- 1Department of Soil and Water, Agronomy College University of the Republic, Montevideo, Uruguay
- 2Department of Crop Production, Agronomy College, University of the Republic, Montevideo, Uruguay
- 3Department of Biometrics, Statistics and Computing, Agronomy College University of the Republic, Montevideo, Uruguay
Introduction: Uruguayan agriculture’s transition to no-till farming and intensified practices, replacing crop-pasture (CP) systems with continuous cropping (CC) rotations, has disrupted biological nitrogen fixation (BNF). Despite this, diversified cropping sequences, including C4 species, have maintained the soil organic carbon (SOC) balance under no-till management, with limited overall impacts on productivity and sustainability. The effects of these changes on wheat productivity and nitrogen use efficiency (NUE) need to be further investigated.
Methods: This study, conducted within a long-term experiment (LTE) under rainfed conditions, compared wheat productivity in CP and CC rotations. Wheat following CP and CC were analyzed over three seasons. Variables measured included soil nitrogen (N) concentration, wheat grain yield (WGY), grain protein concentration (GPC), and NUE. Four N fertilizer levels were applied to each rotation system to assess their impact.
Results: CC rotation consistently outperformed CP in WGY, with 2425 and 1668 kg ha−1 averages, respectively. CP showed slightly higher GPC (10.92%) than CC (10.48%). Nitrate-N levels at tillering positively correlated with WGY and negatively with GPC, but the relationship differed by rotation. Soil NUE indices were higher in CC rotations.
Discussion: The study’s findings highlighted the potential of CC rotation, especially when including C4 species in the crop sequence, to achieve higher wheat productivity in the short term due to healthier soil conditions compared to wheat seeded after post-pasture in CP. Additionally, our study highlights that the effect of the previous crop on yield and NUE in wheat was more relevant than the expected residual effect of the pasture phase in CP, primarily due to the quality of residues and the temporary adverse effects of soil compaction caused by livestock trampling.
1 Introduction
Since the beginning of the 21st century, Uruguay has embraced no-till farming and intensified its agricultural practices, increasing reliance on N-based fertilizers (Ernst et al., 2018; Ernst et al., 2020; Fassana et al., 2022). This intensification, coupled with a shift from crop-pasture (CP) systems to continuous cropping (CC) rotations, primarily soybean (Glycine max L. Merr.), has reduced or eliminated pastures, disrupting the biological nitrogen fixation (BNF) provided by perennial legumes in mixed sward pastures (Lussich Rachetti, 2020). Consequently, this has led to negative N (Quemada and Lassaletta, 2024) and carbon (C) balance compared to previous management practices (Rubio et al., 2021a). The increased use of fertilizers has also heightened the risk of N losses through soil erosion and leaching (Liu et al., 2023). Although this system produces more grain, gaps still exist between the potential and actual wheat yields, which cannot solely be attributed to nutrient deficiencies (Ernst et al., 2018; Hochman and Horan, 2018; Hatfield and Beres, 2019).
There is a growing consensus that crop production intensification should be approached from an ecosystem perspective (Duru et al., 2015; Cassman and Grassini, 2020; Dang et al., 2020). Sustainable intensification, which aims to boost yields from the same land area while reducing environmental impacts, enhancing natural resources, and providing ecosystem services, is increasingly embraced (FAO, 2011). Conservation agriculture, which can support sustainable farming practices, may preserve or enhance soil health by reducing soil disturbance through minimal mechanical tillage, such as no-till. Furthermore, this approach includes two other core crop management principles: implementing crop rotation with a variety of plant species (both annuals and perennials, C3 and C4 species) and maintaining soil cover by retaining residues or using cover crops (FAO, 2011; Griffiths et al., 2022). No-till farming has shown promising effects in specific contexts, such as rainfed agroecosystems in dry climates. However, yield benefits are only realized when combined with the other two conservation agriculture principles (Pittelkow et al., 2015a; Page et al., 2020). When implemented alone, no-till can increase the risk of yield loss for farmers (Pittelkow et al., 2015b; Page et al., 2020). For this reason, the current agricultural system urgently needs a shift toward more sustainable practices, in terms of environmental impact and productivity outcomes, as emphasized by the ecosystem-based approach (FAO, 2011). In this context, it is reassuring to know that pasture or perennial crops play a significant role in recovering lost functional properties and improving intrinsic soil qualities such as water infiltration, nutrient cycling, and biological diversity (Teague and Kreuter, 2020; Mosier et al., 2021; Rubio et al., 2021b). Long-term experiments in Uruguay, Brazil, Argentina, and the US have indicated that integrated crop-livestock systems based on perennial pastures can sustain crop productivity and climate resilience over the long haul while preserving or increasing soil C storage (Franzluebbers, 2013; Pravia et al., 2019). In Uruguay, incorporating pastures helps maintain soil quality and significantly boosts productivity (Grahmann et al., 2020; Rubio et al., 2021b). For example, CP systems resulted in 19% higher SOC and 14% higher total N levels than CC systems, with wheat yields averaging 1 Mg ha−1 higher in CP systems (Grahmann et al., 2020). In the second study, Rubio et al. (2021a) examined the effects of various long-term cropping systems on maize yield response to soil decompaction through deep tillage and different N fertilization rates (Rubio et al., 2021b). Both short-term remediation strategies failed to mitigate the adverse impacts of soil degradation by CC on corn growth. Nevertheless, the yield of maize grown after mixed pastures (grasses and legumes) failed to accurately represent the overall beneficial impact of CP on soil quality, implying that additional short-term issues related to crop rotation, such as the preceding crop, should be considered (Lollato et al., 2019a; Arnhold et al., 2023). These studies have also shown that pasture significantly helps maintain SOC and N content; however, not all rotation systems converge on improved soil productivity. The no response in some systems or crops aligns from several works (Franzluebbers and Stuedemann, 2014; Pittelkow et al., 2015a; Lollato et al., 2019a; Dang et al., 2020), asserting that the beneficial impact of no-till management, compared to the conventional tillage, was more pronounced in summer crops than in winter ones and in CC compared to CP rotations (Ernst et al., 2020). These findings suggest that no-till offered a clear advantage for summer crops within CC systems, with a minor influence on winter crop yields and a small positive impact in integrated crop-livestock systems (Dang et al., 2020). Experiments by Salvo et al. (2010) comparing five cropping systems in Uruguay indicated that integrating pastures into rotation did not modify SOC content and its fractions under the no-till system.
Ernst and coworkers also noted this phenomenon in the same LTE, attributing it to animals consuming pasture biomass, accounting for 84% of the pasture’s dry matter that would otherwise have covered the soil (Ernst et al., 2020). Additionally, annual crops in CC incorporating C4 species rotation under no-till produced more significant crop residues, closely related to C input and soil C sequestration under reduced tillage conditions (Pravia et al., 2019; Baethgen et al., 2021). These adjusted no-till systems that include C4 crops may require increased N inputs, their potential environmental benefits are significant, providing a promising outlook for sustainable farming practices.
Conversely, in Uruguay, no study has compared wheat performance under no-till conditions with equal intensification of the annual cropping phase between wheat seeded after pasture termination (CP rotation) and wheat seeded in continuous annual cropping (CC rotation). The benefits of rotating the annual cropping phase with intensively grazed management in the pasture phase under no-till can be weakened by soil compaction induced by animal trampling, reducing water infiltration, root exploration, and nutrient uptake (Colombi and Keller, 2019; Dang et al., 2020; Shaheb et al., 2021; Stanley et al., 2024). However, livestock treading damage, has been reported to have little influence on subsequent crop yields, as the negative impacts are usually limited to shallow depths (less than 0.15 m) and may only persist temporarily, being mitigated by subsequent natural soil processes like wetting/drying cycles or plant root activity (Bell et al., 2011; Stanley et al., 2024). Soil compaction from non-pugged grazing and its recovery follows a cyclical pattern based on earlier studies (Drewry et al., 2008). These studies measured soil compaction in spring and its natural recovery during summer and autumn on soil grazed by dairy cows. The results indicated significant recovery of soil physical properties (macroporosity) in summer and autumn, with less recovery observed in winter. Enhanced recovery of soil physical condition in summer and autumn in temperate environments may be due to the increased natural soil processes mentioned above.
In summary, no-till farming is a practice that has drawn interest for its potential to significantly enhance crop performance and soil health (Baethgen et al., 2021; Romano et al., 2023; Taylor et al., 2024), holding great benefits for the future of agriculture. There are reported contradictory findings between CC and CP under no-till management regarding crop yields and NUE (Baiyeri et al., 2019; Pravia et al., 2019; Dang et al., 2020; Peterson et al., 2020). When pasture rotation enhances soil structure, organic matter content, and microbial activity, wheat might access and utilize better N in CP systems; conversely, agricultural systems with continuous nutrient depletion and lack of crop diversity lead to soil degradation over time and, while easier to manage, may also have lower N efficiency (Hu et al., 2023). Therefore, the incorporation of pasture or cover crops into crop rotations while using no-till techniques presents an optimistic and practical way to maintain wheat output and improve N utilization (Habbib et al., 2017; Dang et al., 2020; Dong and Zeng, 2024; Yin et al., 2024).
In Uruguay, it is well established that crop-pasture rotation systems consistently enhance crop production. Moreover, most LTE conducted under no-till included grazing (García-Préchac et al., 2004; Salvo et al., 2010; Grahmann et al., 2020; Rovira et al., 2020; Baethgen et al., 2021; Rubio et al., 2025). Therefore, the possible adverse effects of grazing have already been considered within the system as a whole. We hypothesized that including pastures in crop rotation would have further beneficial effects on wheat yields (as the first crop post-pasture) due to improved soil quality and greater availability of soil N, despite potential adverse effects such as soil compaction or invasion of weeds caused by cattle trampling and grazing. Then, the novelty of our study lies in evaluating the wheat performance corresponding to the first crop post-pasture compared with a wheat crop in a CC rotation with the equal intensification of the annual cropping phase to CP in a rainfed environment. The study assessed WGY, GPC, and the NUE as affected by the N response trials in wheat seeded under no-till in CP and CC systems in the same year. This allowed us to infer consistencies or dissimilarities between the rotation systems.
2 Materials and methods
2.1 Experimental site
The study was conducted in a LTE established in 1993 at the EEMAC Experimental Station near Paysandú, Uruguay (32° 22′ 41” South latitude and 58° 02′ 50” West longitude). The site is under the influence of a humid subtropical climate (according to the Köppen climate classification), and it is relatively uniform nationwide since Uruguay is located entirely within the temperate zone. The average annual accumulated rainfall is 1,300 mm, and the average temperature in the winter and the summer are 12°C and 24°C, respectively. The soil of the experimental area is classified as Typic Argiudoll, according to the USDA Soil Taxonomy, with an A horizon of 18 cm with pH 5.7, clay 290 g kg−1, silt 437 g kg−1 and sand 273 g kg−1, located on a slope less than 1%. The soil organic carbon (SOC) and total N at 0 to 15 cm depth were 18.7 g kg−1 and 1.6 g kg−1, respectively. The LTE, initiated in 1994, compared four cropping systems under no-till and conventional tillage conditions: This study evaluated just two no-till cropping systems, CC (i) and CP (ii), because conventional tillage has almost completely disappeared as a tillage system in Uruguay (Table 1). The cropping systems were arranged in non-synchronized randomized replications to ensure the presence of all crop or pasture phases each year, with three replications for CC, and 7 for CP.
The CP is a 7-year rotation alternating between crop and pasture phases. Pasture yields in the spring, ranged from 1,000 to 3,500 kg ha−1, depending on the age of the pasture and the proportion of legumes and weeds. Glyphosate herbicide was applied in plots with 3.5-year pastures two months before wheat seeding. On average, the pasture provided 65 kg N ha−1 annually, with approximately 90% derived from BNF (data not shown). The grazing criteria were as follows: grazing began when pastures reached 2,500 kg of dry matter per hectare, especially in two-year-old pastures. Grazing was also carried out on older pastures (3rd and 4th year), even though their production would have been lower. Grazing ceased when the forage consumption was around 50%, averaging 5 to 7 annual grazing events, and halted if the soil was too wet to avoid animal footprints. The animals withdrew if it rained during grazing.
The grain crops in CP consisted of a succession of wheat (Triticum aestivum L.) and barley (Hordeum vulgare L.) as winter crops and fallow winter conditions if the previous crop was sorghum (Sorghum bicolor L.) and soybean (Glycine max L.) and sorghum as summer crops. In the CC system, an identical grain crop sequence was considered (Figure 1). The soybean crop was defined as a first (Soybean 1) or second (Soybean 2) crop depending on the previous crop’s purpose, i.e., if it was for grain harvest: Soybean 2, and if it was fallow or a cash crop: Soybean 1. As is displayed in Figure 1, while CC had a frequency of C4 crops (sorghum) of 0.14 (or 1 C4 crop every 3. 5 years, Figure 1), under CP rotation, this frequency was 0.07 (or 1 C4 crop every seven years).
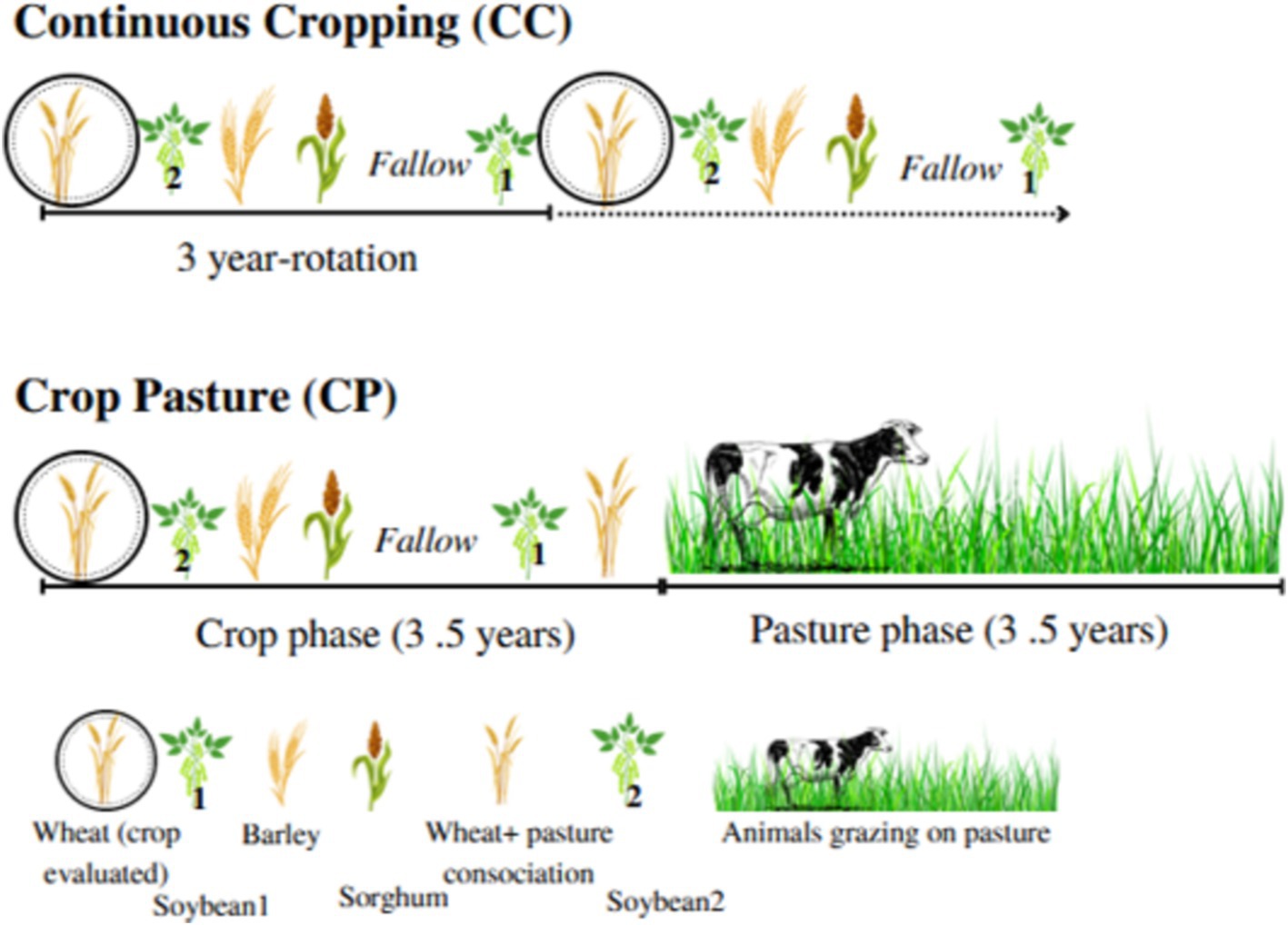
Figure 1. Schematic representation of the treatments: wheat-based rotations evaluated and sequence length for each phase.
2.2 Experimental design and treatments management
In this study, we evaluated wheat performance after 20 years (1994–2014) of two contrasting cropping systems under no-till: CP and CC. In the CP system, wheat is the first annual crop after the long perennial pasture phase, making it a reliable indicator for assessing the rotation’s carryover effects on such a system. The wheat crop under CP was seeded after 3.5 years of pasture, initially composed of a mix of fescues but dominated by Cynodon dactylon at the termination date, particularly in 2015. Under the CC system, where there is no pasture phase, wheat was seeded following the Soybean 1. The wheat cultivar used was Baguette 501, and the crop was sown at the recommended density on June 27th, May 29th, and May 26th in 2014, 2015, and 2016, respectively.
A completely randomized design trial with three replications was conducted exclusively on the wheat seeded within two plots/year of 10 × 50 m size in 2014, 2015, and 2016, each representing a rotation-tillage combination (CP or CC), as shown in Figure 1. The N response was assessed within each plot, setting four fixed and equidistant N levels (0, 30, 60, and 90 kg N ha−1) as urea. The N factor was an essential aspect of our study, as this source of variation allowed us to infer differences between rotations. Each N rate was split into two equal amounts and applied to the wheat crop at the seeding date and when the wheat reached the tillering phenological stage corresponding to Z2.2 of the Zadoks growth scale. This experimental treatment design was conducted in 2015 and 2016, while in 2014, N was applied only during tillering at two N rates (0 and 30 N). Phosphorous was broadcasted without incorporation at 60 kg ha−1 of P2O5 as triple superphosphate at wheat seeding across the entire experimental area to avoid P limitation in crop growth. The topsoil (0–20 cm) had adequate potassium (0.6 cmol+ kg−1) and a cation exchange capacity (CEC) of 14 cmol+ kg−1, so for each experiment, potassium and CEC values were assumed to be non-limiting (Barbazán et al., 2011). The wheat crop was kept free from weeds, pests, insects, and diseases by applying herbicides, insecticides, and fungicides as needed.
2.3 Weather data during the study period (2014–2016)
This research utilized meteorological records from a nearby station at the EEMAC Experimental Station in Paysandú (Latitude: 32° 22′ 41” S Longitude: 58° 03′ 50” W). These records comprised monthly temperature readings (maximum, minimum, and mean) and monthly precipitation data (accumulated per month). On average, the cumulative precipitation for the wheat-growing season, which extends from May to November, was 542 mm. However, in 2014, 2015, and 2016, the cumulative rainfall recorded was 813 mm, 613 mm, and 512 mm, respectively. While the rainfall exceeded the average in 2014, it was typical in 2015 and 2016. Nonetheless, there were instances of soil water saturation, which were most severe from September to November in 2014 and in August of 2015 (Figure 2A).
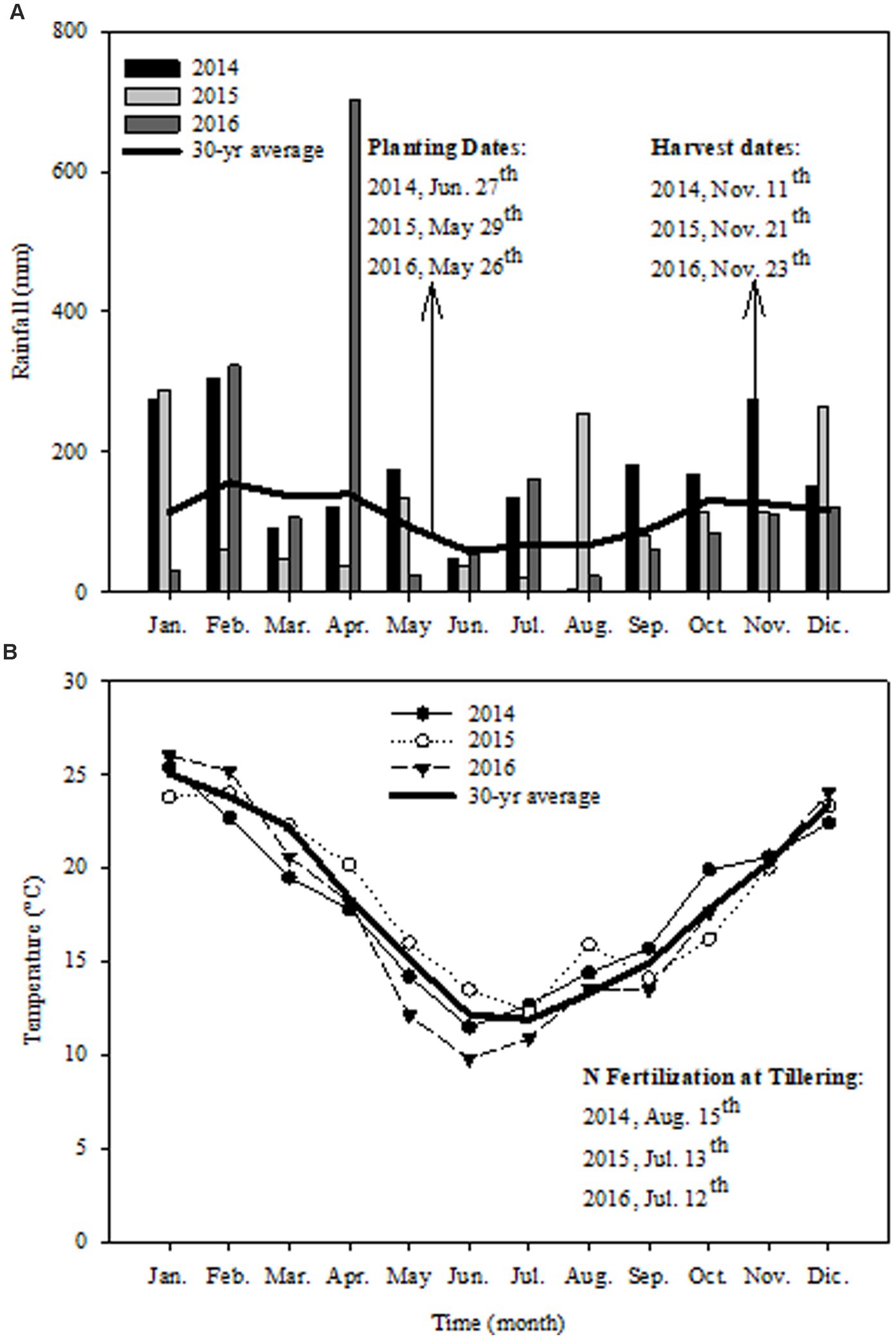
Figure 2. Data on weather from 2014 to 2016 in Paysandú, Uruguay. (A) Monthly rainfall for these years and the 30-year rainfall average. (B) Monthly temperatures and the 30-year temperature average.
In 2014 and 2016, the total rainfall between April and May was significantly higher than the 30-year average, with 298 mm and 726 mm above the average, respectively. However, in 2015, it was 63 mm lower than the 30-year average. In 2016, heavy rainfall occurred 30 days before the wheat seeding, with abundant precipitation over 11 days, ranging from 20 to 188 mm per day. Although the temperature in 2014 was higher than the 30-year average monthly air temperature, and in 2016 was lower, in 2015, it was similar to deviations from the 30-year average, which were relatively small in all three years and thus were unlikely to have a significant impact on crop growth (Figure 2B). The weather throughout the wheat growing season in 2015 was the most similar to the 30-year average. In 2014, it was a rainy and warm spring, while in 2016, the winter was colder than the two previous wheat seasons, and the three months leading up to the crop harvest were dry.
2.4 Soil and plant sampling
During the three-year study, composite soil samples were collected from each plot at 0 to 20 cm depth to assess the N mineral concentration at two stages of the wheat cycle: seeding and tillering. Additionally, one more sampling was done before the seeding of the succeeding soybean crop in December 2015 and 2016. Fifteen subsamples were taken from each plot, using a sharpened stainless-steel probe with a diameter of 2 cm to obtain the samples at the specified times.
At the end of each wheat growing season (November), plants were sampled in a 1 m-row at physiological maturity to assess biomass yield. The plant samples were separated into grain and stover for individual analysis. Each plot was harvested separately, resulting in three yield replicates per system (CP and CC) and per year of study (2014–2016). The grain harvest area was 1.15 × 4.0 m. Threshing was performed using a stationary machine, and the reported grain yield was corrected to a baseline moisture level of 13.5% using a grain moisture meter to measure the grain moisture content (Model: OHAUS MC2000).
2.5 Sample processing and analytical determinations
2.5.1 Soil measurements
Prior to chemical analysis, each soil sample was air-dried and crushed to pass through a 2-mm sieve after removing any visible plant residues. For mineral N, soil extracts were prepared by shaking 10 g of soil with 100 mL of 2 M KCl (Rhine et al., 1998). The concentration of ammonium–N (NH4–N) and nitrate N (NO3–N) in soil samples was analyzed by colorimetric determination. The Griess–Ilosvany method (Mulvaney, 2018) was used to determine NO3–N concentration, while NH4–N concentration was determined using the colorimetric method based on the Berthelot reaction (Rhine et al., 1998). The N-NO3 concentration at 0–20 cm of soil depth is used as a parameter for N diagnostic for Uruguayan wheat crops (Rabuffetti, 2017). Other soil subsamples previously air-dried intended for total N and C determination were oven-dried at 40°C, finely ground (< 200 μm), and analyzed by dry combustion (Rayment and Lyons, 2011) using an elemental analyzer (Flash EA 112) coupled to an isotope ratio mass spectrometer (DeltaPLUS, Finnigan MAT, Bremen, Germany).
2.5.2 Plant measurements
The plant samples were oven-dried at 65°C until the mass remained constant. Finally, soil and dried plant materials were ground in a rotary mill (SampleTek Model 200 Vial Rotator, Lincoln, Nebraska). This step transformed the materials into a fine powder, akin to talcum powder, which was a prerequisite for their analysis by mass spectrometry.
Each plant sample was separated into grain and stover to analyze the dry matter and N content in grain and stover (henceforth Nstover) and grain protein concentration (GPC). The GPC values were estimated based on dry grain N concentration data multiplied by 5.7% (Giunta et al., 2021) and reported as a percentage at 13.5% moisture. The total N (TN) concentration (Dumas method; IAEA, 1990) for the soil and plant samples was determined using an elemental analyzer (Flash EA 112, DeltaPLUS, Finnigan MAT, Bremen, Germany).
Our study employed precise plant measurements to determine four Fertilizer-based N Use Efficiencies (NUEs). The first of these, agronomic efficiency (AE), was estimated from data gathered in three experiments. This estimation was done using the following equation.
Grain yieldF and Grain yieldUF are grain yields of wheat cultivated in plots at a certain level of fertilizer N and in the non-N-fertilized plots, respectively.
Secondly, the RE is the total N amount (grain + stover) difference between crops growing in N-fertilized and non-N-fertilized plots per kg of applied N. This ecophysiological parameter was defined by the Equation (2):
N uptakeF and N uptakeUF are the total N taken up by plants grown in an N-fertilized plot and a control non-N-fertilized plot, respectively, and N applied is the amount of N fertilizer applied.
The IE is the total grain yield produced per unit of N absorbed. This physiological parameter, also named physiological efficiency (PEN), was estimated with the following equation:
The other two indices for assessing NUE in fertilizers were partial factor productivity (PFP) and partial N balance (PNB), neither of which take into account the N supply in the soil, while both fertilizer-based indices mentioned above, RE and AE, consider the background soil N levels by accounting for the N uptake or production in plots that did not receive fertilizer (Mălinaş et al., 2022). The PFP expresses grain yield for each N fertilizer unit used. In contrast, the PNB expresses the grain N uptake for each N fertilizer unit applied.
2.6 Statistical analysis
A segmented model by rotation system allowed for assessing the N response on WGY, GPC, soil mineral concentration, and N efficiency indices. This approach was selected due to the lack of degrees of freedom for the rotation factor in the experimental design, making a direct statistical comparison between rotation systems unfeasible. Nevertheless, inferences could be made from the independent N response trials within each rotation system. Additionally, the response of each system to the applied N, the effect of the year, and the interaction between the N rate and year were considered without restrictions. Replicates were nested within the year in the model. Data from trials conducted in 2015 and 2016 were used, as the experimental design in 2014 was different.
Where:
Yijk = is the response variable in the ijk-th observation.
μ = overall mean.
= is the relative effect of the i-th year effect.
= is the relative effect of the j-th N fertilization effect.
= is the N fertilization by year interaction.
= is the k-th replication nested to the year.
= experimental error.
The approach of the model segmented by rotation was represented as follows:
Model CC = Year + Nrate + Year*Nrate + Replicate (year).
Model CP = Year + Nrate + Year*Nrate + Replicate (year).
A Tukey test with a 95% confidence level was employed to compare treatment means within each rotation in the study. The Shapiro–Wilk and Levene tests checked data normality and homogeneity of variance assumptions. For data analysis, we used SAS ® Studio on Demand for Academics (Cary, NC) and R software (version 4.04). The orthogonal polynomial contrast or comparison analyses tested the response trends of the WGY, GPC, and soil nitrate-N concentration to N applied. To create a predictive model for WGY and GPC, we divided the dataset into two equal subsets for training and validation. We used a linear regression model and SMA regression (package smart in R). Two SMAs (for y against x) were fitted separately for each level of the factor rotation.
3 Results
3.1 Effects of year and N rate segregated by rotation on soil mineral N
Soil NO3–N concentration at the 0–20 cm depth at wheat seeding and before N application was not statistically different between years (2014–2016) in CC, averaging 11.5 mg kg−1, while in CP, it was significantly different (p = 0.0243), being lowest in 2015 (7.0 mg kg−1) and higher and without differences in 2014 and 2016, with 11.6 and 10.2 mg kg−1, respectively (Supplementary Table S1). At tillering (Zadocks 2.2), in 2015 and 2016 and with the four N doses, the effects of year and Nrate on NO3–N concentration were statistically significant (Table 2). The NO3–N increase with the Nrate was quadratic in CC and linear in CP. The NO3–N concentration range varied from 7.2 to 14.6 mg kg−1 in CC and 8.0 to 11.8 mg kg−1 in CP, yielding the lowest values in 0 N and the highest in 60 and 90 N treatments. However, the Year × Nrate interaction effect was not statistically significant in either rotation system.
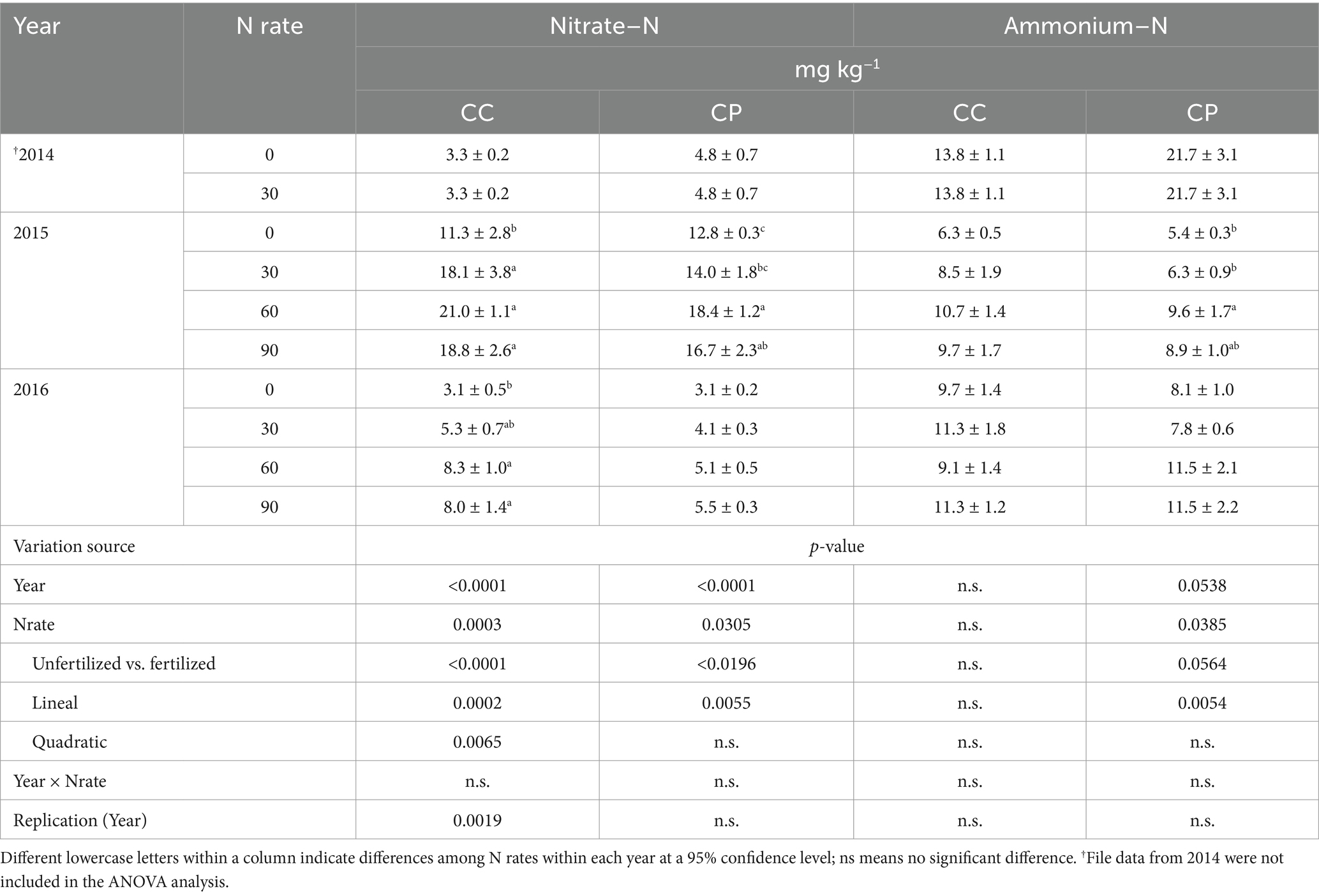
Table 2. Means and standard errors for soil Nitrate–N and Ammonium–N concentration at tillering for wheat crops by experimental year, N rate, and rotation system.
In 2015 and 2016, the N rate had a statistically significant effect on soil NH4–N at tillering in the CP rotation, while in CC, none of the factors tested showed differences in soil NH4–N (Table 2). At the seeding of the soybean crop, the succesor crop after wheat (Supplementary Table S2), both NH4+ and NO3-forms of N were higher in the CP system compared to the CC system. The former was the primary N-form in the soil in both systems but generally had a higher NH4 concentration in CP. Additionally, the year and interaction effects were not significant. In both systems, the NH4–N concentration in all N treatments at tillering was higher than NO3–N concentration in 2014 and 2016. The higher NH4–N to NO3 − N ratio in 2014 and 2016 coincided with the heaviest rainfall during the wheat tillering seasons. On average, NH4–N concentrations were 13.8 and 21.7 mg kg−1 across years in CC and CP, respectively (Table 2).
3.2 Effects of year and N rate segregated by rotation on physical productivity and quality of wheat
3.2.1 Wheat grain yield
Based on the segmented model by rotation system, the ANOVA analysis revealed that the year (2014–2016) effect did not significantly affect WGY in unfertilized plots (0 N). However, considering all N treatments, the ANOVA from 2015 and 2016 data showed that the year and Nrate in both rotations had a statistically significant effect on WGY (Table 3). The lowest yield was observed at 0 N, with 1,520, and 1,080 kg ha−1 in CC and CP, respectively, which statistically differed from the rest of the N treatments. The highest yield was registered at 90 N and 60 N with 3,461 and 2,630 kg ha−1 in CC and CP, respectively.
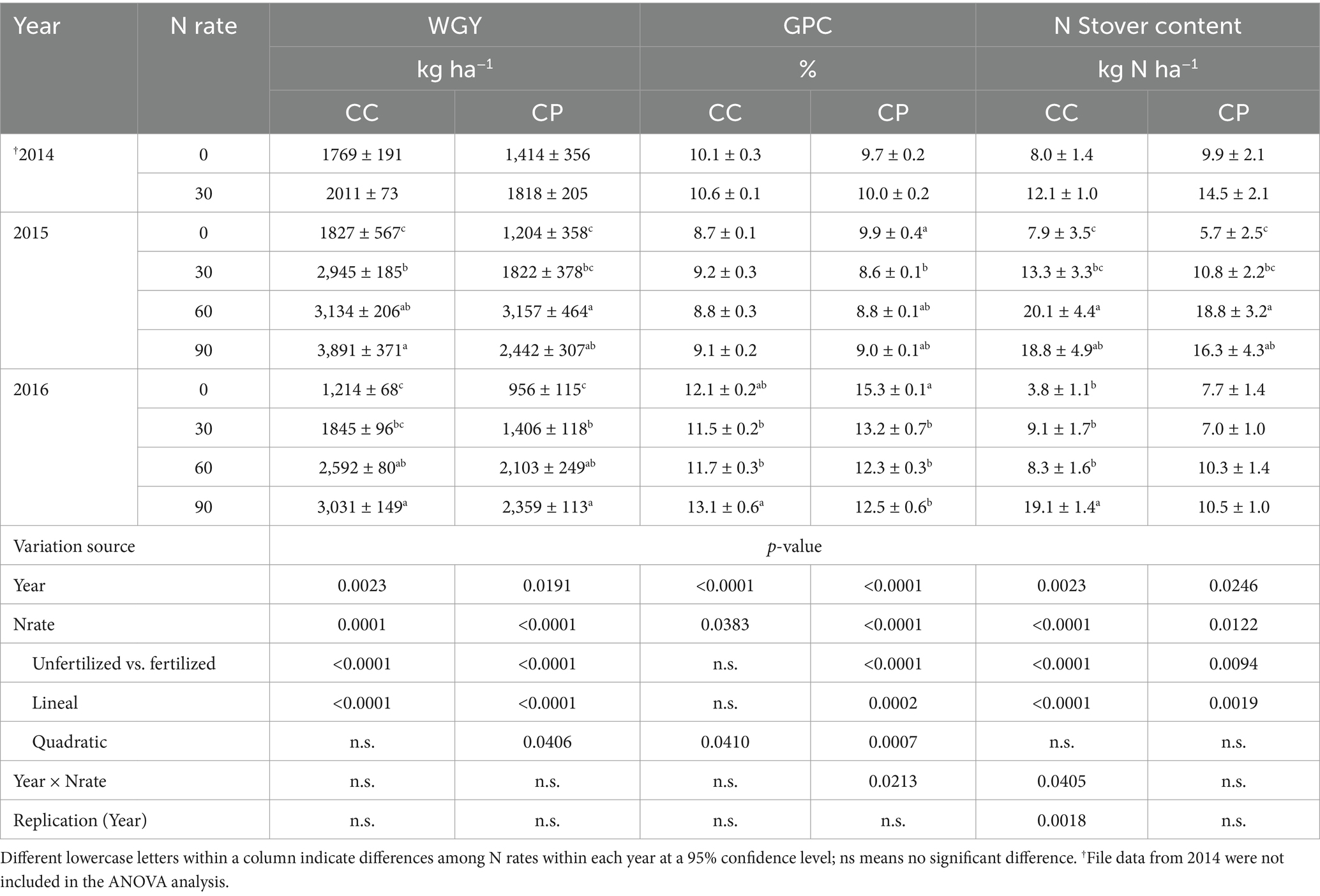
Table 3. Means and standard errors for wheat grain yield (WGY), grain protein concentration (GPC), and N content in the stover for wheat crops by experimental year, N rate, and rotation system: continuous cropping (CC) and crop-pasture (CP).
The highest WGY was consistently observed in the CC system in our three-year study. However, the N response tended to be linear with the N rate in CC and quadratic in CP. As there was no significant interaction effect between Year and Nrate, the yield response to N was similar between years at each rotation system. In 2015, the year of higher productive potential, the yield differences between systems increased, with wheat capitalizing better in CC rotations. In the treatments where no N was applied (0 N), WGYs were higher in 2014 and 2015 than in 2016; still, these differences were not statistically significant.
3.2.2 Grain protein concentration and N Stover content
The GPC measured in wheat cultivated in unfertilized plots was significantly affected by the year in both rotation systems; its average value was11.2% and ranged between 9.7 and 15.39% in CP, while in CC, it was lower on average at 10.4%, oscillating between 8.7 and 12.1%. The ANOVA, including the four N rate treatments from the experiments of 2015 and 2016, showed that year (p = <0.0001 in both rotations) and Nrate (p = 0.0383 and p < 0.0001 in CC and CP, respectively) had a statistically significant effect on GPC (Table 3). In these two years, the maximum values were registered in the treatments 90 N in CC and 0 N in CP, averaging 11.1 and 12.6%, respectively.
The GPC values significantly differed between N rates across years (p = 0.0213) only in CP. The analysis of the year × Nrate interaction effect revealed that in 2016, none of the N fertilized treatments enhanced the GPC compared with wheat crops with 0 N applied, while in 2015, wheat fertilized with the highest N rates (60 and 90 N) were able to reach the GPC achieved by crops non-fertilized. The Nrate effect in CC was consistent across years, showing no N response on GPC values. However, in 2016, the wheat fertilized with the highest dose (90 N) reached the maximum values; still, it did not statistically differ from 0 N.
Similar to the GPC, the N content in the biomass stover significantly differed between Nrate across years (p = 0.0405), but in this case, only in CC. The analysis of this interaction effect revealed that N response on N content in stover differed between years; in 2015, wheat fertilized with high doses of N (60 N and 90 N) reached higher N values in stover than the unfertilized ones, while in 2016, it was only possible with the maximum N rate (90 N). The Nrate effect in CP was consistent across years, showing no N response on Nstover values in 2016; however, in 2015, the wheat fertilized with the medium dose (60 N) reached the maximum values, differing from those treatments with 0 N applied. At this N rate, WGY was also at its highest (Table 3).
3.3 Relationships between nitrate-N at tillering and grain yield and quality segregated by rotation
Higher levels of nitrate-N at tillering were associated with higher WGY and lower GPC, as shown in Figures 3A,B, respectively, for each of the relationships segregated by rotation. Conversely, when considering a similar nitrate−N level, the increase in N rate did not lead to higher GPC levels because there was no association between N rate and GPC (Table 4). However, there was a high and significant negative correlation between NO3–N and GPC, suggesting that the soil mineral N available at tillering was a critical factor affecting GPC (Figure 3B). Grain PC variation was negatively associated with NO3–N measured at tillering; the opposite was the association between NO3–N concentration and WGY. The relationships between those variables resulted in a distinct relationship between GPC and WGY at each system, which was more robust and significant in CP (Figure 3C).
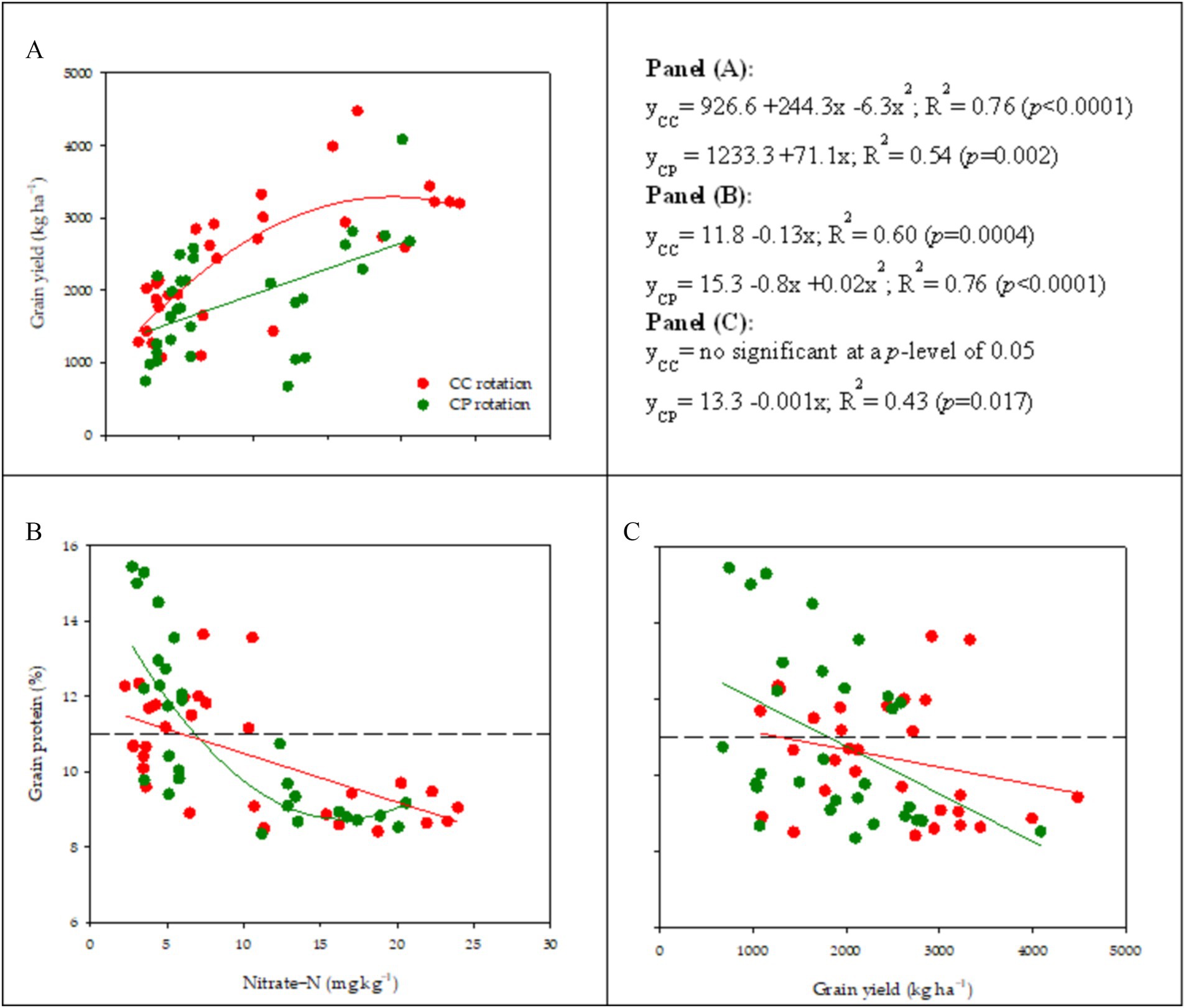
Figure 3. Data relationship between nitrate-N at tillering and wheat grain yield, (A) nitrate-N and grain protein concentration (B), and wheat grain yield and grain protein concentration (C). This was evaluated for the three experimental years (2014–2016) and the four N rates (0, 30, 60, and 90). The dotted line represents the reference value of grain protein concentration (11.5%), which defines the wheat marketing specification in Uruguay.
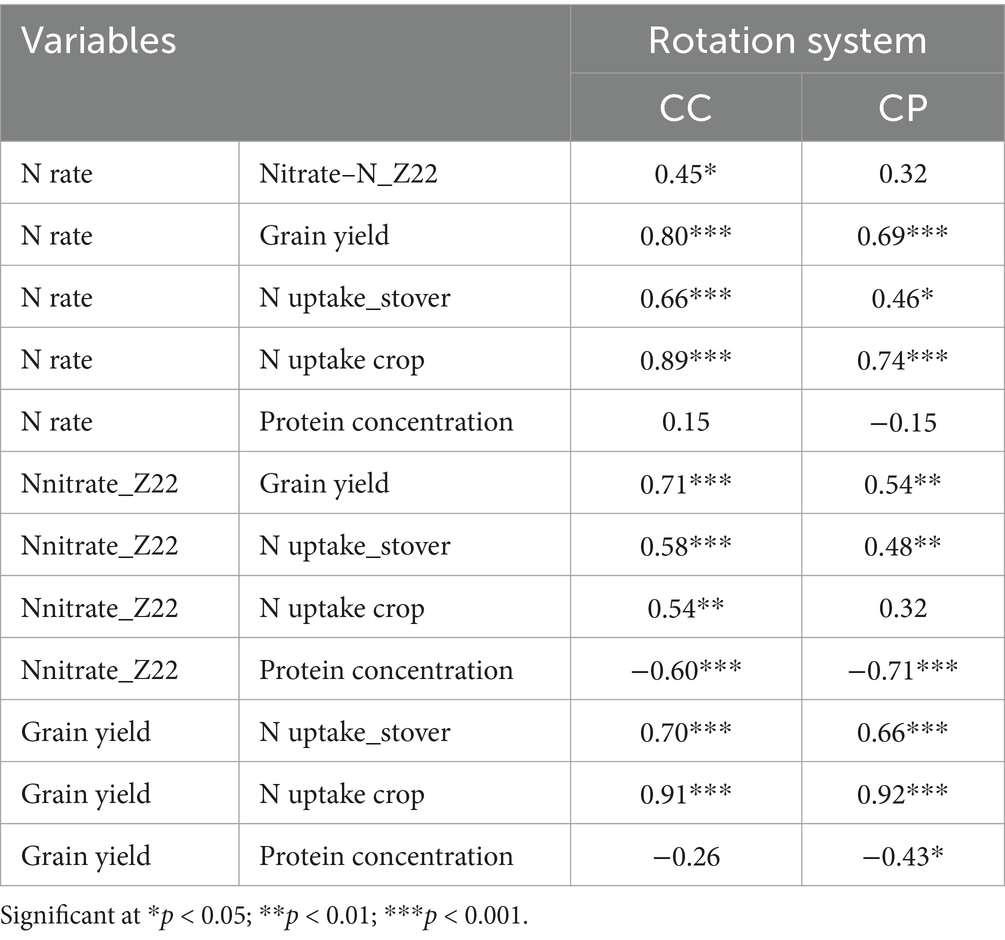
Table 4. Pearson correlation coefficients (r) within each rotation system: continuous cropping (CC) and crop-pasture (CP) across the three experimental years (2014–2016) and all N rates (0, 30, 60, and 90 kg N ha−1).
The N effect on GPC was statistically significant in both systems. In 2016, GPC values in wheat growing in CC were similar between unfertilized and fertilized treatments (Table 3). In contrast, in CP, GPC diminished with increased N supply, similar to findings by Bedoussac and Justes (2010). The N response curves for GPC (represented by a positive quadratic response) demonstrate the dilution effect of GPC, which was clearly shown in CP in 2016 at a fertilization rate of 30 and 60 kg N ha−1, attributable to the increase in grain yield per kg of grain N (Table 3).
A multiple regression model incorporating N rate and NO3–N at tillering accounted for 68% (p < 0.0001, Figure 4) of the variation in wheat grain yield (WGY). The standardized major axis (SMA) regression test revealed that the best model segregated by rotation had an equal slope (0.59). However, different elevations for each rotation level improved prediction accuracy, explaining 82% of the variation in grain yield for CC and 65% for CP. Nevertheless, the model overestimated low yields in CP and underestimated high yields in CC, likely due to differing relationships between N rate and yield (linear in CC, quadratic in CP). For grain protein content (GPC), only NO3–N at tillering was significant, explaining 54% (p < 0.002) of the variation. The SMA regression test for GPC indicated no significant difference in slopes and elevation.
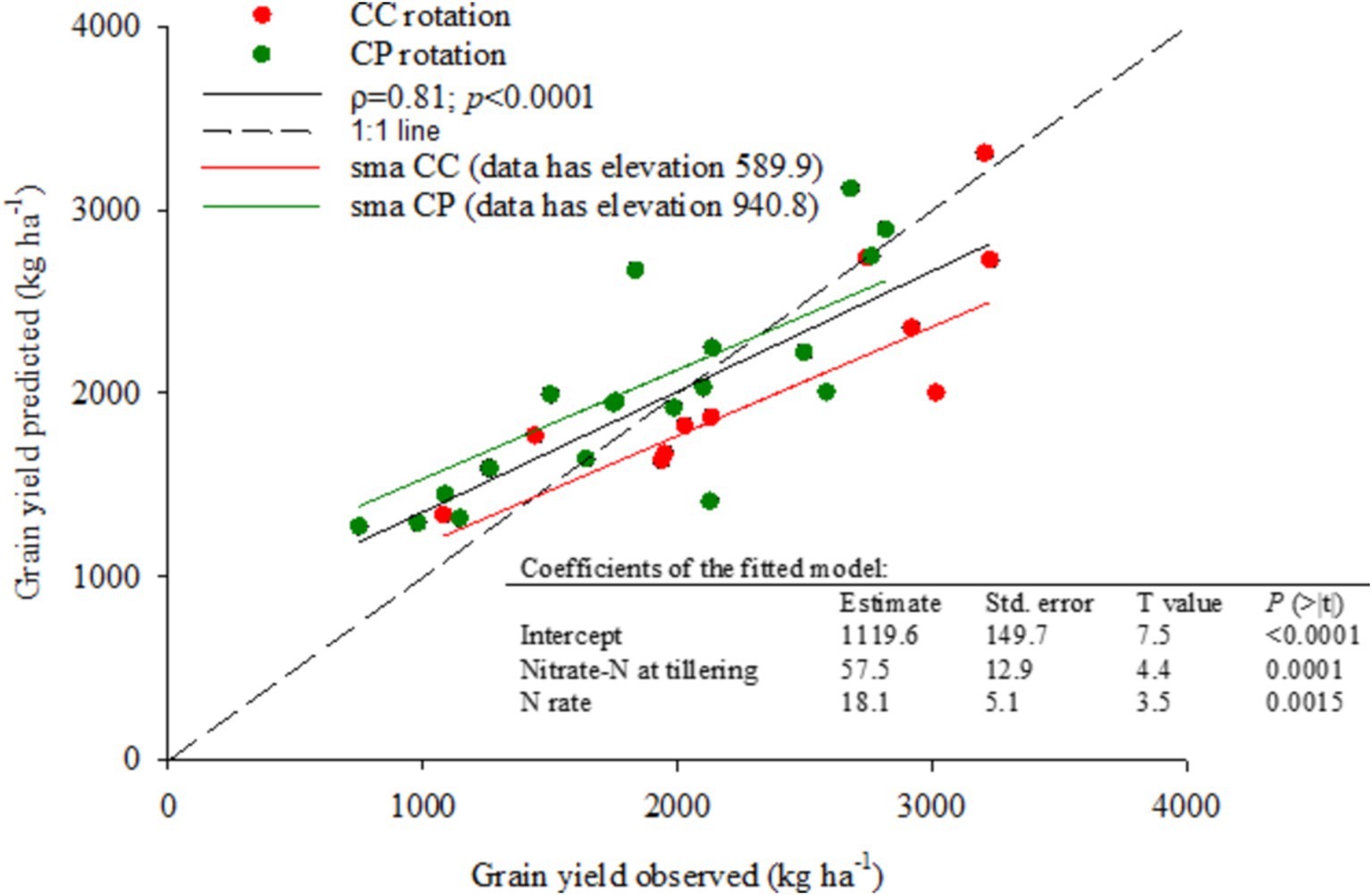
Figure 4. Scatterplot of grain yield observed against predicted and included SMAs: fitted separately for each level of the rotation factor (the red line represents CC, and the green line represents CP rotation). The 1:1 line is given.
3.4 Effects of year and N rate segregated by rotation on NUE indices
Based on 2015 and 2016 data and considering the three fertilized treatments, the ANOVA showed that neither year nor Nrate and its interaction in both rotations had a statistically significant effect on two fertilizer-based indices AE (Equation 1), and RE (Equation 2), and the plant-based index IE (Equation 3) (Table 5). Both indices were higher in CC (22.4 kg of grain kg−1 N added and 55.2%) than in CP (20.4 kg of grain kg−1 N added and 38.3%).
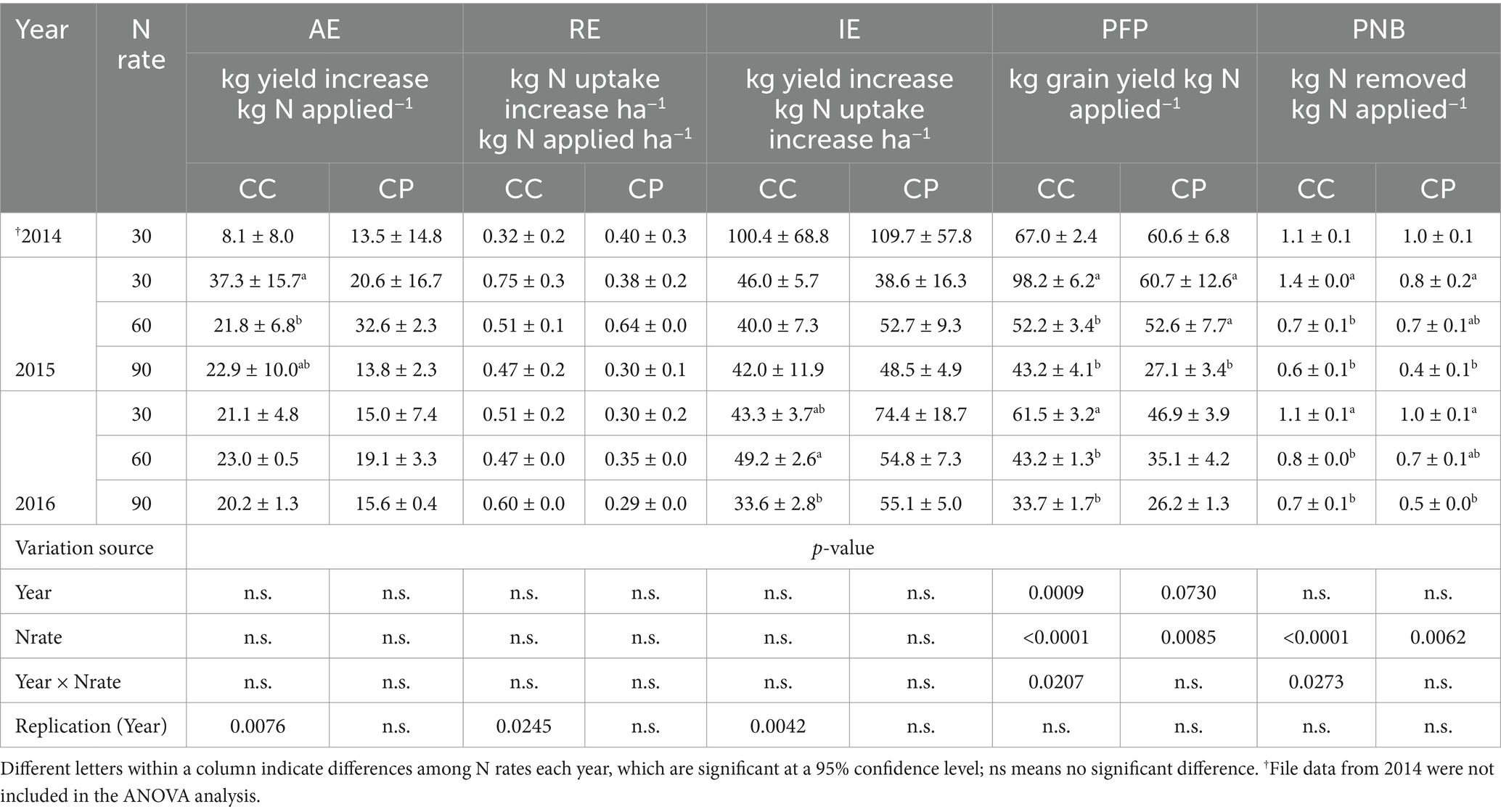
Table 5. Means and standard errors for fertilizer-based indices: agronomic efficiency (AE), recovery efficiency (RE), internal efficiency (IE), partial factor productivity (PFP), and partial nutrient balance (PNB) for wheat crops by year, N rate, and rotation system: continuous cropping (CC) and crop-pasture (CP).
The PFP analysis indicated that the main effects of year and Nrate were statistically significant in both rotation systems. However, the interaction effect of year × Nrate was statistically significant only in the CC rotation system. Regarding PNB, the year’s effect was not statistically significant; however, the Nrate effect was significant in both rotation systems. Similar to PFP, the interaction effect was only significant in CC.
The average AE achieved across years, and N rate was 24.4 and 19.4 kg ka−1 in CC and CP, respectively. Furthermore, the AE values were significantly associated with RE (r = 0.94, p < 0.0001 and r = 0.91, p < 0.0001, respectively) but not with IE in both rotations.
4 Discussion
4.1 Year and N rate effects segregated by rotation on soil mineral N
The presence of Cynodon dactylon was likely responsible for the lowest concentration of NO3–N observed in 2015 in CP at wheat seeding. Its residues probably reduced the soil’s mineral N content by absorbing it and competing with other pasture species, particularly legumes, thereby influencing their endurance toward the end of the pasture cycle. Consequently, the C: N ratio of the pasture residues might have increased, leading to either N immobilization in the soil or limited soil N availability to plants due to slow residue breakdown (Dang et al., 2020). In 2015, NO3–N concentrations at tillering were similar to those at seeding, whereas in 2014 and 2016, they were only a third of those at seeding. The 2015 results could be related to low rainfall during the early stages of crop growth, while those of 2014 and 2016 might be due to heavy Julyrain (135 and 163 mm, respectively), which were more than doubled the expected July rain based on the 30-year historical average. The N response on NO3–N in CP in 2016 was less than in CC, even though the values were similar in 0 N plots under both rotation systems. This finding suggests that a fraction of the N applied at wheat seeding was lost or immobilized in the soil in CP, leading to a lesser increase in soil N availability at tillering.
The noteworthy difference in the quantity and proportion of N forms (ammonium vs. nitrate) between systems observed after the wheat harvest can be attributed to the more favorable soil conditions for net mineralization at this point in the season in CP rotation (Supplementary Table S2). The decomposition process of the remaining pasture residues was in its final phase, likely resulting in a lower rate of N immobilization.
The increased NH4–N to NO3–N ratio in 2014 and 2016 aligned with the periods of heaviest rainfall during the wheat tillering seasons. These findings suggest that soil compaction may have influenced the N dynamics in the CP rotation, resulting in reduced nitrification rates but higher NH4–N accumulation in the soil (Longepierre et al., 2022). Furthermore, soil compaction might uphold higher moisture soil levels, particularly during slower drying periods like winter, leading to N loss through denitrification (Dang et al., 2020; Shaheb et al., 2021). Regarding these changes in soil N dynamics, Booth et al. (2005) showed that nitrification emerged as the primary process for NH4+ transformation at low mineralization rates, with only minor changes observed at higher rates. Conversely, the rate of NH4+ immobilization showed a steady increase across the entire spectrum of mineralization rates. They suggested that nitrifiers effectively outcompete heterotrophic microorganisms for NH4+ when soil C content is low. Based on this, we hypothesize that the competitive capacity of nitrifiers for NH4+ could be higher in CC under soil conditions with a low lability pool C and with a higher C: N ratio under this system (Supplementary Table S3). In contrast, heterotrophic microorganisms may have a higher competition capacity for NH4+ in soils with more fresh available C in the soil rhizosphere, such as CP soil, where the C source would drive N immobilization (Sun et al., 2019).
In our study, under soil no-till conditions, we found no disparities in soil C and N concentration levels between cropping systems rotating with pastures and those focused on continuous annual cropping system (Supplementary Table S3). These findings align with studies indicating that reduced tillage practices are crucial for preserving SOC (Dang et al., 2020; Page et al., 2020; Rubio et al., 2022). Additionally, comparing our C data with that reported by Salvo et al. (2010), whose study was carried out in the same experimental site as this work, it becomes evident that merely combining no-till techniques with crop-pasture rotations may not result in higher SOC levels (Dang et al., 2020; Grant et al., 2020; Wang et al., 2023b). This limitation in C sequestration could be associated with the system’s N balance, which is generally negative and similar between CC and CP when their cropping phase includes C4 species (Pravia et al., 2019; Dang et al., 2020).
4.2 Effects of year and N rate segregated by rotation on physical productivity and quality of wheat
4.2.1 Wheat grain yield and grain protein concentration
The WGY response observed in CP aligned with expectations, as crops following legume pastures showed reduced response to applied N, consistent with previous studies (Peoples et al., 2015; Allen et al., 2021; Herridge et al., 2022; Nurbekov et al., 2024). The maximum yield was achieved with a dose of 60 N. In contrast, the response observed in CC indicates that the optimal N rate would have been above the maximum dose applied (> 90 N). However, the higher WGY under CC suggested that other growth factors, which were less favorable under CP rotation, contributed to better wheat growth under CC. Although in this study, we did not measure physical properties such as bulk density or soil macroporosity, the difference in performance between systems may have stemmed from increased soil compaction under CP rotation due to cattle trampling. Barreto et al. (2022) also found higher surface runoff under CP than CC, attributing this difference to the trampling effect. These authors noted that under the CP system, there would be higher risks of nutrient losses with runoff water. Additionally, there was no indication of the expected increase in soil residual N under CP through elevated soil NO3–N concentrations in this rotation. As mentioned, this higher soil N contribution may occur later in the crop cycle (post-Z22 growth stage). Alternatively, N losses due to denitrification could increase, potentially exacerbated by soil compaction (Dang et al., 2020; Shaheb et al., 2021).
For both systems, the highest WGY was obtained in 2015, a year with more favorable climate conditions, alongside the highest levels of NO3–N during tillering compared to the other evaluated seasons. Furthermore, in 2015, the wheat seeding occurred amidst dry soil conditions, along with a notable presence of Cynodon dactylon in the CP rotation, resulting in poor crop establishment (though not quantified) and uneven emergence, leading to a negative impact on WGY, compared to CC. This weed invades the N-enriched areas in pastures created by the death of legume plants during summer droughts, effectively competing with the most commonly used pasture species (Pañella et al., 2022). Additionally, its underground biomass binds soil aggregates into a dense structure, which, combined with surface compaction from grazing, would lead to poor soil quality (García-Préchac et al., 2004; Dang et al., 2020; Shaheb et al., 2021). In 2016, yields were lower than 2015, possibly due to low NO3–N levels during tillering caused by heavy rainfall in July (163 mm total, with a third falling two weeks after N application at tillering) or due to reduced water availability in the subsequent period, as precipitation from August to October was below the 30-year average. The effect of soil N availability on wheat yield was evident in 2015; even though the NO3–N concentration during tillering was close to the critical range (12–14 mg kg−1) (Fassana et al., 2022), there was a noticeable N response under CC system. As has noted by other works, N assimilation is generally more negatively affected than mineralization by soil drying (Compton and Boone, 2002). Furthermore, in drying soils, NO3-assimilation is impaired before nitrification, suggesting that the overall production rates of inorganic N may increase as soils become drier, which was observed in our study during wheat tillering stage in 2015.
Our results showed that wheat crops seeded after pasture termination yielded less than the wheat-followed a soybean crop under CC. This suggests that the effect of the previous crop (soybean) on wheat yield was more relevant than the expected residual effect of the pasture phase under CP (Lollato et al., 2019b; Griffiths et al., 2022; Arnhold et al., 2023). Grain yield of wheat under CC would have depended more on soil N availability at tillering. In contrast, following a degraded pasture under CP, other factors would limit the wheat yield (Figure 3A). Furthermore, under CP, higher standard errors were recorded in WGY and other measured variables among experimental units, potentially attributed to the trampling and nutrient distribution (Carvalho et al., 2018; Dubeux and Sollenberger, 2020).
The measured GPC values were within the range described by other authors (Ding et al., 2020), being higher under CP than CC, as expected. Additionally, WGY and GPC had a significant negative association in the CP system, confirming that they are usually negatively correlated (Ghimire et al., 2021; Giordano et al., 2023; Figure 3C).
4.3 Relationships between nitrate-N at tillering and grain yield and quality segregated by rotation
The relationship between WGY and GPC is closely linked to N availability. When N supply is low, adding N increases yield but does not change or decrease GPC, with medium N availability, both yield and protein can increase, whereas with high N availability, fertilization mainly affects grain protein concentration (Ma et al., 2019; Ghimire et al., 2021; Giordano et al., 2023; Wang et al., 2023a). This relationship suggests that the negative correlations between WGY and GPC occur when wheat growth is severely N-deficient or when the N supply is low or unsynchronized with the crop’s N demand for its potential yield. For instance, in high-production years like 2015 with favorable weather conditions, even small increases in N availability led to significant yield increases (Table 3). At this point, GPC either did not increase or sometimes decreases with higher N rates (Table 3; Figure 3C) because the increase in N uptake by the crop was small relative to the increase in WGY. Additionally, the negative correlations between WGY and GPC in CP (Figure 3C) could be attributed to other factors limiting wheat yield (Figure 3A). These include increased N immobilization by the pasture residue and the delayed release of this N beyond the critical uptake periods (heading and grain filling stages) of the following wheat crop. Poor soil physical conditions that hinder wheat performance, N uptake, and NUE might have also played a role. This latter factor is particularly plausible as it could reduced the plant’s growth rate and N uptake capacity. Soil N availability for plants depends on the soil’s ability to provide resources and the plant’s sink strength, which enhances crop N demand and, consequently, the efficiency to absorb these resource (Lemaire et al., 2021; Giordano et al., 2023). The higher N response on WGY in 2015 compared with 2016 explains the lower GPC values observed in that year (Table 2). This difference is likely due to differences in the amount and distribution of rainfall (Figure 2A). The wetter season in 2015, with rainfall 100 mm higher than in 2016, may have led to higher N dilution in the plant (Grahmann et al., 2013; Ghimire et al., 2021). Moreover, the differences in NO3-N availability at wheat tillering between years (Table 2) accounted for the year effect on GPC; the greater the NO3-Navailability, as seen in 2015, higher WGY and, consequently, the more significant dilution of grain protein (Ghimire et al., 2021).
4.4 Effects of year and N rate segregated by rotation on NUE indices
The two fertilizer-based indices, RE and AE, were higher under CC than CP (Table 5) and within the typical N range for cereal crops harvested for grain (Ma et al., 2019; Hausherr Lüder et al., 2020; Hu et al., 2023; Li et al., 2023). The AE data remained stable across different N doses in 2016, marked by unfavorable weather conditions and reduced soil N availability during wheat tillering. Comparing AE values across cropping systems, the CP rotation exhibited greater variability among N treatments and generally lower values than CC. This variability in CP is likely due to factors other than temporary N deficiency, as a more significant N deficiency would have led to a greater wheat yield response to applied N. The inherent variability of integrated crop-livestock systems could also explain this fluctuation.
The PFP and PNB indices were higher under CC than CP, suggesting that fertilized wheat in CC, especially at low N rates, likely absorbed more native soil N than unfertilized crops. Consequently, this native soil N might have been mistakenly quantified as coming from the fertilizer, possibly leading to an overestimation of RE (Liang et al., 2017, 2023; Chen et al., 2024). Additionally, both indices decreased with increasing N rates, a trend observed in many studies (Gastal et al., 2015; Sieling and Kage, 2021; Hu et al., 2023; Li et al., 2023). In both rotations, PNB values were higher than 1 for wheat fertilized with low N levels, indicating a potential threat to soil fertility or productivity degradation (Pravia et al., 2019; Congreves et al., 2021). The highest values were seen in CC, where nutrient availability is typically low. Fontaine et al. (2023) reported that soils with significant SOM accumulation, such as those in CP systems, often exhibit reduced N availability for plants. This is attributed to continuous C and nutrient sequestration, driven by high microbial N assimilation, which leads to a limited response to N fertilization because of the low nutrient demand from plants in this scenario (Cotrufo and Lavalle, 2022). Conversely, CC systems are expected to show lower microbial N immobilization in the soil and greater depletion of SOM, particularly in the MAOM-associated fraction (Cotrufo and Lavalle, 2022). This suggests that N immobilization would be higher in CP, which explains the lower AE in CP compared to CC.
The efficiency of the N recovered from the added fertilizer was significantly associated with the AE index, as was seen in another study (Hausherr Lüder et al., 2020). The higher AE in CC due to its higher RE can be explained by an expected lower soil N availability in this system. The results indicate that soil N availability was an important factor limiting wheat yield in both rotations, particularly in the CC. These findings align with Ernst et al. (2020), who, comparing systems at equivalent yield (using as target yield obtained in the system that yielded the least). They found that CC,-including C4 crops, required a slightly more N fertilizer than CP, both systems under no-till management, to achieve comparable grain yield because CC was more dependent on N fertilizer inputs. The lack of pasture legumes in CC led to a lower potentially mineralizable N, which could significantly reduce N uptake in unfertilized treatments by 20% on average (Ernst et al., 2020). In CP, however, the quadratic N response revealed that factors other than N could have limited the wheat performance of that system. The factor limiting the yield response in CP rotation likely disrupted the synchrony between the N demand of crop and the amounts of N provided by both N sources, fertilizer, and soil. In our study, these factors could be identified through data analysis.
4.5 Implications and limitations of the study
Based on the assumption that the total residue dry matter input and soil C were similar between rotations (Supplementary Table S3), a finding also reported by Ernst et al. (2020) comparing similar rotation systems that this study, we propose the following hypothesis. The growth-limiting factor, which could explain the disparities between systems, is likely to be associated with factors other than nutrients and the previous crop effect. These factors could include adverse effects on soil physical properties caused by animal trampling. The discordant result in the CP system, which consistently showed lower WGY, despite the expected higher soil quality and higher soil productivity potential (Ernst et al., 2018; Rubio et al., 2022) compared to CC, could be explained by considering two reasons:
1. Residues quality from tcrop sequences. We compared wheat performance seeded after a degraded pasture due to intensive grazing (invaded by Cynodon dactylon in 2015), which led to a low decomposition rate and potential N immobilization, versus wheat seeded after soybean, which provides a favorable environment for residue breakdown and N response. While incorporating perennial pastures into crop rotations may offer advantages in accumulating SOC, due to greater root and shoot allocation (Mazzilli et al., 2015; Pinto et al., 2021), the low-quality residue left in the soil at the final pasture phase negatively affected the wheat performance. This, in turn, affected the soil’s physical conditions for the subsequent wheat crop, limiting its performance and the efficiency of N recovery from added fertilizer. However, this effect is likely temporary, as soil quality improvement would become evident with the sequence of crops that constitute the agricultural phase (Ernst et al., 2018). When comparing the annual cropping phase only, CC and CP represent a diversified and intensive cropping system (5 crops in 3 years), a relevant variable for mitigating soil quality depletion (Novelli et al., 2017; Allen et al., 2021; Rubio et al., 2022; Alvarez and Ernst, 2024). Consequently, the differences between CP and CC are minimized when crop productivity is evaluated across the entire system (Ernst et al., 2020).
2. Temporary productivity limitation in CP. The limiting factor for productivity in CP might be temporary, affecting only the first crop after pasture termination since the negative impacts of animal trampling typically affect only shallow soil depths. Additionally, the soil’s physical properties, such as macroporosity, may recovered through natural processes (Bell et al., 2011; Dang et al., 2020). The response of wheat growing in compacted soil could be highly dependent on the season, with the influence of the limiting factor and dissimilarities between rotations being more noticeable in high-production years with favorable weather, as observed in 2015.
Our results highlight potential areas for improvement, such as increasing crop diversity by introducing alternative crops like intermediate wheatgrass (Thinopyrum intermedium L.). This perennial grain crop is promising for temperate regions with mild winters (Locatelli Fagúndez, 2023). Its dense and fibrous root system can enhance soil aggregate stability, as was reported with other perennial species following an agricultural phase (García-Préchac et al., 2004); thus, integrating this perennial species into the rotation could help mitigate or reduce soil compaction damage. Lupine cultivation is another promising option for diversifying crops within agricultural systems and reducing N and P fertilizer use (Griffiths et al., 2022; Mori Alvez et al., 2024). For lupines, the potential impact of its roots on improving soil structure alongside other crops and forage pastures has been evaluated (Bodner et al., 2021; Griffiths et al., 2022). Legumes were more effective in stabilizing soil structure than non-legumes, with lupine and lucerne being especially effective (Bodner et al., 2021). The researchers also observed that roots might stabilize some soil fractions for particular plant/soil combinations that influence soil physical properties, such as soil structure through biopore channels, with this impact being more pronounced in species with coarse roots, such as legumes (Boldrin et al., 2022; Wang et al., 2023b; Giuliani et al., 2024). The integration and diversification of functional groups—such as annual winter legumes like lupine—may be a pivotal strategy to reverse soil deterioration processes (Hallama et al., 2019; Fontaine et al., 2023; Nurbekov et al., 2024). An additional adjustment can be to shorten the pasture phase by changing the existing crop arrangement. This shortening in the pasture phase intends to reach the end of its cycle with better aboveground and belowground biomass, a higher legume proportion in mixed pasture swards, and roots in active growth (Hamza and Anderson, 2005; Huang et al., 2020; Zhou et al., 2021), increasing system sustainability and resilience derived from increasing non-provisioning ecosystem services with long pasture phases, such as improved soil physical conditions and the legume persistence at pasture termination.
In this study, we assessed only changes in soil N availability, WGY, N uptake, GPC, and the NUE as affected by the N response trials, inferring from these experiments performed consistencies or dissimilarities between cropping systems. However, this approach may miss other system-wide benefits or tradeoffs.
Due to the limitations of our field experiment, we propose an effective and practical method for a fair comparison of the potential of CP and CC systems. Additionally, this approach allows for direct inferences about the rotation effect, as this experimental design would include three replicates with the rotation factor, minimizing the pre-crop effect observed on wheat crops and isolating the year effect from the rotation effect. This method involves using data from the same long-term experiment, involving more than one crop in the same year of the evaluation, and associating them with the same pre-crop under both systems. For instance, in this approach, we could use winter crops as pre-crops or the fallow period as pre-conditions for summer crops used as test crops (whose crops offer clear advantages under no-till conditions). This proposal must be replicated for more than two years. It is also necessary to consider soil C and N levels and the quantity and quality of residues and correlate this with soil health indicators. Equally important is using the best available knowledge and technologies to reduce the impact of nutrient stress, pests, and weeds affecting crop establishment (e.g., uneven emergence) in both CP and CC systems.
5 Conclusion
Our three-year research study on Uruguayn soils under a long-term experiment spanning over 20 years, revealed that wheat crops in the CC system achieved higher yields than those seeded as the first annual crop after the long perennial pasture phase in the CP system in a rainfed environment. Additionally, the greater N response in CC was likely due to reduced soil N availability, which, combined with healthier soil physical properties, supported improved productivity, This resulted in higher N uptake and better NUE indices compared to the CP system. In contrast, adverse soil conditions in the CP rotation may have impeded the effective utilization of applied N in wheat production. Our findings suggested that under continuous no-till systems, the performance of the first crop seeded after a long perennial pasture phase (3.5 years) was negatively affected by factors such as animal trampling and the quality of crop residues returned to the soil. While such a system would support greater soil fertility and GPC values, it had a detrimental effect on WGY due to temporarily restricted soil physicochemical and biological properties. These restrictions limited the N response, consequently reducing wheat yield and NUE indices. Our hypothesis that integrating pastures into crop rotations would improve wheat yields (as the first crop after the pasture phase) was not supported. The anticipated benefits of enhanced soil quality and N availability were insufficient to counteract the negative impacts of cattle trampling and grazing.
However, increased N immobilization could enhance C and N sequestration in SOM. This N process could be encouraged by temporary soil compaction, creating more conducive soil conditions typically characterized by moisture and relatively low temperatures. For future research, our findings underscore the need to directly assess soil properties linked to physical degradation that affect water dynamics, such as infiltration and retention. It is also crucial to evaluate highly sensitive C and N indicators in response to changes in their balance, including the distribution between POM and MAOM and the potentially mineralizable N from these SOM fractions and soil C respiration. This assessment is highly dependent on factors like soil type, environmental conditions, and the quantity and quality of residues left in the soil. Therefore, future research should focus on characterizing all these aspects within the evaluated system.
Data availability statement
The raw data supporting the conclusions of this article will be made available by the authors, without undue reservation.
Author contributions
CM: Conceptualization, Data curation, Formal analysis, Investigation, Methodology, Project administration, Visualization, Writing – original draft, Writing – review & editing. OE: Resources, Supervision, Visualization, Writing – review & editing. PG: Formal analysis, Methodology, Visualization, Writing – review & editing. CP: Conceptualization, Data curation, Funding acquisition, Investigation, Project administration, Supervision, Visualization, Writing – review & editing.
Funding
The author(s) declare that financial support was received for the research, authorship, and/or publication of this article. This research received funds from IAEA within the Coordinated Research Project D12012 framework, entitled ‘Optimizing Soil, Water and Nutrient Use Efficiency in Integrated Cropping-Livestock Production Systems.’ The National Research of our Institution, the University of the Republic (UdelaR, Uruguay), funded the publication of this article. This work is part of the senior author’s doctoral dissertation at the Doctoral Program in Agricultural Sciences, Agronomy College, UdelaR, Uruguay.
Acknowledgments
The authors thank Pablo Fontes, Andrei Assanelli, Nicola Di Muro, and Daniel Arana for their assistance throughout the field experiment and laboratory work. We also thank the EEMAC staff for their field assistance and collaboration during the entire period of this investigation work (2014-2017). Finally, we thank Dra. (Ing. Agr.) Amabelia del Pino for careful and extensive revision, guiding data analysis, and comprehensive feedback on the work.
Conflict of interest
The authors declare that the research was conducted in the absence of any commercial or financial relationships that could be construed as a potential conflict of interest.
Generative AI statement
Grammarly.com (version 8.926.0) was used during the preparation of this article as a tool to assist with grammar and style corrections.
Publisher’s note
All claims expressed in this article are solely those of the authors and do not necessarily represent those of their affiliated organizations, or those of the publisher, the editors and the reviewers. Any product that may be evaluated in this article, or claim that may be made by its manufacturer, is not guaranteed or endorsed by the publisher.
Supplementary material
The Supplementary material for this article can be found online at: https://www.frontiersin.org/articles/10.3389/fsufs.2024.1460734/full#supplementary-material
References
Allen, B. L., Lenssen, A. W., Sainju, U. M., Jabro, J. D., and Stevens, W. B. (2021). Nitrogen use in spring wheat affected by crop diversification, management, and tillage. Agron. J. 113, 2437–2449. doi: 10.1002/agj2.20686
Alvarez, S., and Ernst, O. (2024). Impact of cropping systems on soil quality. Eur. J. Agron. 158:127197. doi: 10.1016/j.eja.2024.127197
Arnhold, J., Grunwald, D., Braun-Kiewnick, A., and Koch, H. J. (2023). Effect of crop rotational position and nitrogen supply on root development and yield formation of winter wheat. Front. Plant Sci. 14:1265994. doi: 10.3389/fpls.2023.1265994
Baethgen, W. E., Parton, W. J., Rubio, V., Kelly, R. H., and Lutz, M. (2021). Ecosystem dynamics of crop–pasture rotations in a fifty-year field experiment in southern South America: century model and field results. Soil Sci. Soc. Am. J. 85, 423–437. doi: 10.1002/saj2.20204
Baiyeri, P. K., Foleng, H. N., Machebe, N. S., and Nwobodo, C. E. (2019). Crop-livestock interaction for sustainable agriculture. Part VI Agricultural Sustainability in Changing Climate in eds. M. Farooq and M. Pisante (Switzerland: Springer Nature.) Innov. Sustain. Agric. 557–582. doi: 10.1007/978-3-030-23169-9_18
Barbazán, M., Bautes, C., Beux, L., Bordoli, M., Cano, J. D., Ernst, O., et al. (2011). Fertilización potásica en cultivos de secano sin laboreo en Uruguay: rendimiento según análisis de suelos. Agrociencia 15, 93–99. doi: 10.31285/agro.15.597
Barreto, P., Ernst, O., Pérez Bidegain, M., and Perdomo, C. (2022). Effects of grazing, rotation, and tillage on surface runoff in a heavy textured Uruguayan soil. Soil Sci. Soc. Am. J. 86, 1096–1112. doi: 10.1002/saj2.20427
Bedoussac, L., and Justes, E. (2010). The efficiency of a durum wheat-winter pea intercrop to improve yield and wheat grain protein concentration depends on N availability during early growth. Plant Soil 330, 19–35. doi: 10.1007/s11104-009-0082-2
Bell, L. W., Kirkegaard, J. A., Swan, A., Hunt, J. R., Huth, N. I., and Fettell, N. A. (2011). Impacts of soil damage by grazing livestock on crop productivity. Soil Tillage Res. 113, 19–29. doi: 10.1016/j.still.2011.02.003
Bodner, G., Mentler, A., and Keiblinger, K. (2021). “Plant roots for sustainable soil structure Management in Cropping Systems” in The Root Systems in Sustainable Agricultural Intensification. eds. Z. Rengel and I. Djalovic (Hoboken, NJ: John Wiley & Sons), 45–90.
Boldrin, D., Knappett, J. A., Leung, A. K., Brown, J. L., Loades, K. W., and Bengough, A. G. (2022). Modifying soil properties with herbaceous plants for natural flood risk-reduction. Ecol. Eng. 180:106668. doi: 10.1016/j.ecoleng.2022.106668
Booth, M. S., Stark, J. M., and Rastetter, E. (2005). Controls on nitrogen cycling in terrestrial ecosystems: a synthetic analysis of literature data. Ecol. Monogr. 75, 139–157. doi: 10.1890/04-0988
Carvalho, P. C. F., Peterson, C. A., Nunes, P. A. A., Martins, A. P., de Souza Filho, W., Bertolazi, V. T., et al. (2018). Animal production and soil characteristics from integrated crop-livestock systems: toward sustainable intensification. J. Anim. Sci. 96, 3513–3525. doi: 10.1093/jas/sky085
Cassman, K. G., and Grassini, P. (2020). A global perspective on sustainable intensification research. Nat. Sustain. 3, 262–268. doi: 10.1038/s41893-020-0507-8
Chen, S., Guo, J., Guo, R., Huang, B., Huang, J., Wang, M., et al. (2024). Deciphering the active bacteria involving glucose-triggered priming effect in soils with gradient N inputs. Soil Biol. Biochem. 199:109612. doi: 10.1016/j.soilbio.2024.109612
Colombi, T., and Keller, T. (2019). Developing strategies to recover crop productivity after soil compaction—a plant eco-physiological perspective. Soil Tillage Res. 191, 156–161. doi: 10.1016/j.still.2019.04.008
Compton, J. E., and Boone, R. D. (2002). Soil nitrogen transformations and the role of light fraction organic matter in forest soils. Soil Biol. Biochem. 34, 933–943. doi: 10.1016/S0038-0717(02)00025-1
Congreves, K. A., Otchere, O., Ferland, D., Farzadfar, S., Williams, S., and Arcand, M. M. (2021). Nitrogen use efficiency definitions of today and tomorrow. Front. Plant Sci. 12:637108. doi: 10.3389/fpls.2021.637108
Cotrufo, M. F., and Lavallee, J. M. (2022). Soil organic matter formation, persistence, and functioning: A synthesis of current understanding to inform its conservation and regeneration. Adv. Agron. 172, 1–66. doi: 10.1016/bs.agron.2021.11.002
Dang, Y. P., Dalal, R. C., and Menzies, N. W. (2020). No-till farming Systems for Sustainable Agriculture: challenges and opportunities. New York: Springer.
Ding, J., Li, F., Le, T., Wu, P., Zhu, M., Li, C., et al. (2020). Nitrogen management strategies of tillage and no-tillage wheat following rice in the yangtze river basin, China: grain yield, grain protein, nitrogen efficiency, and economics. Agronomy 10:155. doi: 10.3390/agronomy10020155
Dong, F., and Zeng, W. (2024). Effects of fall and winter cover crops on weed suppression in the United States: a Meta-analysis. Sustain. For. 16:192. doi: 10.3390/su16083192
Drewry, J. J., Cameron, K. C., and Buchan, G. D. (2008). Pasture yield and soil physical property responses to soil compaction from treading and grazing – a review. Aust. J. Soil Res. 46, 237–256. doi: 10.1071/SR07125
Dubeux, J. C. B., and Sollenberger, L. E. (2020). “Nutrient cycling in grazed pastures” in Management Strategies for Sustainable Cattle Production in Southern Pastures. eds. M. Rouquette Jr. and G. Aiken (Cambridge, MA: Academic Press), 59–75.
Duru, M., Therond, O., Martin, G., Martin-Clouaire, R., Magne, M. A., Justes, E., et al. (2015). How to implement biodiversity-based agriculture to enhance ecosystem services: a review. Agron. Sustain. Dev. 35, 1259–1281. doi: 10.1007/s13593-015-0306-1
Ernst, O. R., Dogliotti, S., Cadenazzi, M., and Kemanian, A. R. (2018). Shifting crop-pasture rotations to no-till annual cropping reduces soil quality and wheat yield. F. Crop. Res. 217, 180–187. doi: 10.1016/j.fcr.2017.11.014
Ernst, O. R., Kemanian, A. R., Mazzilli, S., Siri-Prieto, G., and Dogliotti, S. (2020). The dos and don’ts of no-till continuous cropping: evidence from wheat yield and nitrogen use efficiency. F. Crop. Res. 257:107934. doi: 10.1016/j.fcr.2020.107934
FAO (2011). Save and grow a policymaker’s guide to the sustainable intensification of smallholder crop production. Rome: Food and Agriculture Organization of the United Nations.
Fassana, C. N., Hoffman, E. M., Berger, A., and Ernst, O. (2022). Nitrogen nutrition index at GS 3.3 is an effective tool to adjust nitrogen required to reach attainable wheat yield. Agrociencia Uruguay 26:924. doi: 10.31285/AGRO.26.924
Fontaine, S., Abbadie, L., Aubert, M., Barot, S., Bloor, J. M. G., Derrien, D., et al. (2023). Plant–soil synchrony in nutrient cycles: learning from ecosystems to design sustainable agrosystems. Glob. Chang. Biol. 30:e17034. doi: 10.1111/gcb.17034
Franzluebbers, A. J. (2013). Pursuing robust agroecosystem functioning through effective soil organic carbon management. Carbon Manag. 4, 43–56. doi: 10.4155/cmt.12.78
Franzluebbers, A. J., and Stuedemann, J. A. (2014). Crop and cattle production responses to tillage and cover crop management in an integrated crop-livestock system in the southeastern USA. Eur. J. Agron. 57, 62–70. doi: 10.1016/j.eja.2013.05.009
García-Préchac, F., Ernst, O., Siri-Prieto, G., and Terra, J. A. (2004). Integrating no-till into crop-pasture rotations in Uruguay. Soil Tillage Res. 77, 1–13. doi: 10.1016/j.still.2003.12.002
Gastal, F., Lemaire, G., Durand, J. L., and Louarn, G. (2015). “Quantifying crop responses to nitrogen and avenues to improve nitrogen-use efficiency” in Crop Physiology: Applications for Genetic Improvement and Agronomy. 2nd ed (Cambridge, MA: Academic Press), 161–206.
Ghimire, D., Das, S., Mueller, N. D., Creech, C. F., Santra, D., Baenziger, P. S., et al. (2021). Effects of cultivars and nitrogen management on wheat grain yield and protein. Agron. J. 113, 4348–4368. doi: 10.1002/agj2.20836
Giordano, N., Sadras, V. O., and Lollato, R. P. (2023). Late-season nitrogen application increases grain protein concentration and is neutral for yield in wheat. A global meta-analysis. F. Crop. Res. 290:108740. doi: 10.1016/j.fcr.2022.108740
Giuliani, L. M., Hallett, P. D., and Loades, K. W. (2024). Effects of soil structure complexity to root growth of plants with contrasting root architecture. Soil Tillage Res. 238:106023. doi: 10.1016/j.still.2024.106023
Giunta, F., Mefleh, M., Pruneddu, G., and Motzo, R. (2021). Role of nitrogen uptake and grain number on the determination of grain nitrogen content in old durum wheat cultivars. Agronomy 11:42. doi: 10.3390/agronomy11010042
Grahmann, K., Rubio Dellepiane, V., Terra, J. A., and Quincke, J. A. (2020). Long-term observations in contrasting crop-pasture rotations over half a century: statistical analysis of chemical soil properties and implications for soil sampling frequency. Agric. Ecosyst. Environ. 287:106710. doi: 10.1016/j.agee.2019.106710
Grahmann, K., Verhulst, N., Buerkert, A., Ortiz-Monasterio, I., and Govaerts, B. (2013). Nitrogen use efficiency and optimization of nitrogen fertilization in conservation agriculture. CAB Rev. Perspect. Agric. Vet. Sci. Nutr. Nat. Resour. 8, 1–19. doi: 10.1079/PAVSNNR20138053
Grant, R. F., Dyck, M., and Puurveen, D. (2020). Nitrogen and phosphorus control carbon sequestration in agricultural ecosystems: modelling carbon, nitrogen, and phosphorus balances at the breton plots with ecosys under historical and future climates1. Can. J. Soil Sci. 100, 408–429. doi: 10.1139/cjss-2019-0132
Griffiths, M., Delory, B. M., Jawahir, V., Wong, K. M., Bagnall, G. C., Dowd, T. G., et al. (2022). Optimisation of root traits to provide enhanced ecosystem services in agricultural systems: a focus on cover crops. Plant Cell Environ. 45, 751–770. doi: 10.1111/pce.14247
Habbib, H., Hirel, B., Verzeaux, J., Roger, D., Lacoux, J., Lea, P., et al. (2017). Investigating the combined effect of tillage, nitrogen fertilization and cover crops on nitrogen use efficiency in winter wheat. Agronomy 7:66. doi: 10.3390/agronomy7040066
Hallama, M., Pekrun, C., Lambers, H., and Kandeler, E. (2019). Hidden miners – the roles of cover crops and soil microorganisms in phosphorus cycling through agroecosystems. Plant Soil 434, 7–45. doi: 10.1007/s11104-018-3810-7
Hamza, M. A., and Anderson, W. K. (2005). Soil compaction in cropping systems: a review of the nature, causes and possible solutions. Soil Tillage Res. 82, 121–145. doi: 10.1016/j.still.2004.08.009
Hatfield, J. L., and Beres, B. L. (2019). Yield gaps in wheat: path to enhancing productivity. Front. Plant Sci. 10:1603. doi: 10.3389/fpls.2019.01603
Hausherr Lüder, R. M., Qin, R., Richner, W., Stamp, P., Streit, B., Herrera, J. M., et al. (2020). Small-scale variation in nitrogen use efficiency parameters in winter wheat as affected by N fertilization and tillage intensity. Sustain. For. 12:3621. doi: 10.3390/su12093621
Herridge, D. F., Giller, K. E., Jensen, E. S., and Peoples, M. B. (2022). Quantifying country-to-global scale nitrogen fixation for grain legumes II. Coefficients, templates and estimates for soybean, groundnut and pulses. Plant Soil 474, 1–15. doi: 10.1007/s11104-021-05166-7
Hochman, Z., and Horan, H. (2018). Causes of wheat yield gaps and opportunities to advance the water-limited yield frontier in Australia. F. Crop. Res. 228, 20–30. doi: 10.1016/j.fcr.2018.08.023
Hu, S., Qiao, B., Yang, Y., Rees, R. M., Huang, W., Zou, J., et al. (2023). Optimizing nitrogen rates for synergistically achieving high yield and high nitrogen use efficiency with low environmental risks in wheat production – evidences from a long-term experiment in the North China plain. Eur. J. Agron. 142:126681. doi: 10.1016/j.eja.2022.126681
Huang, N., Athmann, M., and Han, E. (2020). Biopore-induced deep root traits of two winter crops. Agric. 10, 1–16. doi: 10.3390/agriculture10120634
IAEA (1990). Use of nuclear techniques in studies of soil-plant relationships. Training course series no 2 (Hardarson G, ed). Vienna, Austria: International Atomic Energy Agency. 223. Available at: https://inis.iaea.org/collection/NCLCollectionStore/_Public/21/086/21086585.pdf
Lemaire, G., Tang, L., Bélanger, G., Zhu, Y., and Jeuffroy, M. H. (2021). Forward new paradigms for crop mineral nutrition and fertilization towards sustainable agriculture. Eur. J. Agron. 125:126248. doi: 10.1016/j.eja.2021.126248
Li, B., Ma, X., Zhao, B., Wang, L., and Ata-Ul-Karim, S. T. (2023). In-season assessment of agronomic nitrogen use efficiency and its components in winter wheat using critical nitrogen dilution curve. Front. Plant Sci. 14:1128799. doi: 10.3389/fpls.2023.1128799
Liang, Z., Rasmussen, J., Poeplau, C., and Elsgaard, L. (2023). Priming effects decrease with the quantity of cover crop residues – potential implications for soil carbon sequestration. Soil Biol. Biochem. 184:109110. doi: 10.1016/j.soilbio.2023.109110
Liang, X., Yuan, J., Yang, E., and Meng, J. (2017). Responses of soil organic carbon decomposition and microbial community to the addition of plant residues with different C:N ratio. Eur. J. Soil Biol. 82, 50–55. doi: 10.1016/j.ejsobi.2017.08.005
Liu, J., Wang, Y., Li, Y., Peñuelas, J., Zhao, Y., Sardans, J., et al. (2023). Soil ecological stoichiometry synchronously regulates stream nitrogen and phosphorus concentrations and ratios. Catena 231:107357. doi: 10.1016/j.catena.2023.107357
Locatelli Fagúndez, A. (2023). Genetic and phenotypic characterization of Tinopiro (Thinopyrum intermedium) as a dual-purpose perennial crop in the southern cone: doctoral thesis abstract. Agrociencia Uruguay 27:e1463. doi: 10.31285/agro.27.1463
Lollato, R. P., Diaz, D. A. R., Dewolf, E., Knapp, M., Peterson, D. E., and Fritz, A. K. (2019a). Agronomic practices for reducing wheat yield gaps: a quantitative appraisal of progressive producers. Crop Sci. 59, 333–350. doi: 10.2135/cropsci2018.04.0249
Lollato, R. P., Figueiredo, B. M., Dhillon, J. S., Arnall, D. B., and Raun, W. R. (2019b). Wheat grain yield and grain-nitrogen relationships as affected by N, P, and K fertilization: a synthesis of long-term experiments. F. Crop. Res. 236, 42–57. doi: 10.1016/j.fcr.2019.03.005
Longepierre, M., Feola Conz, R., Barthel, M., Bru, D., Philippot, L., Six, J., et al. (2022). Mixed effects of soil compaction on the nitrogen cycle under pea and wheat. Front. Microbiol. 12:822487. doi: 10.3389/fmicb.2021.822487
Lussich Rachetti, F. (2020). Variabilidad de la fijación biológica de nitrógeno de leguminosas forrajeras en Uruguay: posibles causas y consecuencias nutricionales. Montevideo: University of the Republic, 1–85.
Ma, G., Liu, W., Li, S., Zhang, P., Wang, C., Lu, H., et al. (2019). Determining the optimal N input to improve grain yield and quality in winter wheat with reduced apparent N loss in the North China plain. Front. Plant Sci. 10:181. doi: 10.3389/fpls.2019.00181
Mălinaş, A., Vidican, R., Rotar, I., Mălinaş, C., Moldovan, C. M., and Proorocu, M. (2022). Current status and future prospective for nitrogen use efficiency in wheat (Triticum aestivum L.). Plan. Theory 11:217. doi: 10.3390/plants11020217
Mazzilli, S. R., Kemanian, A. R., Ernst, O. R., Jackson, R. B., and Piñeiro, G. (2015). Greater humification of belowground than aboveground biomass carbon into particulate soil organic matter in no-till corn and soybean crops. Soil Biol. Biochem. 85, 22–30. doi: 10.1016/j.soilbio.2015.02.014
Mori Alvez, C., Perdomo Varela, C., González Barrios, P., Bentos Guimaraes, A., and del Pino Machado, A. (2024). Lupine cultivation affects Soil’s P availability and nutrient uptake in four contrasting soils. Agronomy 14:389. doi: 10.3390/agronomy14020389
Mosier, S., Apfelbaum, S., Byck, P., Calderon, F., Teague, R., Thompson, R., et al. (2021). Adaptive multi-paddock grazing enhances soil carbon and nitrogen stocks and stabilization through mineral association in southeastern U.S. grazing lands. J. Environ. Manag. 288:112409. doi: 10.1016/j.jenvman.2021.112409
Mulvaney, R. L. (2018). “Nitrogen-inorganic forms” in Methods of soil analysis, part 3: Chemical methods (Hoboken, NJ: John Wiley & Sons), 1123–1184.
Novelli, L. E., Caviglia, O. P., and Piñeiro, G. (2017). Increased cropping intensity improves crop residue inputs to the soil and aggregate-associated soil organic carbon stocks. Soil Tillage Res. 165, 128–136. doi: 10.1016/j.still.2016.08.008
Nurbekov, A., Kosimov, M., Islamov, S., Khaitov, B., Qodirova, D., Yuldasheva, Z., et al. (2024). No-till, crop residue management and winter wheat-based crop rotation strategies under rainfed environment. Front. Agron. 6:1453976. doi: 10.3389/fagro.2024.1453976
Page, K. L., Dang, Y. P., and Dalal, R. C. (2020). The ability of conservation agriculture to conserve soil organic carbon and the subsequent impact on soil physical, chemical, and biological properties and yield. Front. Sustain. Food Syst. 4:31. doi: 10.3389/fsufs.2020.00031
Pañella, P. G., Guido, A., Jaurena, M., Cardozo, G., and Lezama, F. (2022). Fertilization and overseeding legumes on native grasslands leads to a hardly reversible degraded state. Appl. Veg. Sci. 25:12693. doi: 10.1111/avsc.12693
Peoples, M. B., Hauggaard-Nielsen, H., and Jensen, E. S. (2015). “The potential environmental benefits and risks derived from legumes in rotations” in Nitrogen fixation in crop production (Hoboken, NJ: Wiley), 349–385.
Peterson, C. A., Deiss, L., and Gaudin, A. C. M. (2020). Commercial integrated crop-livestock systems achieve comparable crop yields to specialized production systems: a meta-analysis. PLoS One 15:e0231840. doi: 10.1371/journal.pone.0231840
Pinto, P., Rubio, G., Gutiérrez, F., Sawchik, J., Arana, S., and Piñeiro, G. (2021). Variable root:shoot ratios and plant nitrogen concentrations discourage using just aboveground biomass to select legume service crops. Plant Soil 463, 347–358. doi: 10.1007/s11104-021-04916-x
Pittelkow, C. M., Liang, X., Linquist, B. A., Van Groenigen, L. J., Lee, J., Lundy, M. E., et al. (2015a). Productivity limits and potentials of the principles of conservation agriculture. Nature 517, 365–368. doi: 10.1038/nature13809
Pittelkow, C. M., Linquist, B. A., Lundy, M. E., Liang, X., van Groenigen, K. J., Lee, J., et al. (2015b). When does no-till yield more? A global meta-analysis. F. Crop. Res. 183, 156–168. doi: 10.1016/j.fcr.2015.07.020
Pravia, M. V., Kemanian, A. R., Terra, J. A., Shi, Y., Macedo, I., and Goslee, S. (2019). Soil carbon saturation, productivity, and carbon and nitrogen cycling in crop-pasture rotations. Agric. Syst. 171, 13–22. doi: 10.1016/j.agsy.2018.11.001
Quemada, M., and Lassaletta, L. (2024). Fertilizer dependency: a new indicator for assessing the sustainability of agrosystems beyond nitrogen use efficiency. Agron. Sustain. Dev. 44:44. doi: 10.1007/s13593-024-00978-0
Rhine, E. D., Mulvaney, R. L., Pratt, E. J., and Sims, G. K. (1998). Improving the Berthelot reaction for determining ammonium in soil extracts and water. Soil Sci. Soc. Am. J. 62, 473–480. doi: 10.2136/sssaj1998.03615995006200020026x
Romano, I., Bodenhausen, N., Basch, G., Soares, M., Faist, H., Trognitz, F., et al. (2023). Impact of conservation tillage on wheat performance and its microbiome. Front. Plant Sci. 14:1211758. doi: 10.3389/fpls.2023.1211758
Rovira, P., Ayala, W., Terra, J., García-Préchac, F., Harris, P., Lee, M. R. F., et al. (2020). The ‘Palo a pique’ long-term research platform: first 25 years of a crop–livestock experiment in Uruguay. Agronomy 10:441. doi: 10.3390/agronomy10030441
Rubio, V., Diaz-Rossello, R., Quincke, J. A., and van Es, H. M. (2021a). Quantifying soil organic carbon’s critical role in cereal productivity losses under annualized crop rotations. Agric. Ecosyst. Environ. 321:107607. doi: 10.1016/j.agee.2021.107607
Rubio, V., Núñez, A., Berger, A., and van Es, H. (2025). Biomass inputs drive agronomic management impacts on soil health. Agric. Ecosyst. Environ. 378:109316. doi: 10.1016/j.agee.2024.109316
Rubio, V., Quincke, A., and Ernst, O. (2021b). Deep tillage and nitrogen do not remediate cumulative soil deterioration effects of continuous cropping. Agron. J. 113, 5584–5596. doi: 10.1002/agj2.20927
Rubio, V., Sawchik, J., and van Es, H. (2022). Soil health benefits from sequence intensification, fertilization, and no-tillage in annual cropping systems. Soil Secur. 9:100074. doi: 10.1016/j.soisec.2022.100074
Salvo, L., Hernández, J., and Ernst, O. (2010). Distribution of soil organic carbon in different size fractions, under pasture and crop rotations with conventional tillage and no-till systems. Soil Tillage Res. 109, 116–122. doi: 10.1016/j.still.2010.05.008
Shaheb, M. R., Venkatesh, R., and Shearer, S. A. (2021). A review on the effect of soil compaction and its management for sustainable crop production. J. Biosyst. Eng. 46, 417–439. doi: 10.1007/s42853-021-00117-7
Sieling, K., and Kage, H. (2021). Apparent fertilizer N recovery and the relationship between grain yield and grain protein concentration of different winter wheat varieties in a long-term field trial. Eur. J. Agron. 124:126246. doi: 10.1016/j.eja.2021.126246
Stanley, P. L., Wilson, C., Patterson, E., Machmuller, M., and Cotrufo, M. F. (2024). Ruminating on soil carbon: applying current understanding to inform grazing management. Glob. Chang. Biol. 30:e17223. doi: 10.1111/gcb.17223
Sun, L., Xia, Z., Sang, C., Wang, X., Peng, B., Wang, C., et al. (2019). Soil resource status affects the responses of nitrogen processes to changes in temperature and moisture. Biol. Fertil. Soils 55, 629–641. doi: 10.1007/s00374-019-01379-2
Taylor, K. M., Nelsen, T. S., Scow, K. M., and Lundy, M. E. (2024). No-till annual wheat increases plant productivity, soil microbial biomass, and soil carbon stabilization relative to intermediate wheatgrass in a Mediterranean climate. Soil Tillage Res. 235:105874. doi: 10.1016/j.still.2023.105874
Teague, R., and Kreuter, U. (2020). Managing grazing to restore soil health, ecosystem function, and ecosystem services. Front. Sustain. Food Syst. 4:534187. doi: 10.3389/fsufs.2020.534187
Wang, Y., Peng, Y., Lin, J., Wang, L., Jia, Z., and Zhang, R. (2023a). Optimal nitrogen management to achieve high wheat grain yield, grain protein content, and water productivity: a meta-analysis. Agric. Water Manag. 290:108587. doi: 10.1016/j.agwat.2023.108587
Wang, Y., Wu, P., Qiao, Y., Li, Y., Liu, S., Gao, C., et al. (2023b). The potential for soil C sequestration and N fixation under different planting patterns depends on the carbon and nitrogen content and stability of soil aggregates. Sci. Total Environ. 897:165430. doi: 10.1016/j.scitotenv.2023.165430
Yin, X., Song, Z., Shi, S., Bai, Z., Jiang, Y., Zheng, A., et al. (2024). Developments and prospects of multiple cropping in China. Farming Syst. 2:100083. doi: 10.1016/j.farsys.2024.100083
Keywords: wheat productivity, N use efficiency, soil N dynamic, no-till systems, residues quality
Citation: Mori Alvez C, Ernst Benech O, González Barrios P and Perdomo Varela C (2024) Wheat productivity and nitrogen use efficiency in no-till systems: a comparative analysis of crop-pasture and continuous cropping rotations in Uruguay. Front. Sustain. Food Syst. 8:1460734. doi: 10.3389/fsufs.2024.1460734
Edited by:
Prabhu Govindasamy, National Research Centre for Banana (ICAR), IndiaReviewed by:
Zina Flagella, University of Foggia, ItalyMuhammad Saqlain Zaheer, Khwaja Fareed University of Engineering and Information Technology (KFUEIT), Pakistan
Copyright © 2024 Mori Alvez, Ernst Benech, González Barrios and Perdomo Varela. This is an open-access article distributed under the terms of the Creative Commons Attribution License (CC BY). The use, distribution or reproduction in other forums is permitted, provided the original author(s) and the copyright owner(s) are credited and that the original publication in this journal is cited, in accordance with accepted academic practice. No use, distribution or reproduction is permitted which does not comply with these terms.
*Correspondence: Cristina Mori Alvez, Y3Jpc3RpbmFtb3JpQGZhZ3JvLmVkdS51eQ==