- 1AgroBioSciences Department (AgBs), College for Agriculture and Environmental Science (CAES), Mohammed VI Polytechnic University (UM6P), Benguerir, Morocco
- 2National Agricultural Research Institute (INRA), Tangier, Morocco
- 3Algal Biotechnology Center, MAScIR, Mohamed VI Polytechnic University, Benguerir, Morocco
Agriculture plays a pivotal role in Africa, contributing significantly to sustainable farming practices and the establishment of resilient food systems. Within this context, the use of various types of biostimulants, including microbial biostimulants such as Plant Growth-Promoting microorganisms (PGPM) and non-microbial products like Algal extract, humic acid, and protein hydrolysates, as well as biopesticides, emerges as a promising strategy to bolster sustainable agriculture, particularly in the realm of organic berry production. These substances have the potential to enhance crop growth, fortify stress tolerance, and optimize nutrient absorption, benefiting both human health and the environment. This paper aims to explore the opportunities and challenges associated with incorporating plant biostimulants into organic berry production within the African agricultural sector. To achieve this objective, an extensive and comprehensive review encompassing scientific literature, policy documents, and global data was conducted. The primary focus of this review was to investigate the current state of biostimulant adoption in organic berry farming within the African agricultural sector, with a specific emphasis on identifying potential opportunities and discussing the benefits derived from their application. Additionally, we addressed the challenges encountered and proposed practical approaches to achieving sustainable agriculture. The findings and conclusions of our review reveal the transformative potential of biostimulants in organic berry production. The evidence points to remarkable advancements in plant growth, plant health, overall yield, and fruit nutritional quality. By implementing these substances, we can also minimize the ecological footprint of agricultural practices. However, several challenges remain, including limited accessibility, insufficient awareness and knowledge regarding biostimulant usage, and a shortage of research specific to African agriculture. To overcome these challenges and achieve sustainable agriculture, this paper recommends practical approaches such as raising awareness, investing in research and development, and promoting the use of biostimulants through policy interventions and capacity-building programs. We underscore the importance of stakeholder participation and local adaptations for effectively integrating biostimulants in African agriculture. The significance of integrating plant biostimulants in organic berry production lies in advancing sustainable agriculture. This paper aims to explore the opportunities and challenges associated with incorporating plant biostimulants into organic berry production within Africa.
1 Introduction
To sustain the projected 9.7 billion global population by 2050, crops must experience a significant boost in productivity, estimated at 60–100%. However, accomplishing this goal while preserving the integrity of the agricultural system presents a challenge (Coronado-apodaca et al., 2022; Rodrigues et al., 2022; Smith et al., 2015). Agriculture holds immense significance in Africa, serving as a crucial driver of economic and social development. It serves as a cornerstone, supplying food, income, and employment opportunities for millions of individuals continent-wide (Godoy et al., 2014; Rodrigues et al., 2022). Technology has improved agricultural productivity and product quality, but it also faces challenges such as soil degradation, pests/diseases, and its impact on human health (Xu et al., 2018; Tripathi et al., 2022).
In recent decades, consumers have become increasingly conscious of the food they consume, with a greater focus on health, ethics, and environmental impact. The agricultural industry has responded to these demands by shifting toward sustainability, with an emphasis on reducing the industry’s impact on the environment and promoting the growth of high-value-added crops such as berries (Pylak et al., 2019; Rodrigues et al., 2022; Smith et al., 2015). Afterward, incorporating berry crops in organic farming can yield many benefits for farmers, consumers, and the environment (Muller et al., 2017; Sangiorgio et al., 2021). Ecologically clean or organic berries are based on the research and development of safe methods, to increase productivity while maintaining quality (Baez-Rogelio et al., 2017; Gomiero et al., 2011; Peigné et al., 2022).
In this context, the African continent’s rich diversity of climates and soil types offers immense potential for producing a wide variety of organic berries. Moreover, organic berry production in Africa can significantly contribute to food security and economic development. However, it often faces limitations due to various stressors that can negatively impact yields and fruit quality (Coronado-apodaca et al., 2022; Godoy et al., 2014). Therefore, it is essential to identify and implement innovative approaches to enhance the productivity and sustainability of organic berry production in Africa, while also maintaining or improving the nutritional and sensory qualities of the fruit. Furthermore, a deep understanding of how pre-harvest factors influence the quality of berry fruits could lead to the adoption of best practices that markedly improve overall fruit quality (Di Vittori et al., 2018; Sangiorgio et al., 2021).
Using biopreparations such as biopesticides and biostimulants derived from natural sources holds great promise for improving the productivity and sustainability of berries in Africa. Primarily, biopesticides represent a class of pest management solutions derived from natural origins such as animals, plants, bacteria, and certain minerals. These products find application in agriculture for pest and disease control. Distinguished by their reduced environmental impact compared to synthetic pesticides, biopesticides are designed to target specific pests while posing minimal risk to non-target organisms and ecosystems. They are characterized by their rapid degradation and may encompass substances like pheromones, microbial agents, plant extracts, and genetically modified organisms. Biopesticides serve to diminish pesticide residues in agricultural produce, foster sustainable agricultural practices, and offer safer pest control alternatives that safeguard human health and environmental well-being (Baker et al., 2020; Booth et al., 2022; Godlewska et al., 2021). On a similar note, plant biostimulants, as defined by du Jardin (2015), constitute substances or microorganisms applied to plants with the aim of enhancing nutrient efficiency, stress resilience, and overall crop quality attributes, irrespective of their nutrient content. These biostimulants, in particular, are gaining popularity due to their effectiveness in increasing crop production, specifically in challenging conditions. They can include compounds such as seaweed extracts, humic substances, amino acids, as well as beneficial microorganisms like plant growth promoting rhizobacteria (PGPR) and arbuscular mycorrhizal fungi (AMF) (Celiktopuz et al., 2020; Jiménez-Arias et al., 2021; Lau et al., 2022; Zulfiqar et al., 2024). Multiple studies have demonstrated that biostimulants can significantly boost the yields of berry crops, including strawberries, blueberries, and raspberries. This increase is often credited to improved water use efficiency and nutrient uptake, along with reduced losses from diseases and pests. Biostimulants are also known to enhance fruit quality by improving attributes like size, shape, and color. Furthermore, they can increase the concentrations of beneficial compounds such as antioxidants and vitamins (Baez-Rogelio et al., 2017; Egamberdieva et al., 2018). This approach aligns with the principles of organic agriculture, which focuses on natural inputs to improve crop growth and soil health, leading to synergistic effects on the crops and reduced reliance on synthetic inputs (Jindo et al., 2022; Rouphael and Colla, 2020; Sharma et al., 2014). Despite potential benefits, the adoption of these biopreparations in organic berry production in Africa is limited, and challenges need to be addressed to promote their wider use (Baez-Rogelio et al., 2017; Jindo et al., 2022). Low knowledge and awareness among berry farmers and stakeholders regarding biopreparations is a significant issue, particularly for small-scale farmers who face limited access to information and support services (Jindo et al., 2022).
This research topic comprises an aggregation of numerous scientific contributions focusing on the utilization of plant biostimulants across diverse berry crops. The study delves into the physiological, cellular, and molecular mechanisms that govern the interactions between berry plants and biostimulants across varying management practices and environmental contexts. It offers valuable insights into the operational dynamics of microbial and non-microbial biostimulants, with implications extending to commercial enterprises, the scientific community, and extension specialists. By unraveling the agricultural significance of biostimulants—including their roles in enhancing quality, optimizing nutrient utilization efficiency, and improving stress tolerance—researchers are poised to pioneer a new wave of biostimulants. These innovative products can be custom-tailored to leverage synergistic effects and complementary mechanisms, ultimately assisting African farmers in surmounting their distinct agricultural challenges.
2 Overview on the status of organic farming in Africa
In pursuit of sustainable production systems, organic agriculture focuses on conserving and reusing resources (Song et al., 2023). It employs natural methods and materials for pest control, soil fertility, and animal health, while also avoiding synthetic chemicals and GMOs (Coronado-apodaca et al., 2022; Wenhai et al., 2019; Wittwer et al., 2021). Organic production methods are expected to reduce reliance on fossil fuel inputs. In Africa, organic farming has gained popularity as a means to improve food security, increase small-scale farm profitability, and alleviate the environmental impact of agriculture. However, challenges such as low soil fertility and pest pressure, the lack of governmental support, institutionalization, and access to information and resources, are among the critical factors affecting the optimal extension of organic food system in Africa (Bettiol et al., 2004; Rodrigues et al., 2022; Thi et al., 2021).
In 2020, organic agricultural land worldwide reached 74.9 million ha, with Oceania having the most land at 35.9 million ha, followed by Europe, Latin America, Asia, Northern America, and Africa. There were over 3.4 million organic producers globally, with almost 91% in Asia, Africa, and Europe. India ranked first with the highest number of organic producers, followed by Ethiopia and Tanzania. In Africa, organic agricultural land surpassed 2 million ha, representing 0.2% of total agricultural land and 2.8% of global organic agricultural land. Organic agriculture is practiced in 35 African countries, with Tunisia, Ethiopia, and Sierra Leone being the leading countries. These countries are leaders in organic farming due to a conducive climate, high demand for organic products, and government support. African organic farmers primarily focus on growing coffee, cocoa, tea, and horticultural products such as vegetables, fruits, and herbs. Over 60% of Africa’s organic exports are coffee (Willer et al., 2020).
Tunisia and Ethiopia have made notable contributions to the advancement of organic farming in Africa (FAOSTAT, 2019). Tunisia boasts the most extensive t certified organic land area in the Arab world and Africa, covering 336,000 hectares and ranking 23rd globally (ProFound Advisers in Development, Organics and Development, Markus Arbenz, 2020). The Tunisian government has implemented more than 20 decrees and orders and established several structures and institutions to establish a comprehensive framework for the organic agricultural sector. Notably, organic product exports have witnessed a significant growth from 7 million euros in 2004 to nearly 240 million euros in 2018. Tunisia supports organic production through subsidies, leveraging its substantial olive oil production capacities (Willer et al., 2020). Similarly, Ethiopia has provided financial support and technical assistance to organic farmers. The number of certified organic farms has risen, indicating a growing demand for organic products both locally and internationally (UNCTAD, 2019).
Organic agriculture is well-suited for small-scale, labor-intensive African farming systems that utilize local resources and serve a variety of needs (Bettiol et al., 2004; Kahu et al., 2010). Studies confirm the benefits of organic farming in Africa, including reduced health risks, enhanced resource conservation, agricultural resilience, increased income, and market stability (Xu et al., 2018; Conti et al., 2014; Wittwer et al., 2021). This manual labor-reliant method thrives in countries with significant agricultural workforces, offering improved food security, local food access, land restoration, climate resilience, and biodiversity protection. Organic farming plays a crucial role in poverty alleviation, market access, and affordability (Altaf et al., 2019; Backer et al., 2018; Kirchmann et al., 2016; Thi et al., 2021).
In the specific context of Africa, organic farming faces a range of challenges: adapting to diverse climates, managing pests organically, accessing expensive inputs, obtaining certification, addressing water scarcity, and ensuring farmer education. By implementing innovative solutions and tailored support, organic berry farming can thrive, enhancing sustainability and productivity within the African agricultural sector (Figure 1). In the upcoming section, we will examine why organic berry farming is vital in Africa and how it can enhance the sustainability and productivity of the agricultural sector.
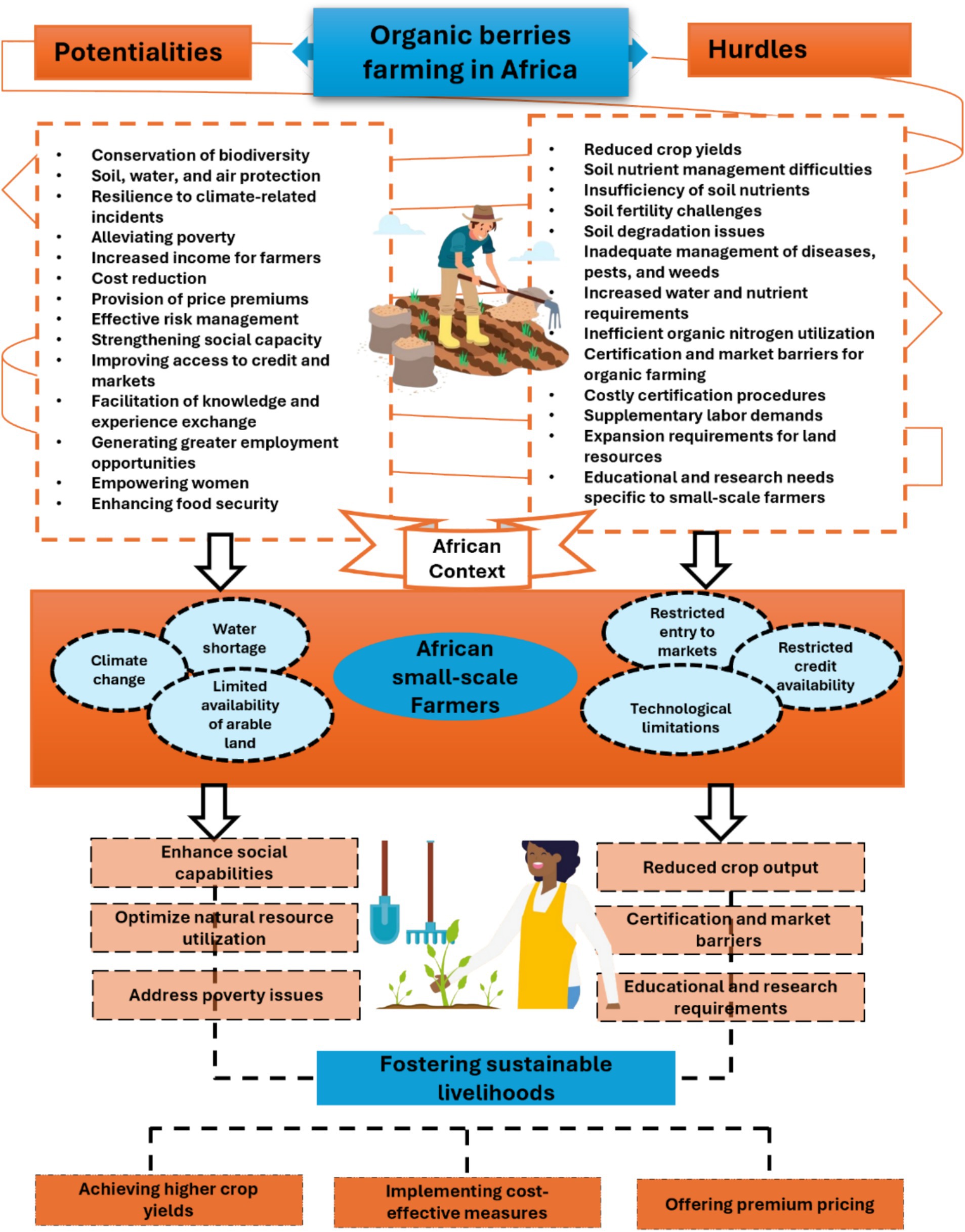
Figure 1. A framework for evaluating the potentialities and hurdles of organic berry farming for small-scale farmers in Africa.
3 Why organic berries?
The global berries market reached a value of 233.69 billion in 2023 and is expected to grow at a CAGR of 5.5% from 2024 to 2032, reaching nearly 378.63 billion by 2032 (Expert Market Research, 2024). Consumer awareness and increasing purchasing power play vital roles in driving the growth of the berries market (Mazzoni et al., 2016; Romanazzi and Feliziani, 2016). As consumers become more informed about the health benefits of berries, their demand has risen (Vidovic, 2018). The expansion of retail outlets, such as supermarkets and convenience stores, has led to improved availability and accessibility of both fresh and processed berries, further bolstering market growth. Additionally, the surge in popularity of superfoods, attributable to the demanding lifestyles of the modern working population, has contributed to the increased consumption of berries. Manufacturers have responded to this trend by incorporating berries into various convenience foods and beverages, thereby enhancing their nutritional profiles and stimulating market expansion (Mattila et al., 2006). Moreover, berries are favored as a convenient and healthy snacking option due to their appealing taste and ease of consumption, aligning with the prevailing trend of health-conscious snacking. Consequently, the rising demand for berries as snacks has played a significant role in propelling the overall growth trajectory of the berries industry (Rana and Paul, 2017; Senger et al., 2022; Sobekova et al., 2011).
The berries market exhibits natural segmentation into two distinct categories: organic and conventional, based on the nature of the products from a typological perspective, the industry encompasses diverse categories, including strawberry, cranberry, blueberry, and others (Bilawal et al., 2021; Mazzoni et al., 2016; Romanazzi and Feliziani, 2016). In terms of applications, the market can be classified into various sectors such as food and beverages, personal care and cosmetics, pharmaceuticals, dietary supplements, and more (Figure 2). Distribution channels within the industry are categorized into direct and indirect channels (Expert Market Research, 2024).
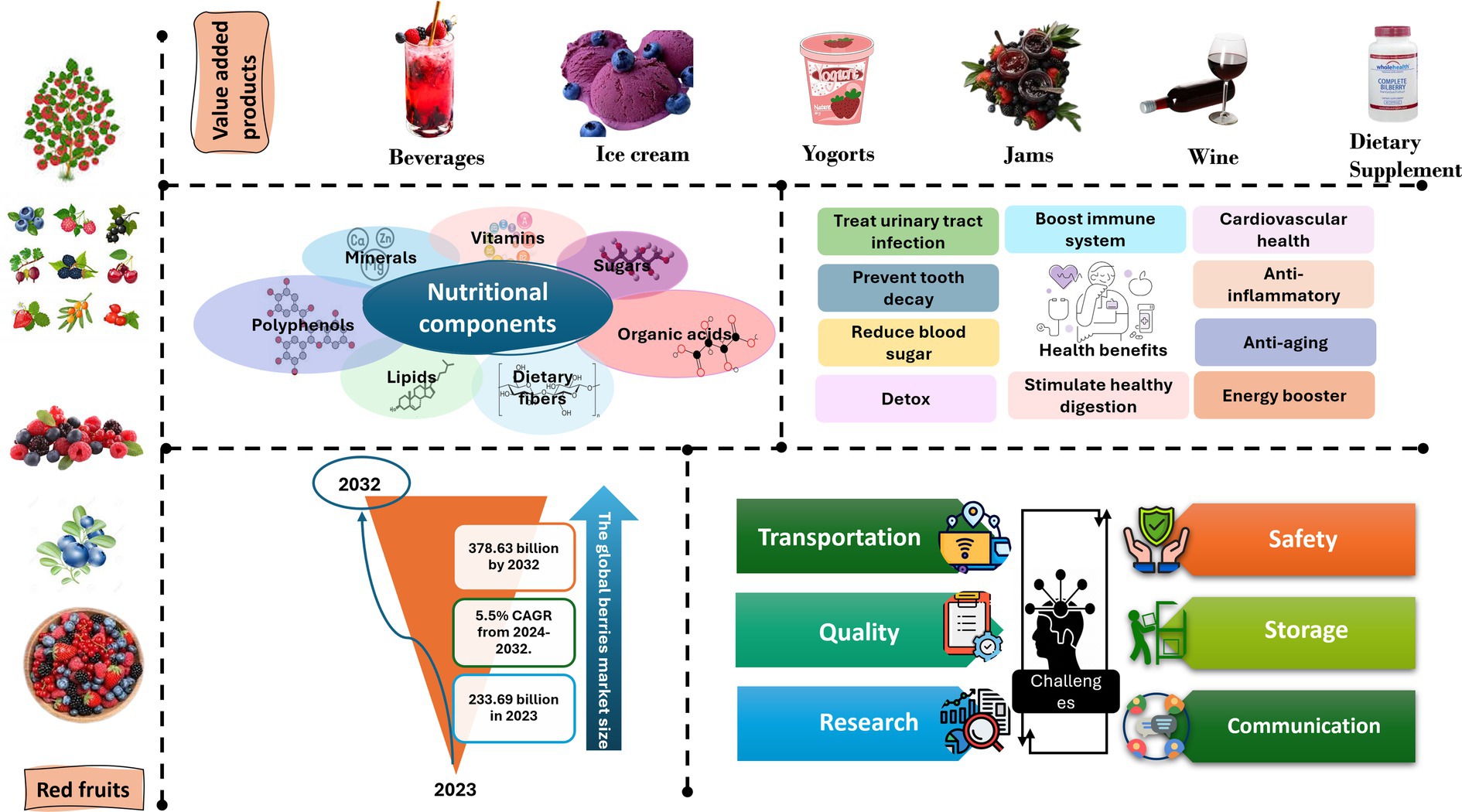
Figure 2. Comprehensive examination of berries’ value-added products, nutritional components, health benefits, global market size, and challenges.
Recent advancements in research have greatly contributed to the expansion of the market by enhancing our scientific knowledge regarding the health advantages associated with berries. Berries not only promote overall well-being but also serve as a preventive measure against a range of chronic diseases such as cancer, neurodegenerative disorders, and heart conditions. Additionally, the growing inclination toward consuming foods with medicinal properties to prevent illnesses has also played a significant role in driving market growth. Major companies introducing new varieties and flavors of berries have further bolstered the industry’s progress. The rising popularity of superfoods, including leafy vegetables, berries, grapes, nuts, and seeds, has made a substantial contribution to the market’s expansion (Bilawal et al., 2021; Horvitz, 2017; Petriccione et al., 2015; Raspberry et al., 2010; Romanazzi and Feliziani, 2016). Berries have become a common ingredient in various food items like muffins and pancakes. Furthermore, the globalization of dietary preferences has also contributed to the market’s growth (Figure 2).
The market is poised for growth due to the prevailing trend of clean labels and whole foods (Basha and Lal, 2019; Poore and Nemecek, 2018). As consumers increasingly prioritize natural, authentic, and minimally processed food choices, there is a significant upswing in the demand for berries. This heightened demand serves as a pivotal driver for the expansion of the market (Mazzoni et al., 2016; Romanazzi and Feliziani, 2016). Berries like blackberry (Pérez-Gallardo et al., 2015), bilberry (Karppinen et al., 2016), blackcurrant (Dubin et al., 2017), blueberry (Caspersen et al., 2016), cranberry (Salo et al., 2021), raspberry (Rom et al., 2010), and strawberry (Rom et al., 2010), are popular due to their high phenolic compound content, which provides antioxidant, antibacterial, anti-inflammatory, anticancer, and cardioprotective properties (Bilawal et al., 2021; Horvitz, 2017; Petriccione et al., 2015; Romanazzi and Feliziani, 2016). Berries also contain high levels of sugars and organic acids that affect their taste, and changes in their composition can impact fruit quality (Hidalgo and Almajano, 2017; Skrovankova et al., 2015). Furthermore, berries are rich in antioxidant phenolics, including flavonoids, stilbenes, tannins, and phenolic acids, as well as flavonols such as quercetin, kaempferol, and myricetin and their derivatives. These compounds have potential health benefits as dietary antioxidants (Maksimovic et al., 2011; Skrovankova et al., 2015). The association of anthocyanins with anti-cancer and anti-cardiovascular disease properties and their recognition as the most significant health-promoting compounds in berries has been widely reported (López et al., 2021; Paredes-López et al., 2010; Vidovic, 2018).
There is a notable surge in the demand for organic food among health-conscious consumers, which is significantly driving the consumption of organic fresh berries. These berries are sought after for their rich nutritional composition. Additionally, the escalating demand for organic berries in processed food items, fueled by enhancing disposable incomes, is providing a substantial impetus to the market’s growth. The adoption of organic farming methods, which prioritize natural inputs and pest control, has gained widespread recognition due to its potential to yield high-quality fruits while minimizing environmental impact (Ersoy et al., 2011; Ochmian et al., 2015; Sablani et al., 2011). The adoption of organic farming techniques has unveiled a host of benefits, including a delightful enhancement in fruit quality, a gentle ecological footprint, and the alluring prospect of commanding higher market prices, surpassing those of conventional practices (Conti et al., 2014). Intriguing research findings have unveiled an intriguing dimension, showcasing the intricate relationship between agricultural systems and the application of phytochemicals to crops, as wonderfully illustrated in Table 1. Moreover, the cultivation methods employed have been discovered to bestow upon berry fruits their unique qualities, presenting an enchanting tapestry of flavors and textures (Anjos et al., 2020; Ochmian et al., 2015).
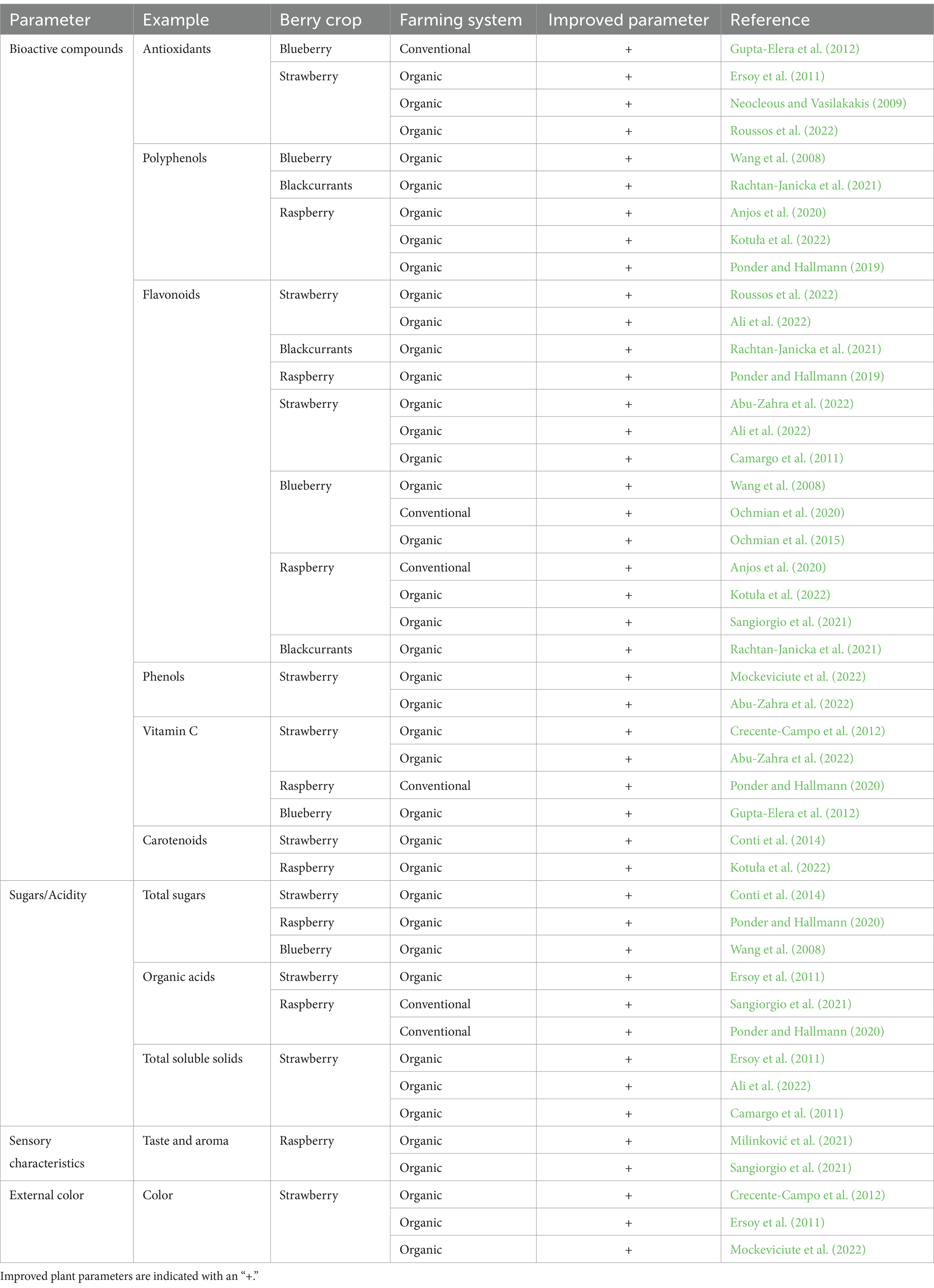
Table 1. A comprehensive review of multiple studies comparing the phytochemical contents in berry crops from both organic and conventional production methods.
Multiple studies have demonstrated that organic berries exhibit a higher concentration of beneficial phytochemicals when compared to conventionally grown counterparts (Table 1). Organic blueberries and blackcurrants exhibited a greater polyphenol content compared to those grown conventionally (Rachtan-Janicka et al., 2021; Wang et al., 2008). Similarly, organically produced raspberries exhibit significantly higher levels of polyphenols (Anjos et al., 2020; Kotuła et al., 2022). Compared to conventionally grown berries, organic cultivation of strawberries, blueberries, and raspberries resulted in notable increases in key bioactive compounds. These compounds include antioxidants, flavonoids, anthocyanins, ascorbic acid, carotenoids, phenols, total sugars, total soluble solids, and organic acids, as evidenced by the findings summarized in Table 1.
Marketable berries are primarily evaluated based on their visual properties, focusing on fruit size and hue. This emphasis is due to the belief that the color of berries is a crucial factor in determining their overall quality, and it is often the first aspect consumers consider. Studies have shown a positive correlation between berry color, antioxidant capacity (particularly anthocyanins and ascorbic acid), and nutritional value. Organic berries typically have darker and less vivid hues, with a tendency toward a redder tone. An attractive red color from anthocyanins is considered one of the strawberries’ most important quality traits (Crecente-Campo et al., 2012; Ersoy et al., 2011; Mockeviciute et al., 2022).
While some researchers argue in favor of organic farming techniques, others remain skeptical of any significant differences between the two cultivation methods. Some studies suggest that certain bioactive compounds, such as anthocyanins, vitamin C, and organic acid levels, may decrease in organically grown berries compared to conventionally cultivated ones (Ochmian et al., 2020; Ponder and Hallmann, 2020; Sangiorgio et al., 2021). However, organic cultivation of berries offers various environmental benefits. Organic farming methods minimize the use of synthetic pesticides and fertilizers, which can negatively impact soil and water quality (Kirchmann et al., 2016). Organic farming practices prioritize soil health, which is critical for producing high-quality products (Caspersen et al., 2016). Studies indicate that organic farming methods can enhance soil fertility by increasing soil-beneficial microorganisms diversity and bolstering the soil’s resilience to stress (Milinković et al., 2021; Mokrani and Nabti, 2022). The following section will examine the different agricultural techniques used in organic berry farming and their potential implementation in the African context to boost organic berry production. The analysis of these practices will provide valuable insights into how to foster sustainable and productive organic berry cultivation in Africa.
4 Agri-practices in organic berries-based food systems
4.1 Microbial biostimulants
The use of microbial biostimulants in berry production has expanded significantly in recent decades (Calvo et al., 2014; Zulfiqar et al., 2024). Reports suggest that the use of these eco-friendly solutions can promote plant growth through various physiological processes, including nutrient uptake and better root development (Calvo et al., 2014) (Figure 3).
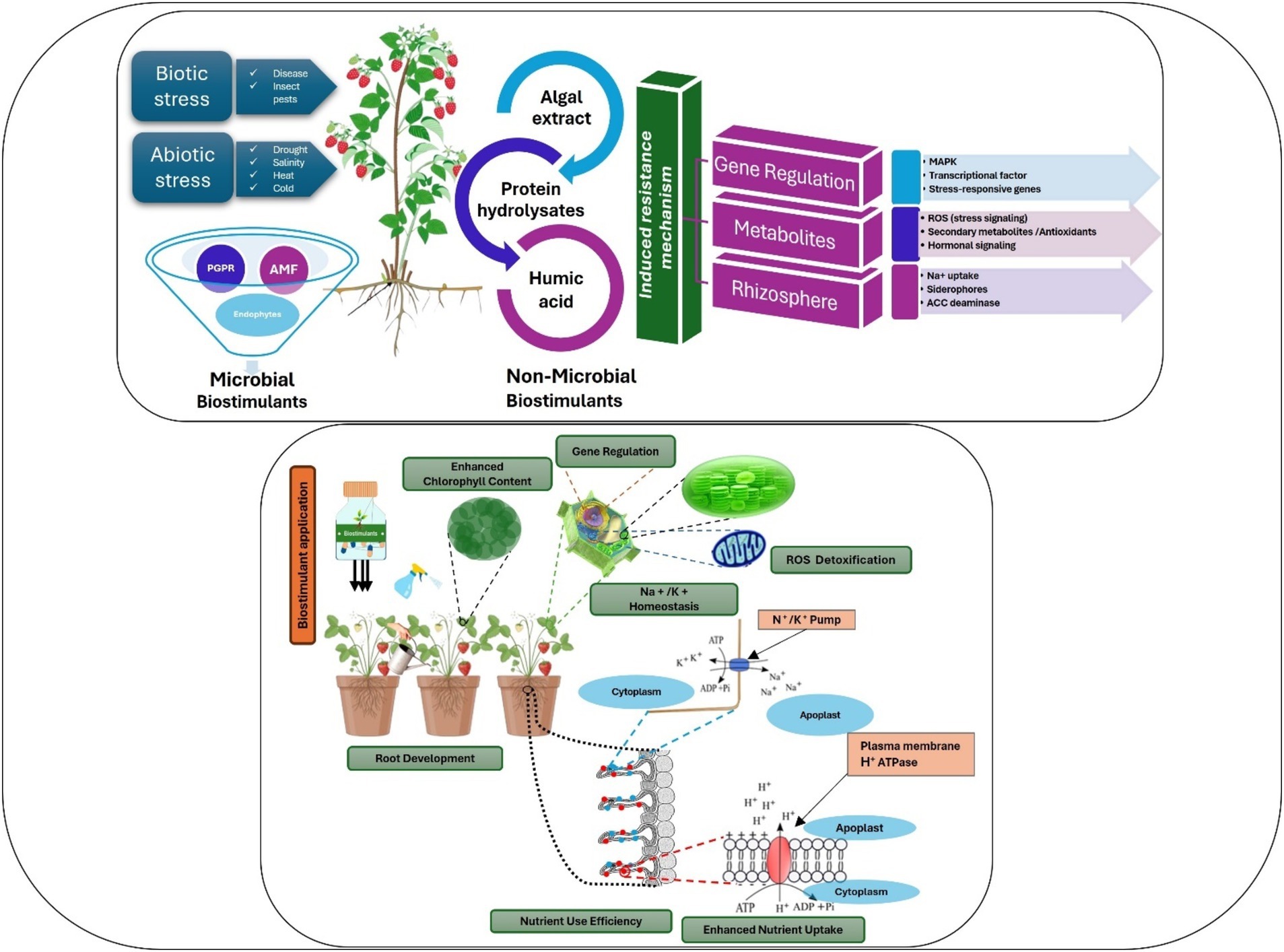
Figure 3. Targeting key metabolic processes by microbial and non-microbial biostimulants at whole-plant, physiological, and molecular levels.
4.1.1 Plant growth, yield, and nutrient uptake
The application of microbial inoculants is commonly associated with beneficial outcomes, including enhanced nutrient uptake, improved nutrient status in berry crops, and subsequently, augmented growth and yield. Numerous genera of plant growth-promoting rhizobacteria (PGPR) possess the capability to fix atmospheric nitrogen, exemplified by Beijerinckia spp. (Esitken et al., 2003), Klebsiella pneumoniae (PeŠaković et al., 2013), Pantoea agglomerans (Xie et al., 2017), Azotobacter spp. (Rueda et al., 2016), and Azospirillum spp. (Pedraza et al., 2010). However, Azospirillum spp. is the most studied among all these genera (Kapoore et al., 2021), with frequent documentation of nitrogen fixation in a variety of berry crops (Guerrero-molina et al., 2012).
Plant growth promoting rhizobacteria strains have exhibited remarkable potential in enhancing plant growth, yield, and nutrient composition across a range of berry crops, including strawberries, mulberries, raspberries, and blueberries (Table 2). Notably, the utilization of Azospirillum spp. and Azotobacter spp. in hydroponic systems has resulted in improvements in growth, yield, leaf nitrogen concentration, and soluble solids content in strawberries, attributed to mechanisms like nitrogen fixation and enhanced mineral uptake (Kapoore et al., 2021; Rueda et al., 2016; Saritha and Tollamadugu, 2019). Azotobacter spp. offers additional advantages, including phytohormone production, stress alleviation, degradation of pesticides and oil globules, as well as metabolization of heavy metals (Tripathi et al., 2022; Yadav et al., 2010). Moreover, Bacillus tequilensis has been reported to promote plant growth in mulberry plants, as demonstrated by Xu et al. (2018).
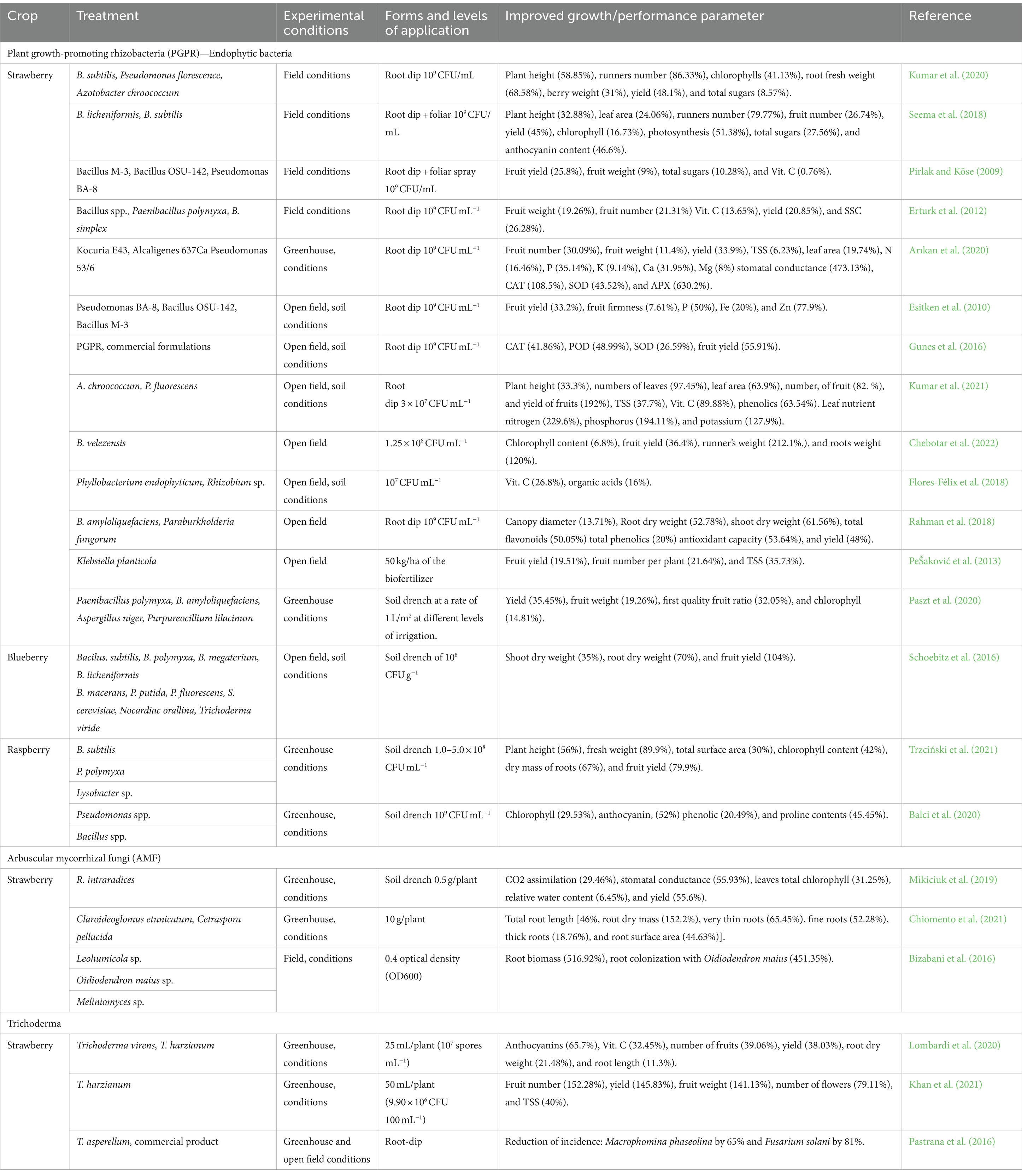
Table 2. Overview of literature reporting the stimulation of growth through microbial biostimulants.
Other soil microbes can improve plant nutrient uptake by increasing the solubilization of soil nutrients. Poor soil availability of absorbable forms of phosphorus (P) is a significant issue for agricultural systems, even with the addition of P fertilizers, as P easily bonds to soil particles and becomes unavailable to plants (Calvo et al., 2014). Bacterial genera such as Pseudomonas spp. (Malusa et al., 2008), Bacillus spp., Burkholderia spp., Streptomyces spp. (Rahman et al., 2018), Achromobacter spp., and Azospirillum spp. (Rueda et al., 2016) were identified as phosphorus solubilizers through mechanisms involving organic acid production and phosphatase synthesis (Vaikuntapu et al., 2014). The process of organic-P solubilization entails the dephosphorylation of phytates through the action of acid phosphatases and phytases, which are produced by microorganisms in the rhizosphere (Cochard et al., 2022). Organic phosphate in soils is mostly present as phytate, which can be microbially solubilized through phytase production (Bona et al., 2015; Kumawat et al., 2023; Tripathi et al., 2016). Additionally, Wang et al. (2022) examined the microbial diversity in blueberry plant rhizosphere soil, isolating iPSB like Buttiauxella and Paraburkholderia. These bacteria exhibited traits such as phosphorus solubilization, auxin production, and nitrogen fixation, indicating their potential to enhance soil for blueberry growth (Wang et al., 2022). Furthermore, soil microorganisms can dissolve potassium minerals present in rocks, such as micas, illite, and orthoclases. This process is accomplished through the excretion of organic acids that facilitate the dissolution of rock-bound potassium or the chelation of silicon ions, thereby increasing the availability of potassium. For instance, Bacillus edaphicus was reported to induce the synthesis of organic acids. These organic acids directly contribute to the dissolution of rock potassium or the chelation of silicon ions, thereby promoting increased potassium uptake (Calvo et al., 2014; Peris-felipo et al., 2020).
Numerous studies have provided evidence of the positive impact of mycorrhizal fungi on plant growth and nutrient uptake. The effects of different mycorrhizal fungi on plant growth can vary, exhibiting specificity toward specific plant cultivars, soil conditions, geographical locations, and sampling periods (Bona et al., 2015; Cai et al., 2021; Guo et al., 2021). By inoculating ericaceous plants with ericoid mycorrhizal (ErM) fungi boosts growth significantly. While natural colonization rates are low (below 15%), deliberate inoculation can raise them to around 30%, particularly benefiting blueberry cultivars. Research highlights the positive effects of ErM fungi on root growth, fresh weights, and overall plant health, with specific inoculations showing notable increases in plant dry weight (Wei et al., 2022). For example, the introduction of Leohumicola, Oidiodendron maius, and Meliniomyces ErM fungal species to blueberry roots resulted in enhanced growth of berry plants, although the specific responses varied among different varieties (Bizabani et al., 2016).
In a similar vein, a field experiment revealed that the application of arbuscular mycorrhizal fungi (AMF) and plant growth-promoting rhizobacteria (PGPR) yielded maximum plant biomass and improved uptake of nutrients such as nitrogen, phosphorus, potassium, calcium, and magnesium (Altaf et al., 2019; Saritha and Tollamadugu, 2019). Furthermore, co-inoculating strawberries with a combination of AMF and/or Pseudomonas fluorescens enhanced flower and fruit production, as well as increasing fruit size, particularly when nitrogen and phosphorus levels were low (Bona et al., 2015; Tripathi et al., 2022). Overall, plant growth is stimulated by ErM fungi through the breakdown of organic nitrogen compounds, establishment of extensive root networks for enhanced nutrient absorption, and promotion of vertical root growth in plants like cranberries. Larger root systems improve nutrient uptake efficiency, while ErM fungi also produce growth-promoting hormones and upregulate genes to bolster plant growth and stress resilience (Wei et al., 2022). However, it should be noted that a study investigating the co-inoculation of strawberries with both AMF and T. harzianum did not show an increase in plant growth compared to inoculation with either organism individually (Malusa et al., 2008). While mycorrhizal fungi generally play a significant role in promoting plant growth and enhancing nutrient uptake, their effects can vary depending on factors such as the specific plant species, soil fertility levels, and the specific types and combinations of mycorrhizal fungi utilized.
4.1.2 Resistance to abiotic stress
The use of microbial biostimulants is an efficient strategy to improve plant resistance to several abiotic stresses (drought, salinity, cold, heat…). In this context, PGPR can improve plant tolerance to drought stress through the production of exopolysaccharides, which improve the soil’s water-holding capacity (Ojuederie et al., 2019; Tripathi et al., 2022; Zulfiqar et al., 2024). As an example, the application of Pseudomonas putida improved drought stress tolerance in chickpea plants. This bacteria achieves this by regulating membrane integrity, promoting the accumulation of osmolytes, and enhancing the scavenging ability of reactive oxygen species (ROS). Additionally, P. putida positively influences stress responses, leading to the differential expression of genes related to ethylene biosynthesis, salicylic acid, jasmonate transcription activation, and antioxidant enzymes (Tiwari et al., 2016). Similarly, the use of microbial biostimulants containing S. kloosii and K. erythromyxa has shown positive results in reducing salinity’s negative effects on strawberries (Karlidag et al., 2013). These microorganisms produce ACC deaminase, which reduces the effects of salt stress by lowering the levels of the plant hormone ethylene. Inoculation with bacteria like Variovorax paradoxus, PGPR-producing ACC, and Bacillus amyloliquefaciens can enhance salt stress tolerance in various plants by improving peroxidase/catalase activity, reducing Na+ levels in the plant, upregulating stress response genes, and causing an increase in photosynthetic rate, balanced ion homeostasis, decreased stomatal resistance, and improved biomass in pea plants, okra, and maize seedlings, respectively (Chen et al., 2016; Habib et al., 2016). Moreover, PGPR can synthesize secondary metabolites and volatile organic compounds (VOCs) that contribute to the enhancement of plant growth and tolerance to stress. These VOCs play a role as signal molecules in inducing systemic tolerance to abiotic stress, such as drought, owing to their unique properties including low molecular weight, low boiling point, and high vapor pressure (Fincheira and Quiroz, 2018).
Arbuscular mycorrhizal fungi improves plant adaptation to drought stress by enhancing water and nutrient uptake, modifying root architecture, and inducing physiological and enzymatic activities related to plant antioxidant responses. AMF’s ability to access inaccessible soil pores boosts plant growth and water-use efficiency, increasing crop yield (Bahadur et al., 2019; Ojuederie et al., 2019; Zhang et al., 2018). AMF-mediated mechanisms involve modifications in plant hormone content in response to drought stress (Gouda et al., 2018; Jajoo, 2021), highlighting their significant role in improving plant resilience to drought stress and their potential to enhance crop productivity in water-limited environments. Several studies demonstrate that inoculation with AMF improves the resistance of berry crops to abiotic stress (Table 2). AMF inoculation has been shown to improve the growth and drought tolerance of strawberry plants (Borkowska, 2002; Moradtalab et al., 2019) and blueberry plants (Gui et al., 2021). However, studies on the interaction between AMF and berries plant are still scarce and further research is needed to determine the optimal AMF species, inoculation methods, rates, and timing for different berry crops and abiotic stress conditions.
Silicon (Si), found abundantly in the earth’s crust, benefits plant growth by forming a protective layer on leaves, improving nutrient uptake efficiency, and aiding in heat stress mitigation. It enhances photosynthesis, reduces cellular damage, and is a safe and effective fertilizer for cultivating strawberries in controlled environments (Etesami and Ryong, 2018; Sato, 2018; Weber et al., 2018). In a study by Jeong (2022), optimized silicon (Si) application methods were employed to bolster the heat resistance of strawberry transplants. The outcomes showcased enhanced growth and stress resilience under high temperatures with Si treatments, especially when applied to daughter plants consistently. Additionally, the research explored the effects of silicon application on Fortuna strawberries grown in diverse substrates with varying iron levels. The findings highlighted improved growth and fruit quality associated with silicon treatments. This suggests that silicon application shows potential for increasing both the yield and quality of Fortuna strawberries, presenting agricultural benefits. Further investigations across various strawberry varieties and dosage levels are needed to obtain a more comprehensive understanding of the impact on yield and fruit shelf-life (Peris-felipo et al., 2020).
4.1.3 Resistance to biotic stress
Biological management is an effective and long-lasting alternative to chemical pesticides in berries cropping systems (Dorais and Alsanius, 2015; Kaiser and Ernst, 2010; Scortichini, 2022). In organic berry farming, biological control is an essential cultural control method that entails the introduction of beneficial insects or microorganisms, giving a sustainable and ecologically acceptable alternative to the use of conventional pesticides (Enebe and Babalola, 2019; Scortichini, 2022; Walters et al., 2013). Several studies have evaluated the efficacy of biological control agents, including Pseudomonas spp., Bacillus spp., Streptomyces spp., Enterobacter spp., and Trichoderma spp., in controlling root and shoot infections in organically cultivated berry crops (Table 2).
Primarily, Bacillus sp. is a well-known biological control agent that can be applied to the surface of berries to create a protective barrier against decay-causing pathogens (Yamamoto et al., 2014). Several research studies have provided evidence of the efficacy of Bacillus subtilis in reducing decay caused by Penicillium expansum, Botrytis cinerea, and Rhizopus stolonifer in strawberries. Furthermore, it has demonstrated effective control fruit mummification and shoot blight in organic blueberries (Dorais and Alsanius, 2015; Pylak et al., 2019; Scortichini, 2022). The antagonistic strain B. amyloliquefaciens can reduce the virulence of strawberry anthracnose caused by Colletotrichum gloeosporioides (Mochizuki et al., 2012). Additionally, Bacillus tequilensis is an endophytic bacterium obtained from a healthy mulberry stem, exhibiting potent antifungal properties against Mulberry Fruit Sclerotiniose (135). For instance, research findings indicate that the colonization of plants by Bacillus amyloliquefaciens enhances their defense response against Rhizoctonia solani through the modulation of phytohormone signaling, maintenance of elicitor levels, production of secondary metabolites, and regulation of reactive oxygen species and ROS scavengers (Boček et al., 2012). Moreover, the inoculation of Cotton plants with Bacillus spp. results in an augmented secretion of gossypol and jasmonic acid, leading to reduced feeding by Spodoptera exigua larvae. Inoculated plants also exhibit higher transcript levels of genes involved in allelochemical and jasmonate synthesis, along with the suppression of the pest (Backer et al., 2018).
Besides, the colonization of plants by PGPR confers other benefits such as improving tissue health and physiological functions, inducing tolerance against southern blight disease, and eliciting induced systemic resistance (ISR) against various pathogens. The activation of pathogenesis-related genes, facilitated by phytohormone signaling pathways and defense regulatory proteins, serves as a key mechanism through which PGPR triggers ISR in plants. Moreover, bacterial signal compounds and microbe-associated molecular triggers can modulate the induction of ISR in plants. Notably, both pathogen cell-surface factors and analogs of salicylic acid and jasmonic acid can effectively elicit ISR and systemic acquired resistance (SAR) in plants. Lastly, within root exudates, there are chemical components that mimic AHL signals, promoting beneficial associations in the rhizosphere while inhibiting the proliferation of pathogenic bacteria (Backer et al., 2018; Lowe et al., 2012; Singh et al., 2016).
Scientists discovered that Trichoderma harzianum produces harmful enzymes, such as viriden, peptaboils, and sesquiterpenes, which impede the growth and development of other species. This makes the fungus a formidable mycoparasite, as it destroys pathogen cell walls through the synthesis of chitinase, making it effective against several well-known diseases, including Botrytis, Rhizopus, and Verticillium (Pylak et al., 2019). Research studies have demonstrated the effectiveness of T. asperellum in enhancing the growth and productivity of organic strawberries. Additionally, it provides protection against Botrytis cinerea, as well as reducing infection by Phytophthora cactorum and Verticillium dahliae. Incorporating this fungus into the soil promotes growth during the growing season, protects against the detrimental impact of Botrytis cinerea both during and after harvest, and results in fewer infections (Dorais and Alsanius, 2015; Scortichini, 2022). Moreover, researchers found that Pantoea agglomerans, an endophytic fungus isolated from healthy mulberry roots, inhibits Pseudomonas syringae, while P. fluorescens Pf-5 strain prevents B. cinerea from spreading on strawberry plants. Nonetheless, a recent study conducted by Pánek et al. (2021) shed light on the potential drawbacks of using Integral Pro (B. subtillis) and a strain of Pseudomonas sp. in strawberry plants. Surprisingly, these applications caused a decline in the overall health of the plants, even in the absence of Phytophthora cactorum. In contrast, another study highlighted the beneficial effects of two strains of Lactobacillus plantarum, which effectively suppressed the growth of X. fragariae in strawberries (Pylak et al., 2019).
Commercial biopreparations have promising results as an alternative to conventional fungicides for reducing the severity of several berry plant pathogens. Polyversum WP, derived from Pythium oligandrum, effectively reduces Botrytis cinerea, leaf spot, and powdery mildew infections in strawberry plants, with efficacy similar to chemical fungicides. While not a universal solution, it promotes resistance, produces hydrolytic enzymes, and suppresses B. cinerea. Nevertheless, certain studies have indicated its ineffectiveness against Mycosphaerella fragariae and B. cinerea (Boček et al., 2012; Pylak et al., 2019). Moreover, they show potential as effective biopreparations against specific plant pathogens, such as Phytophthora cactorum, and further research is needed to explore their effectiveness and mechanisms of action (Pánek et al., 2021).
4.1.4 Quality attributes—postharvest
Microbial biostimulants have demonstrated their efficacy in significantly enhancing the quality attributes of berry crops. The efficacy of these biostimulants in enhancing the nutritional content, flavor, color, and aroma of berries has been proven, which increased their market value and consumer appeal (Fernandes et al., 2022; Islam et al., 2020; Zulfiqar et al., 2024). For instance, inoculation of blackberries with Pseudomonas fluorescens resulted in enhanced fruit yield and better flavonoids and other phenolic substances (Ramos-Solano et al., 2014). Castellanos‐Morales et al. (2010) also obtained favorable results when inoculating strawberry plants with the arbuscular mycorrhizal (AM) fungus Glomus intraradices. Likewise, the co-inoculation of G. intraradices and Pseudomonas fluorescens yielded positive outcomes in enhancing strawberry plant growth (Palencia, 2013). Bioinoculants can also affect the concentration of vitamins in berries, including vitamins C and B9. High levels of vitamin C were observed in strawberries inoculated with Paenibacillus polymyxa (Erturk et al., 2012). A strain of Phyllobacterium succeeded in producing biofilms that can increase the vitamin C content in berries, thus improving their overall quality (Bona et al., 2015; Flores-Félix et al., 2015, 2018).
Microbial biostimulants can enhance the flavor, aroma, color, and appearance of berries by balancing organic acids and soluble sugars and increasing the levels of anthocyanins (Bona et al., 2016; Lingua et al., 2013). Several bacterial species, including Pseudomonas, Bacillus, Paraburkholderia, Alcaligenes, Acinetobacter, Serratia, and Arthrobacter, were identified as useful for improving the quality of berry crops (Mockeviciute et al., 2022). The utilization of Pseudomonas spp. and Bacillus spp. resulted in elevated levels of total sugar and total soluble solids, accompanied by a decrease in sugar content and titratable acidity in strawberries. Moreover, the application of B. subtilis enhanced the sweetness and overall flavor of blueberries (Pirlak and Köse, 2009; Seema et al., 2018). The introduction of a mixture of arbuscular mycorrhizal fungi (AMF) and/or two Pseudomonas strains to strawberry plants resulted in significant improvements in the qualitative and nutritional characteristics of the fruits. Notably, the concentrations of sugars, ascorbic acid, and folic acid exhibited substantial boosts as a result of this inoculation (Bona et al., 2015). Pseudomonas fluorescens and Rhizophagus irregularis significantly increased the quantities of VOCs responsible for the aroma and flavor of strawberries (Todeschini et al., 2018). Furthermore, the utilization of Paraburkholderia fungorum and B. amylolequifaciens boosted the levels of anthocyanins in strawberry fruits. Anthocyanins are the pigments responsible for the captivating blue, red, and purple hues exhibited by berries (Lingua et al., 2013). Similarly, strawberry co-inoculation with PGPR strains and mycorrhizal fungi and the application of a G. mosseae biostimulant amplified their anthocyanin content. Likewise, P. fluorescens improved the color and texture of strawberries (Bona et al., 2015; Jiménez-Gómez et al., 2017). Nevertheless, blackberries that were inoculated with Pseudomonas sp. did not exhibit any alterations in the anthocyanin content (Ramos-Solano et al., 2014).
4.2 Non-microbial biostimulants
4.2.1 Algal extracts based biostimulants
Algae-based biostimulants from macroalgae and microalgae improve plant growth, development, and resilience against abiotic stressors. Seaweed extracts function as chelators (Spinelli et al., 2010), enhancing mineral nutrient absorption, stimulating root development (Chen et al., 2021; Johnson et al., 2024; Nofal et al., 2024), and acting as biostimulants, promoting seed germination, plant growth, and resilience to stressors (Drocco et al., 2024; El Boukhari et al., 2023; Mattner et al., 2018). Although microalgae have seen less research for agricultural use than macroalgae, studies have shown that they can affect various plant processes, improve soil water-holding capacity, and increase nutrient availability (Kapoore et al., 2021; Ronga et al., 2019) (Figure 3).
4.2.1.1 Effect on plant nutrient uptake, stress resistance, and quality attributes
Seaweed extracts enhance shoot growth and boost crop yield in various berry crop species, including strawberries (Table 3). Studies on Ecklonia maxima and Ascophyllum nodosum demonstrate increased root growth in some strawberry cultivars. Nevertheless, studies conducted on cultivars such as Camarosa and Salut have demonstrated that the effects of seaweed extracts can vary depending on the specific cultivar and growth conditions (Alam et al., 2013; Kapoore et al., 2021; Righini et al., 2018). Extracts from Duvillaea potatorum, A. nodosum, and Sargassum sp. have also been discovered to have similar effects on field-grown strawberries (Kapoore et al., 2021; Mattner et al., 2018). Seaweed extracts contain high levels of auxins and cytokinins, which are phytohormones linked to enhancing root growth, total root volume, and root length. Additionally, the hydrophilic nature of seaweed extracts enables them to be easily absorbed by plants through their cuticles and stomata (Battacharyya et al., 2015). In addition, algae extract serves as a natural plant cell sap rich in enzymes and vitamins. This extract has the potential to enhance crop yield by facilitating the absorption of nutrients and transfer of solutes within plants (Plaza et al., 2018). Actiwave serves as a prime example of a metabolic enhancer derived from A. nodosum. It boasts a meticulously balanced and consistent formulation, incorporating kahydrin, alginic acid, and betaines. The synergistic effect of these components significantly contributes to the product’s efficacy. Researchers have observed that administering Actiwave to strawberry plants via soil drenching leads to a notable increase in the weight of their roots (Shukla et al., 2019; Spinelli et al., 2010). The research conducted on A. nodosum extracts has demonstrated that applying seaweed extract to strawberry plants’ foliage can increase the diversity and activity of the rhizosphere microbiome (Table 3). Furthermore, root-drench applications of seaweed extracts can act as prebiotics and enhance soil microbial activity, leading to improved growth of strawberries.
Biostimulatory effects on plant growth and yield have been reported for various microalgae species, including Dunaliella salina, Chlorella vulgaris, C. infusionum, Spirulina maxima, Scenedesmus platensis, S. quadricauda, and Acutodesmus dimorphus, as noted in studies conducted by Cho et al. (2022), Colla and Rouphael (2020), and Fernandes et al. (2022). S. obiliquus extracts increased strawberry root development, while cyanobacteria improved root development and root/shoot ratio in strawberries, This leads to enhanced nutrient and water uptake from the soil (Chiaiese et al., 2018; Cho et al., 2022; Santini et al., 2021). Chlorella vulgaris extract, among other microalgae biostimulants, exerts positive effects on plant growth and yield. These positive effects are linked to the presence of essential primary metabolites, such as arginine and tryptophan, as well as amino acids, vitamins, osmolytes like proline and glycine betaine, and polysaccharides like β-glucan (Celiktopuz et al., 2020; Cho et al., 2022; Kapoore et al., 2021; Ronga et al., 2019). Amino acids and polysaccharides interact with leucine-rich repeat membrane receptors, modulating the expression of genes related to cell expansion (Fernandes et al., 2022; Ronga et al., 2019). Furthermore, various microalgae strains possess phytohormone-like activities, including cytokinins and auxins, crucial for promoting plant growth and increasing crop yield (Cho et al., 2022; Colla and Rouphael, 2020; Fernandes et al., 2022).
Seaweed extracts, such as A. nodosum, protect plants from abiotic stresses and promote plant development by containing chemicals like cytokinins, auxins, amino acids, betaines, vitamins, gibberellins, polyamines, and brassinosteroids (Fernandes et al., 2022; Righini et al., 2018; Ronga et al., 2019). For example, A. nodosum extract increases plant tolerance to drought and saline stress through alginic acid and betaines (Spinelli et al., 2010). Soil conditioners like alginic acid enhance the rhizosphere’s capacity to store water, while osmoprotectants like betaines help plants better withstand the effects of drought. There is evidence that Sargassum vulgare extracts may ameliorate seed germination in the face of saline stress, much as Actiwave (Righini et al., 2018). By directly scavenging reactive oxygen species (ROS), seaweed extracts increase antioxidant activity in plants, protecting them from damage caused by stress-induced free radicals (Kapoore et al., 2021; Tarakhovskaya et al., 2007).
Algae extract (EA) has potential applications in various fields due to its bioactive chemicals with antibacterial, antiviral, anticancer, antioxidant, and antifungal properties (Table 3). However, research on the direct impact of EA on fungal diseases in berry crops and their potential to induce plant resistance remains limited. EA can be effective against some fungal infections but not others. For example, a 0.5% A. nodosum extract reduced the prevalence of Colletotrichum acutatum on strawberry plants but had no effect against B. cinerea on certain cultivars (Aguado et al., 2014; Righini et al., 2018). Similarly, E. maxima extracts were ineffective against B. cinerea on strawberries. EA can also increase phenolic compound levels, stimulating the synthesis of defense-related proteins and enzymes, and contain polysaccharides and oligosaccharides that trigger resistance mechanisms in plants as elicitors and signal transduction molecules (Righini et al., 2018).
Algal extracts boost fruit quality in berry crops by modulating their nutritional and functional properties (Table 3). Extracts derived from brown algae are commonly used to improve the quality of organic berries (Lau et al., 2022; Morrison, 2020). For example, the application of Ascophyllum nodosum treatments has been shown to result in increased accumulation of total anthocyanins in strawberry fruits, leading to improved fruit quality (Fernandes et al., 2022; Soppelsa et al., 2019). Similarly, a study conducted by Weber et al. (2018) on the combined application of A. nodosum and silicon resulted in early fruit production with higher levels of anthocyanins as well as 10% more marketable yields. Nevertheless, when Sargassum spp., A. nodosum, and Laminaria spp. were applied as foliar sprays, they exhibited only minor positive effects on fruit soluble solid content, titratable acidity, and firmness, while no significant impact was observed on ascorbic acid content (El-Miniawy et al., 2014). Similarly, an extract from E. maxima applied to strawberries demonstrated no influence on total soluble solids, total acids, and fruit quality, but led to a notable 56% increase in the concentration of ascorbic acid (Eshghi et al., 2013).
4.2.2 Humic extract-based biostimulants
Humic substances (HS) naturally occur in soil organic matter and play a vital role in promoting root system growth, facilitating nutrient assimilation, and acting as antioxidants (Calvo et al., 2014; du Jardin, 2015; Garza-Alonso et al., 2022). As plant biostimulants, HS exhibit favorable impacts on the growth and development of strawberry plants, strawberries included (Table 4).
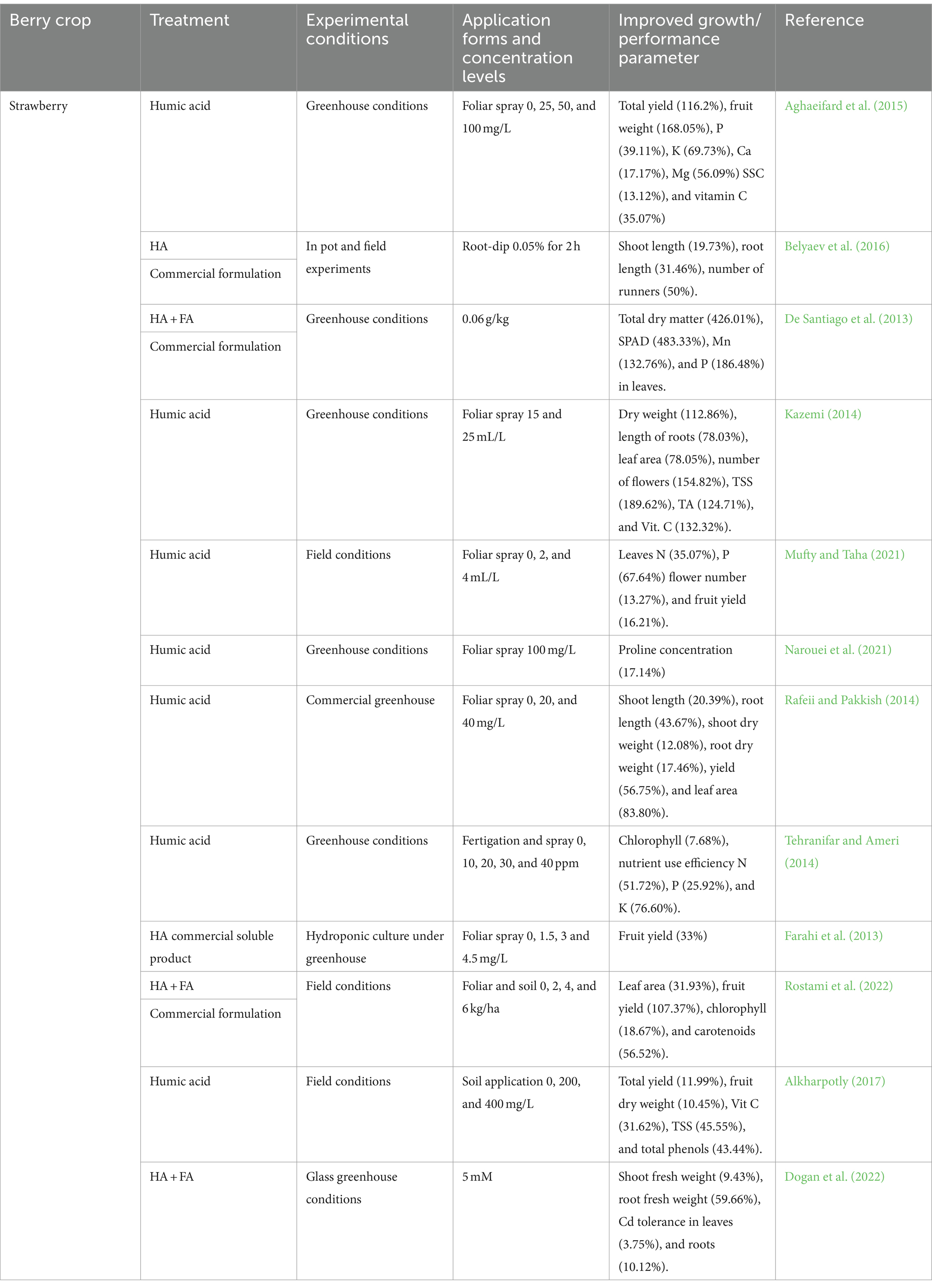
Table 4. In-depth exploration of the impact of humic substances on growth and quality parameters in berry crops.
4.2.2.1 Effect on plant metabolism, nutrients uptake, and stress resistance
Humic substances can benefit plants in multiple ways, including altering soil structure and fertility, influencing nutrient uptake, and modifying root architecture. They also impact plant metabolism and are directly absorbed by roots (Halpern et al., 2015; Vioratti Telles de Moura et al., 2023). The effects observed are highly contingent on the molecular weight, concentration, and specific source of the humic fractions applied. The positive effects of humic substances on plant growth and nutrient absorption are further supported by the proton pump activity of the plasma membrane H + -ATPase enzyme. Moreover, humic substances can modify plant metabolic pathways and influence gene expression and metabolite profiles (Milinković et al., 2021). Through their ability to enhance peroxidase activity and increase proline content, research studies have demonstrated that humic substances play a crucial role in mitigating the detrimental effects of abiotic stresses, including water and salinity stresses, on plants. In general, humic substances exhibit significant beneficial effects on plant development and growth (Alice et al., 2016; Milinković et al., 2021; Tripathi et al., 2022; Zuzunaga-rosas et al., 2024).
Several humic acids and humic substances were shown to induce various morphological changes in plants, leading to alterations in plant growth (Drocco et al., 2024; du Jardin, 2015). Table 4 provides a comprehensive summary of publications describing the effects of humic substances, including humic acids, on the development and physiology of various berry crops. Multiple studies have demonstrated that various humic acids and humic substances can induce morphological changes in plants, thereby influencing plant growth (du Jardin, 2015). A comprehensive compilation of studies detailing of humic substances effects, including humic acids, on the development and physiology of different berry crops can be accessed in Table 4. Humic acids were reported to primarily stimulate root growth and promote berry crop quality, which includes an enhancement in plant height (Neri et al., 2022), photosynthetic capacity, and chlorophyll content, in strawberries (Rostami et al., 2022), resulting in higher yields and improved fruit quality (Aghaeifard et al., 2015; Rafeii and Pakkish, 2014). Greenhouse trials with strawberries indicated significant increases in overall yield resulting from humic acid, including the fruit set, mean fruit weight, fruit length, fruit diameter, number of flowers and fruit per plant, and yield per plant (Arancon et al., 2006; Kazemi, 2014; Mufty and Taha, 2021; Rafeii and Pakkish, 2014). Foliar applied humic acid on strawberry plants has been shown to raise the levels of leaf potassium, phosphorus, calcium, magnesium, soluble solids concentrations, titratable acidity, vitamin C, and red tone (Aghaeifard et al., 2015; Jiménez-Arias et al., 2021). Humic substances can improve mineral uptake, raise cation exchange capacity, enhance pH buffering capacity, and influence soil structure. Additionally, they can elevate the microbial population in the soil, indirectly influencing plant growth (Halpern et al., 2015).
Humic acids improve berry crops’ abiotic stress tolerance by compensating for negative effects, activating antioxidant enzymes, and boosting osmotic adjustment. Humic acid-treated strawberry plants exhibited improved vegetative traits, enabling them to counteract the adverse effects of salinity, unlike those exposed to salt sources. The enhanced physiological and oxidative responses, subsequent increase in yield, and improved vegetative growth in the humic acid-treated plants were due to improved osmotic adjustment and increased activation of antioxidant enzymes (Garriga et al., 2015; Ghaderi et al., 2018; Saidimoradi et al., 2019; Zuzunaga-rosas et al., 2024). Furthermore, the promotion of shoot growth resulting from humic acid nutrition may be attributed to the stimulation of root growth, proliferation of root hairs, and initiation of new roots (Fan et al., 2014). However, a study on Vaccinium corymbosum L. showed that a microbial consortium outperformed humic substances leading to significant increases in plant growth and fruit yield. Over 2 years, the consortium boosted shoot dry weight by 35%, root dry weight by 70%, and total fruit yield by 104%. Humic substances improved fruit quality, while the combined treatment enhanced soil health indicators, positively affecting soil quality (Schoebitz et al., 2019).
4.2.3 The potential of protein hydrolysates
The byproducts of thermal, enzymatic, or chemical hydrolysis of animal or plant proteins are known as protein hydrolysates (PHs). These products can be classified as either PHs or individual amino acids (AAs) (Cardarelli et al., 2024; Drocco et al., 2024; Johnson et al., 2024; Marfà et al., 2009; Pylak et al., 2019). Furthermore, these formulations could feature a blend of essential nutrients, including proteins, peptides, free amino acids, lipids, carbohydrates, and phytohormones, synergistically enhancing their stimulatory effects on plants (Calvo et al., 2014; Zuzunaga-rosas et al., 2024).
4.2.3.1 Effect on plant growth, yield, nutrient uptake, and stress tolerance
Protein hydrolysates and amino acids display promise as alternative plant nutrients and growth regulators, positively impacting the quality, yield, and growth of berry crops. Recent studies have demonstrated their ability to stimulate root and shoot growth, promote plant height, and boost overall plant vigor. For instance, Marfà et al. (2009) demonstrated that applying PHs (Porcine blood) resulted in significant improvements in strawberry plant biomass, as well as enhanced flowering. Soppelsa et al. (2019) observed that AA and PHs treatment amplified chlorophyll concentration, leaf area, and root biomass in strawberry plants. Talukder et al. (2018) reported that hydroxyproline and glutamic acid application in a hydroponic strawberries system fostered plant growth and fruit yield, associated with heightened growth and mineral content in leaves, crown, and roots, as well as increased fruit number and weight. Arginine, a polyamine, sprayed on strawberry plants, enhanced yield, primary and secondary fruit weight, and achenes (Cardarelli et al., 2024; Mohseni et al., 2017). Furthermore, Soppelsa et al. (2019) demonstrated that alfalfa hydrolysate promoted strawberry fruits and roots biomass accumulation, along with increased overall leaf area. Conversely, porcine blood, a protein hydrolysate, expanded the number of flowers and fruit weight in strawberry plants (Marfà et al., 2009).
Protein hydrolysates act on various physiological mechanisms in plants, including the indirect stimulation of plant-associated microbes and the activation of crucial enzymes involved in primary and secondary metabolisms. B vitamins, specifically vitamin B1, also increase root and leaf area by serving as a cofactor in key metabolic pathways (Soppelsa et al., 2019). Additionally, plants rapidly absorb amino acids and peptides through their roots and leaves. Some protein-based products also contain plant growth regulators, like triacontanol and IAA, further amplifying their influence on plant development. In strawberry production, PHs enhance vegetative growth, antioxidant chemicals, chlorophyll content, and tissue mineral content (Calvo et al., 2014; Drocco et al., 2024). However, additional research is necessary to elucidate the mode of action of these biostimulants on various berry crops, including their growth, stress tolerance, and postharvest quality. By studying their effects on different crops, researchers can develop tailored and effective strategies for sustainable agriculture.
4.2.4 Chitosan and other biopolymers
At the forefront of sustainable solutions, biopolymers, among them cellulose, collagen, chitin alginate, and chitosan—play pivotal roles in diverse industries, such as agriculture. Chitosan, derived from arthropod exoskeletons and marine waste, is a widely utilized bioproduct in biopreparations. Its popularity stems from its exceptional absorption capacity, biodegradability, biocompatibility, and non-toxicity (Li et al., 2017; Petriccione et al., 2015; Pylak et al., 2019). Chitosan is pivotal in bolstering plant resilience against pathogens and environmental stresses, while also fostering vegetative growth (El-Araby et al., 2023; Garza-Alonso et al., 2022). Furthermore, it serves as an effective disease control agent for berries, proving its utility in both pre-and post-harvest applications (Romanazzi and Feliziani, 2016).
4.2.4.1 Effect on plant metabolism, nutrient uptake, and stress resistance
Chitosan application in plants offers a range of benefits, including increased biomass, and elevated levels of photosynthetic pigments and antioxidants. It can be applied through various methods, such as seed priming, soil drenching, or foliar spraying (Faqir et al., 2021). Although its impact on mineral concentration has been less studied, the use of chitosan in strawberry cultivation has been reported to enhance fruit quality, prolong postharvest life, and bolster the antioxidant system (Garza-Alonso et al., 2022). By applying a chitosan solution to the foliage, the strawberry plants experienced an increase in growth and the production of several types of antioxidants. This suggests that chitosan could enhance plant growth and improve produce quality by elevating the concentration of bioactive compounds (du Jardin, 2015; El-araby et al., 2022; Faqir et al., 2021). However, there is limited data available on its effects on plant physiology.
Chitosan exhibits numerous PGP properties, serving as a signaling and growth regulator, as well as a pest and disease resistance agent. It enhances plant defense mechanisms by stimulating photochemistry and photosynthesis-related enzymes and influences nuclear deformation and apoptosis. Its electrophysiological properties even suggest potential herbicidal applications (El-Araby et al., 2023; Faqir et al., 2021). Chitosan’s physiological effects are due to its polycationic nature, allowing it to bind to a wide range of cellular components, including cell wall constituents, plasma membranes, and DNA. Furthermore, chitosan can interact with specific receptors, triggering the activation of defense genes, a process that mirrors the function of plant defense elicitors (du Jardin, 2015; Garza-Alonso et al., 2022).
Chitosan activates specific receptors and pathways, triggering the accumulation of hydrogen peroxide and Ca2+ leakage, resulting in significant physiological changes. This makes chitosan useful in agricultural applications, particularly in protecting plants against fungal pathogens. Additionally, chitosan can enhance tolerance to abiotic stressors by influencing both primary and secondary metabolisms. Chitosan can induce stomatal closure through an ABA-dependent mechanism, offering protection against environmental stressors (du Jardin, 2015; El-araby et al., 2022; Garza-Alonso et al., 2022). Furthermore, chitosan’s insolubility in water gives it excellent antibacterial, antifungal, and antimicrobial capabilities (Faqir et al., 2021). Multiple successful experimental trials of chitosan applications extending the storage life of fruit berries are documented in the literature (Table 5). Chitosan is effective in post-harvest disease management and is available in commercial formulations (El-Araby et al., 2023; Pylak et al., 2019; Romanazzi et al., 2015). It has been proven to efficiently suppress postharvest diseases such as gray mold and Rhizopus rot in strawberries (Feliziani et al., 2015; Romanazzi et al., 2015). Chitosan-treated berries show increased levels of PAL activity, chitinase, -1,3-glucanase, glutathione, and ascorbic acid (Eikemo et al., 2003; Romanazzi et al., 2015). Edible coatings made from chitosan, chito-oligosaccharides, carboxymethyl cellulose, and hydroxypropyl methylcellulose, as well as chitosan and essential oils or beeswax, have had a positive impact on berries (Petriccione et al., 2015; Romanazzi and Feliziani, 2016). However, additional research is required to optimize their application in organic berry production and comprehensively understand their potential.
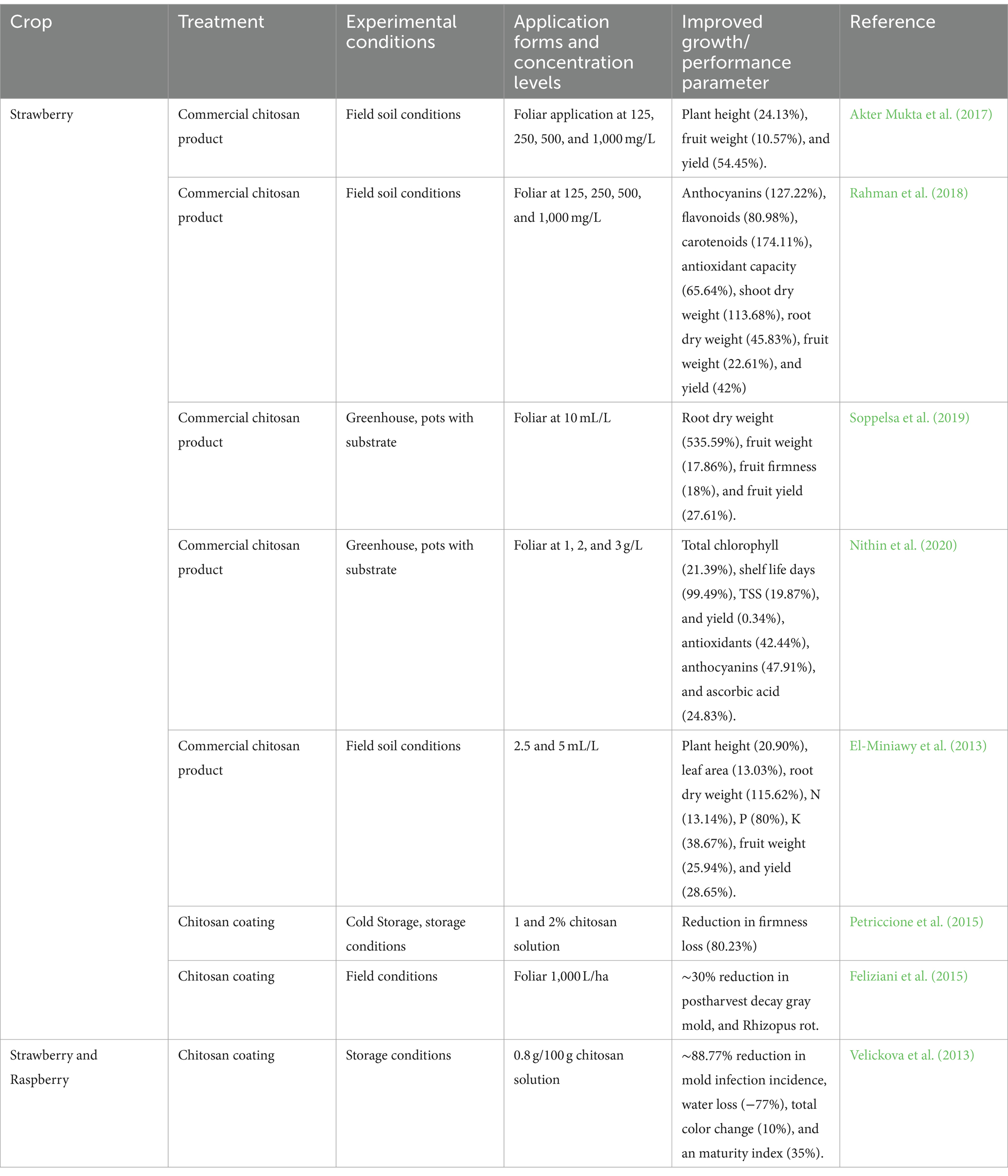
Table 5. Overview of literature reporting the stimulation of growth through biological biostimulants.
5 Conclusion and future prospects
Promoting sustainable agricultural practices in African regions is crucial for enhancing agricultural productivity, ensuring environmental sustainability, and conserving biodiversity (Jouzi et al., 2017). To achieve this, farmers must prioritize continuous education and training in organic farming techniques, biostimulants, and biopesticides. These practices reduce reliance on harmful chemicals, enhance soil health, preserve biodiversity, and improve the resilience of agricultural ecosystems. Integrating biostimulants and biopesticides into berry cultivation, such as strawberries, is a significant step toward sustainable agriculture (Abdollahzadeh et al., 2015; Zulfiqar et al., 2024). By adopting these practices, farmers can mitigate the negative impacts of conventional chemical inputs and enhance the health and vitality of their crops (Krishnan et al., 2016). This holistic approach to farming acknowledges the interconnectedness of soil health, plant growth, and environmental sustainability. For instance, in regions of Africa with acidic soils, phosphate rock plays a pivotal role in sustainable agriculture. Its slow-release nature provides a steady supply of phosphorus to plants, addressing nutrient deficiencies effectively. Unlike quick-release phosphorus fertilizers, phosphate rock minimizes nutrient leaching and runoff, promoting long-term soil health and environmental sustainability (Mir et al., 2022). When combined with biostimulants, phosphate rock enhances soil fertility, crop vigor, and yields, demonstrating the benefits of sustainable agriculture (Wang et al., 2022).
Moreover, research on biocontrol methods for strawberry pest control across different agricultural systems has highlighted factors influencing the adoption of biocontrol agents (BCAs), such as personal experience, confidence levels, fear of losses, and limited promotion of these methods. Educating farmers on the benefits of biostimulants and biopesticides can increase their confidence in adopting sustainable practices (Moser et al., 2008; Zulfiqar et al., 2024). Embracing a holistic approach to farming and leveraging tools like Fuzzy Cognitive Mapping can enhance the adoption of preventive pest management strategies and bridge the knowledge gap between farmers and researchers, promoting sustainable agricultural practices (Bardenhagen et al., 2020; Luo et al., 2022).
Overcoming obstacles to sustainable farming in Africa, such as resource constraints and climate-related challenges, necessitates supportive policies and programs. Collaborative efforts involving government entities, industries, academia, and other stakeholders are vital for driving the adoption of eco-friendly and sustainable farming practices across the continent (Mawar et al., 2021). Additionally, exploring the responses of farmers cultivating various crops to sustainable strategies can offer valuable insights for advancing agricultural sustainability on a broader scale. Success stories in organic farming across Africa, like those of Sebastian Scott in Zimbabwe, Fortress Farms in Kenya, and Kivu Hills Coffee in the Democratic Republic of Congo, exemplify the positive outcomes of transitioning to organic methods. These farmers have achieved high-quality organic berry production by focusing on soil health, pest control, biodiversity, and market access. Key success factors in this transition include training, soil management, pest control, market access, and robust support networks. Building farmer trust and ensuring the availability of bio-based agricultural inputs in the market are critical for higher adoption rates of organic practices. The growing consumer demand for healthier and environmentally friendly produce is reshaping the market toward organic berries, providing opportunities for farmers to cater to this expanding segment and meet the escalating demand for organic products. Furthermore, the Organic Trade Association (OTA) plays a significant role in promoting and protecting the growth of organic trade globally. Their advocacy efforts, industry insights, and research findings can provide valuable perspectives on the organic farming industry, market trends, consumer preferences, and the benefits of organic practices. Collaborating with organizations like OTA can enhance the knowledge sharing and advocacy efforts toward fostering sustainable agricultural practices in Africa and beyond (Giz, 2021).
Additionally, to the aforementioned, gaining farmer trust is mandatory to improve higher transition rate toward organic practices, which is highly contingent on the agro-efficiency of the market available bio-based agri-inputs. Successful adoption of novel biosolutions depends on famer awareness, product consistency and manufacturer claims; three critical factors that are often lacking. In this framework, the current review demonstrates through numerous studies, the potential of different biostimulant categories in enhancing organic berry crop production and mitigating growth, biotic, and abiotic stresses. Our study underscores significant gaps that warrant further fundamental research. This research aims to elucidate how both microbial and non-microbial biostimulants enhance nutrient uptake, plant growth, and stress tolerance. These investigations may also facilitate the identification of markers indicative of beneficial responses, providing valuable insights for informing the development of biostimulant products. Blending various biostimulant categories, such as microbial inoculants with humic substances or seaweed extracts, shows the potential for providing more reliable benefits to crop production. To propel a substantial surge in crop productivity, setting sights on a 60–100% surge to align with the needs of the anticipated global population of 9.7 billion by 2050, it is imperative to prioritize ongoing research and foster collaboration among stakeholders from the public and private sectors. These endeavors are critical for unleashing the potential of organic berry production in Africa.
Author contributions
SM: Conceptualization, Methodology, Writing – original draft, Formal analysis, Visualization. AH: Funding acquisition, Validation, Writing – review & editing. MB: Writing – review & editing. HE: Writing – review & editing. KL: Funding acquisition, Project administration, Resources, Supervision, Validation, Writing – review & editing.
Funding
The author(s) declare financial support was received for the research, authorship, and/or publication of this article. The project was funded by OCP Foundation UM6P/INRA collaboration - BioBerries Project.
Conflict of interest
The authors declare that the research was conducted in the absence of any commercial or financial relationships that could be construed as a potential conflict of interest.
Publisher’s note
All claims expressed in this article are solely those of the authors and do not necessarily represent those of their affiliated organizations, or those of the publisher, the editors and the reviewers. Any product that may be evaluated in this article, or claim that may be made by its manufacturer, is not guaranteed or endorsed by the publisher.
References
Abdollahzadeh, G., Sharif, M., and Damalas, C. A. (2015). Perceptions of the bene fi cial and harmful effects of pesticides among Iranian rice farmers in fl uence the adoption of biological control. Crop Prot. 75, 124–131. doi: 10.1016/j.cropro.2015.05.018
Abu-Zahra, T. R., Al-Ismail, K., and Shatat, F. (2022). Effect of organic and conventional systems on fruit quality of strawberry (fragaria × ananassa duch) grown under plastic house conditions in the Jordan Valley. 2006, 2022. Acta Hortic. 741, 159–172. doi: 10.17660/ActaHortic.2007.741.18
Aghaeifard, F., Babalar, M., Fallahi, E., and Ahmadi, A. (2015). Influence of humic acid and salicylic acid on yield, fruit quality, and leaf mineral elements of strawberry (Fragaria ananassa Duch.) cv. J. Plant Nutr. 39:4167. doi: 10.1080/01904167.2015.1088023
Aguado, A., Pastrana, A. M., De Los Santos, B., Romero, F., Sánchez, M. C., and Capote, N. (2014). Efficiency of natural products in the control of colletotrichum acutatum monitored by real-time PCR. Acta Hortic. 1049, 329–334. doi: 10.17660/ActaHortic.2014.1049.44
Akter Mukta, J., Rahman, M., As Sabir, A., Gupta, D. R., Surovy, M. Z., Rahman, M., et al. (2017). Chitosan and plant probiotics application enhance growth and yield of strawberry. Biocatal. Agric. Biotechnol. 11, 9–18. doi: 10.1016/j.bcab.2017.05.005
Alam, M. Z., Braun, G., Norrie, J., and Hodges, D. M. (2013). Effect of Ascophyllum extract application on plant growth, fruit yield and soil microbial communities of strawberry. Can. J. Plant Sci. 8, 23–36. doi: 10.4141/CJPS2011-260
Alice, B., Melo, G.De, Motta, F. L., Helena, M., and Santana, A. (2016). Humic acids: structural properties and multiple functionalities for novel technological developments. Mater. Sci. Eng. C 62, 967–974. doi: 10.1016/j.msec.2015.12.001
Ali, Q., Kurubas, M. S., Ustun, H., Balkhi, M., and Erkan, M. (2022). Evaluation of foliar organic fertilizer, biofertilizer and biological fungicide on the antioxidant compounds and postharvest quality attributes of strawberry fruit. Erwerbs-obstbau 64, 365–376. doi: 10.1007/s10341-022-00659-w
Alkharpotly, A. (2017). Impact of soil humic acid soil application and seaweed extract foliar Spray on growth, yield, and fruits quality of strawberry plants grown under Aswan conditions. J. Soil Sci. Agric. Eng. 8, 307–315. doi: 10.21608/jssae.2017.37496
Al-Shatri, A. H. N., Pakyürek, M., and Yaviç, A. (2020). Effect of seaweed application on nutrient uptake of strawberry cv. Albion grown under the environmental conditions of northern Iraq. Appl. Ecol. Environ. Res. 18, 1267–1279. doi: 10.15666/aeer/1801_12671279
Altaf, M. A., Shahid, R., Qadir, A., Naz, S., and Ren, M. (2019). Potential role of plant growth promoting rhizobacteria (PGPR) to reduce potential role of plant growth promoting rhizobacteria (PGPR) to reduce chemical fertilizer in horticultural crops. Int. J. Res. Agric. For. 6, 21–30.
Anjos, R., Cosme, F., Gonçalves, A., Nunes, F. M., Vilela, A., and Pinto, T. (2020). Effect of agricultural practices, conventional vs organic, on the phytochemical composition of ‘Kweli’ and ‘Tulameen’ raspberries (Rubus idaeus L.). Food Chem. 328:126833. doi: 10.1016/j.foodchem.2020.126833
Arancon, N. Q., Edwards, C. A., Lee, S., and Byrne, R. (2006). Effects of humic acids from vermicomposts on plant growth. Eur. J. Soil Biol. 42, 65–69. doi: 10.1016/j.ejsobi.2006.06.004
Arıkan, Ş., İpek, M., Eşitken, A., Pırlak, L., Figen, M., and Turan, M. (2020). Plant growth promoting rhizobacteria mitigate deleterious combined effects of salinity and lime in soil in strawberry plants. J. Plant Nutr. 43, 2028–2039. doi: 10.1080/01904167.2020.1766073
Backer, R., Rokem, J. S., Ilangumaran, G., Lamont, J., Praslickova, D., Ricci, E., et al. (2018). Plant growth-promoting rhizobacteria: context, mechanisms of action, and roadmap to commercialization of biostimulants for sustainable agriculture. Front. Plant Sci. 9:1473. doi: 10.3389/fpls.2018.01473
Baez-Rogelio, A., Morales-García, Y. E., Quintero-Hernández, V., and Muñoz-Rojas, J. (2017). Next generation of microbial inoculants for agriculture and bioremediation. Microb. Biotechnol. 10, 19–21. doi: 10.1111/1751-7915.12448
Bahadur, A., Batool, A., Nasir, F., Jiang, S., Mingsen, Q., and Zhang, Q. (2019). Mechanistic insights into arbuscular mycorrhizal fungi-mediated drought stress tolerance in plants. Int. J. Mol. Sci. 20:4199. doi: 10.3390/ijms20174199
Baker, B. P., Green, T. A., and Loker, A. J. (2020). Biological control and integrated pest management in organic and conventional systems. Biol. Control 140:104095. doi: 10.1016/j.biocontrol.2019.104095
Balci, G., Keles, H., and Çakmakçi, R. (2020). The effects of biofertilizers on some physological responses in heritage raspberries. J. Agric. Nat. 3, 1422–1427. doi: 10.18016/ksutarimdoga.vi.652416
Bardenhagen, C. J., Howard, P. H., and Gray, S. A. (2020). Farmer mental models of biological pest control: associations with adoption of conservation practices in blueberry and cherry orchards. Front. Sustain. Food Syst. 4:54. doi: 10.3389/fsufs.2020.00054
Basha, M. B., and Lal, D. (2019). Indian consumers’ attitudes towards purchasing organically produced foods: an empirical study. J. Clean. Prod. 215, 99–111. doi: 10.1016/j.jclepro.2018.12.098
Battacharyya, D., Babgohari, M. Z., Rathor, P., and Prithiviraj, B. (2015). Seaweed extracts as biostimulants in horticulture. Sci. Hortic. 196, 39–48. doi: 10.1016/j.scienta.2015.09.012
Belyaev, A. A., Lelyak, A. A., Shternshis, M., and Shpatova, T. (2016). The use of Bacillus spp. Strains for biocontrol of Ramularia leaf spot on strawberry and improving plant health in Western Siberia. Res. J. Pharm., Biol. Chem. Sci. 7:1594.
Bettiol, W., Ghini, R., Galvão, J. A. H., and Siloto, R. C. (2004). Organic and conventional tomato cropping systems. Sci. Agric. 61, 253–259. doi: 10.1590/s0103-90162004000300002
Bilawal, A., Ishfaq, M., Gantumur, M., Qayum, A., Shi, R., Ali, S., et al. (2021). A review of the bioactive ingredients of berries and their applications in curing diseases. Food Biosci. 44:101407. doi: 10.1016/j.fbio.2021.101407
Bizabani, C., Fontenla, S., and Dames, J. F. (2016). Ericoid fungal inoculation of blueberry under commercial production in South Africa. Sci. Hortic. 209, 173–177. doi: 10.1016/j.scienta.2016.06.029
Boček, S., Salaš, P., Sasková, H., and Mokričková, J. (2012). Effect of Alginure® (seaweed extract), Myco-sin® vin (sulfuric Clay) and Polyversum® (Pythium Oligandrum Drechs.) on yield and disease control in organic strawberries. Acta Univ. Agric. Silvicult. Mendelian. Brunensis 60, 19–28. doi: 10.11118/actaun201260080019
Bona, E., Lingua, G., Manassero, P., Cantamessa, S., Marsano, F., Todeschini, V., et al. (2015). AM fungi and PGP pseudomonads increase flowering, fruit production, and vitamin content in strawberry grown at low nitrogen and phosphorus levels. Mycorrhiza 25, 181–193. doi: 10.1007/s00572-014-0599-y
Bona, E., Lingua, G., and Todeschini, V. (2016). Effect of Bioinoculants on the Quality of Crops. New Delhi: Springer.
Booth, J., Schenk, P. M., and Mirzaee, H. (2022). Microbial biopesticides against bacterial, fungal and oomycete pathogens of tomato, cabbage and chickpea. Appl. Microbiol. 2, 288–301. doi: 10.3390/applmicrobiol2010021
Borkowska, B. (2002). Growth and photosynthetic activity of micropropagated strawberry plants inoculated with endomycorrhizal fungi (AMF) and growing under drought stress. Acta Physiol. Plant. 24, 365–370. doi: 10.1007/s11738-002-0031-7
Cai, B., Vancov, T., Si, H., Yang, W., Tong, K., Chen, W., et al. (2021). Isolation and characterization of endomycorrhizal fungi associated with growth promotion of blueberry plants. J. Fungi 7, 7–584. doi: 10.3390/jof7080584
Calvo, P., Nelson, L., and Kloepper, J. W. (2014). Agricultural uses of plant biostimulants. Plant Soil 383, 3–41. doi: 10.1007/s11104-014-2131-8
Camargo, L. K., Resende, J. T. V.De, Tominaga, T. T., Kurchaidt, S. M., Camargo, C. K., and Figueiredo, A. S. T. (2011). Postharvest quality of strawberry fruits produced in organic and conventional systems. Hortic. Bras. 29, 577–583. doi: 10.1590/s0102-05362011000400022
Cardarelli, M., Chami, A.El, Rouphael, Y., Ciriello, M., Bonini, P., Erice, G., et al. (2024). Plant biostimulants as natural alternatives to synthetic auxins in strawberry production: physiological and metabolic insights. Front. Plant Sci. 14:1337926. doi: 10.3389/fpls.2023.1337926
Caspersen, S., Svensson, B., Håkansson, T., Winter, C., Khalil, S., and Asp, H. (2016). Blueberry—soil interactions from an organic perspective. Sci. Hortic. 208, 78–91. doi: 10.1016/j.scienta.2016.04.002
Castellanos‐Morales, V., Villegas, J., Wendelin, S., Vierheilig, H., Eder, R., and Cárdenas‐Navarro, R. (2010). Root colonisation by the arbuscular mycorrhizal fungus Glomus intraradices alters the quality of strawberry fruits (Fragaria× ananassa Duch.) at different nitrogen levels. J. Sci. Food Agric. 90, 1774–1782. doi: 10.1002/jsfa.3998
Celiktopuz, E., Kapur, B., Sarıdas, M. A., and Kargı, S. P. (2020). Response of strawberry fruit and leaf nutrient concentrations to the application of irrigation levels and a biostimulant. J. Plant Nutr. 44, 153–165. doi: 10.1080/01904167.2020.1806310
Chebotar, V. K., Chizhevskaya, E. P., Vorobyov, N. I., Bobkova, V. V., Pomyaksheva, L. V., Khomyakov, Y. V., et al. (2022). The quality and productivity of strawberry (Fragaria × ananassa Duch.) improved by the inoculation of PGPR Bacillus velezensis BS89 in field experiments. Agronomy 12:2600. doi: 10.3390/agronomy12112600
Chen, D., Zhou, W., Yang, J., Ao, J., Huang, Y., Shen, D., et al. (2021). Effects of seaweed extracts on the growth, physiological activity, cane yield and sucrose content of sugarcane in China. Front. Plant Sci. 12:659130. doi: 10.3389/fpls.2021.659130
Chen, L., Liu, Y., Wu, G., Veronican, K., Shen, Q., and Zhang, N. (2016). Induced maize salt tolerance by rhizosphere inoculation of Bacillus amyloliquefaciens SQR9. Physiol. Plant. 158, 34–44. doi: 10.1111/ppl.12441
Chiaiese, P., Corrado, G., Colla, G., Kyriacou, M. C., and Rouphael, Y. (2018). Renewable sources of plant biostimulation: microalgae as a sustainable means to improve crop performance. Front. Plant Sci. 9:1782. doi: 10.3389/fpls.2018.01782
Chiomento, J. L. T., De Nardi, F. S., Filippi, D., dos Trentin, T., Dornelles, A. G., Fornari, M., et al. (2021). Morpho-horticultural performance of strawberry cultivated on substrate with arbuscular mycorrhizal fungi and biochar. Sci. Hortic. 282:110053. doi: 10.1016/j.scienta.2021.110053
Cho, G., Jo, G. S., Lee, Y., and Kwak, Y. (2022). Effect of Scenedesmus sp. CHK0059 on strawberry microbiota community. J. Microbiol. Biotechnol. 32, 862–868. doi: 10.4014/jmb.2205.05016
Cochard, B., Giroud, B., Crovadore, J., Chablais, R., Arminjon, L., and Lefort, F. (2022). Endophytic PGPR from tomato roots: isolation, in vitro characterization and in vivo evaluation of treated tomatoes (Solanum lycopersicum L.). Microorganisms 10:765. doi: 10.3390/microorganisms10040765
Colla, G., and Rouphael, Y. (2020). Microalgae: new source of plant biostimulants. Agronomy 10, 1–4. doi: 10.3390/agronomy10091240
Conti, S., Villari, G., Faugno, S., Melchionna, G., Somma, S., and Caruso, G. (2014). Effects of organic vs. conventional farming system on yield and quality of strawberry grown as an annual or biennial crop in southern Italy. Sci. Hortic. 180, 63–71. doi: 10.1016/j.scienta.2014.10.015
Coronado-apodaca, K. G., Martínez-ruiz, M., Iqbal, H. M. N., Sosa-hernandez, J. E., and Parra-saldívar, R. (2022). Agro-food sustainability transitions: new Frontiers for food security. Curr. Opin. Environ. Sci. Health 31:100412. doi: 10.1016/j.coesh.2022.100412
Crecente-Campo, J., Nunes-Damaceno, M., Romero-Rodríguez, M. A., and Vázquez-Odériz, M. L. (2012). Color, anthocyanin pigment, ascorbic acid and total phenolic compound determination in organic versus conventional strawberries (Fragaria×ananassa Duch, cv Selva). J. Food Compos. Anal. 28, 23–30. doi: 10.1016/j.jfca.2012.07.004
De Corato, U., Salimbeni, R., De Pretis, A., Avella, N., and Patruno, G. (2017). Antifungal activity of crude extracts from brown and red seaweeds by a supercritical carbon dioxide technique against fruit postharvest fungal diseases. Postharvest Biol. Technol. 131, 16–30. doi: 10.1016/j.postharvbio.2017.04.011
De Santiago, A., Carmona, E., Quintero, J. M., and Delgado, A. (2013). Effectiveness of mixtures of vivianite and organic materials in preventing iron chlorosis in strawberry. Span. J. Agric. Res. 11, 208–216. doi: 10.5424/sjar/2013111-2671
Di Vittori, L., Mazzoni, L., Battino, M., and Mezzetti, B. (2018). Pre-harvest factors influencing the quality of berries. Sci. Hortic. 233, 310–322. doi: 10.1016/j.scienta.2018.01.058
Dogan, M., Bolat, I., Karakas, S., and Dikilitas, M. (2022). Remediation of cadmium stress in strawberry plants using humic acid and remediation of cadmium stress in strawberry plants using humic acid and silicon applications. Lifestyles 12:1962. doi: 10.3390/life12121962
Dorais, M., and Alsanius, B. (2015). Advances and trends in organic fruit and vegetable farming research. Hortic. Rev. 43:185. doi: 10.1002/9781119107781.ch04
Drocco, C., Habteselassie, M., Itle, R. A., and Pennisi, S. V. (2024). Commercial biostimulants had minimal effects on greenhouse grown blueberry vegetative growth. Sci. Hortic. 336:113433. doi: 10.1016/j.scienta.2024.113433
Dubin, E., Dumas, A. S., Rebours, A., Jamin, E., Ginet, J., Lees, M., et al. (2017). Detection of blackcurrant adulteration by Aronia berry using high resolution mass spectrometry, variable selection and combined PLS regression models. Food Anal. Methods 10, 683–693. doi: 10.1007/s12161-016-0638-8
du Jardin, P. (2015). Plant biostimulants: definition, concept, main categories and regulation. Sci. Hortic. 196, 3–14. doi: 10.1016/j.scienta.2015.09.021
Egamberdieva, D., Hua, M., Reckling, M., Wirth, S., and Bellingrath-Kimura, S. D. (2018). Potential effects of biochar-based microbial inoculants in agriculture. Environ. Sustain. 1, 19–24. doi: 10.1007/s42398-018-0010-6
Eikemo, H., Stensvand, A., Tronsmo, A. M., Norwegian, T., Ås, N., and Isolates, P. (2003). Induced resistance as a possible means to control diseases of strawberry caused by Phytophthora spp. Plant Dis. 87, 345–350. doi: 10.1094/PDIS.2003.87.4.345
El-araby, A., El Ghadraoui, L., and Errachidi, F. (2022). Usage of biological chitosan against the contamination of post-harvest treatment of strawberries by Aspergillus niger. Front. Sustain. Food Syst. 6:881434. doi: 10.3389/fsufs.2022.881434
El-Araby, A., Janati, W., Ullah, R., Ercisli, S., and Errachidi, F. (2023). Chitosan, chitosan derivatives, and chitosan-based nanocomposites: eco-friendly materials for advanced applications (a review). Front. Chem. 11, 1–21. doi: 10.3389/fchem.2023.1327426
El Boukhari, M. E. M., Barakate, M., Drissi, B. E., Bouhia, Y., and Lyamlouli, K. (2023). Seaweed extract biostimulants differentially act in mitigating drought stress on Faba bean (Vicia faba L.). J. Plant Growth Regul. 42, 5642–5652. doi: 10.1007/s00344-023-10945-w
El ghanam, A. A. (2015). Bio-suppression of strawberry fruit rot disease caused by Botrytis cinerea. J. Plant Pathol. Microbiol. s3:5. doi: 10.4172/2157-7471.1000s3-005
El-Miniawy, S. M., Ragab, M. E., and Metwally, S. M. A. A. Y. (2014). Influence of foliar spraying of seaweed extract on growth, yield and quality of strawberry plants. J. Appl. Sci. Res. 10, 88–94. doi: 10.5555/20143214254
El-Miniawy, S. M., Ragab, M. E., Youssef, S. M., and Metwally, A. A. (2013). Response of strawberry plants to foliar spraying of chitosan. Res. J. Agric. Biol. Sci. 9, 366–372.
Enebe, M. C., and Babalola, O. O. (2019). The impact of microbes in the orchestration of plants’ resistance to biotic stress: a disease management approach. Appl. Microbiol. Biotechnol. 103, 9–25. doi: 10.1007/s00253-018-9433-3
Ersoy, N., Üniversitesi, S., Fakültesi, Z., Bölümü, B. B., and Türkiye, K. (2011). Some physico-chemical properties and antioxidant capacities of organically and conventionally cultivated strawberry‘Camarosa’. Selcuk J. Agric. Food Sci. 25, 73–78.
Erturk, Y., Ercisli, S., and Cakmakci, R. (2012). Yield and growth response of strawberry to plant growth-promoting Rhizobacteria inoculation. J. Plant Nutr. 35, 817–826. doi: 10.1080/01904167.2012.663437
Eshghi, S., Zare, M., Jamali, B., Gharaghani, A., and Farahi, M. H. (2013). Vegetative and reproductive parameters of “Selva” strawberry as influenced by Algaren, Drin and Green hum foliar application. Agric. Commun. 1, 27–32.
Esitken, A., Karlidag, H., Ercisli, S., Turan, M., and Sahin, F. (2003). The effect of spraying a growth promoting bacterium on the yield, growth and nutrient element composition of leaves of apricot (Prunus armeniaca L. cv. Hacihaliloglu). Aust. J. Agric. Res. 54, 377–380. doi: 10.1071/AR02098
Esitken, A., Yildiz, H. E., Ercisli, S., Figen Donmez, M., Turan, M., and Gunes, A. (2010). Effects of plant growth promoting bacteria (PGPB) on yield, growth and nutrient contents of organically grown strawberry. Sci. Hortic. 124, 62–66. doi: 10.1016/j.scienta.2009.12.012
Etesami, H., and Ryong, B. (2018). Ecotoxicology and environmental safety silicon (Si): review and future prospects on the action mechanisms in alleviating biotic and abiotic stresses in plants. Ecotoxicol. Environ. Saf. 147, 881–896. doi: 10.1016/j.ecoenv.2017.09.063
Expert Market Research. (2024). Berries market size, growth, industry share & report | 2032. Retrieved from https://www.expertmarketresearch.com/reports/berries-market#faq-sec-div
Fan, H., Wang, X., Sun, X., Li, Y., Sun, X., and Zheng, C. (2014). Scientia Horticulturae effects of humic acid derived from sediments on growth, photosynthesis and chloroplast ultrastructure in chrysanthemum. Sci. Hortic. 177, 118–123. doi: 10.1016/j.scienta.2014.05.010
FAOSTAT (2019). Available at: http://faostat3.fao.org.
Faqir, Y., Ma, J., and Chai, Y. (2021). Chitosan in modern agriculture production. Plant Soil Environ. 2021, 679–699. doi: 10.17221/332/2021-PSE
Farahi, M. H., Aboutalebi, A., Eshghi, S., Dastyaran, M., and Yosefi, F. (2013). Foliar application of humic acid on quantitative and qualitative characteristics of “aromas” strawberry in soilless culture. Agric. Commun. 1, 13–16.
Feliziani, E., Landi, L., and Romanazzi, G. (2015). Preharvest treatments with chitosan and other alternatives to conventional fungicides to control postharvest decay of strawberry. Carbohydr. Polym. 132, 111–117. doi: 10.1016/j.carbpol.2015.05.078
Fernandes, Â., Chaski, C., Pereira, C., Kosti, M., Rouphael, Y., Sokovi, M., et al. (2022). Water stress alleviation effects of biostimulants on greenhouse-grown tomato fruit. Horticulturae 8:645. doi: 10.3390/horticulturae8070645
Fincheira, P., and Quiroz, A. (2018). Microbial volatiles as plant growth inducers. Microbiol. Res. 208, 63–75. doi: 10.1016/j.micres.2018.01.002
Flores-Félix, J. D., Silva, L. R., Rivera, L. P., Marcos-García, M., García-Fraile, P., Martínez-Molina, E., et al. (2015). Plants probiotics as a tool to produce highly functional fruits: the case of Phyllobacterium and vitamin C in strawberries. PLoS One 10, 1–10. doi: 10.1371/journal.pone.0122281
Flores-Félix, J. D., Velázquez, E., García-Fraile, P., González-Andrés, F., Silva, L. R., and Rivas, R. (2018). Rhizobium and Phyllobacterium bacterial inoculants increase bioactive compounds and quality of strawberries cultivated in field conditions. Food Res. Int. 111, 416–422. doi: 10.1016/j.foodres.2018.05.059
Garriga, M., Muñoz, C. A., Caligari, P. D. S., and Retamales, J. B. (2015). Effect of salt stress on genotypes of commercial (Fragaria x ananassa) and Chilean strawberry (F. chiloensis). Sci. Hortic. 195, 37–47. doi: 10.1016/j.scienta.2015.08.036
Garza-Alonso, C. A., Olivares-Sáenz, E., González-Morales, S., La, F., Juárez-Maldonado, A., González-Fuentes, J. A., et al. (2022). Strawberry biostimulation: from mechanisms of action to plant growth and fruit quality. Plan. Theory 11:3463. doi: 10.3390/plants11243463
Ghaderi, N., Hatami, M., Mozafari, A., and Siosehmardeh, A. (2018). Change in antioxidant enzymes activity and some morpho-physiological characteristics of strawberry under long-term salt stress. Physiol. Mol. Biol. Plants 24, 833–843. doi: 10.1007/s12298-018-0535-2
Godlewska, K., Ronga, D., and Michalak, I. (2021). Plant extracts—importance in sustainable agriculture. Ital. J. Agron. 16:1851. doi: 10.4081/ija.2021.1851
Godoy, D., Dewbre, J.P., Amegnaglo, C.J., Soglo, Y. Y., Akpa, A. F., Bickel, M., et al. (2014). The Future of Food and Agriculture: Trends and Challenges. Food and Agriculture Organization of the United Nations (FAO).
Gomiero, T., Pimentel, D., and Paoletti, M. G. (2011). Environmental impact of different agricultural management practices: conventional vs. organic agriculture. Crit. Rev. Plant Sci. 30, 95–124. doi: 10.1080/07352689.2011.554355
Gouda, S., Kerry, R. G., Das, G., Paramithiotis, S., Shin, H. S., and Patra, J. K. (2018). Revitalization of plant growth promoting rhizobacteria for sustainable development in agriculture. Microbiol. Res. 206, 131–140. doi: 10.1016/j.micres.2017.08.016
Guerrero-molina, M. F., Winik, B. C., and Pedraza, R. O. (2012). More than rhizosphere colonization of strawberry plants by Azospirillum brasilense. Appl. Soil Ecol. 61, 205–212. doi: 10.1016/j.apsoil.2011.10.011
Gui, L. X., Lu, S. S., Chen, Q., Yang, L., and Xiao, J. X. (2021). iTRAQ-based proteomic analysis reveals positive impacts of arbuscular mycorrhizal fungi inoculation on photosynthesis and drought tolerance in blueberry. Trees Struct. Funct. 35, 81–92. doi: 10.1007/s00468-020-02015-5
Gunes, A., Turan, M., Kitir, N., Tufenkci, M. S., Mesut, K., and Ertan, C. (2016). Effects of bio-Bor fertilizer applications on fruit yield. Antioxid. Enzy. Act. Freeze Injury Strawberry 58, 177–184. doi: 10.1007/s10341-016-0274-x
Guo, X., Yuan, L., Shakeel, M., Wan, Y., Song, Z., and Wang, D. (2021). Isolation and identification of endophytic fungi with screening of promotion growth on mycorrhizal fungi in blueberry. Rhizosphere 19:100389. doi: 10.1016/j.rhisph.2021.100389
Gupta-Elera, G., Garrett, A., Martinez, A., Kraus, R. D., Robison, R., and O’Neill, K. (2012). A comparison of antioxidant properties in organic and conventional blueberries. J. Food Res. 1:1. doi: 10.5539/jfr.v1n3p1
Habib, S. H., Kausar, H., and Saud, H. M. (2016). Plant growth-promoting Rhizobacteria enhance salinity stress tolerance in okra through ROS-scavenging enzymes. Biomed. Res. Int. 2016, 1–10. doi: 10.1155/2016/6284547
Halpern, M., Bar-Tal, A., Ofek, M., Minz, D., Muller, T., and Yermiyahu, U. (2015). The use of biostimulants for enhancing nutrient uptake. Adv. Agron. 130. doi: 10.1016/bs.agron.2014.10.001
Hidalgo, G. I., and Almajano, M. P. (2017). Red fruits: extraction of antioxidants, phenolic content, and radical scavenging determination: A review. Antioxidants 6. doi: 10.3390/ANTIOX6010007
Holden, D., and Ross, R. (2017). Six years of strawberry trials in commercial fields demonstrate that an extract of the brown seaweed Ascophyllum nodosum improves yield of strawberries. Acta Hortic. 1156, 249–254. doi: 10.17660/ActaHortic.2017.1156.38
Islam, T., Gan, H. M., Ziemann, M., Hussain, H. I., Arioli, T., and Cahill, D. (2020). Phaeophyceaean (brown algal) extracts activate plant defense systems in Arabidopsis thaliana challenged with Phytophthora cinnamomi. Front. Plant Sci. 11:852. doi: 10.3389/fpls.2020.00852
Jajoo, A. (2021). Role of arbuscular mycorrhizal fungi as an underground saviuor for protecting plants from abiotic stresses. Physiol. Mol. Biol. Plants 27, 2589–2603. doi: 10.1007/s12298-021-01091-2
Jeong, B. R. (2022). Foliar silicon spray to strawberry plants during summer cutting propagation enhances resistance of transplants to high temperature stresses. doi: 10.3389/fsufs.2022.938128
Jiménez-Arias, D., García-Machado, F. J., Morales-Sierra, S., García-García, A. L., Herrera, A. J., Valdés, F., et al. (2021). A beginner’s guide to osmoprotection by biostimulants. Plan. Theory 10, 1–24. doi: 10.3390/plants10020363
Jiménez-Gómez, A., Celador-Lera, L., Fradejas-Bayón, M., and Rivas, R. (2017). Plant probiotic bacteria enhance the quality of fruit and horticultural crops. AIMS Microbiol 3, 483–501. doi: 10.3934/microbiol.2017.3.483
Jindo, K., Goron, T. L., Pizarro-tob, P., and Sudre, P. (2022). Application of biostimulant products and biological control agents in sustainable viticulture: A review. Front. Plant Sci. 13:932311. doi: 10.3389/fpls.2022.932311
Johnson, R., Joel, J. M., and Puthur, J. T. (2024). Biostimulants: the futuristic sustainable approach for alleviating crop productivity and abiotic stress tolerance. J. Plant Growth Regul. 43, 659–674. doi: 10.1007/s00344-023-11144-3
Jouzi, Z., Azadi, H., Taheri, F., Zarafshani, K., Gebrehiwot, K., Van Passel, S., et al. (2017). Organic farming and small-scale farmers: main opportunities and challenges. Ecol. Econ. 132, 144–154. doi: 10.1016/j.ecolecon.2016.10.016
Kahu, K., Klaas, L., and Kikas, A. (2010). Effect of cultivars and different growing technologies on strawberry yield and fruit quality. Agron. Res. 8, 589–593.
Kaiser, C., and Ernst, M. (2010). Organic Blackberries and Raspberries. 5. Available online at: https://www.uky.edu/Ag/CCD/introsheets/organicbrambles.pdf (Accessed December 28, 2023).
Kapoore, R. V., Wood, E. E., and Llewellyn, C. A. (2021). Algae biostimulants: a critical look at microalgal biostimulants for sustainable agricultural practices. Biotechnol. Adv. 49:107754. doi: 10.1016/j.biotechadv.2021.107754
Karlidag, H., Yildirim, E., Turan, M., Pehluvan, M., and Donmez, F. (2013). Plant growth-promoting rhizobacteria mitigate deleterious effects of salt stress on strawberry plants (Fragaria × ananassa). HortScience 48, 563–567. doi: 10.21273/hortsci.48.5.563
Karppinen, K., Zoratti, L., Sarala, M., Carvalho, E., Hirsimäki, J., Mentula, H., et al. (2016). Carotenoid metabolism during bilberry (Vaccinium myrtillus L.) fruit development under different light conditions is regulated by biosynthesis and degradation. BMC Plant Biol. 16:95. doi: 10.1186/s12870-016-0785-5
Kazemi, M. (2014). The impact of foliar humic acid sprays on reproductive biology and fruit quality of strawberry. Thai J. Agric. Sci. 47, 221–225. doi: 10.5555/20153141923
Khan, F., Kim, N. E., Bhujel, A., Jaihuni, M., Lee, D. H., and Basak, J. K. (2021). Assessment of combined trichoderma-enriched biofertilizer and nutrients solutions on the growth and yield of strawberry plants. J. Biosyst. Eng. 46, 225–235. doi: 10.1007/s42853-021-00102-0
Kirchmann, H., Kätterer, T., Bergström, L., Börjesson, G., and Bolinder, M. A. (2016). Flaws and criteria for design and evaluation of comparative organic and conventional cropping systems. Field Crop Res. 186, 99–106. doi: 10.1016/j.fcr.2015.11.006
Kotuła, M., Kapusta-Duch, J., Smoleń, S., and Doskočil, I. (2022). Phytochemical composition of the fruits and leaves of raspberries (Rubus idaeus L.)—conventional vs. organic and those wild grown. Appl. Sci. 12, 1–21. doi: 10.3390/app122211783
Krishnan, S., Nandwani, D., Smith, G., and Kankarta, V. (2016). “Sustainable urban agriculture: a growing solution to urban food deserts” in Organic Farming for Sustainable Agriculture. Sustainable Development and Biodiversity. ed. D. Nandwani, vol. 9 (Cham: Springer).
Kumar, P., Sharma, N., Sharma, S., and Gupta, R. (2020). Rhizosphere stochiometry, fruit yield, quality attributes and growth response to PGPR transplant amendments in strawberry (Fragaria × ananassa Duch.) growing on solarized soils. Sci. Hortic. 265:109215. doi: 10.1016/j.scienta.2020.109215
Kumar, Y., Sajwan, P., Uniyal, S., and Mishra, A. C. (2021). Enhancement in yield and nutritive qualities of strawberry fruits by the application of organic manures and biofertilizers. Sci. Hortic. 283:110038. doi: 10.1016/j.scienta.2021.110038
Kumawat, K. C., Sharma, B., and Nair, R. M. (2023). Plant growth-promoting rhizobacteria: salt stress alleviators to improve crop productivity for sustainable agriculture development. Front. Plant Sci. 13:1101862. doi: 10.3389/fpls.2022.1101862
Lau, S. E., Fei, W., Teo, A., Yang, E., Boon, T., and Tan, C. (2022). Microbiome engineering and plant biostimulants for sustainable crop improvement and mitigation of biotic and abiotic stresses. Discov. Food 2. doi: 10.1007/s44187-022-00009-5
Li, L., Sun, J., Gao, H., Shen, Y., Li, C., Yi, P., et al. (2017). Effects of polysaccharide-based edible coatings on quality and antioxidant enzyme system of strawberry during cold storage. Int. J. Poly. Sci. 2017, 1–8. doi: 10.1155/2017/9746174
Lingua, G., Bona, E., Manassero, P., Marsano, F., Todeschini, V., Cantamessa, S., et al. (2013). Arbuscular mycorrhizal fungi and plant growth-promoting pseudomonads increases anthocyanin concentration in strawberry fruits (Fragaria x ananassa var. Selva) in conditions of reduced fertilization. Int. J. Mol. Sci. 14, 16207–16225. doi: 10.3390/ijms140816207
Lombardi, N., Caira, S., Troise, A. D., Scaloni, A., Vitaglione, P., Vinale, F., et al. (2020). Trichoderma applications on strawberry plants modulate the physiological processes positively affecting fruit production and quality. Front. Microbiol. 11, 1–17. doi: 10.3389/fmicb.2020.01364
López, J., Vera, C., Bustos, R., and Florez, J. (2021). Native berries of Chile: a comprehensive review on nutritional aspects, functional properties, and potential health benefits. J. Food Meas. Charact. 15, 1139–1160. doi: 10.1007/s11694-020-00699-4
Lowe, A., Rafferty-mcardle, S. M., and Cassells, A. C. (2012). Effects of AMF- and PGPR-root inoculation and a foliar chitosan spray in single and combined treatments on powdery mildew disease in strawberry. Agric. Food Sci. 2011, 28–38. doi: 10.23986/afsci.4997
Luo, J., Zhao, C., Chen, Q., and Li, G. (2022). Using deep belief network to construct the agricultural information system based on internet of things. J. Supercomput. 78, 379–405. doi: 10.1007/s11227-021-03898-y
Maksimovic, V., Nikolic, M., Bogdanovic, J., Maletic, R., Milatovic, D., and Milivojevic, J. (2011). Chemical and antioxidant properties of cultivated and wild fragaria and rubus berries. J. Food Qual. doi: 10.1111/j.1745-4557.2010.00360.x
Malusa, E., Sas-paszt, L., Popinska, W., Zurawicz, E., and Sas-paszt, L. (2008). The effect of a substrate containing arbuscular mycorrhizal fungi and rhizosphere microorganisms (Trichoderma, Bacillus, Pseudomonas, and Streptomyces) and foliar fertilization on growth response and rhizosphere pH of three strawberry cultivars. Int. J. Fruit Sci. 6:8362. doi: 10.1300/J492v06n04
Marfà, O., Cáceres, R., Polo, J., and Ródenas, J. (2009). Animal protein hydrolysate as a biostimulant for transplanted strawberry plants subjected to cold stress. Acta Hortic. 842, 315–318. doi: 10.17660/ActaHortic.2009.842.57
Mattila, P., Hellström, J., and Törrönen, R. (2006). Phenolic acids in berries, fruits, and beverages. J. Agric. Food Chem. 54, 7193–7199. doi: 10.1021/jf0615247
Mattner, S. W., Milinkovic, M., and Arioli, T. (2018). Increased growth response of strawberry roots to a commercial extract from Durvillaea potatorum and Ascophyllum nodosum. J. Appl. Phycol. 30, 2943–2951. doi: 10.1007/s10811-017-1387-9
Mawar, R., Manjunatha, B. L., and Kumar, S. (2021). Commercialization, diffusion and adoption of bioformulations for sustainable disease management in Indian Arid Agriculture: prospects and challenges. Circ. Econ. Sustain. 1, 1367–1385. doi: 10.1007/s43615-021-00089-y
Mazzoni, L., Perez-Lopez, P., Giampieri, F., Alvarez-Suarez, J. M., Gasparrini, M., Forbes-Hernandez, T. Y., et al. (2016). The genetic aspects of berries: from field to health. J. Sci. Food Agric. 96, 365–371. doi: 10.1002/jsfa.7216
Mikiciuk, G., Sas-Paszt, L., Mikiciuk, M., Derkowska, E., Trzciński, P., Głuszek, S., et al. (2019). Mycorrhizal frequency, physiological parameters, and yield of strawberry plants inoculated with endomycorrhizal fungi and rhizosphere bacteria. Mycorrhiza 29, 489–501. doi: 10.1007/s00572-019-00905-2
Milinković, M., Vranić, D., Đurić, M., and Paunović, S. M. (2021). Chemical composition of organically and conventionally grown fruits of raspberry (Rubus idaeus L.) cv. Willamette. Acta Agric. Serb. 26, 83–88. doi: 10.5937/AASer2151083M
Mir, M. I., Hameeda, B., Quadriya, H., Kumar, B. K., Ilyas, N., Kee Zuan, A. T., et al. (2022). Multifarious indigenous diazotrophic rhizobacteria of rice (Oryza sativa L.) rhizosphere and their effect on plant growth promotion. Front. Nutr. 8:781764. doi: 10.3389/fnut.2021.781764
Mochizuki, M., Yamamoto, S., and Aoki, Y. (2012). Isolation and characterisation of Bacillus amyloliquefaciens S13-3 as a biological control agent for anthracnose caused by Colletotrichum gloeosporioides. Biocontrol Sci. Tech. 22, 697–709. doi: 10.1080/09583157.2012.679644
Mockeviciute, R., Jurkoniene, S., Gaveliene, V., Jankovska-Bortkeviˇc, E., Šocik, B., Armalyt, G., et al. (2022). Effects induced by the agricultural application of probiotics on antioxidant potential of strawberries. Plan. Theory 11:831. doi: 10.3390/plants11060831
Mohseni, F., Pakkish, Z., and Panahi, B. (2017). Arginine impact on yield and fruit qualitative characteristics of strawberry. Agric. Conspec. Sci. 82, 19–26.
Mokrani, S., and Nabti, E. (2022). Applied sciences recent trends in microbial approaches for soil desalination.
Moradtalab, N., Hajiboland, R., Aliasgharzad, N., and Hartmann, T. E. (2019). Silicon and the association with an mitigate the adverse effects of drought stress on strawberry. Agronomy 9:41. doi: 10.3390/agronomy9010041
Morrison, L. (2020). A concise review of the brown macroalga Ascophyllum nodosum (Linnaeus) Le Jolis. J. Appl. Phycol. 32, 3561–3584. doi: 10.1007/s10811-020-02246-6
Moser, R., Pertot, I., Elad, Y., and Raffaelli, R. (2008). Farmers’ attitudes toward the use of biocontrol agents in IPM strawberry production in three countries. Biol. Control 47, 125–132. doi: 10.1016/j.biocontrol.2008.07.012
Mufty, R. K., and Taha, S. M. (2021). Response two strawberry cultivars (Fragaria X Ananassa Duch.) for foliar application of two organic fertilizers. IOP Conf. Ser. Earth Environ. Sci. 910, 012033–012010. doi: 10.1088/1755-1315/910/1/012033
Muller, A., Schader, C., El-Hage Scialabba, N., Brüggemann, J., Isensee, A., Erb, K. H., et al. (2017). Strategies for feeding the world more sustainably with organic agriculture. Nat. Commun. 8, 1290–1213. doi: 10.1038/s41467-017-01410-w
Narouei, Z., Sedaghathoor, S., Kaviani, B., and Ansari, M. H. (2021). Biochemical and physiological traits of strawberry as influenced by organic acids and deficit irrigation under colored netting. Agric. Nat. Resour. 55, 1023–1038. doi: 10.34044/J.ANRES.2021.55.6.13
Neocleous, D., and Vasilakakis, M. (2009). Antioxidants—enhancing and understanding in strawberry fruits. Acta Hortic. 838, 193–198. doi: 10.17660/ActaHortic.2009.838.32
Neri, J. C., Meléndez-Mori, J. B., Tejada-Alvarado, J. J., Vilca-Valqui, N. C., Huaman-Huaman, E., Oliva, M., et al. (2022). An optimized protocol for micropropagation and acclimatization of strawberry (Fragaria × ananassa Duch.) variety ‘aroma.’. Agronomy 12:968. doi: 10.3390/agronomy12040968
Nithin, K. M., Madaiah, D., Shivakumar, B. S., Kumar, M. D., and Dhananjaya, B. C. (2020). Influence of chitosan foliar application on quality and biochemical traits of strawberry (Fragaria × Ananassa Duch.) under naturally ventilated polyhouse. Int. J. Curr. Microbiol. App. Sci. 9, 243–250. doi: 10.20546/ijcmas.2020.909.030
Nofal, A., Azzazy, M., Ayyad, S., Abdelsalm, E., Abousekken, M. S., and Tammam, O. (2024). Evaluation of the brown alga, Sargassum muticum extract as an antimicrobial and feeding additives. Braz. J. Biol. 84, e259721–e259729. doi: 10.1590/1519-6984.259721
Ochmian, I., Błaszak, M., Piwowarczyk, R., and Lachowicz, S. (2020). The impact of cultivation systems on the nutritional and phytochemical content, and microbiological contamination of highbush blueberry. Sci. Rep. 10, 1–14. doi: 10.1038/s41598-020-73947-8
Ochmian, I., Kozos, K., Chełpiński, P., and Szczepanek, M. (2015). Comparison of berry quality in highbush blueberry cultivars grown according to conventional and organic methods. Turk. J. Agric. For. 39, 174–181. doi: 10.3906/tar-1404-18
Ojuederie, O. B., Olanrewaju, O. S., and Babalola, O. O. (2019). Plant growth promoting rhizobacterial mitigation of drought stress in crop plants: implications for sustainable agriculture. Agronomy 9:712. doi: 10.3390/agronomy9110712
Palencia, P. (2013). Effect of arbuscular mycorrhizal fungi on quality of strawberry fruit in soilless growing system. Acta Hortic. 1013, 493–498. doi: 10.17660/ActaHortic.2013.1013.61
Pánek, M., Hanáček, A., Wenzlová, J., Maňasová, M., and Zouhar, M. (2021). A comparison of the ability of some commercially produced biological control agents to protect strawberry plants against the plant pathogen phytophthora cactorum. Agriculture 11. doi: 10.3390/agriculture11111086
Paredes-López, O., Cervantes-Ceja, M. L., Vigna-Pérez, M., and Hernández-Pérez, T. (2010). Berries: improving human health and healthy aging, and promoting quality life—a review. Plant Foods Hum. Nutr. 65, 299–308. doi: 10.1007/s11130-010-0177-1
Pastrana, A. M., Basallote-ureba, M. J., Aguado, A., Akdi, K., and Capote, N. (2016). Biological control of strawberry soil-borne pathogens Macrophomina phaseolina and Fusarium solani, using Trichoderma asperellum and Bacillus spp 55, 109–120. doi: 10.14601/Phytopathol_Mediterr-16363
Paszt, L. S., Sumorok, B., Derkowska, E., Trzciński, P., Lisek, A., Grzyb, Z. S., et al. (2020). Effect of microbiologically enriched fertilizers on the vegetative growth of strawberry plants in container-based cultivation. J. Res. Appl. Agric. Eng. 65, 21–29. doi: 10.5555/20193417381
Pedraza, R. O., Motok, J., Salazar, S. M., Ragout, A. L., Mentel, M. I., Tortora, M. L., et al. (2010). Growth-promotion of strawberry plants inoculated with Azospirillum brasilense. World J. Microbiol. Biotechnol. 26, 265–272. doi: 10.1007/s11274-009-0169-1
Peigné, J., Casagrande, M., Payet, V., David, C., Sans, F. X., Blanco-Moreno, J. M., et al. (2022). How organic farmers practice conservation agriculture in Europe. Renew. Agric. Food Syst. 31, 72–85. doi: 10.1017/S1742170514000477
Pérez-Gallardo, A., García-Almendárez, B., Barbosa-Cánovas, G., Pimentel-González, D., Reyes-González, L. R., and Regalado, C. (2015). Effect of starch-beeswax coatings on quality parameters of blackberries (Rubus spp.). J. Food Sci. Technol. 52, 5601–5610. doi: 10.1007/s13197-014-1665-3
Peris-felipo, F. J., Benavent-gil, Y., and Hernández-apaolaza, L. (2020). Silicon beneficial effects on yield, fruit quality and shelf-life of strawberries grown in different culture substrates under different iron status. Plant Physiol. Biochem. 152, 23–31. doi: 10.1016/j.plaphy.2020.04.026
PeŠaković, M., Karaklajić-Stajić, Ž., Milenković, S., and Mitrović, O. (2013). Biofertilizer affecting yield related characteristics of strawberry (Fragaria×ananassa Duch.) and soil micro-organisms. Sci. Hortic. 150, 238–243. doi: 10.1016/j.scienta.2012.11.016
Petriccione, M., Mastrobuoni, F., Pasquariello, M. S., Zampella, L., Nobis, E., Capriolo, G., et al. (2015). Effect of chitosan coating on the postharvest quality and antioxidant enzyme system response of strawberry fruit during cold storage. Food Secur. 4, 501–523. doi: 10.3390/foods4040501
Pirlak, L., and Köse, M. (2009). Effects of plant growth promoting rhizobacteria on yield and some fruit properties of strawberry. J. Plant Nutr. 32, 1173–1184. doi: 10.1080/01904160902943197
Plaza, B. M., Gómez-Serrano, C., Acién-Fernández, F. G., and Jimenez-Becker, S. (2018). Effect of microalgae hydrolysate foliar application (Arthrospira platensis and Scenedesmus sp.) on Petunia x hybrida growth. J. Appl. Phycol. 30, 2359–2365. doi: 10.1007/s10811-018-1427-0
Ponder, A., and Hallmann, E. (2019). The effects of organic and conventional farm management and harvest time on the polyphenol content in different raspberry cultivars. Food Chem. 301:125295. doi: 10.1016/j.foodchem.2019.125295
Ponder, A., and Hallmann, E. (2020). The nutritional value and vitamin C content of different raspberry cultivars from organic and conventional production. J. Food Compos. Anal. 87:103429. doi: 10.1016/j.jfca.2020.103429
Poore, J., and Nemecek, T. (2018). Reducing food’s environmental impacts through producers and consumers. Science 992, 987–992. doi: 10.1126/science.aaq0216
ProFound Advisers in Development, Organics and Development, Markus Arbenz (2020). Boosting Organic Trade in Africa, IFOAM - Organics International, Bonn / Deutsche Gesellschaft für Internationale Zusammenarbeit (GIZ) GmbH, Bonn / Eschborn.
Pylak, M., Oszust, K., and Frąc, M. (2019). Review report on the role of bioproducts, biopreparations, biostimulants and microbial inoculants in organic production of fruit. Rev. Environ. Sci. Biotechnol. 18, 597–616. doi: 10.1007/s11157-019-09500-5
Rachtan-Janicka, J., Ponder, A., and Hallmann, E. (2021). The effect of organic and conventional cultivations on antioxidants content in blackcurrant (Ribes nigrum l.) species. Appl. Sci. 11. doi: 10.3390/app11115113
Rafeii, S., and Pakkish, Z. (2014). Improvement of vegetative and reproductive growth of ‘camarosa’ strawberry: role of humic acid, Zn, and B. Agric. Conspec. Sci. 79, 239–244.
Rahman, M., Sabir, A. A., Mukta, J. A., Khan, M. M. A., Mohi-Ud-Din, M., Miah, M. G., et al. (2018). Plant probiotic bacteria Bacillus and Paraburkholderia improve growth, yield and content of antioxidants in strawberry fruit. Sci. Rep. 8, 2504–2511. doi: 10.1038/s41598-018-20235-1
Ramos-Solano, B., Garcia-Villaraco, A., Gutierrez-Mañero, F. J., Lucas, J. A., Bonilla, A., and Garcia-Seco, D. (2014). Annual changes in bioactive contents and production in field-grown blackberry after inoculation with Pseudomonas fluorescens. Plant Physiol. Biochem. 74, 1–8. doi: 10.1016/j.plaphy.2013.10.029
Rana, J., and Paul, J. (2017). Journal of retailing and consumer services consumer behavior and purchase intention for organic food: A review and research agenda. J. Retail. Consum. Serv. 38, 157–165. doi: 10.1016/j.jretconser.2017.06.004
Raspberry, O., Commission, BFarms, N., Giant, C., and Farms, B.Group, S. M, et al. (2010). Recent trends and advances in berry health benefits. Adv. Nutr. 58, 3869–3870. doi: 10.1021/jf902806j
Righini, H., Roberti, R., and Baraldi, E. (2018). Use of algae in strawberry management. Hydrobiologia 30, 3551–3564. doi: 10.1007/s10811-018-1478-2
Rodrigues, J. P. B., Liberal, Â., Petropoulos, S. A., Ferreira, I. C. F. R., Oliveira, M. B. P. P., Fernandes, Â., et al. (2022). Agri-food surplus, waste and loss as sustainable biobased ingredients: a review. Molecules 27:5200. doi: 10.3390/molecules27165200
Romanazzi, G., and Feliziani, E. (2016). “Use of chitosan to control postharvest decay of temperate fruit: effectiveness and mechanisms of action” in Chitosan in the Preservation of Agricultural Commodities (Elsevier).
Romanazzi, G., Feliziani, E., Baños, S. B., and Sivakumar, D. (2015). Shelf life extension of fresh fruit and vegetables by chitosan treatment. Crit. Rev. Food Sci. Nutr. 57, 37–41. doi: 10.1080/10408398.2014.900474
Rom, C. R., Garcia, M. E., Johnson, D. T., Popp, J., Friedrich, H., and McAfee, J. (2010). High tunnel production of organic blackberries and raspberries in Arkansas. Acta Hortic. 873, 269–276. doi: 10.17660/ActaHortic.2010.873.29
Ronga, D., Biazzi, E., Parati, K., Carminati, D., Carminati, E., and Tava, A. (2019). Microalgal biostimulants and biofertilisers in crop productions. Agronomy 9, 1–22. doi: 10.3390/agronomy9040192
Rostami, M., Shokouhian, A., and Mohebodini, M. (2022). Effect of humic acid, nitrogen concentrations and application method on the morphological, yield and biochemical characteristics of strawberry ‘Paros’. Int. J. Fruit Sci. 22, 203–214. doi: 10.1080/15538362.2021.2022566
Rouphael, Y., and Colla, G. (2020). Editorial: Biostimulants in Agriculture. Front. Plant Sci. 11:40. doi: 10.3389/fpls.2020.00040
Roussos, P. A., Triantafillidis, A., Kepolas, E., Peppas, P., Piou, A., Zoti, M., et al. (2022). Effects of integrated and organic management on strawberry (cv. Camarosa) plant growth, nutrition, fruit yield, quality, nutraceutical characteristics, and soil fertility status. Horticulturae 8:184. doi: 10.3390/horticulturae8020184
Rueda, D., Valencia, G., Soria, N., Rueda, B. B., Manjunatha, B., Kundapur, R. R., et al. (2016). Effect of Azospirillum spp. and Azotobacter spp. on the growth and yield of strawberry (Fragaria vesca) in hydroponic system under different nitrogen levels. J. Appl. Pharm. Sci. 6, 048–054. doi: 10.7324/JAPS.2016.600108
Sablani, S. S., Andrews, P. K., Davies, N. M., Walters, T., Saez, H., and Bastarrachea, L. (2011). Effects of air and freeze drying on phytochemical content of conventional and organic berries. Dry. Technol. 29, 205–216. doi: 10.1080/07373937.2010.483047
Saidimoradi, D., Ghaderi, N., and Javadi, T. (2019). Salinity stress mitigation by humic acid application in strawberry (Fragaria x ananassa Duch.). Sci. Hortic. 256:108594. doi: 10.1016/j.scienta.2019.108594
Salo, H. M., Nguyen, N., Alakärppä, E., Klavins, L., Hykkerud, A. L., Karppinen, K., et al. (2021). Authentication of berries and berry-based food products. Compr. Rev. Food Sci. Food Saf. 20, 5197–5225. doi: 10.1111/1541-4337.12811
Sangiorgio, D., Cellini, A., Spinelli, F., Farneti, B., Khomenko, I., Muzzi, E., et al. (2021). Does organic farming increase raspberry quality, aroma and beneficial bacterial biodiversity? Microorganisms 9. doi: 10.3390/microorganisms9081617
Santini, G., Biondi, N., Rodolfi, L., and Tredici, M. R. (2021). Plant biostimulants from cyanobacteria: an emerging strategy to improve yields and sustainability in agriculture. Plan. Theory 10:643. doi: 10.3390/plants10040643
Saritha, M., and Tollamadugu, N. V. K. V. P. (2019). “The status of research and application of biofertilizers and biopesticides: global scenario” in Recent Developments in Applied Microbiology and Biochemistry. Elsevier.
Sato, J. (2018). Productivity and quality of organic strawberries pre-harvest treated with silicon. Hortic. Bras. 36, 40–46. doi: 10.1590/s0102-053620180107
Schoebitz, M., López, M. D., Serri, H., Aravena, V., Zagal, E., Roldán, A., et al. (2019). Characterization of bioactive compounds in blueberry and their impact on soil properties in response to plant biostimulants characterization of bioactive compounds in blueberry and their impact on soil properties in response to plant biostimulants. Commun. Soil Sci. Plant Anal. 50, 2482–2494. doi: 10.1080/00103624.2019.1667374
Schoebitz, M., López, M. D., Serrí, H., Martínez, O., and Zagal, E. (2016). Combined application of microbial consortium and humic substances to improve the growth performance of blueberry seedlings. Rev. Ciencia Suelo Nutr. Veg. 16, 1010–1023. doi: 10.4067/S0718-95162016005000074
Scortichini, M. (2022). Sustainable management of diseases in horticulture: conventional and new options. Horticulturae 8:517. doi: 10.3390/horticulturae8060517
Seema, K., Mehta, K., and Singh, N. (2018). Studies on the effect of plant growth promoting rhizobacteria (PGPR) on growth, physiological parameters, yield and fruit quality of strawberry cv. Chandler. J. Pharmacog. Phytochem. 7, 383–387.
Senger, E., Osorio, S., Olbricht, K., Shaw, P., Predieri, S., Karhu, S., et al. (2022). Towards smart and sustainable development of modern berry cultivars in. Europe 111, 1238–1251. doi: 10.1111/tpj.15876
Sharma, H. S. S., Fleming, C., Selby, C., Rao, J. R., and Martin, T. (2014). Plant biostimulants: a review on the processing of macroalgae and use of extracts for crop management to reduce abiotic and biotic stresses. J. Appl. Phycol. 26, 465–490. doi: 10.1007/s10811-013-0101-9
Shukla, P. S., Mantin, E. G., Adil, M., Bajpai, S., Critchley, A. T., and Prithiviraj, B. (2019). Ascophyllum nodosum-based biostimulants: sustainable applications in agriculture for the stimulation of plant growth, stress tolerance, and disease management. Front. Plant Sci. 10:655. doi: 10.3389/fpls.2019.00655
Singh, U. B., Malviya, D., Wasiullah, S., Singh, S., Pradhan, J. K., Singh, B. P., et al. (2016). Bio-protective microbial agents from rhizosphere eco-systems trigger plant defense responses provide protection against sheath blight disease in rice (Oryza sativa L.). Microbiol. Res. 192, 300–312. doi: 10.1016/j.micres.2016.08.007
Skrovankova, S., Sumczynski, D., Mlcek, J., Jurikova, T., and Sochor, J. (2015). Bioactive compounds and antioxidant activity in different types of berries. Int. J. Mol. Sci. 16, 24673–24706. doi: 10.3390/ijms161024673
Smith, L. G., Williams, A. G., and Pearce, B. D. (2015). The energy efficiency of organic agriculture: A review. Renew. Agric. Food Syst. 30, 280–301. doi: 10.1017/S1742170513000471
Sobekova, K., Thomsen, M. R., and Ahrendsen, B. L. (2011). Market trends and consumer demand for fresh berries. Appl. Stud. Agribus. Commer. 7, 11–14. doi: 10.19041/Apstract/2013/2-3/1
Song, D., Chen, X., Wang, M., Wu, Z., and Xiao, X. (2023). 3D-printed flexible sensors for food monitoring. Chem. Eng. J. 474:146011. doi: 10.1016/j.cej.2023.146011
Soppelsa, S., Kelderer, M., Casera, C., Bassi, M., Robatscher, P., Matteazzi, A., et al. (2019). Foliar applications of biostimulants promote growth, yield and fruit quality of strawberry plants grown under nutrient limitation. Agronomy 9, 1–22. doi: 10.3390/agronomy9090483
Spinelli, F., Fiori, G., Noferini, M., Sprocatti, M., and Costa, G. (2010). A novel type of seaweed extract as a natural alternative to the use of iron chelates in strawberry production. Sci. Hortic. 125, 263–269. doi: 10.1016/j.scienta.2010.03.011
Talukder, M. R., Asaduzzaman, M., Tanaka, H., and Asao, T. (2018). Light-emitting diodes and exogenous amino acids application improve growth and yield of strawberry plants cultivated in recycled hydroponics. Scientia Horticulturae 239, 93–103. doi: 10.1016/j.scienta.2018.05.033
Tarakhovskaya, E. R., Maslov, Y. I., and Shishova, M. F. (2007). Phytohormones in algae. Russ. J. Plant Physiol. 54, 163–170. doi: 10.1134/S1021443707020021
Tehranifar, A., and Ameri, A. (2014). Effect of humic acid on nutrient uptake and physiological characteristics of Fragaria ×ananassa “Camarosa.”. Acta Hortic. 1049, 391–394. doi: 10.17660/ActaHortic.2014.1049.54
Thi, L., Sen, H., Bond, J., Tien, N., and Gia, H. (2021). Farmers’ barriers to the access and use of climate information in the mountainous regions of Thừa Thiˆen Huế province, Vietnam. Clim. Serv. 24:100267. doi: 10.1016/j.cliser.2021.100267
Tiwari, S., Lata, C., Chauhan, P. S., and Nautiyal, C. S. (2016). Pseudomonas putida attunes morphophysiological, biochemical and molecular responses in Cicer arietinum L. During drought stress and recovery. Plant Physiol. Biochem. 99, 108–117. doi: 10.1016/j.plaphy.2015.11.001
Todeschini, V., Aitlahmidi, N., Mazzucco, E., Marsano, F., Gosetti, F., Robotti, E., et al. (2018). Impact of beneficial microorganisms on strawberry growth, fruit production, nutritional quality, and volatilome. Front. Plant Sci. 9:1611. doi: 10.3389/fpls.2018.01611
Tripathi, S., Nayan, R., Shrivastava, N., Singh, S., Chatterjee, A., Varma, A., et al. (2022). Field crops research microbial biofortification: a sustainable route to grow nutrient-rich crops under changing climate. Field Crop Res. 287:108662. doi: 10.1016/j.fcr.2022.108662
Tripathi, V. K., Kumar, S., Kumar, K., Kumar, S., and Dubey, V. (2016). Influence of Azotobacter, Azospirillum and PSB on vegetative growth, flowering, yield and quality of strawberry cv. Chandler. Progress. Hortic. 48:48. doi: 10.5958/2249-5258.2016.00009.9
Trzciński, P., Frąc, M., Lisek, A., Przybył, M., Frąc, M., and Sas-Paszt, L. (2021). Growth promotion of raspberry and strawberry plants by bacterial inoculants. Acta Sci. Polonor. Hortor. Cultus 20, 71–82. doi: 10.24326/asphc.2021.6.8
UNCTAD. (2019). World Investment Report 2019: Special economic zones. New York and Geneva: United Nations, 126–206.
Vaikuntapu, P. R., Dutta, S., Samudrala, R. B., Rao, V. R. V. N., Kalam, S., and Podile, A. R. (2014). Preferential promotion of Lycopersicon esculentum (tomato) growth by plant growth promoting bacteria associated with tomato. Indian J. Microbiol. 54, 403–412. doi: 10.1007/s12088-014-0470-z
Velickova, E., Winkelhausen, E., Kuzmanova, S., and Alves, V. D. (2013). Impact of chitosan-beeswax edible coatings on the quality of fresh strawberries (Fragaria ananassa cv Camarosa) under commercial storage conditions. LWT Food Sci. Technol. 52, 80–92. doi: 10.1016/j.lwt.2013.02.004
Vioratti Telles de Moura, O., Luiz Louro Berbara, R., França de Oliveira Torchia, D., Fernanda Oliveira da Silva, H., Augusto van Tol de Castro, T., Carlos Huertas Tavares, O., et al. (2023). Humic foliar application as sustainable technology for improving the growth, yield, and abiotic stress protection of agricultural crops. A review. J. Saudi Soc. Agric. Sci. 22, 493–513. doi: 10.1016/j.jssas.2023.05.001
Walters, D. R., Ratsep, J., and Havis, N. D. (2013). Controlling crop diseases using induced resistance: challenges for the future. J. Exp. Bot. 64, 1263–1280. doi: 10.1093/jxb/ert026
Wang, M., Sun, H., and Xu, Z. (2022). Analysis of blueberry plant rhizosphere bacterial diversity and selection of plant growth promoting rhizobacteria. Curr. Microbiol. 79, 331–313. doi: 10.1007/s00284-022-03031-z
Wang, S. Y., Chen, C. T., Sciarappa, W., Wang, C. Y., and Camp, M. J. (2008). Fruit quality, antioxidant capacity, and flavonoid content of organically and conventionally grown blueberries. J. Agric. Food Chem. 56, 5788–5794. doi: 10.1021/jf703775r
Weber, N., Schmitzer, V., Jakopic, J., and Stampar, F. (2018). First fruit in season: seaweed extract and silicon advance organic strawberry (Fragaria × ananassa Duch.) fruit formation and yield. Sci. Hortic. 242, 103–109. doi: 10.1016/j.scienta.2018.07.038
Wei, X., Zhang, W., Zulfiqar, F., Zhang, C., and Chen, J. (2022). Ericoid mycorrhizal fungi as biostimulants for improving propagation and production of ericaceous plants. Front. Plant Sci. 13:1027390. doi: 10.3389/fpls.2022.1027390
Wenhai, L., Cusack, C., Baker, M., Tao, W., Mingbao, C., Paige, K., et al. (2019). Successful blue economy examples with an emphasis on international perspectives. Science 6:261. doi: 10.3389/fmars.2019.00261
Willer, H., Schlatter, B., Trávníˇcek, J., Kemper, L., and Lernoud, J. (2020). The World of Organic Agriculture Statistics and Emerging Trends 2020. Frick, Switzerland: FiBL; Bonn, Germany: IFOAM. Available online: https://shop.fibl.org/CHen/mwdownloads/download/link/id/1294/?ref=1 (Accessed March 8, 2020).
Wittwer, R. A., Bender, S. F., Hartman, K., Hydbom, S., Lima, R. A. A., Loaiza, V., et al. (2021). Organic and conservation agriculture promote ecosystem multifunctionality. Sci. Adv. 7:eabg6995. doi: 10.1126/sciadv.abg6995
Xie, J., Shu, P., Strobel, G., Chen, J., Wei, J., and Xiang, Z. (2017). Pantoea agglomerans SWg2 colonizes mulberry tissues, promotes disease protection and seedling growth. Biol. Control 113, 9–17. doi: 10.1016/j.biocontrol.2017.06.010
Xu, L., Geelen, D., Hadavi, E., Geelen, D., and Xu, L. (2018). Developing biostimulants from agro-food and industrial by-products. Front. Plant Sci. 9:1567. doi: 10.3389/fpls.2018.01567
Yadav, S. K., Khokhar, U. U., and Yadav, R. P. (2010). Integrated nutrient management for strawberry cultivation. Ind. J. Hortic. 78, 445–450. doi: 10.5958/0974-0112.2021.00064.5
Yamamoto, S., Shiraishi, S., and Suzuki, S. (2014). Are cyclic lipopeptides produced by Bacillus amyloliquefaciens S13-3 responsible for the plant defence response in strawberry against Colletotrichum gloeosporioides? Lett. Appl. Microbiol. 60, 379–386. doi: 10.1111/lam.12382
Zhang, H., Xu, N., Li, X., Long, J., Sui, X., Wu, Y., et al. (2018). Arbuscular mycorrhizal fungi (Glomus mosseae) improves growth, photosynthesis and protects photosystem II in leaves of lolium perenne L. in cadmium contaminated soil. Front. Plant Sci. 9, 1–16. doi: 10.3389/fpls.2018.01156
Zulfiqar, F., Moosa, A., Ali, H. M., Bermejo, N. F., and Munn, S. (2024). A sufficiently effective tool for sustainable agriculture in the era of climate change? Plant Physiol. Biochem. Biostimul. 211:108699. doi: 10.1016/j.plaphy.2024.108699
Keywords: plant biostimulants, biopesticide, organic berry, sustainable agriculture, African agriculture, food system, postharvest
Citation: Mounaimi S, Hamim A, El Boukhari MEM, Elarroussi H and Lyamlouli K (2024) A comprehensive review of integrating biostimulants and biopesticides for organic berry farming: exploring challenges and opportunities for Africa. Front. Sustain. Food Syst. 8:1452823. doi: 10.3389/fsufs.2024.1452823
Edited by:
Muhammad Saqlain Zaheer, Khwaja Fareed University of Engineering and Information Technology (KFUEIT), PakistanReviewed by:
Juan Fernando Hirzel, Agricultural Research Institute (Chile), ChileHafiz M. Usman Aslam, Colorado State University, United States
Copyright © 2024 Mounaimi, Hamim, El Boukhari, Elarroussi and Lyamlouli. This is an open-access article distributed under the terms of the Creative Commons Attribution License (CC BY). The use, distribution or reproduction in other forums is permitted, provided the original author(s) and the copyright owner(s) are credited and that the original publication in this journal is cited, in accordance with accepted academic practice. No use, distribution or reproduction is permitted which does not comply with these terms.
*Correspondence: Sana Mounaimi, bW91bmFpbWkuc2FuYUB1bTZwLm1h; Karim Lyamlouli, a2FyaW0ubHlhbWxvdWxpQHVtNnAubWE=