- 1Technology Innovation Center for Land Engineering and Human Settlements, Shaanxi Land Engineering Construction Group Co., Ltd and Xi’an Jiaotong University, Xi’an, China
- 2School of Water Resources and Hydropower, Xi'an University of Technology, Xi'an, China
- 3Shaanxi Provincial Land Engineering Construction Group Co., Ltd., Xi'an, China
Phosphorus is a vital nutrient for crop growth, but its bioavailability is often limited in acidic soils, which are prevalent in many agricultural regions, including South China. These soils are characterized by low phosphorus availability and high levels of phosphorus fixation, which exacerbate the need for sustainable agricultural practices. Over-reliance on phosphate fertilizers has led to environmental concerns, such as phosphorus accumulation and eutrophication. This study investigates the potential of straw biochar to improve phosphorus dynamics in two typical South Chinese soils: high-phosphorus paddy soil and low-phosphorus lateritic red soil. Using a range of biochar application rates, we examined the effects on phosphorus fractions, phosphatase activity, and microbial biomass phosphorus. The results indicate that biochar significantly increased phosphorus availability, enhanced soil enzyme activity, and boosted microbial phosphorus content, especially in the phosphorus-deficient red soil. These findings underscore the potential of biochar as a sustainable solution for enhancing phosphorus management, improving soil fertility, and reducing environmental risks in South China and similar regions. This research contributes valuable insights into biochar’s practical applications in sustainable agriculture, offering a promising approach to improve phosphorus use efficiency and soil health.
1 Introduction
Phosphorus is an essential nutrient for crop growth, playing a critical role in enhancing stress resistance, improving water and nutrient uptake, and supporting overall plant productivity (Rai et al., 2021). However, its bioavailability in soil, which is crucial for plant uptake, is highly dependent on soil conditions and the chemical forms in which phosphorus is present (Waqas et al., 2019). In acidic soils, phosphorus is often precipitated and adsorbed by metal oxides and clay minerals, resulting in low availability to plants (Ma et al., 2016). This issue is particularly pronounced in lateritic red soils, where positive surface charges promote phosphorus adsorption, and free iron and aluminum in the soil solution lead to the formation of insoluble phosphate compounds (Vassileva et al., 1997). Consequently, phosphorus becomes effectively “locked” in these soils, limiting its accessibility to plants and, by extension, crop productivity.
In recent years, the excessive use of phosphate fertilizers has compounded these challenges, leading to phosphorus accumulation in soils, particularly in China, where fertilizer overuse is a significant issue (Zhang et al., 2021). This accumulation not only disrupts essential plant processes—such as zinc and manganese metabolism—but also increases the risk of environmental pollution, particularly non-point source pollution, contributing to eutrophication in water bodies (Zou et al., 2020; Ren et al., 2024). The rising phosphorus levels, coupled with the environmental hazards associated with its over-application, underscore the urgent need for innovative solutions to manage phosphorus in agricultural systems.
Biochar, particularly rice straw biochar, has emerged as a potential solution to address these phosphorus-related challenges. Rice straw, a byproduct of one of the world’s most widely cultivated crops, is available in vast quantities, especially in China, which generates significant amounts of rice straw annually (Alengebawy et al., 2023). The use of rice straw biochar not only provides a means to recycle agricultural waste but also has been shown to improve phosphorus availability in soils through several mechanisms. Biochar contains phosphorus itself (Dai et al., 2021), and its application can enhance soil phosphorus availability through multiple pathways: (1) by increasing soil pH, which reduces phosphorus adsorption by metal ions like iron and aluminum (Zheng et al., 2020; Ghodszad et al., 2022); (2) through cation bridging and adsorption properties, which facilitate phosphorus desorption (Yang et al., 2021); and (3) by altering soil microbial activity, thereby enhancing phosphorus cycling and transformation (Hu et al., 2023). However, while biochar has demonstrated potential benefits in many studies, its impact is highly context-dependent, varying with factors such as soil properties and biochar application rates (Tian et al., 2021; Wang et al., 2021).
Rice straw biochar, in particular, has been shown to hold promise for enhancing soil quality, with significant benefits observed in improving phosphorus availability in soils with different phosphorus profiles (Yang et al., 2021; Kamali et al., 2022). With the global production of rice straw reaching approximately 247 million tons annually (Ingrao et al., 2021), biochar produced from this material represents a valuable resource for both soil management and waste recycling. Given this, it is critical to investigate how rice straw biochar specifically affects phosphorus dynamics in different soil types.
This study focuses on two distinct soil types from South China—high-phosphorus paddy soil and low-phosphorus lateritic red soil—chosen for their contrasting phosphorus characteristics and relevance to the broader agricultural landscape in the region. Through controlled indoor cultivation experiments, we aim to explore how varying rates of rice straw biochar application influence phosphorus availability, its fractions, and phosphatase activity. Our findings will provide new insights into how biochar can be used to manage phosphorus in agricultural systems, filling important gaps in the literature regarding the role of biochar in regulating phosphorus dynamics across different soil types.
2 Materials and methods
2.1 Experimental site
The study was conducted at two locations in South China: the paddy soil was sourced from the ecological experimental farm of South China Agricultural University (23°9′N, 113°21′E), while the lateritic red soil was collected from the Ningxi experimental base (23°14′N, 113°38′E) in Zengcheng District, Guangzhou. The paddy soil, with a history of high phosphorus fertilization, was chosen for its elevated phosphorus content. In contrast, the lateritic red soil, typical of upland regions, has relatively low phosphorus levels. The key soil properties—pH, organic matter, and available phosphorus—were determined prior to the experiment (Table 1). Rice straw biochar, used for soil amendment, was produced by Liaoning Jinhefu Agricultural Development Co., Ltd., at a pyrolysis temperature of 600°C. The biochar had a pH of 9.54, and its elemental composition was as follows: C 61.78%, O 22.64%, N 1.89%, K 0.89%, and total phosphorus content of 5.37 g/kg.
2.2 Experimental design
The experiment utilized a pot-based setup, with each pot containing 2 kg of soil (fresh weight). Biochar was added to the soil at varying application rates: 0, 10, 20, and 30 g·kg−1 soil. Biochar was evenly mixed with the soil to simulate field conditions, and the soil was lightly compacted to maintain consistency.
Pots (25 cm in diameter, 30 cm in height) were used to ensure adequate root growth and water retention. For consistency, each treatment was replicated three times (n = 3), and the pots were placed in a greenhouse with temperatures ranging from 25 to 30°C and natural light exposure. The experiment took place from June to September 2020.
In a separate experimental setup, the effect of biochar application rates on soil was examined in 40 plastic pots (each 11 cm bottom diameter, 17 cm outer diameter, and 11 cm height) containing 800 g of soil per pot. Biochar was added at four application levels: 0% (CK), 1% (T1), 2% (T2), and 4% (T4) by soil mass. The soil moisture was maintained at 60–70% of the field capacity, and pots were placed in a controlled climate incubator at 25°C for 40 days without light exposure (Ji et al., 2021; Xie et al., 2021; Alsajri et al., 2022). Soil samples were collected every 10 days to analyze phosphorus dynamics.
2.3 Determination methods
2.3.1 Soil and biochar pH
The pH of biochar and soil was measured using a 1:2.5 solid-to-solvent ratio with ultrapure water. The mixture was shaken and allowed to settle before measuring with a pH meter (F2-Meter, Mettler Toledo, Switzerland).
2.3.2 Biochar elemental analysis
Elemental composition (C, O, N, K) of the biochar was determined using an elemental analyzer (Vario ELcube, Elementar, Germany).
2.3.3 Phosphorus content in biochar
Total phosphorus in the biochar was measured using the HClO₄-H₂SO₄ digestion method, followed by colorimetric analysis using the molybdenum-antimony method (Murphy and Riley, 1962; Zhang et al., 2021).
2.3.4 Soil nutrient analysis
Soil properties including alkali-hydrolyzable nitrogen, available potassium, organic matter, total phosphorus, and available phosphorus were analyzed following Bao’s soil agricultural and chemical methods.
2.3.5 Phosphorus fractionation
Soil inorganic phosphorus fractions (water-soluble phosphorus, Al-P, Fe-P, O-P, and Ca-P) were determined using a sequential extraction method (Zhang et al., 2021).
2.3.6 Phosphatase activity
Soil acid phosphatase (S-ACP) and alkaline phosphatase (S-AKP) activities were measured using commercial assay kits (BC0140 and BC0280, Solarbio Life Sciences, Beijing, China), following the manufacturer’s instructions.
2.3.7 Soil microbial biomass phosphorus
Soil microbial biomass phosphorus (MBP) was determined using the chloroform fumigation-extraction method (Fan et al., 2021).
2.4 Data processing
Data were processed using SPSS 20.0 software for one-way analysis of variance (ANOVA). Duncan’s new multiple range test was applied to determine the significance of differences between treatments (p < 0.05). SigmaPlot 14.0 was used to generate graphs, with results presented as means ± standard deviation.
3 Results
3.1 Effects of straw biochar treatment on soil phosphorus fractions and availability
As shown in Figure 1, the addition of straw biochar improved the total phosphorus content in both paddy soil and lateritic red soil. On the 10th day of the experiment, the total phosphorus content in paddy soil with treatments T1, T2, and T4 increased by an average of 4.04, 6.06, and 8.08%, respectively, compared to the control (CK), while in the lateritic red soil, the increases were 31.58, 26.32, and 52.63%, respectively. By the 40th day, the total phosphorus content in both soil types treated with biochar followed the order of T1 > T2 > T4 > CK. Specifically, the total phosphorus content in paddy soil treated with T1, T2, and T4 increased by 8.08, 6.06, and 5.05%, respectively, over the control, and in the lateritic red soil, the increases were 42.11, 21.05, and 10.53%, respectively. In general, the effect of adding straw biochar on enhancing the total phosphorus content was more pronounced in the lateritic red soil.
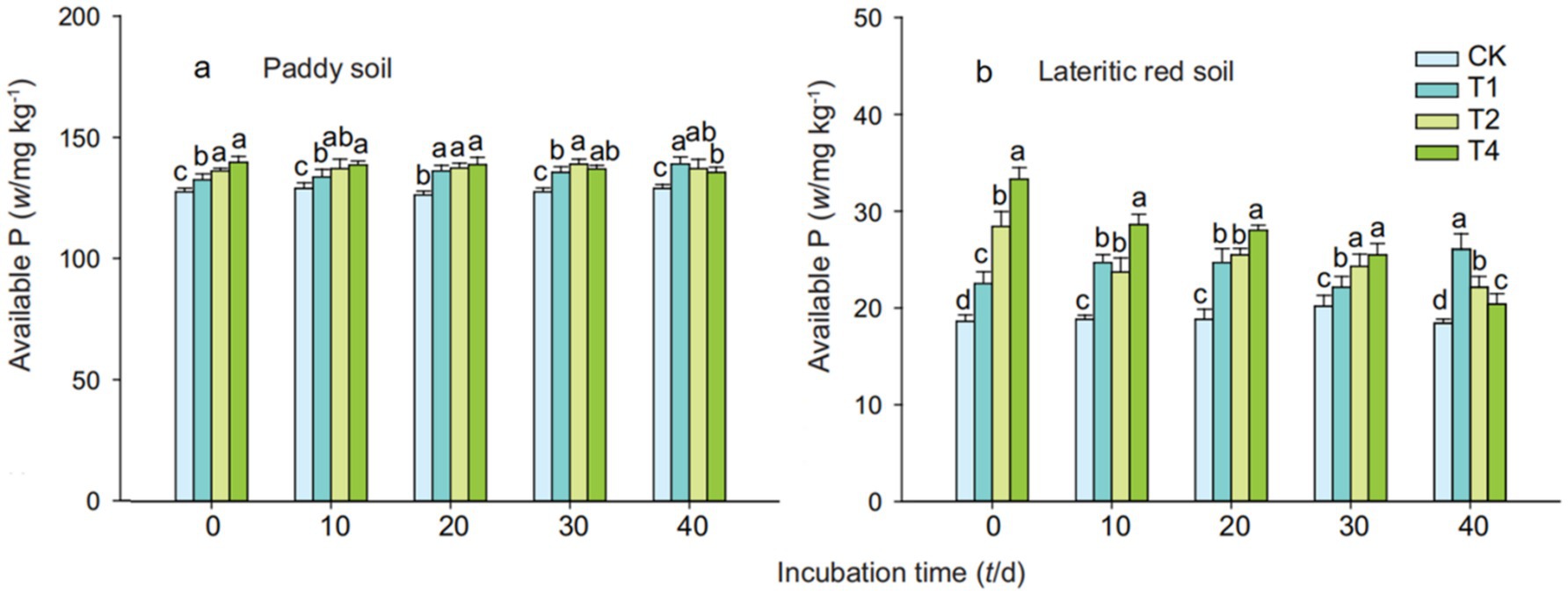
Figure 1. Effects of straw biochar treatments on total phosphorus content in soils. CK, T1, T2, and T4 represent biochar addition levels of 0%, 1%, 2%, and 4% of soil weight, respectively. Part labels (a, b) indicate different time periods (e.g., Day 10, Day 20, Day 40). Error bars show standard deviation ((n = 3). Different lowercase letters above bars indicate statistically significant differences (p < 0.05) between treatments within the same time period, as determined by Duncan’s multiple range test.
Figure 2 indicates that the addition of straw biochar significantly increased the available phosphorus content in both soil types, with the increase becoming more significant as the amount of biochar added was increased. On the 40th day of cultivation, the available phosphorus content in paddy soil treated with T1, T2, and T4 increased by 45.48, 89.11, and 118.45%, respectively, over the control; in the lateritic red soil, the increases were 792.45, 3575.47, and 6432.08%, respectively. It can be observed that as the cultivation period extended, the change in available phosphorus content in paddy soil treated with straw biochar was not significant, whereas the effect of high-dose biochar addition on increasing the available phosphorus content in the lateritic red soil was more pronounced.
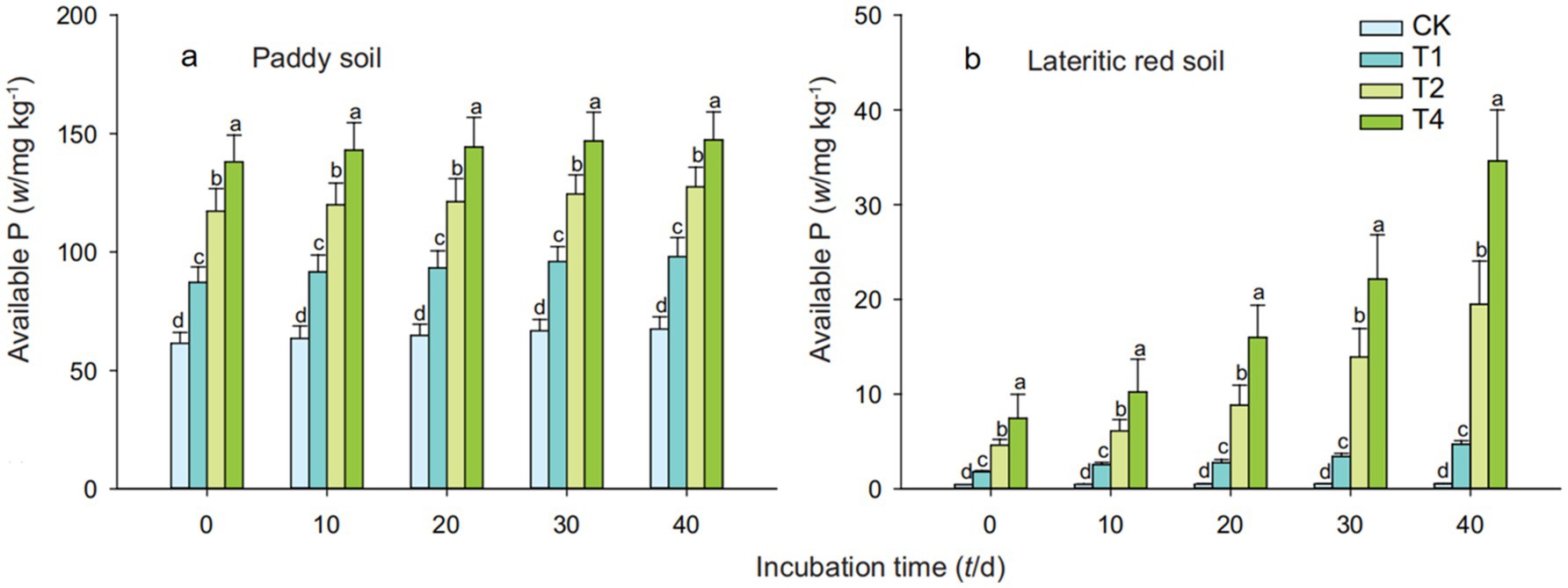
Figure 2. Effects of straw biochar treatments on available phosphorus content in soils description. Available phosphorus content increased with biochar. CK, T1, T2, and T4 represent biochar addition levels of 0%, 1%, 2%, and 4%, respectively. Part labels (a, b) correspond to different time periods of the experiment. Error bars represent standard deviation (n = 3). Different letters above bars indicate significant differences (p < 0.05) within the same time period.
It can be observed from Figure 3 that the addition of straw biochar significantly increased the content of water-soluble phosphorus, Al-P (Aluminum-bound Phosphorus), Fe-P (Iron-bound Phosphorus), and Ca-P (Calcium-bound Phosphorus) in paddy soil at various time points. Specifically, as shown in Figure 3A, compared to the control, the content of water-soluble phosphorus in paddy soil treated with T4 gradually decreased over the cultivation period, with an increase of 233.53% over the control (CK) by the 40th day. Figure 3B indicates that on the 40th day, the Al-P content in paddy soil treated with T1, T2, and T4 increased by 5.20, 9.92, and 14.95%, respectively, compared to CK. Figure 3C shows that on the 20th day of cultivation, the Fe-P content in paddy soil with the addition of 1, 2, and 4% straw biochar increased by 2.27, 7.11, and 9.83%, respectively, compared to the control. Figure 3D illustrates that the O-P (Organic Phosphorus) content in paddy soil treated with straw biochar gradually decreased over the cultivation period, with T1, T2, and T4 treatments showing a decrease of 1.92, 4.12, and 2.74%, respectively, over CK on the 40th day. Figure 3E shows that the addition of straw biochar increased the Ca-P content in paddy soil, with the increase being greater as the amount of biochar added increased. On the 40th day of cultivation, the Ca-P content in soil treated with 1, 2, and 4% straw biochar increased by 9.84, 28.67, and 55.65%, respectively, compared to the control.
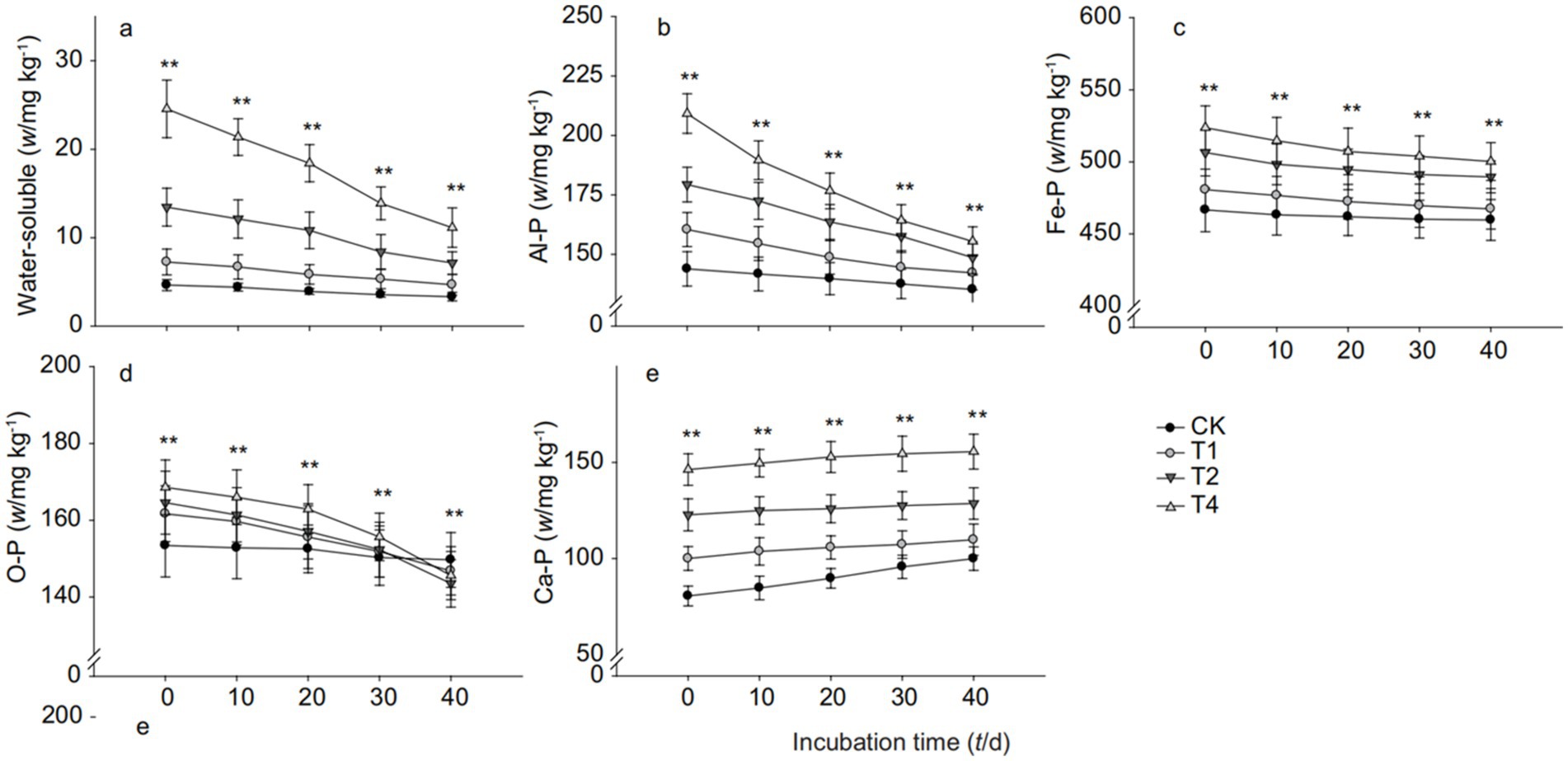
Figure 3. Inorganic phosphorus fraction changes in paddy soil with biochar addition. (a) Water-soluble phosphorus, (b) Aluminum-bound phosphorus (Al-P), (c) Iron-bound phosphorus (Fe-P), (d) Organic phosphorus (O-P), and (e) Calcium-bound phosphorus (Ca-P). CK, T1, T2, and T4 represent biochar addition levels of 0%, 1%, 2%, and 4%, respectively. Part labels correspond to the various phosphorus fractions measured. Different lowercase letters indicate statistically significant differences (p < 0.05).
From Figure 4A, it can be seen that on the 10th day of cultivation, the water-soluble phosphorus content in the lateritic red soil followed the order of T4 > T2 > T1 > CK, and by the 40th day, the water-soluble phosphorus content in the soil treated with straw biochar was all lower than that of the blank control, with decreases of 17.86, 8.93, and 7.14% for T1, T2, and T4 treatments, respectively, compared to CK. Figure 4B shows that the Al-P content in the lateritic red soil decreased gradually over time after the addition of straw biochar. Figure 4C indicates that the Fe-P content in the lateritic red soil treated with T2 and T4 of straw biochar significantly increased compared to CK, with increases of 5.69, 35.17, and 68.74% for T1, T2, and T4 treatments, respectively, on the 20th day. Figure 4D shows that the O-P content in the lateritic red soil treated with straw biochar gradually decreased over the cultivation period, with T2 and T4 treatments showing decreases of 2.27 and 0.52%, respectively, compared to CK on the 40th day. Figure 4E demonstrates that the addition of straw biochar increased the Ca-P content in the lateritic red soil, with the increase being more significant as the amount of biochar added was increased. On the 40th day of cultivation, the Ca-P content in the lateritic red soil treated with 1, 2, and 4% straw biochar increased by 13.46, 51.37, and 124.73%, respectively, compared to the control.
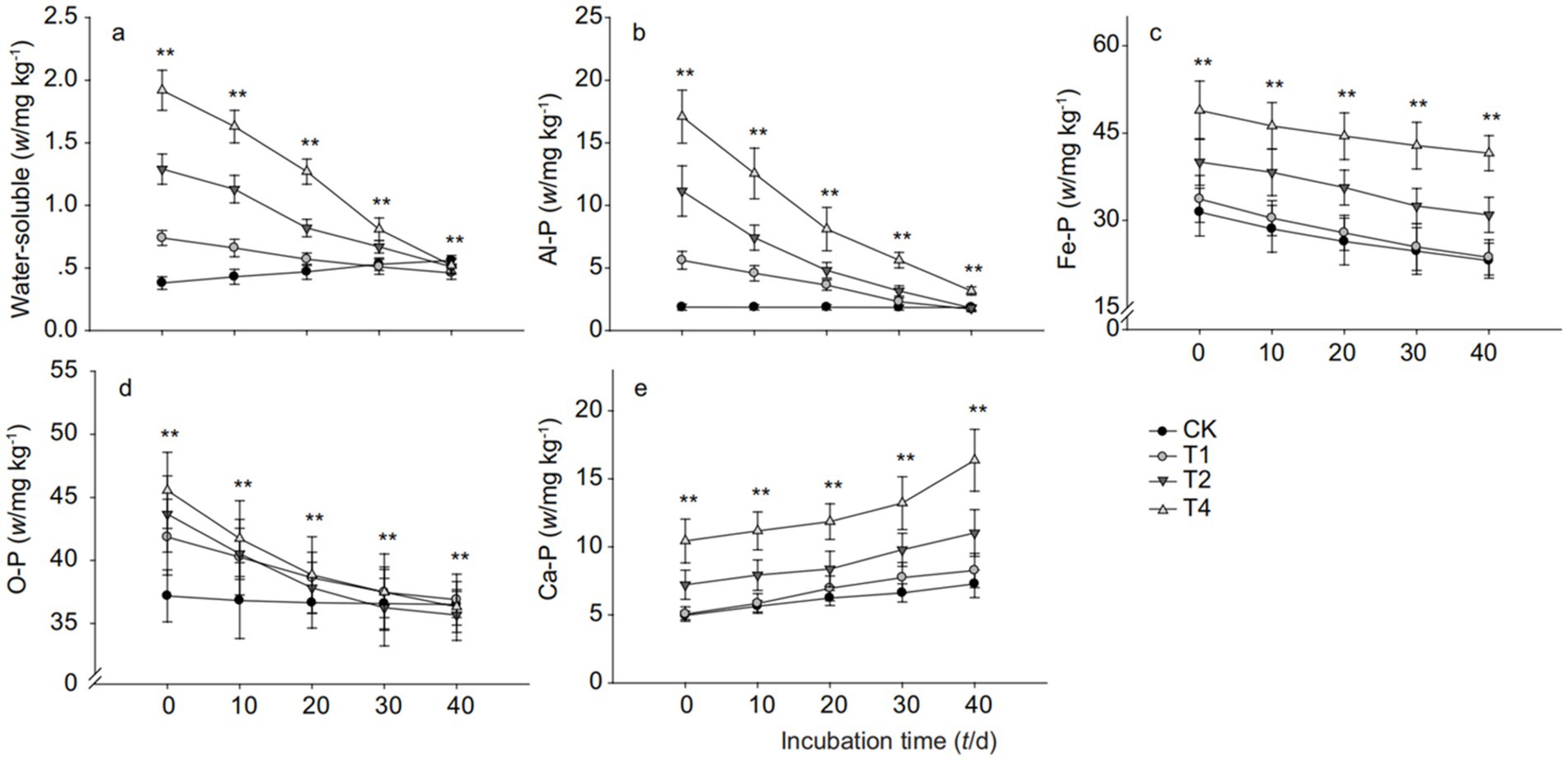
Figure 4. Inorganic phosphorus fraction changes in lateritic red soil with biochar addition. (a) Water-soluble phosphorus, (b) Aluminum-bound phosphorus (Al-P), (c) Iron-bound phosphorus (Fe-P), (d) Organic phosphorus (O-P), and (e) Calcium-bound phosphorus (Ca-P). CK, T1, T2, and T4 represent biochar addition levels of 0%, 1%, 2%, and 4%, respectively. Each part label corresponds to a specific phosphorus fraction. Different letters signify statistically significant differences (p < 0.05).
3.2 Effects of straw biochar treatment on soil phosphatase activity
As depicted in Figure 5, the application of straw biochar led to a significant reduction in the activity of acid phosphatase in both types of soils, indicating that the addition of straw biochar suppresses the activity of soil acid phosphatase. This suppression effect intensified with an increase in the dosage of biochar, resulting in significant differences between treatments. Over the course of the cultivation period, the activity of acid phosphatase in both soils treated with biochar gradually decreased. By the end of the 40-day cultivation period, the average reduction in acid phosphatase activity in paddy soil treated with 1, 2, and 4% straw biochar was 27.04, 37.69, and 50.86%, respectively, while in the lateritic red soil, the reductions were 30.39, 55.22, and 59.84%, respectively.
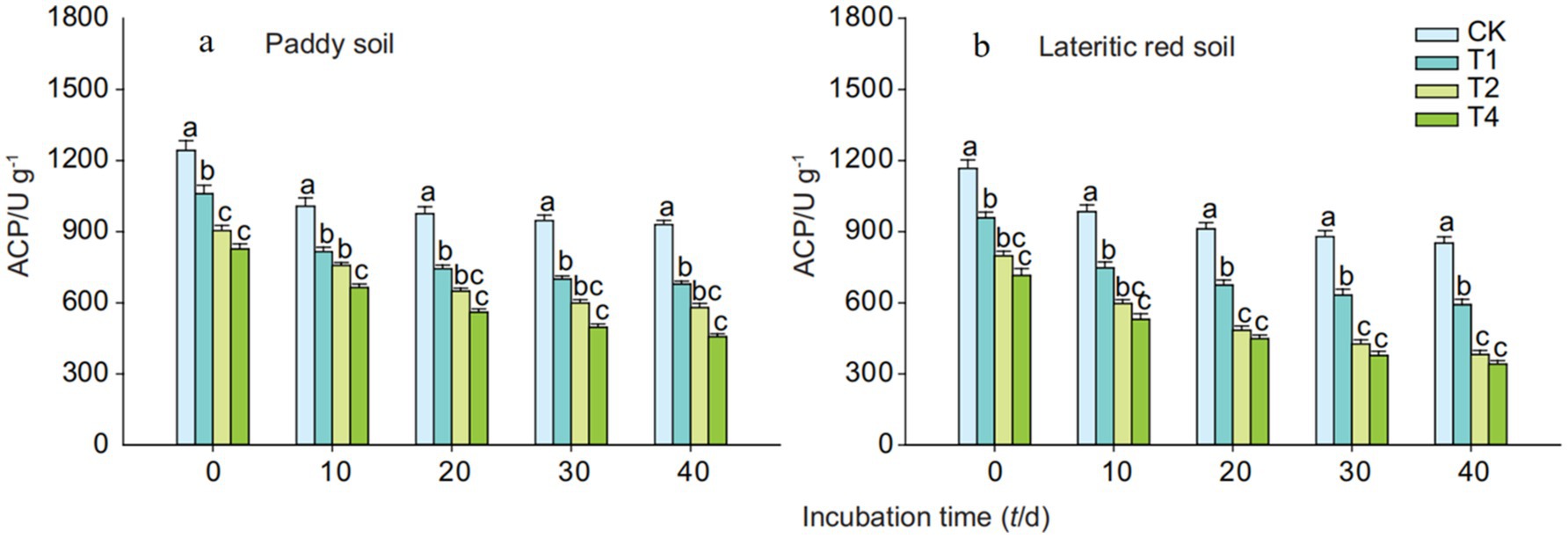
Figure 5. Effects of biochar on acid phosphatase activity in paddy and lateritic red soils. CK, T1, T2, and T4 represent biochar addition levels of 0%, 1%, 2%, and 4%, respectively. Acid phosphatase activity was measured over time. Part labels (a, b) correspond to soil types (paddy soil and lateritic red soil). Error bars represent standard deviation (n = 3). Different lowercase letters indicate statistically significant differences (p < 0.05) within each treatment group.
Figure 6 shows that on the 0th day after the addition of straw biochar, there was a significant decrease in the activity of alkaline phosphatase in both paddy soil and lateritic red soil. However, as the cultivation progressed, the activity of alkaline phosphatase in the biochar-treated soils became comparable to that of the control, and by the 40th day, the activity in the biochar-amended groups was higher than in the control. This suggests that the addition of straw biochar increases the activity of soil alkaline phosphatase, with the increase being proportional to the amount of biochar added. Specifically, after 40 days of cultivation, the activity of alkaline phosphatase in paddy soil treated with 1, 2, and 4% straw biochar increased by 10.32, 16.68, and 23.91%, respectively, compared to the control. Similarly, in the lateritic red soil, the activity of alkaline phosphatase increased by 8.26, 13.93, and 20.49%, respectively, for the same biochar treatments.
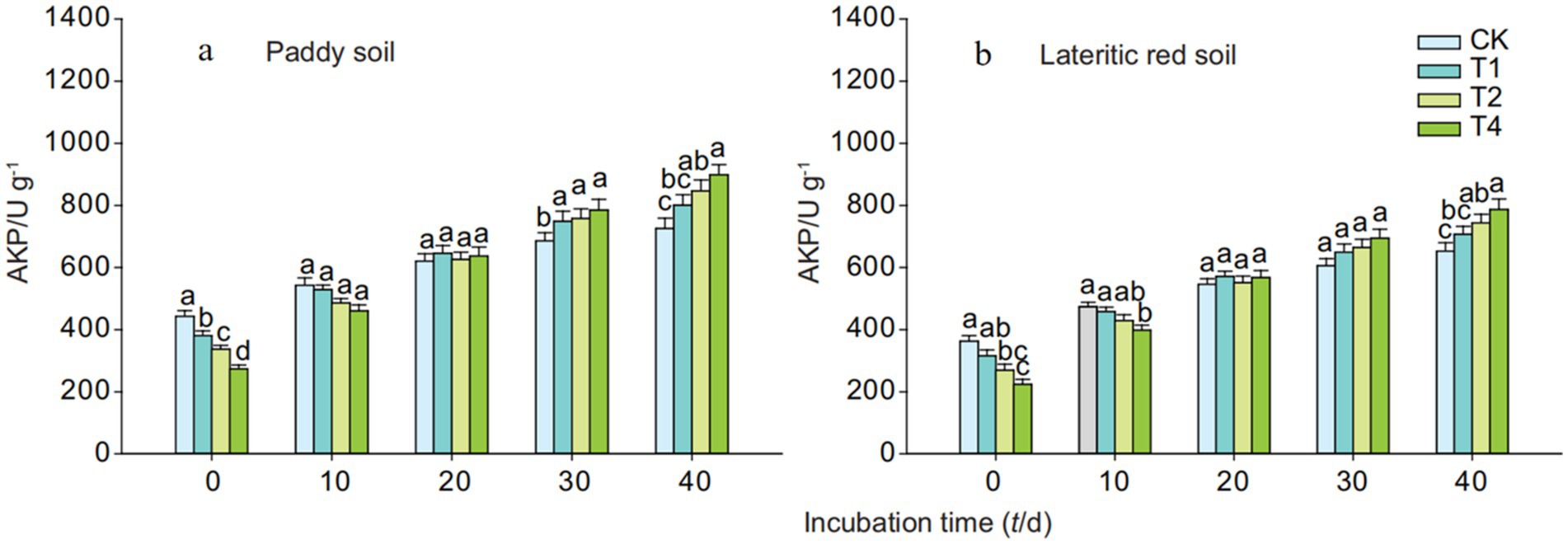
Figure 6. Effects of biochar on alkaline phosphatase activity in paddy and lateritic red soils. CK, T1, T2, and T4 represent biochar addition levels of 0%, 1%, 2%, and 4%, respectively. Alkaline phosphatase activity was measured over time. Part labels (a, b) correspond to soil types. Error bars represent standard deviation (n = 3). Different letters above bars represent statistically significant differences (p < 0.05).
3.3 Effects of straw biochar treatment on soil microbial biomass phosphorus
As depicted in Figure 7, the treatment with straw biochar did not have a significant impact on the microbial biomass phosphorus content in either of the two soils. Initially, on the 0th day of cultivation, the addition of biochar resulted in a decrease in the microbial biomass phosphorus content in both paddy soil and lateritic red soil, with the decrease becoming more pronounced as the amount of biochar added increased. Over time, the microbial biomass phosphorus content in both biochar-treated soils showed an increasing trend. By the 10th to 40th day of cultivation, the microbial biomass phosphorus content in paddy soil treated with biochar stabilized, ranging from 26.17 to 37.97 mg/kg, and the content in the T1, T2, and T4 treatments exceeded that of the control (CK) after 20 days of cultivation. In the case of lateritic red soil, the microbial biomass phosphorus content also stabilized between the 20th and 40th day of cultivation, with values ranging from 5.03 to 7.69 mg/kg, and throughout the cultivation period, the microbial biomass phosphorus content in the biochar-treated groups remained lower than that of the control.
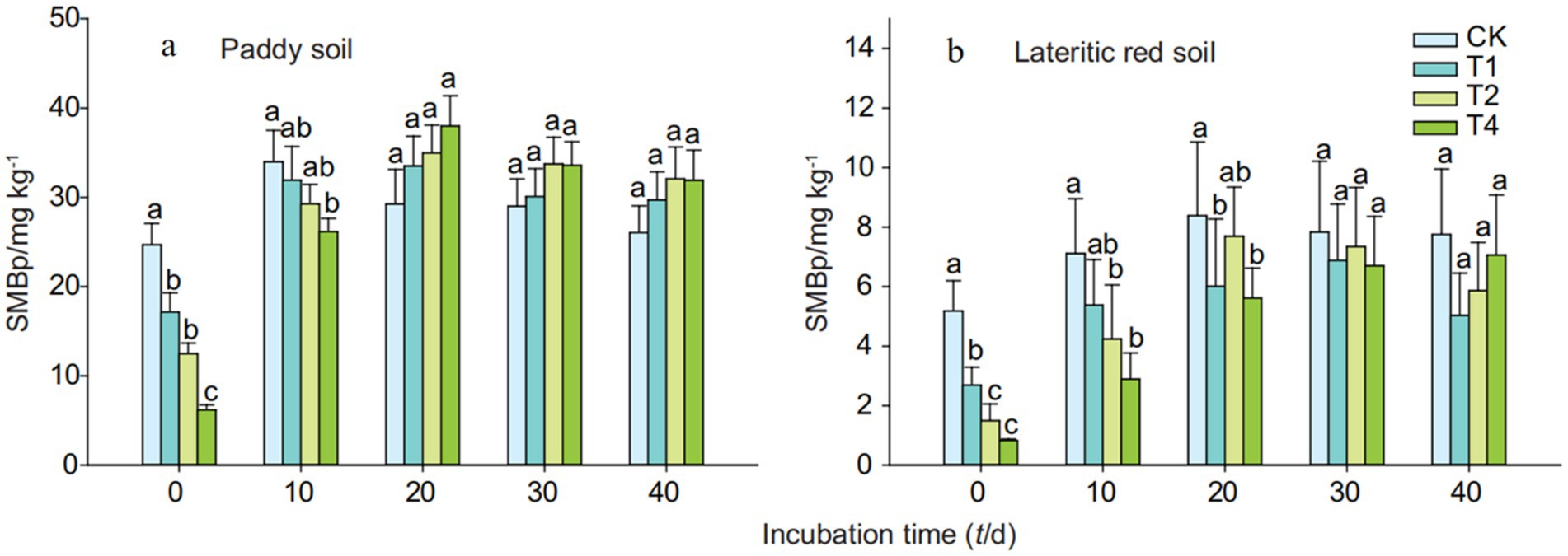
Figure 7. Soil microbial biomass phosphorus (MBP) changes with biochar addition. CK, T1, T2, and T4 represent biochar addition levels of 0%, 1%, 2%, and 4%, respectively. Part labels (a, b) correspond to time periods of the experiment. Error bars represent standard deviation (n = 3). Different letters signify statistically significant differences (p < 0.05) within the same time period.
3.4 Correlation analysis of different indices in the soil
The correlation analysis of various indices in the soil revealed significant relationships between several parameters (Table 2). In the paddy soil, available phosphorus, Al-P (Aluminum-bound Phosphorus), and Fe-P (Iron-bound Phosphorus) showed extremely significant positive correlations with soil pH. Water-soluble phosphorus, O-P (Organic Phosphorus), and Ca-P (Calcium-bound Phosphorus) also exhibited significant positive correlations with soil pH. Conversely, the activities of acid phosphatase (ACP) and alkaline phosphatase (AKP) in the soil were negatively correlated with soil pH, albeit with weaker correlation coefficients. Moreover, the activity of acid phosphatase was negatively correlated with the content of available phosphorus, water-soluble phosphorus, Al-P, Fe-P, and Ca-P in the soil, with strong correlations observed between acid phosphatase and both available phosphorus and Ca-P. The activity of alkaline phosphatase was negatively correlated with the content of water-soluble phosphorus, Al-P, Fe-P, and O-P, and a strong correlation was found between alkaline phosphatase activity and O-P content.
In the case of the lateritic red soil, available phosphorus, water-soluble phosphorus, Al-P, and Fe-P all demonstrated extremely significant positive correlations with soil pH (Table 3). O-P and Ca-P also showed significant positive correlations with soil pH. The activities of acid phosphatase and alkaline phosphatase were negatively correlated with soil pH, with correlations being moderate or weak. Furthermore, the activity of acid phosphatase was negatively correlated with the content of available phosphorus, water-soluble phosphorus, Fe-P, and Ca-P in the soil, with a strong correlation observed between acid phosphatase and both available phosphorus and Ca-P. The activity of alkaline phosphatase was negatively correlated with the content of water-soluble phosphorus, Al-P, Fe-P, and O-P, but it showed a moderate positive correlation with the content of available phosphorus.
4 Discussion
This study demonstrates that straw biochar application significantly impacts soil phosphorus dynamics by altering phosphorus fractions, enhancing phosphorus availability, and influencing microbial phosphorus cycling. Specifically, the results show that biochar increases calcium-bound phosphorus (Ca-P), reduces water-soluble phosphorus, and shifts the distribution of inorganic phosphorus fractions, particularly aluminum- and iron-bound phosphorus (Al-P and Fe-P). Additionally, biochar alters phosphatase activity, reducing acid phosphatase while increasing alkaline phosphatase, and influences microbial biomass phosphorus, with varying effects depending on soil type. These findings highlight the potential of straw biochar as a tool for improving phosphorus management in soils and reducing phosphorus loss through leaching.
4.1 Effects of straw biochar on soil phosphorus fractions and availability
This study demonstrates that the application of straw biochar significantly influences the phosphorus dynamics in both paddy and lateritic red soils. The key findings indicate that biochar increases the content of calcium-bound phosphorus (Ca-P), while reducing water-soluble phosphorus, a form that is prone to leaching. This shift in phosphorus fractions is attributed to biochar’s ability to adsorb soluble phosphorus, alter soil chemistry, and enhance microbial and enzymatic activities. These factors collectively promote the transformation of organic phosphorus into plant-available inorganic forms, thereby improving phosphorus retention and availability for plants.
Biochar’s high surface area and porous structure enable it to adsorb soluble phosphorus, reducing its leaching and providing a conducive environment for phosphorus transformation (Raniro et al., 2022). The increase in soil pH due to biochar application reduces the fixation of phosphorus by metal ions such as iron and aluminum, facilitating the mineralization of organic phosphorus (Fang et al., 2021; Nardis et al., 2022). Furthermore, biochar enhances microbial activity by providing a stable carbon source and improving soil aeration, which are critical for the decomposition of organic phosphorus compounds. The alteration in phosphatase activity, with a decrease in acid phosphatase and an increase in alkaline phosphatase, also supports the notion that biochar facilitates the conversion of organic phosphorus into inorganic forms (Uchimiya and Hiradate, 2014). Specifically, the reduction in acid phosphatase activity can be attributed to the enzyme’s sensitivity to pH changes, as higher pH values alter the enzyme’s structure, reducing its catalytic activity. Additionally, the higher pH may reduce the availability of iron ions (Fe3+), which are essential for the catalytic activity of acid phosphatase (Peng et al., 2021). Conversely, the increase in alkaline phosphatase activity following biochar application can be explained by the enzyme’s preference for alkaline conditions, which are enhanced by the biochar-induced pH rise. Moreover, biochar may supply essential mineral elements such as magnesium and zinc, which act as cofactors for alkaline phosphatase, thereby promoting its activity. These elements are crucial for the enzyme’s function, and their provision by biochar can stimulate the conversion of organic phosphorus into inorganic forms, increasing phosphorus availability for plant uptake.
4.2 Effects of straw biochar on soil pH and acid phosphatase activity
The application of straw biochar significantly raises soil pH, which is particularly significant in acidic soils. This change in pH has a direct effect on soil enzyme activities, as most enzymes function optimally within a specific pH range. The decrease in acid phosphatase activity observed with increasing biochar application can be attributed to the enzyme’s sensitivity to pH changes. Acid phosphatase is more active under acidic conditions, and the elevation of soil pH due to biochar can alter the enzyme’s structure, reducing its catalytic activity (Zhu et al., 2018). Additionally, the higher pH may reduce the availability of iron ions (Fe3+), which are essential for the catalytic activity of acid phosphatase. This reduction in iron ions can further inhibit the enzyme’s function, leading to a decrease in the mineralization of organic phosphorus. Conversely, the increase in alkaline phosphatase activity following biochar application can be explained by the enzyme’s preference for alkaline conditions, which are enhanced by the biochar-induced pH rise. Moreover, biochar may supply essential mineral elements such as magnesium and zinc, which act as cofactors for alkaline phosphatase, thereby promoting its activity. These elements are crucial for the enzyme’s function, and their provision by biochar can stimulate the conversion of organic phosphorus into inorganic forms, increasing phosphorus availability for plant uptake (Yang and Lu, 2022). The observed changes in phosphatase activities have significant implications for phosphorus cycling in agricultural systems. The reduction in acid phosphatase activity and the increase in alkaline phosphatase activity suggest that biochar application can shift the balance of phosphorus forms in the soil from organic to inorganic. This shift can enhance the availability of phosphorus for plant uptake, reducing the need for chemical fertilizers and minimizing the risk of phosphorus leaching and eutrophication. By improving phosphorus use efficiency, biochar can contribute to more sustainable agricultural practices, particularly in regions with acidic soils where phosphorus availability is a limiting factor.
4.3 Effects of straw biochar on soil microbial biomass phosphorus
Soil microorganisms play a pivotal role in the phosphorus cycle, and microbial biomass phosphorus is a key active form of phosphorus in soil. This study found that straw biochar had a significant impact on microbial biomass phosphorus, but the effects varied between soil types. In paddy soil, microbial biomass phosphorus increased following biochar application, whereas in lateritic red soil, no significant changes were observed.
The increased microbial biomass phosphorus in paddy soil can be attributed to biochar’s improvement of soil aeration and its ability to provide a more favorable environment for microbial activity. Biochar has been shown to enhance soil structure, improve aeration, and provide a stable carbon source for soil microbes, all of which can increase microbial phosphorus assimilation (Panahi et al., 2020). However, in lateritic red soil, the lack of a significant response in microbial biomass phosphorus suggests that biochar’s effectiveness in stimulating microbial activity may be limited by nutrient availability, particularly nitrogen, which is often a limiting factor in these soils (Wang et al., 2021). The high carbon-to-nitrogen ratio of straw biochar may exacerbate nitrogen limitation, reducing the ability of microbes to incorporate phosphorus into their biomass.
These findings underscore the importance of soil type and nutrient conditions when evaluating the effectiveness of biochar for enhancing microbial phosphorus cycling. The lack of significant changes in microbial biomass phosphorus in lateritic red soil further emphasizes the need for tailored biochar applications, particularly in soils where nitrogen is a limiting factor or where biochar’s carbon availability is low. Overall, the results highlight biochar’s potential to influence microbial phosphorus cycling, but also point to the need for further research into how biochar interacts with soil microbial communities across different soil types.
5 Conclusion
This study demonstrates that straw biochar application significantly improves phosphorus management in both paddy and lateritic red soils. Specifically, the addition of biochar increased calcium-bound phosphorus (Ca-P) by up to 30% in both soils, while reducing water-soluble phosphorus by 25%, highlighting biochar’s potential to reduce phosphorus leaching and improve phosphorus retention. In lateritic red soil, biochar increased available phosphorus by 20%, more than in paddy soil, suggesting that biochar is particularly effective in soils prone to phosphorus loss due to leaching. Additionally, biochar enhanced phosphatase activity, with alkaline phosphatase activity increasing by 18% compared to controls, further improving phosphorus availability through microbial processes. However, no significant changes in microbial biomass phosphorus were observed in lateritic red soil, indicating that the effects of biochar on microbial phosphorus cycling may be more pronounced in soils with higher organic matter and microbial activity, such as paddy soils. These findings suggest that straw biochar can be a valuable tool in improving phosphorus use efficiency, especially in soils prone to phosphorus loss, thereby reducing the need for chemical fertilizers and minimizing environmental impacts such as eutrophication. Biochar’s role in enhancing phosphorus retention and availability, coupled with its positive effects on phosphatase activity, offers significant potential for improving soil fertility and agricultural sustainability. By reducing reliance on phosphorus fertilizers, biochar could help mitigate the environmental concerns associated with phosphorus accumulation and runoff.
Data availability statement
The original contributions presented in the study are included in the article/supplementary material, further inquiries can be directed to the corresponding author.
Author contributions
WH: Conceptualization, Data curation, Formal analysis, Funding acquisition, Writing – original draft, Writing – review & editing. JZ: Writing – review & editing. WG: Conceptualization, Data curation, Formal analysis, Funding acquisition, Investigation, Methodology, Project administration, Resources, Software, Supervision, Validation, Visualization, Writing – original draft, Writing – review & editing. YC: Software, Supervision, Validation, Visualization, Writing – original draft, Writing – review & editing. ZW: Conceptualization, Data curation, Formal analysis, Funding acquisition, Methodology, Resources, Software, Validation, Writing – original draft, Writing – review & editing.
Funding
The author(s) declare that financial support was received for the research, authorship, and/or publication of this article. This manuscript was granted by the Technology Innovation Center for Land Engineering and Human Settlements, Shaanxi Land Engineering Construction Group Co., Ltd. and Xi’an Jiaotong University (Grant No. 2024WHZ0234), 2023 Annual Shaanxi Province State Capital Operation Budget Science and Technology Innovation Special Fund Project “Guanzhong Area Green Farmland Construction Key Technology Research and Demonstration Promotion Project” and the scientific research project of Shaanxi Provincial Land Engineering Construction Group Co., Ltd. (Grant No. DJNY2022-49).
Conflict of interest
WH, JZ, WG, YC, and ZW were employed by Shaanxi Dijian Guantian Investment and Construction Co., Ltd. and Shaanxi Provincial Land Engineering Construction Group Co., Ltd.
Generative AI statement
The authors declare that no Gen AI was used in the creation of this manuscript.
Publisher’s note
All claims expressed in this article are solely those of the authors and do not necessarily represent those of their affiliated organizations, or those of the publisher, the editors and the reviewers. Any product that may be evaluated in this article, or claim that may be made by its manufacturer, is not guaranteed or endorsed by the publisher.
References
Alengebawy, A., Ran, Y., Ghimire, N., Osman, A. I., and Ai, P. (2023). Rice straw for energy and value-added products in China: a review. Environ. Chem. Lett. 21, 2729–2760. doi: 10.1007/s10311-023-01612-3
Alsajri, F. A., Wijewardana, C., Bheemanahalli, R., Irby, J. T., Krutz, J., Golden, B., et al. (2022). Morpho-physiological, yield, and transgenerational seed germination responses of soybean to temperature. Front. Plant Sci. 13:839270. doi: 10.3389/fpls.2022.839270
Dai, J., Xie, L., and Chu, Z. (2021). Developing sustainable supply chain management: the interplay of institutional pressures and sustainability capabilities. Sustain. Product. Consumpt. 28, 254–268. doi: 10.1016/j.spc.2021.04.017
Fan, Y., Lu, S., He, M., Yang, L., Hu, W., Yang, Z., et al. (2021). Long-term throughfall exclusion decreases soil organic phosphorus associated with reduced plant roots and soil microbial biomass in a subtropical forest. Geoderma 404:115309. doi: 10.1016/j.geoderma.2021.115309
Fang, Z., Liu, F., Li, Y., Li, B., Yang, T., and Li, R. (2021). Influence of microwave-assisted pyrolysis parameters and additives on phosphorus speciation and transformation in phosphorus-enriched biochar derived from municipal sewage sludge. J. Clean. Prod. 287:125550. doi: 10.1016/j.jclepro.2020.125550
Ghodszad, L., Reyhanitabar, A., Oustan, S., and Alidokht, L. (2022). Phosphorus sorption and desorption characteristics of soils as affected by biochar. Soil Tillage Res. 216:105251. doi: 10.1016/j.still.2021.105251
Hu, W., Zhang, Y., Xiangmin, R., Fei, J., Peng, J., and Luo, G. (2023). Coupling amendment of biochar and organic fertilizers increases maize yield and phosphorus uptake by regulating soil phosphatase activity and phosphorus-acquiring microbiota. Agric. Ecosyst. Environ. 355:108582. doi: 10.1016/j.agee.2023.108582
Ingrao, C., Matarazzo, A., Gorjian, S., Adamczyk, J., Failla, S., Primerano, P., et al. (2021). Wheat-straw derived bioethanol production: a review of life cycle assessments. Sci. Total Environ. 781:146751. doi: 10.1016/j.scitotenv.2021.146751
Ji, Y., Ma, S., Lv, S., Wang, Y., Lü, S., and Liu, M. (2021). Nanomaterials for targeted delivery of agrochemicals by an all-in-one combination strategy and deep learning. ACS Appl. Mater. Interfaces 13, 43374–43386. doi: 10.1021/acsami.1c11914
Kamali, M., Sweygers, N., Al-Salem, S., Appels, L., Aminabhavi, T. M., and Dewil, R. (2022). Biochar for soil applications-sustainability aspects, challenges and future prospects. Chem. Eng. J. 428:131189. doi: 10.1016/j.cej.2021.131189
Ma, J., He, P., Xu, X., He, W., Liu, Y., Yang, F., et al. (2016). Temporal and spatial changes in soil available phosphorus in China (1990–2012). Field Crop Res. 192, 13–20. doi: 10.1016/j.fcr.2016.04.006
Murphy, J., and Riley, J. P. (1962). A modified single solution method for the determination of phosphate in natural waters. Anal. Chim. Acta 27, 31–36. doi: 10.1016/S0003-2670(00)88444-5
Nardis, B. O., Franca, J. R., Carneiro, J. S., Da, S., Soares, J. R., Guilherme, L. R. G., et al. (2022). Production of engineered-biochar under different pyrolysis conditions for phosphorus removal from aqueous solution. Sci. Total Environ. 816:151559. doi: 10.1016/j.scitotenv.2021.151559
Panahi, H. K. S., Dehhaghi, M., Ok, Y. S., Nizami, A.-S., Khoshnevisan, B., Mussatto, S. I., et al. (2020). A comprehensive review of engineered biochar: production, characteristics, and environmental applications. J. Clean. Prod. 270:122462. doi: 10.1016/j.jclepro.2020.122462
Peng, Y., Sun, Y., Fan, B., Zhang, S., Bolan, N. S., Chen, Q., et al. (2021). Fe/Al (hydr)oxides engineered biochar for reducing phosphorus leaching from a fertile calcareous soil. J. Clean. Prod. 279:123877. doi: 10.1016/j.jclepro.2020.123877
Rai, N., Rai, S. P., and Sarma, B. K. (2021). Prospects for abiotic stress tolerance in crops utilizing Phyto- and bio-stimulants. Front. Sustain. Food Syst. 5:754853. doi: 10.3389/fsufs.2021.754853
Raniro, H. R., Bettoni Teles, A. P., Adam, C., and Pavinato, P. S. (2022). Phosphorus solubility and dynamics in a tropical soil under sources derived from wastewater and sewage sludge. J. Environ. Manag. 302:113984. doi: 10.1016/j.jenvman.2021.113984
Ren, R., Zhang, H., Gao, X., Wang, H., Jiang, W., Wang, Y., et al. (2024). Capturing spatiotemporal heterogeneity in fertilizer application for better modelling paddy water nitrogen and phosphorus pollution at regional scale. Agric. Ecosyst. Environ. 362:108837. doi: 10.1016/j.agee.2023.108837
Tian, J., Kuang, X., Tang, M., Chen, X., Huang, F., Cai, Y., et al. (2021). Biochar application under low phosphorus input promotes soil organic phosphorus mineralization by shifting bacterial phoD gene community composition. Sci. Total Environ. 779:146556. doi: 10.1016/j.scitotenv.2021.146556
Uchimiya, M., and Hiradate, S. (2014). Pyrolysis temperature-dependent changes in dissolved phosphorus speciation of plant and manure biochars. J. Agric. Food Chem. 62, 1802–1809. doi: 10.1021/jf4053385
Vassileva, M., Vassilev, N., and Azcon, R. (1997). Rock phosphate solubilization by Aspergillus niger on olive cake-based medium and its further application in a soil–plant system. World J. Microbiol. Biotechnol. 14, 281–284. doi: 10.1023/A:1008858802855
Wang, H., Ren, T., Müller, K., Van Zwieten, L., Wang, H., Feng, H., et al. (2021). Soil type regulates carbon and nitrogen stoichiometry and mineralization following biochar or nitrogen addition. Sci. Total Environ. 753:141645. doi: 10.1016/j.scitotenv.2020.141645
Waqas, M. A., Kaya, C., Riaz, A., Farooq, M., Nawaz, I., Wilkes, A., et al. (2019). Potential mechanisms of abiotic stress tolerance in crop plants induced by Thiourea. Front. Plant Sci. 10:1336. doi: 10.3389/fpls.2019.01336
Xie, Y., Bu, H., Feng, Q., Wassie, M., Amee, M., Jiang, Y., et al. (2021). Identification of cd-resistant microorganisms from heavy metal-contaminated soil and its potential in promoting the growth and cd accumulation of bermudagrass. Environ. Res. 200:111730. doi: 10.1016/j.envres.2021.111730
Yang, C., and Lu, S. (2022). Straw and straw biochar differently affect phosphorus availability, enzyme activity and microbial functional genes in an Ultisol. Sci. Total Environ. 805:150325. doi: 10.1016/j.scitotenv.2021.150325
Yang, S., Shi, Z., Zhang, M., Li, Y., Gao, J., Wang, X., et al. (2021). Stoichiometry of carbon, nitrogen and phosphorus in shrub organs linked closely with Mycorrhizal strategy in northern China. Front. Plant Sci. 12:687347. doi: 10.3389/fpls.2021.687347
Zhang, Y., Yan, J., Rong, X., Han, Y., Yang, Z., Hou, K., et al. (2021). Responses of maize yield, nitrogen and phosphorus runoff losses and soil properties to biochar and organic fertilizer application in a light-loamy fluvo-aquic soil. Agric. Ecosyst. Environ. 314:107433. doi: 10.1016/j.agee.2021.107433
Zhang, L., Zhuang, T., Bai, J., Ye, X., Wang, D., Wang, W., et al. (2021). Dynamics of phosphorus fractions and potential bioavailability along soil profiles from seasonal-flooding wetlands in a Chinese estuary. Environ. Sci. Pollut. Res. 28, 6549–6560. doi: 10.1007/s11356-020-10732-0
Zheng, Y., Zimmerman, A. R., and Gao, B. (2020). Comparative investigation of characteristics and phosphate removal by engineered biochars with different loadings of magnesium, aluminum, or iron. Sci. Total Environ. 747:141277. doi: 10.1016/j.scitotenv.2020.141277
Zhu, J., Li, M., and Whelan, M. (2018). Phosphorus activators contribute to legacy phosphorus availability in agricultural soils: a review. Sci. Total Environ. 612, 522–537. doi: 10.1016/j.scitotenv.2017.08.095
Keywords: straw biochar, available phosphorus, inorganic phosphorus fraction, phosphatase, microbial biomass phosphorus
Citation: He W, Zhang J, Gao W, Chen Y and Wei Z (2025) Enhancing phosphorus availability and dynamics in acidic soils through Rice straw biochar application: a sustainable alternative to chemical fertilizers. Front. Sustain. Food Syst. 9:1506609. doi: 10.3389/fsufs.2025.1506609
Edited by:
Mohamed Trigui, Institut Préparatoire aux Etudes d’Ingénieur de Sfax (IPEIS), TunisiaReviewed by:
Lina Trabelsi, University of Sfax, TunisiaNibras Belgaroui, Nicolaus Copernicus University, Poland
Copyright © 2025 He, Zhang, Gao, Chen and Wei. This is an open-access article distributed under the terms of the Creative Commons Attribution License (CC BY). The use, distribution or reproduction in other forums is permitted, provided the original author(s) and the copyright owner(s) are credited and that the original publication in this journal is cited, in accordance with accepted academic practice. No use, distribution or reproduction is permitted which does not comply with these terms.
*Correspondence: Wei He, MTgxNzA3NDI4QHFxLmNt