhtari, S., Khomeiri, M., and Saris, P. (2022). Mycotoxin detoxification of food by lactic acid bacteria. Food Contamination 9:1. doi: 10.1186/s40550-021-00087-w
Nikolić, I., Berić, T., Dimkić, I., Popović, T., Lozo, J., Fira, D., et al. (2019). Biological control of Pseudomonas syringae pv. Aptata on sugar beet with Bacillus pumilus SS-10.7 and Bacillus amyloliquefaciens (SS-12.6 and SS-38.4) strains. J. Appl. Microbiol. 126, 165–176. doi: 10.1111/jam.14070
Nurbailis, N., Djamaan, A., Rahma, H., and Liswarni, Y. (2019). Potential of culture filtrate from Trichoderma spp. as biofungicide to Colletotrichum gloeosporioides causing anthracnose disease in chili. Biodiversitas Journal of Biological Diversity. 20. doi: 10.13057/biodiv/d201020
O’Brien, S., Hodgson, D. J., and Buckling, A. (2014). Social evolution of toxic metal bioremediation in Pseudomonas aeruginosa. Proc. R. Soc. B Biol. Sci. 281:20140858. doi: 10.1098/rspb.2014.0858
Olanrewaju, O. S., Glick, B. R., and Babalola, O. O. (2019). Mechanisms of action of plant growth promoting bacteria. World J. Microbiol. Biotechnol. 35:33. doi: 10.1007/s11274-019-2604-1
Pacwa-Płociniczak, M., Płaza, G. A., Piotrowska-Seget, Z., and Cameotra, S. S. (2011). Environmental applications of biosurfactants: recent advances. Int. J. Mol. Sci. 12, 633–654. doi: 10.3390/ijms12010633
Patel, S., and Saraf, M. (2017). Applications of bioactive compounds in agriculture: their role in biotic stress management and crop productivity. Biotechnol. Rep. 15, 63–69. doi: 10.1016/j.btre.2017.06.001
Pellegrini, M., Pagnani, G., Bernardi, M., Mattedi, A., Spera, D. M., and Gallo, M. D. (2020). Cell-free supernatants of plant growth-promoting Bacteria: a review of their use as biostimulant and microbial biocontrol agents in sustainable agriculture. Sustain. For. 12:9917. doi: 10.3390/su12239917
Perczak, A., Goliński, P., Bryła, M., and Waśkiewicz, A. (2018). The efficiency of lactic acid bacteria against pathogenic fungi and mycotoxins. Arch. Ind. Hyg. Toxicol. 69, 32–45. doi: 10.2478/aiht-2018-69-3051
Pieterse, C. M. J., and Van Loon, L. C. (2007). “Signalling cascades involved in induced resistance” in Induced resistance for plant defence. eds. D. Walters, A. C. Newton, and G. Lyon (London: Blackwell), 65–88.
Pieterse, C. M. J., Zamioudis, C., Berendsen, R. L., Weller, D. M., Van Wees, S. C. M., and Bakker, P. A. H. M. (2014). Induced systemic resistance by beneficial microbes. Annu. Rev. Phytopathol. 52, 347–375. doi: 10.1146/annurev-phyto-082712-102340
Posada, L. F., Ramírez, M., Ochoa-Gómez, N., Cuellar-Gaviria, T. Z., Argel-Roldan, L. E., Ramírez, C. A., et al. (2016). Bioprospecting of aerobic endospore-forming bacteria with biotechnological potential for growth promotion of banana plants. Sci. Hortic. 212, 81–90. doi: 10.1016/j.scienta.2016.09.040
Radhakrishnan, R., Kang, S. M., Baek, I. Y., and Lee, I. J. (2014). Characterization of plant growth-promoting traits of Penicillium species against the effects of high soil salinity and root disease. J. Plant Interact. 9, 754–762. doi: 10.1080/17429145.2014.930524
Rasamiravaka, T., Vandeputte, O. M., Pottier, L., Huet, J., Rabemanantsoa, C., Kiendrebeogo, M., et al. (2015). Pseudomonas aeruginosa biofilm formation and quorum sensing are positively regulated by plant secondary metabolites. Microbes Environ. 30, 1, 58–66. doi: 10.1264/jsme2.ME14144
Rashid, R., Chelliah, R., and Rahman, S. (2021). Biosurfactant production and quorum sensing inhibition by Pseudomonas aeruginosa. J. Appl. Microbiol. 130, 785–797. doi: 10.1111/jam.14896
Reddy, M. S., Naresh, B., Leela, T., Prashanthi, M., Madhusudhan, N. C., Dhanasri, G., et al. (2010). Biodegradation of phenanthrene with biosurfactant production by a new strain of Brevibacillus sp. Bioresour. Technol. 101, 7980–7983. doi: 10.1016/j.biortech.2010.04.054
Rizvi, A., and Khan, M. S. (2018). Heavy metal-induced oxidative damage and root morphology alterations of maize (Zea mays L.) plants and stress mitigation by metal tolerant nitrogen-fixing Azotobacter chroococcum. Ecotoxicol. Environ. Saf. 157, 9–20. doi: 10.1016/j.ecoenv.2018.03.063
Rondina, A. B. L., dos Santos Sanzovo, A. W., Guimarães, G. S., Wendling, J. R., Nogueira, M. A., and Hungria, M. (2020). Changes in root morphological traits in soybean co-inoculated with Bradyrhizobium spp. and Azospirillum brasilense or treated with A. brasilense exudates. Biol. Fertil. Soils 56, 537–549. doi: 10.1007/s00374-020-01453-0
San Keskin, N. O., Celebioglu, A., Sarioglu, O. F., Uyar, T., and Tekinay, T. (2018). Encapsulation of living bacteria in electrospuncyclodextrin ultrathin fibers for bioremediation of heavy metals and reactive dye from waste water. Colloids Surf. B 161, 169–176. doi: 10.1016/j.colsurfb.2017.10.047
Santos, V. B., and Araújo, W. L. Plant growth promoting rhizobacteria (2017). “Fundamentals and applications” in Plant-microbe interactions in agro-ecological perspectives. eds. D. Singh, H. B. Singh, and R. Prabha (Singapore: Springer), 145–168.
Santoyo, G., Moreno-Hagelsieb, G., del Carmen, O.-M. M., and Glick, B. R. (2021). Plant growth-promoting bacterial endophytes. Microbiol. Res. 240:126549. doi: 10.1016/j.micres.2020.126549
Sarathy, J. P., Gruber, G., and Dick, T. (2019). Re-understanding the mechanisms of action of the anti-mycobacterial drug Bedaquiline. Antibiotics 8:261. doi: 10.3390/antibiotics8040261
Sellem, I., Triki, M. A., Elleuch, L., Cheffi, M., Chakchouk, A., Smaoui, S., et al. (2017). The use of newly isolated Streptomyces strain TN258 as potential biocontrol agent of potato tubers leak caused by Pythium ultimum. J. Basic Microbiol. 57, 393–401. doi: 10.1002/jobm.201600604
Sifour, M., Al-Jilawi, M. H., and Aziz, G. M. (2007). Emulsification properties of biosurfactant produced from Pseudomonas aeruginosa RB 28. Pak. J. Biol. Sci. 10, 1331–1335. doi: 10.3923/pjbs.2007.1331.1335
Silambarasan, S., Logeswari, P., Valentine, A., Cornejo, P., and Kannan, V. R. (2020). Pseudomonas citronellolis strain SLP6 enhances the phytoremediation efficiency of Helianthus annuus in copper contaminated soils under salinity stress. Plant Soil 457, 241–253. doi: 10.1007/s11104-020-04734-7
Simpson, R. (2010). Stabilization of Proteins for Storage. Cold Spring Harbor protocols 2010 :pdb.top79. doi: 10.1101/pdb.top79
Singh, P., Kim, Y. J., Zhang, D., and Yang, D. C. (2016). Biological synthesis of nanoparticles from plants and microorganisms. Trends Biotechnol. 34, 588–599. doi: 10.1016/j.tibtech.2016.02.006
Sivojiene, D., Kacergius, A., Baksiene, E., Maseviciene, A., and Zickiene, L. (2021). The Influence of organic fertilizers on the abundance of soil microorganism communities, agrochemical indicators, and yield in east Lithuanian light soils. Plants 10:2648. doi: 10.3390/plants10122648
Sornsenee, P., Chatatikun, M., Mitsuwan, W., Kongpol, K., Kooltheat, N., Sohbenalee, S., et al. (2021). Lyophilized cell-free supernatants of Lactobacillus isolates exhibited antibiofilm, antioxidant, and reduces nitric oxide activity in lipopolysaccharide-stimulated RAW 264.7 cells. PeerJ 9:e12586. doi: 10.7717/peerj.12586
Sravya, K., and Sangeetha, S. (2022). Feasibility study on bioremediation techniques to contaminated soils. Proc. Mater. Today 51, 2556–2560. doi: 10.1016/j.matpr.2021.12.364
Subramanian, S., Souleimanov, A., and Smith, D. L. (2021). Thuricin17 production and proteome differences in Bacillus thuringiensis NEB17 cell-free supernatant under NaCl stress. Front. Sustain. Food Syst. 5:630628. doi: 10.3389/fsufs.2021.630628
Šuchová, K., Fehér, C., Ravn, J. L., Bedő, S., Biely, P., and Geijer, C. (2022). Cellulose- and xylan-degrading yeasts: Enzymes, applications and biotechnological potential. Biotechnol. Adv. 59:107981. doi: 10.1016/j.biotechadv.2022.107981
Tewari, S., Pooniya, V., and Sharma, S. (2020). Next generation bioformulation prepared by amalgamating Bradyrhizobium, cell free culture supernatant, and exopolysaccharides enhances the indigenous rhizospheric rhizobial population, nodulation, and productivity of pigeon pea. Appl. Soil Ecol. 147:103363. doi: 10.1016/j.apsoil.2019.103363
Trinh, L. L., Le, K. N., Le Lam, H. A., and Nguyen, H. H. (2025). Cell-free supernatants from plant growth-promoting rhizobacteria Bacillus albus strains control aspergillus flavus disease in peanut and maize seedlings. Beni-Suef Univ. J. Basic Appl. Sci. 14:4. doi: 10.1186/s43088-025-00594-1
Umar, A., Zafar, A., Wali, H., Siddique, M. P., Qazi, M. A., Naeem, A. H., et al. (2021). Low-cost production and application of lipopeptide for bioremediation and plant growth by Bacillus subtilis SNW3. AMB Express 11:165. doi: 10.1186/s13568-021-01327-0
Uroz, S., Dessaux, Y., and Oger, P. (2009). Quorum sensing and quorum quenching: the yin and yang of bacterial communication. Chembiochem 10, 205–216. doi: 10.1002/cbic.200800521
Veliz, E. A., Martínez-Hidalgo, P., and Hirsch, A. M. (2017). Chitinase-producing bacteria and their role in biocontrol. AIMS microbiology 3, 689–705. doi: 10.3934/microbiol.2017.3.689
Villegas-escobar, V., González-jaramillo, L. M., Ramírez, M., Natalia, R., Sierra-zapata, L., Orduz, S., et al. (2018). Lipopeptides from Bacillus sp. EA-CB0959: active metabolites responsible for in vitro and in vivo control of Ralstonia solanacearum biol. Control 125, 20–28. doi: 10.1016/j.scienta.2017.07.034
Wang, Q., Xiong, D., Zhao, P., Yu, X., Tu, B., and Wang, G. (2011). Effect of applying an arsenic-resistant and plant growth-promoting rhizobacterium to enhance soil arsenic phytoremediation by Populus deltoides LH05-17. J. Appl. Microbiol. 111, 1065–1074. doi: 10.1111/j.1365-2672.2011.05142.x
Wang, X. Q., Zhao, D. L., Shen, L. L., Jing, C. L., and Zhang, C. S. (2018). “Application and mechanisms of Bacillus subtilis in biological control of plant disease” in Role of Rhizospheric microbes in soil. ed. V. Meena (Singapore: Springer), 225–250. doi: 10.1007/978-981-10-8402-7_9
Xu, Y., Yu, Y., Shen, Y., Li, Q., Lan, J., Wu, Y., et al. (2021). Effects of Bacillus subtilis and Bacillus licheniformis on growth performance, immunity, short chain fatty acid production, antioxidant capacity, and cecal microflora in broilers. Poult. Sci. 100:101358. doi: 10.1016/j.psj.2021.101358
Yaghoubian, I., Msimbira, L. A., and Smith, D. L. (2022). Cell-free supernatant of Bacillus strains canImprove seed vigor index of corn (Zea mays L.) under salinity stress. Front. Sustain. Food Syst. 6:857643. doi: 10.3389/fsufs.2022.857643
Yuan, Y., Wu, K., Liu, F., and Dong, X. (2020). Isolation and identification of plant growth-promoting bacteria and their effects on sustainable crop production. J. Plant Nutr. 43, 1447–1464.
Yadav, R., and Yadav, N. (2023). Secondary Metabolites Production in Plant Tissue Culture: A Review. International Educational Scientific Research Journal 2, 63–64. Available at: https://www.researchgate.net/publication/367284273_SECONDARYMETABOLITESPRODUCTIONINPLANT_TISSUECULTUREAREVIEW/link/63ca67c6d9fb5967c2ee8beb/download?_tp=eyJjb250ZXh0Ijp7InBhZ2UiOiJwdWJsaWNhdGlvbiIsInByZXZpb3VzUGFnZSI6bnVsbCwic3ViUGFnZSI6bnVsbH19
Zhang, S., Deng, Z., Borham, A., Ma, Y., Wang, Y., Hu, J., et al. (2023). Significance of Soil Siderophore-Producing Bacteria in Evaluation and Elevation of Crop Yield. Horticulturae 9:370. doi: 10.3390/horticulturae9030370
Keywords: agrobiotechnology, biofertilizers, bioinoculants, bioremediation, sustainable food production
Citation: Alori ET, Onaolapo AO and Ibaba AL (2025) Cell free supernatant for sustainable crop production. Front. Sustain. Food Syst. 9:1549048. doi: 10.3389/fsufs.2025.1549048
Received: 20 December 2024; Accepted: 10 March 2025;
Published: 26 March 2025.
Edited by:
Raffaella Balestrini, National Research Council (CNR), ItalyReviewed by:
V. S. Saravanan, Pondicherry University, IndiaCopyright © 2025 Alori, Onaolapo and Ibaba. This is an open-access article distributed under the terms of the Creative Commons Attribution License (CC BY). The use, distribution or reproduction in other forums is permitted, provided the original author(s) and the copyright owner(s) are credited and that the original publication in this journal is cited, in accordance with accepted academic practice. No use, distribution or reproduction is permitted which does not comply with these terms.
*Correspondence: Elizabeth Temitope Alori, YWxvcml0b3BlQHlhaG9vLmNvbQ==
†ORCID: Elizabeth Temitope Alori, https://orcid.org/0000-0003-0291-4773
Disclaimer: All claims expressed in this article are solely those of the authors and do not necessarily represent those of their affiliated organizations, or those of the publisher, the editors and the reviewers. Any product that may be evaluated in this article or claim that may be made by its manufacturer is not guaranteed or endorsed by the publisher.
Research integrity at Frontiers
Learn more about the work of our research integrity team to safeguard the quality of each article we publish.