- 1Environmental Systems Analysis Group, Department of Geosciences, Eberhard Karls University of Tübingen, Tübingen, Germany
- 2Sustainable Food Systems Engineering Group, Department of Food Science and Technology, University of Georgia, Athens, GA, United States
- 3Environmental Biotechnology Group, Department of Geosciences, University of Tübingen, Tübingen, Germany
- 4AG Angenent, Max Planck Institute for Developmental Biology, Tübingen, Germany
- 5The Novo Nordisk Foundation CO2 Research Center (CORC), Aarhus University, Aarhus, Denmark
- 6Cluster of Excellence – Controlling Microbes to Fight Infections, University of Tübingen, Tübingen, Germany
- 7New Materials Institute, University of Georgia, Athens, GA, United States
- 8Institute for Integrative and Precision Agriculture, University of Georgia, Athens, GA, United States
Food insecurity in Ethiopia is an immediate humanitarian crisis that is expected to worsen due to population growth and climate change. This study applied GIS-based approaches to evaluate the feasibility of deploying an emergent type of single-cell protein (SCP) technology to supplement the nutritional needs of Ethiopian citizens who are most vulnerable to drought. The technology—power-to-protein (PtP)—uses H2 and O2 from water electrolysis and CO2 from woody biomass combustion in a two-stage bioprocess to produce nutrient-rich protein powder for human consumption. Population density, land use, and other geographical data were used to identify optimal site locations for these PtP systems based on two deployment strategies: large centralized plants vs. small decentralized units. The model also accounted for biomass availability, collection, and distribution logistics. The analysis revealed three sites that are both (highly) vulnerable/food-insecure and accessible within walking distance. The identified sites are proximate to the urban areas of Mekele in northern Ethiopia, Addis Ababa in central Ethiopia, and Hawasa south of Addis Ababa. If centralized PtP were deployed, the protein requirements of these populations could be sustained for several months, assuming a modest biomass collection radius of 35 km. Decentralized PtP deployment was similarly effective, requiring a distribution density of 5.4–11.0 PtP units per km2 under conservative estimates and 0.76–1.1 units per km2 under optimistic estimates. Lastly, a theoretical comparison showed that PtP is more efficient than conventional agricultural food production regarding biomass-to-protein conversion yields. Overall, our study suggests that PtP technology would be a feasible approach to supplement the nutritional needs of Ethiopian people in times of drought-related emergencies. However, given logistical limitations and considering social preference factors, it would be more practical to implement PtP in conjunction with standard emergency food aid measures.
1 Introduction
Anthropogenic greenhouse gas emissions (GHGs), land use change, and other activities that impact the global carbon balance are changing the climate. As a result, the frequency and severity of extreme weather and climate events are increasing (Teshome and Zhang, 2019). Droughts are among the most severe natural disasters affecting ecosystems, agriculture, and human societies because of their long-term impacts on water resource availability, agricultural production, and economic activity (Edwards et al., 2019; Naumann et al., 2021; Orimoloye et al., 2022). Drought can be defined as an anomalous moisture deficit relative to a normal baseline (Wilhite and Glantz, 1985). Many processes contribute to a drought event beyond simple climatic parameters such as temperature and precipitation. Thus, the scientific study of drought spans various fields such as meteorology, climatology, hydrology, ecology, and agronomy. In recent years, the focus on drought vulnerabilities has increased due to multiple drought events in many regions of the world such as the United States (Ault, 2020) East Africa (Anderson et al., 2023), Australia (Hoque et al., 2021), and the Sahel (Noureldeen et al., 2020).
Global aridity is expected to increase in many regions as global temperatures continue to rise due to climate change. Aridity is a permanent deficit of water availability that is caused by low average annual precipitation or high spatial or temporal variability. The low moisture levels restrict the carrying capacity of the local environment (i.e., the maximum population size of a species in an ecosystem that can be sustained by the available food, water, and resources) (Wang, 2022). Precipitation and evapotranspiration are expected to increase overall through an intensified hydrological cycle that is fueled by a warmer climate (Ficklin et al., 2022). Projections predict certain regions of the world will receive more precipitation (mesic areas, e.g., wet tropics) while others will receive less (semi-arid regions, e.g., subtropics) (Chou et al., 2009; Chou et al., 2013; Dong et al., 2020; Seager et al., 2010). Moreover, evapotranspiration is expected to increase in already dry areas, leading to an even more arid climate. Global warming exacerbates this effect, leading to higher frequencies of drought.
The impacts of droughts on agriculture can result in famine and forced migration, natural resource degradation, and suppressed economic activity. There are direct and indirect effects that follow a drought event. The primary direct impacts of droughts in the agricultural sector are reduced production and increased food prices through crop failure and pasture losses. Indirect effects can include reduced supplies to downstream industries (e.g., food producers) (Trnka et al., 2020).With nearly 1.4 billion people (18% of the world population) relying on agriculture as the primary source of income, droughts are putting the livelihood of many at risk (Meza et al., 2020). Especially vulnerable are communities that rely on agriculture as their primary source of nutrition, which is often the case in low-to medium-income countries (Moges and Bhat, 2021). Rainfed agriculture is the leading practice in those countries, and if they are affected by drought, their food supply can be at risk (Jaramillo et al., 2020). Therefore, identifying strategies to strengthen drought resilience in those countries is a high priority.
Developing sustainable technologies to circumvent or at least complement conventional agriculture is imperative. Power-to-protein (PtP) is a promising technology that offers solutions to some of the issues that are caused by a growing world population (Molitor et al., 2019; Sillman et al., 2020). PtP combines water electrolysis and gas fermentation to convert carbon dioxide (CO2), hydrogen (H2), and oxygen (O2) gas into protein for human food or animal feed, using (renewable) electric power (Mishra et al., 2020). The protein from these single-celled organisms is called single-cell protein (SCP). Compared to conventional animal-or plant-based protein production, PtP confers considerable environmental advantages such as lower land requirements, water requirements, and fewer GHG emissions (Salazar-López et al., 2022). Moreover, PtP can be operated in self-contained bioreactors independent of arable land, liberating it from climate and geography. Nutrient utilization efficiency in bioreactor systems is also considerably higher than in conventional agriculture, reducing the demand for synthetic fertilizers, which have a high GHG footprint (Pikaar et al., 2017). In addition, PtP production reduces the need for expanding agricultural land, lessening deforestation, habitat destruction, and biodiversity loss (Humpenöder et al., 2022). Finally, PtP qualifies as a carbon capture and utilization technology when the CO2 is sourced from industrial off gases, power plants, biomass processing facilities (e.g., biogas and bioethanol plants), or direct air capture.
PtP bioreactor systems typically involve one or two stages. For instance, hydrogen-oxidizing bacteria (HOB) can be grown on H2, O2, and CO2 to produce microbial biomass in a single-stage bioreactor (Hu et al., 2020). Alternatively, intermediate compounds, such as methane or acetate, can be made from H2 and CO2 fermentation in a first-stage bioreactor and then fed to microbes to produce biomass in a second-stage bioreactor. Mishra et al. (2020) performed a theoretical analysis of various SCP technologies and determined that the two-stage bioprocess would yield more protein than the single-stage HOB process for a given amount of available energy. In addition, yeast can be selected for growth in the two-stage bioprocess, providing a more socially accepted protein source than the bacterial biomass grown in the HOB process. Yeast also contains high levels of protein, with a broad spectrum of essential amino acids, lipids, complex carbohydrates, vitamins, and minerals (Bratosin et al., 2021; Salazar-López et al., 2022; Schmitz et al., 2024; Yang et al., 2022).
Ethiopia is Africa’s second most populated country after Nigeria. Over 80% of Ethiopia’s population depends on rainfed agriculture for their livelihoods (Mohamed, 2017). Since agricultural production primarily serves subsistence purposes (CSA, 2021), the population is particularly vulnerable to climate variability and weather-related shocks such as droughts. Ethiopia has a history of severe droughts, which have worsened in recent years (Sohnesen, 2020). These droughts put much of the Ethiopian population at risk (Mohamed, 2017). Ethiopia is already one of the largest recipients of humanitarian food assistance (Teshome and Zhang, 2019). Therefore, improving the resilience of the Ethiopian population to droughts and the associated food shortages is critical. The deployment of PtP as a complementary technology to conventional agriculture can bolster the self-sufficiency and security of the Ethiopian people.
Here, this GIS and technological modeling study aimed to identify the regions in Ethiopia most vulnerable to drought and assess the feasibility of using two-stage PtP technology as a disaster relief measure. In this hypothetical disaster-relief scenario, drought-afflicted crops would be combusted to produce the CO2 needed for the PtP process. The protein generated from the PtP system would then supply the nutritional needs of Ethiopian citizens during these food shortages. We modeled two PtP deployment strategies –centralized and decentralized—for the entire country of Ethiopia and compared their feasibility considering local protein demand, drought vulnerability, and feedstock supply logistics. Finally, we assessed the resource-use efficiency of PtP technology relative to conventional food production under non-drought conditions to inform future development and policy decisions.
2 Methodology
2.1 Study area and study period
With a total population of approximately 127 M people, Ethiopia is Africa’s second most populated country. This results in a population density of 128.7 people per km2 (UN, 2024). While predominantly rural, Ethiopia has seen increasing urbanization. The capital, Addis Ababa, is the largest city, with ~4 million people, followed by Dire Dawa and Mekelle. Ethiopia is characterized by significant socio-economic diversity, marked by rapid growth, widespread poverty, and a predominantly agrarian economy. Indeed, agriculture underpins much of Ethiopia’s economy, contributing around 40% of the gross domestic product and employing about 75% of the population (USAID, n.d.). There is a significant rural–urban divide, with urban areas experiencing better access to education, healthcare, and employment opportunities, while rural regions, especially in areas prone to conflict or drought, have limited access (Tom et al., 2020). Geographically, Ethiopia is one of Africa’s largest countries and is home to various landscapes and climatic zones. Ethiopia’s climate is dictated by the seasonal intertropical convergence zone and the associated atmospheric circulation patterns (Beltrando and Camberlin, 1993). The diverse topography also creates regional climates. The shift from lowlands to highlands establishes a gradient of environments throughout the country, encompassing extremely arid to highly humid conditions (Fazzini et al., 2015) (Figure 1). The diverse topography also affects the temperature and precipitation patterns across the country. There are three seasons in Ethiopia: (1) the dry season – which extends from Oct. to Jan., (2) the short rainy season – which extends from Feb. to May, and (3) the rainy season (‘kiremt’), which extends from Jun. to Sep. (Asfaw et al., 2018). Most annual crop production in the country depends on the rainfall of the kiremt season. Overall, crops are cultivated on a total area of 145,638.8 km2, with teff, maize, and sorghum being the three most cultivated crops with regard to the the cultivated area (CSA, 2021). The harvest season (‘meher’) follows kiremt, extending from Sep. to Feb., and accounts for over 90% of annual harvested crops (Dorosh and Rashid, 2013, p. 61). Analysis of long-term precipitation data revealed that, on average, January is the driest month (Elzopy et al., 2021). January is also when crop growth is at its fullest. Therefore, January was selected as the focal month for the analysis because this is the period most vulnerable to drought.
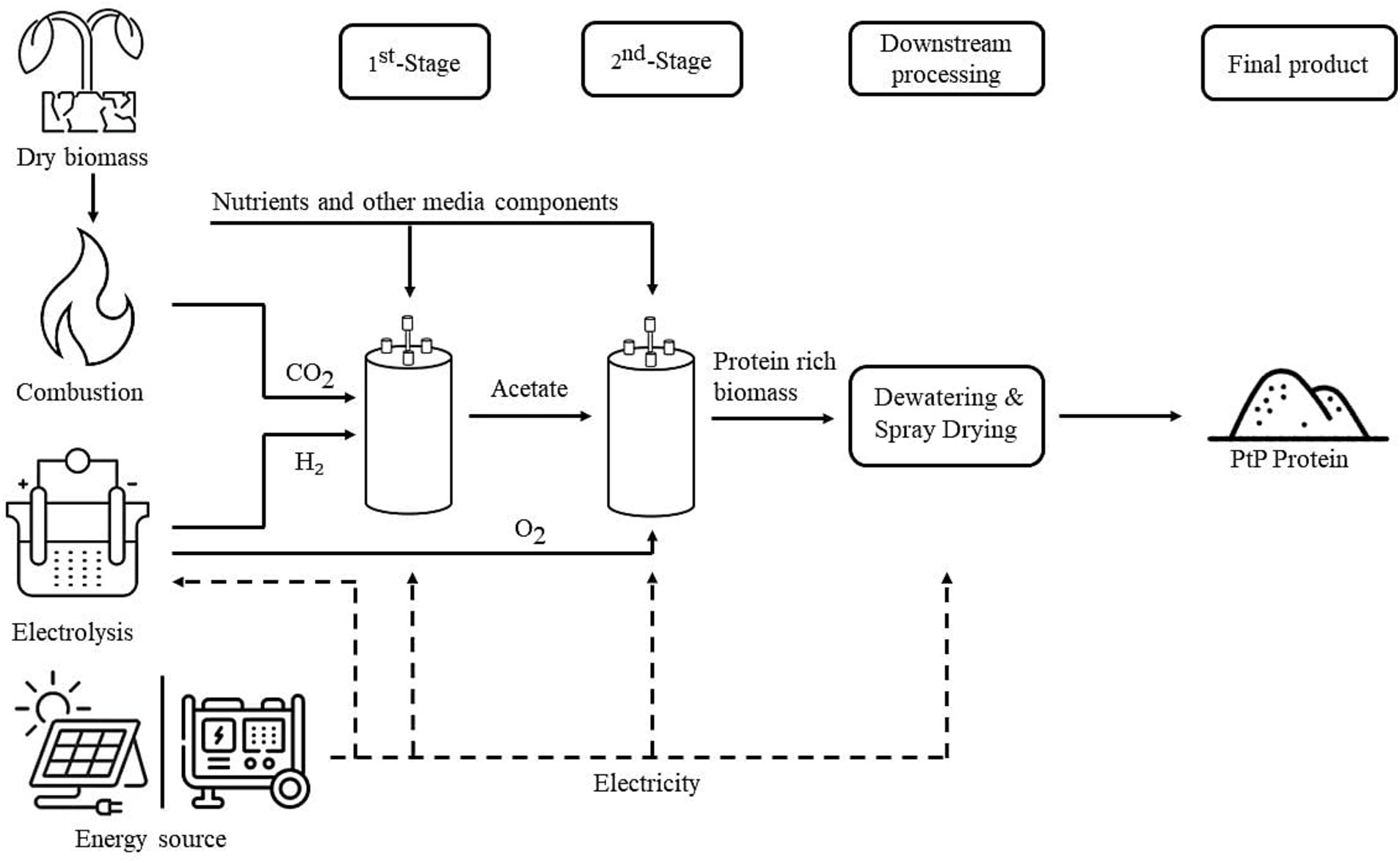
Figure 1. Overview of the PtP bioprocess, including substrates, pre-processing steps, bioreactor stages, downstream processing, and power supply.
2.2 Power-to-protein design and implementation
An efficient PtP systems is the two-stage PtP bioprocess with acetate as an intermediate (Mishra et al., 2020). Therefore, this PtP system was selected as the model system for disaster relief in Ethiopia. Here, drought-affected woody biomass is combusted to provide oxygen-cleaned CO2 as the carbon-source for the PtP process. At the same time, H2O is electrolyzed to provide H2 and O2 to serve as the electron donor and electron acceptor, respectively, for microbial growth and metabolism. In the 1st-Stage bioreactor, the CO2 and H2 are fed to acetogenic bacteria (i.e., Clostridium ljungdahlii PETC [DSM 13528]), which produce acetate. Next, the acetate and O2 are fed to yeast (i.e., Saccharomyces cerevisiae S288C [ATCC 204508]) in the 2nd-Stage bioreactor, generating protein-rich yeast biomass. (Figure 2). The 1st-and 2nd-Stage bioreactors are supplied with nutrient media containing nitrogen, minerals, vitamins, and trace metals. The protein is extracted from the raw biomass through a series of downstream processes, including harvesting, heat-shock treatment, dewatering, and spray drying. The final product the PtP system generates is a protein powder with 45% protein by weight. Nutrient and H2O-containing effluents produced during downstream processing are recycled to the 1st-and 2nd-Stage bioreactors to improve resource-use efficiency.
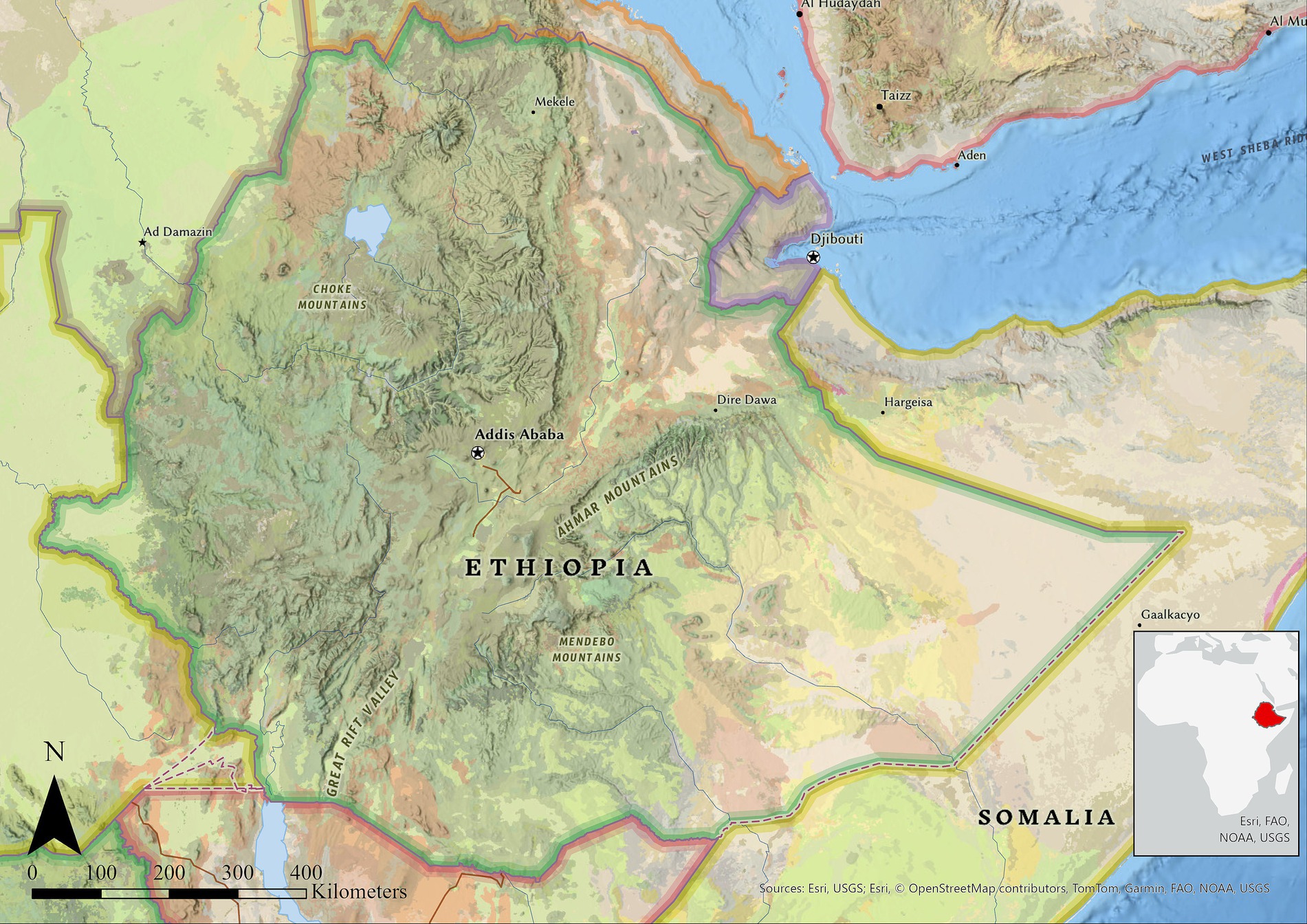
Figure 2. Geographical overview of Ethiopia. Ethiopia has a wide variety of landscapes, including pronounced highland and lowland areas. The varying topography of the country gives rise to distinct climatic zones, including arid regions (illustrated in yellow and brown) and humid areas (illustrated in green).
Two PtP deployment strategies were modeled to evaluate the logistical feasibility of PtP implementation: centralized and decentralized. Centralized PtP facilities were modeled and scaled based on crop biomass availability within a defined collection radius around the centralized site. Decentralized PtP units were designed to fit within modular shipping containers for mobility. Multiple PtP units would be deployed to drought-affected locations to meet the local protein demand. The number of units deployed was determined based on unit throughput and local biomass availability. The electricity for electrolysis and equipment operation in the centralized PtP facilities would be supplied by grid power. In contrast, a combination of grid power, solar panels, and diesel generators would provide electricity for the decentralized PtP units. Further details regarding the model assumptions and system design are given in Supplementary Tables S1, S2.
2.3 Datasets
Data from GIS datasets were used to define a reference map of food-insecure regions in Ethiopia. Data included: (1) land cover (LC), (2) population density (PD), (3) population count (PC), and (4) drought occurrence in Ethiopia during January 2020. The reference map was cross-evaluated with Ethiopia’s existing road network data to determine PtP site locations. Crop yield data for the 2020 meher season was retrieved from Ethiopia’s Central Statistical Agency (CSA) and used to calculate total biomass production. Detailed information on agricultural data can be found in Supplementary Table S3.
2.3.1 Landcover
Landcover data was obtained from the European Space Agency. The dataset provides a one-year temporal resolution and 300-m spatial resolution of landcover for 1992 through 2020. The European Space Agency generated the dataset assuming baseline landcover and annual landcover changes. Baseline data was developed using various machine learning and image classification methods from the ENVISAT mission archive for the period from 2003 to 2012. Annual landcover changes were measured from 1992 to 2018 at 1 km resolution from various satellite observations. The landcover data were classified using the classification system outlined by the Food and Agriculture Organization (FAO) (ESA, 2017).
2.3.2 Population density and population count
Population density and population count data was obtained from the WorldPop organization. WorldPop utilizes a statistical model that incorporates census and projection data based on administrative units, settlement data (i.e., buildings, settlement types), and detailed geospatial information (i.e., elevation, slope, vegetation, and accessibility to major cities) to generate population density and population count datasets at a resolution of 1 km and 100 m, respectively (WorldPop, 2020).
2.3.3 Drought occurrence
Drought occurrence data was gathered from the National Oceanic and Atmospheric Administration (NOAA). Global and regional vegetation health products (VHP) is a classification system that estimates vegetation health using various indices such as plant greenness, thermal parameters (surface temperatures), and their interactions. VHP provides a vegetation health index (VHI) derived from advanced very high-resolution radiometers onboard polar-orbiting satellites. VHP data with a 4-km spatial resolution and 7-day composite temporal resolution were generated from 1981 to the present. VHP was used as proxy data for vegetation health and drought. A VHP value below 40 indicates unfavorable conditions characterized by drought stress in plants, which may result in crop loss (NOAA, 2022).
2.4 Evaluation of food insecurity vulnerability
Data regarding arable land, population density, and VHI were used to qualify drought conditions and estimate food insecurity vulnerability. First, agricultural areas were defined by analyzing individual pixels based on the landcover dataset. Areas impacted by drought were cross-evaluated with population density and VHI data. Food insecurity was defined using the following two criteria. Criterion (1): a population density value equal to or above the 95th percentile. This range of population density values ensured that agricultural areas with high population densities were selected. A high population density indicates that many people would be affected by drought due to crop loss. Criterion (2): a VHI value below 40. This range of VHI values indicates adverse impacts on crops, including the initiation of crop mortality (NOAA, 2022). Pixels that meet the criteria were considered food insecure areas in this study. A heatmap was created to show the areas vulnerable to food insecurity.
2.5 Site determination for PtP deployment
An index was created to determine possible PtP deployment sites in a drought scenario, based on the VHI and population density criteria, using the calculate composite index tool in ArcGIS Pro software (v3.1.1, ESRI, Redlands, CA, USA). The index calculation results in a value ranging from 0 to 1. The index was further divided into five vulnerability levels, from not vulnerable to extremely vulnerable (Table 1). The vulnerability grouping levels were subjectively defined to establish explicit cut-off criteria for PtP site selection. Only extremely vulnerable sites were considered for PtP deployment (i.e., index >0.8). Moreover, only sites within walking distance of the Ethiopian road network were considered. This prerequisite was set because many individuals lack access to long-distance transportation. Individuals frequently rely on walking as their primary means of reaching the nearest food market. The model assumed a 9.1-km walking distance, which, according to several studies, represents the average walking distance citizens undertake to access food markets in low-to medium-income countries (Asfaw et al., 2012; Destaw and Fenta, 2021; Gecho, 2017; Mengistu et al., 2021; Usman and Callo-Concha, 2021). Walking distance data from these studies is provided in Supplementary Table S4.
2.6 Agricultural dataset and protein estimation
Crop biomass production data in Ethiopia is necessary to calculate the theoretical protein production from the chosen PtP sites. Data on cultivation areas and yields of major crops grown in Ethiopia was obtained from the 2019/2020 agricultural sample survey report of Ethiopia’s Federal Central Statistical Agency (CSA, 2021). An overview of the workflow from data processing to the calculations of protein production is depicted in Figure 3. An expanded view of the crop cover, population density, and VHI data plot overlay is presented in Supplementary Figure S1. The biomass yield for each crop per cultivated land area was calculated and then summed to obtain the total biomass for PtP (Equation 1).
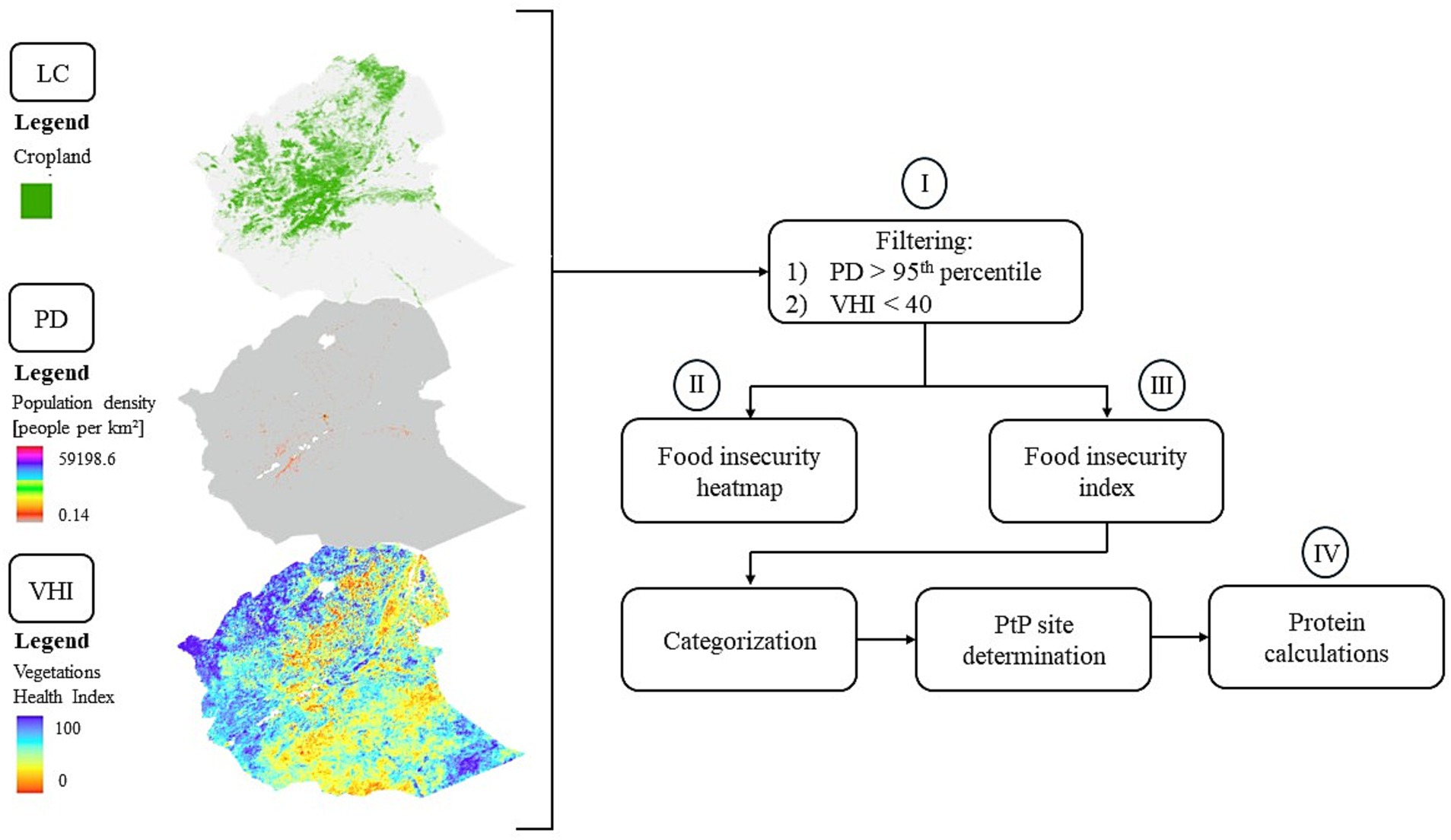
Figure 3. Overview of the methodological approach: (I) data processing and subsequent filtering to obtain relevant data; (II) food insecurity heatmap generation; (III) computation of food insecurity index with subsequent categorization to identify potential Power-to-Protein (PtP) sites; and (IV) protein production calculations at the identified PtP site locations. LC – land cover; PD – population density; VHI – vegetation health index.
Where mB is the amount of biomass [kg], Aagr is the agricultural area [km2], fcr is the fraction of arable land occupied by the crop [km2/km2], Y is the crop yield [kg/km2], R is the residue-to-crop ratio [kg/kg], and fdm is the fraction of dry matter in crop plants [kg/kg]. A detailed description of the equation parameters and assumptions is given in the Supplementary section S1.1.
The calculated crop biomass in areas that are affected by drought represents the feedstock available for PtP production. The areas considered for crop biomass calculation were defined as circular areas with increasing radii around the potential PtP site locations, increasing by 10-kilometer increments from 10 to 90 km. Collection areas start to overlap beyond 90 km. The model assumes complete biomass combustion and recovery of CO2 as a substrate for protein production. The stoichiometry in Equation 2 describes biomass combustion. A stoichiometric approach was also used to estimate the yield of microbial biomass from the 1st-Stage (Equation 3) and 2nd-Stage (Equation 4) bioprocess based on the growth equations of acetate-producing bacteria and heterotrophic microbes (Mishra et al., 2020). The stoichiometric equations are as follows:
Given the stoichiometric amount of microbial biomass (C5H7O2N) in the 2nd-Stage, the theoretical amount of protein in biomass through the PtP process was calculated using the following equation (Equation 5):
Where mSCP is the amount of protein [kg], n equals the moles of microbial biomass in the 2nd-Stage [kmol], Mmic is the molar mass of the microbial biomass [kg/kmol], fP is the protein fraction of the microbial biomass [kg/kg], fN is the product loss within the protein production chain [kg/kg], and fhs is the fraction of protein remaining after heat shock treatment [kg/kg]. A detailed description of the equation parameters can be found in the Supplementary section S1.2. For the calculation, we assumed a daily recommended dietary protein allowance of 56 g per day (Molitor et al., 2019) for individuals with an average weight of 70 kg. In many instances, the protein supply from PtP was sufficient to supply the protein requirement of the local population for an extended period. Finally, crop data was collected and analyzed to compare PtP protein yields to conventional plant-based and animal-based protein yields during non-drought conditions (Supplementary Table S3). Six kilograms of crop protein was assumed necessary to produce 1 kg of animal protein (Ritala et al., 2017).
2.7 Uncertainty analysis
An uncertainty analysis was conducted for important PtP parameters contributing to protein yield. The uncertainty analysis involved defining a lower-and upper-bound value for each parameter based on literature data. The best-case scenario was determined by assuming the optimal value for each parameter within the specified range, while the worst-case scenario assumed the least optimal value. The parameters and associated values used in the uncertainty analysis are provided in Supplementary Table S5.
3 Results and discussion
3.1 Several major cities in Ethiopia are highly vulnerable to drought
The analysis identified 16,660 food-insecure locations in Ethiopia (equivalent total area = 1,499 km2) and their spatial distribution (Figure 4). Most of the highly vulnerable regions were located near major urban areas of central Ethiopia, clustering around the cities of Addis Ababa (3.95 million people), Nazareth (Adama) (0.48 million people), and Hawasa (0.58 million people) (ESS, 2023). Less vulnerable locations tended to cluster in the periphery of these major urban areas and between other large cities, such as Jimma (0.26 million), Dire Dawa (0.36 million), Dessie (0.28 million), and Mekele (0.48 million) (ESS, 2023). Fewer clusters were seen in the non-central, rural regions due to the low population density in these areas. Indeed, population density was the primary factor contributing to food insecurity, according to the model. However, the population’s proximity to (and dependency on) local crop production also played an important role. Due to Ethiopia’s climate and geography, most of the arable land is concentrated in central Ethiopia. Here, the elevated highlands provide favorable conditions for agriculture (Figure 1). In contrast, Ethiopia’s southern and northern regions are mostly arid, rendering them unfavorable for crop production. Thus, the populations residing in central Ethiopia are at considerably greater risk of drought-related food shortages than those in non-central regions.
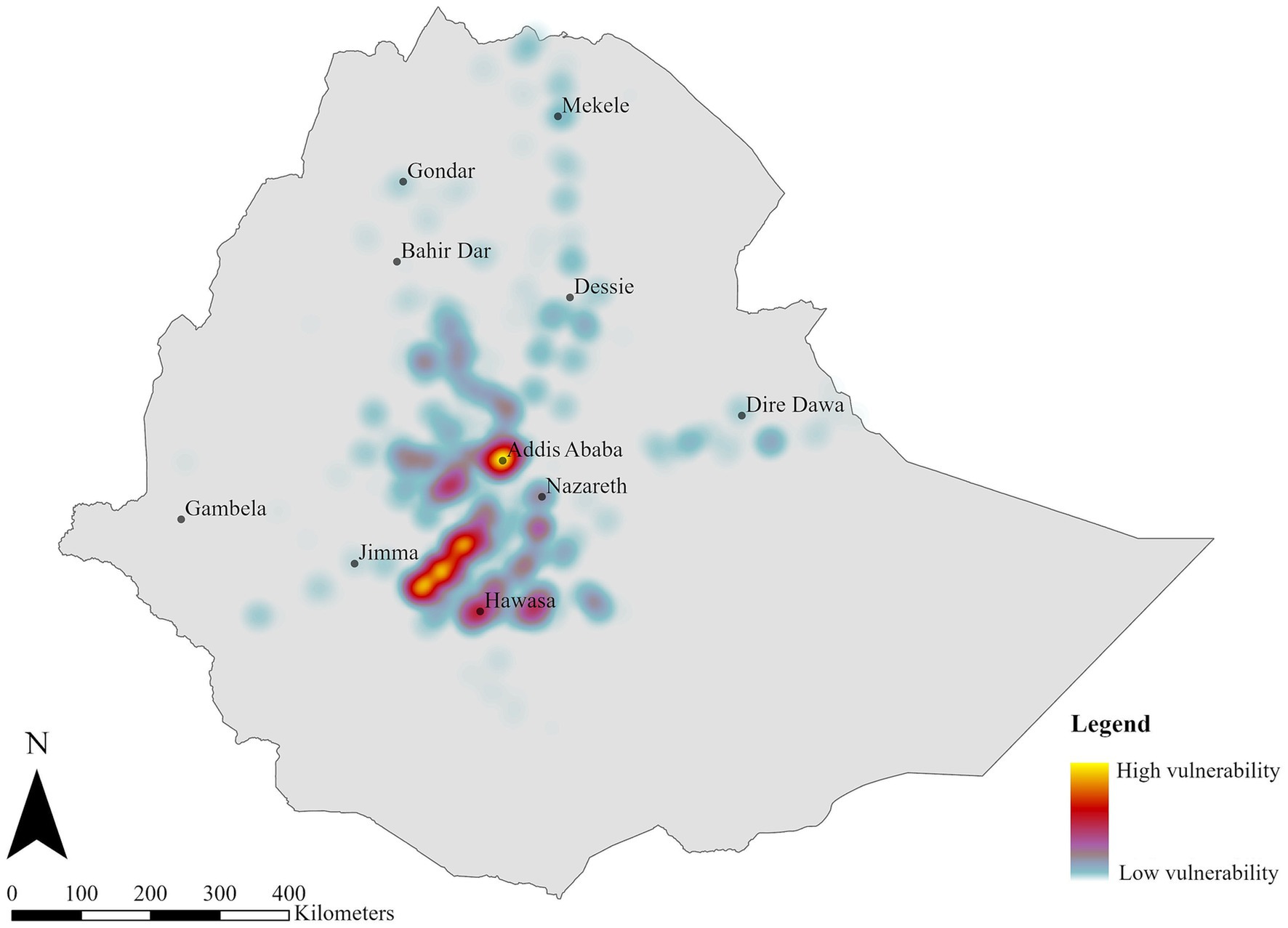
Figure 4. Summary results of GIS-based PtP siting analysis for Ethiopia. Food insecurity heatmap indicating areas with low (blue) to high (yellow) vulnerability.
The model also identified 520 suitable PtP sites for drought-related disaster relief by cross-evaluating the food-insecure areas with Ethiopia’s road network, which is buffered by a 9.1-km walking distance (Figure 5). The majority of qualifying PtP sites occurred within three primary zones surrounding the urban area of Mekele in northern Ethiopia, the urban area of Addis Ababa in central Ethiopia, and the urban area of Hawasa south of Addis Ababa. These potential PtP sites corresponded closely to the food-insecure regions that were identified previously. Three optimal PtP sites were subsequently ascertained by aggregating the individual sites within a given zone and weighing their food insecurity index values (Table 2; Figure 5).
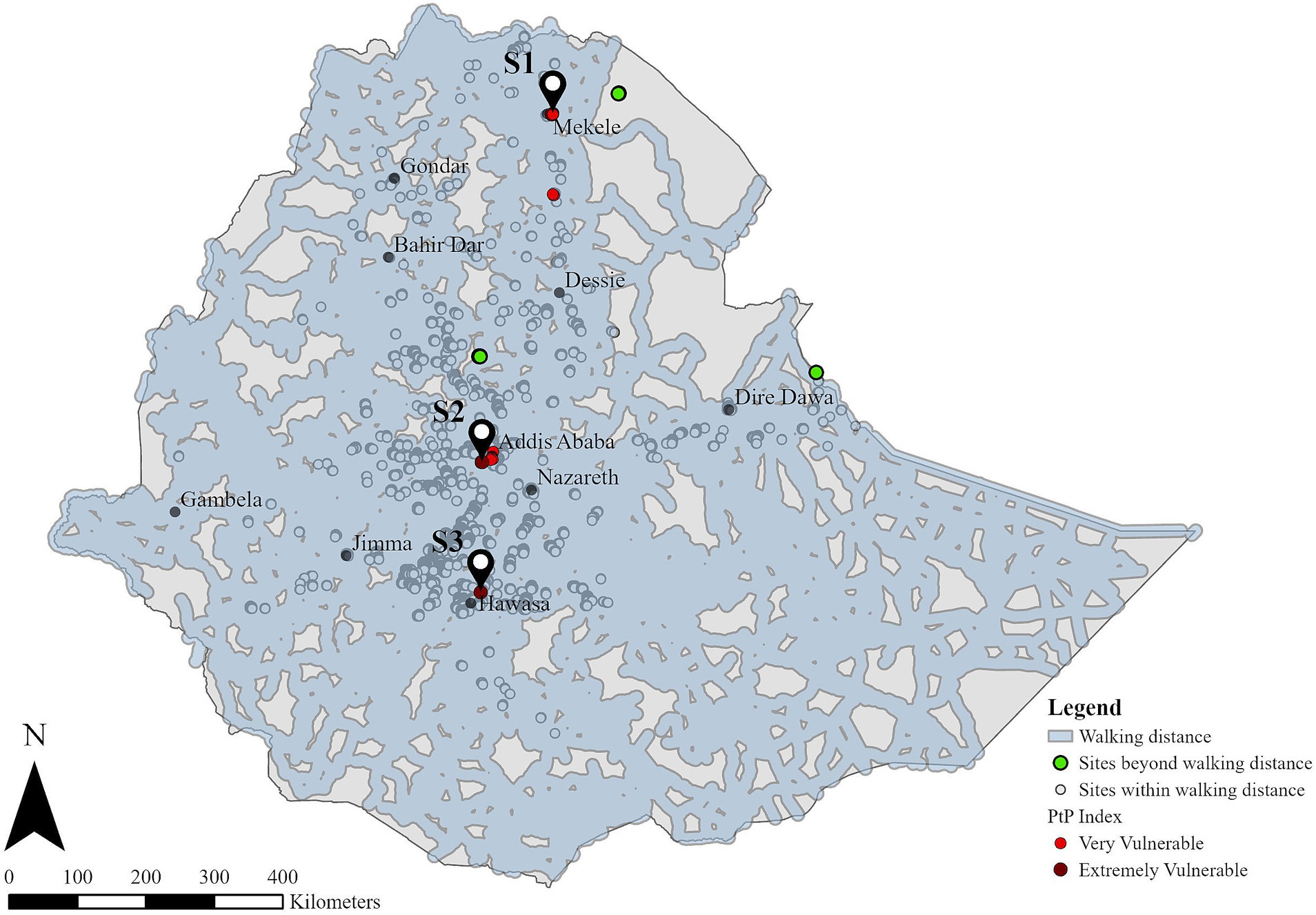
Figure 5. Results of PtP site accessibility evaluation constrained by walking distance. Displayed are potential PtP sites within walking distance (grey circles) and beyond walking distance (green circles). Individual circles represent possible PtP sites in Ethiopia, including those in very vulnerable areas (red circles) and extremely vulnerable areas (Sites S1–S3, dark red circles).
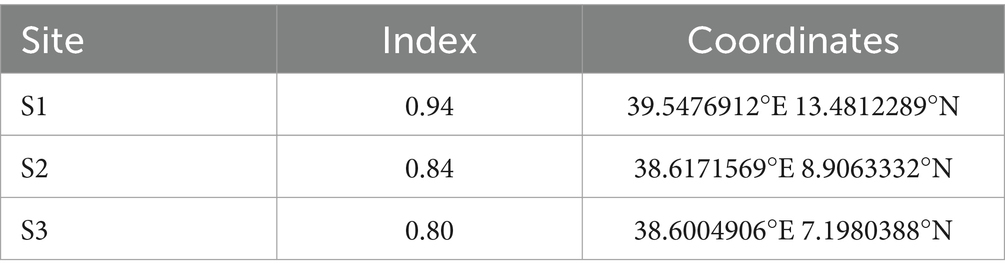
Table 2. PtP site locations close to the urban areas of Mekele (S1), Addis-Ababa (S2), and Hawasa (S3) with their respective index value and geospatial coordinates.
The optimal sites occurred proximate to Mekele (S1), Addis Ababa (S2), and Hawasa (S3). The complete set of PtP sites with their corresponding drought vulnerability index values in the zones proximate to Mekele, Addis Ababa, and Hawasa are shown in Supplementary Figures S2–S4. Lowering the cut-off criteria to the second highest risk category (i.e., very vulnerable) qualified 30 additional PtP sites, most of which still cluster around these urban areas. These results suggest that PtP siting in urban areas is flexible, offering many viable site locations so long as the PtP sites fall within a few kilometers of the metropolitan area. Only two sites south of Mekele were spatially isolated (Figure 5), which may justify the deployment of a fourth PtP plant in that zone. Also, multiple food-insecure areas were identified between Hawasa and Jimma (Figure 4), indicating that this region is somewhat vulnerable to drought-related insecurity. However, according to the model criteria, none of these regions qualified as very or extremely vulnerable (Supplementary Figure S5).
The model was fairly robust and yielded sensible results. Most of the PtP sites occurred in or near urban zones where the population density is high, the road network is interconnected, and agricultural lands for crop production are proximate. Furthermore, the model disqualified only a few PtP sites for exceeding the maximum walking distance constraint. These results were expected and consistent with the population distribution data, which shows that most of the population lives within 9.1 km of a serviceable road. Lastly, very few PtP sites occurred in the peripheral, non-central regions of the country where the population density is low, serviceable roads are more divergent and less interconnected, and crop production is less (Figure 5).
3.2 Centralized PtP plants satisfy protein demands for extended periods after a drought
While the citizens surrounding the three urban sites are highly vulnerable to food shortages, centralized PtP deployment would satisfy their protein demand for several months following a drought, assuming a modest biomass collection radius of 35 km (Figure 6). Expanding the biomass collection radius should increase the total protein output of the PtP plant, and thus extend the duration of the protein supply. However, this trend did not hold in all cases. For example, the protein supply duration for the PtP site near Mekele (S1) increases drastically for a collection radius of up to 40 km because Mekele is the only urbanized area near the PtP site (Figure 6). At the same time, agriculture is prevalent in the areas immediately surrounding the city center, which leads to higher biomass availability per individual. However, the supply period declines considerably beyond 40 km due to the mountain ridge east of Mekele (Figure 1). This topographical feature delineates the area where most agricultural activities cease, resulting in little additional biomass for PtP. Also, the protein supply period in S2 decreases between a radius of 10 and 20 km due to the inclusion of the capital city, Addis Ababa, within the 20 km radius (Figure 6). Here, the inclusion of agricultural land is increasing slower than the population count. After a 30 km radius, the protein supply period increases again because agriculture is more prevalent outside Addis Ababa. In the case of S3, the supply period increases quickly, then exhibits diminishing returns beyond the 20 km radius due to the inclusion of the urban area of Hawasa and the relatively low food crop productivity beyond the urban area (Figure 6).
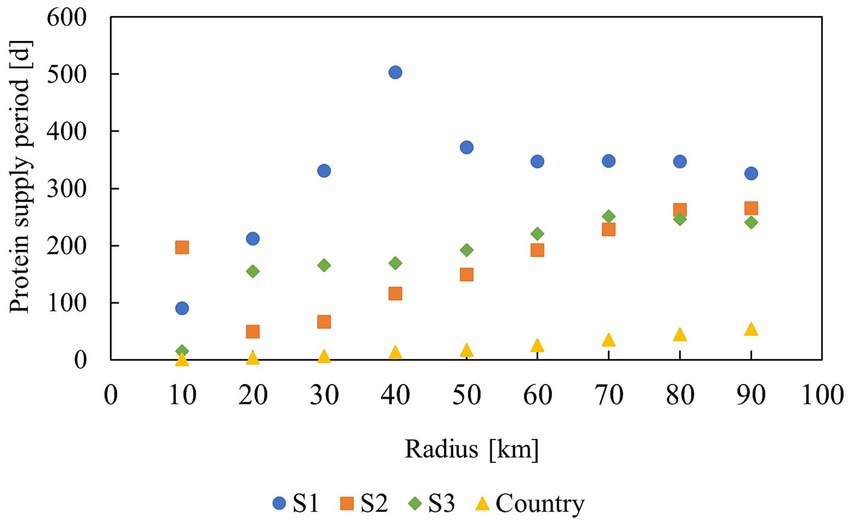
Figure 6. Protein supply periods for the sites close to the urban areas of Mekele (S1), Addis-Ababa (S2), and Hawasa (S3) and the entire population of Ethiopia based on increasing biomass collection radii.
The long protein-supply periods predicted by the model suggest that crop biomass availability for PtP in Ethiopia is generally high, including in the food-insecure zones, which is consistent with an agrarian-based society. Moreover, these results underscore the high protein-conversion efficiency of PtP technology. However, there are supply logistics to consider. First, PtP cannot be scaled to process the biomass quantities collected in these larger collection areas at a single facility. Multiple PtP facilities would need to be deployed in a centralized location to circumvent this issue. In addition, transporting biomass from large geographic areas into a single urban area would be challenging and could overwhelm public roads and local civil infrastructure. Indeed, most of Ethiopia’s economic activity relies on vehicular transport. Also, while the train network in Ethiopia is undergoing an expansion, it is currently limited and not suited for extensive freight transport. Therefore, decentralized PtP deployment may be more feasible than centralized PtP deployment because the supply routes would be distributed over a wider area and manageable by vehicular transport.
3.3 Mobile PtP units can complement food aid programs to address local food insecurity
Under the given assumptions, a single mobile shipping container with the dimensions 12 × 2.4 × 2.7 m (77.7 m3) could conservatively accommodate a 1st-Stage bioreactor with a capacity of 1983 L and a 2nd-Stage bioreactor with a capacity of 3,000 L. In continuous operation, a PtP unit of this scale would yield approximately 38 kg of protein per day, enough to cover the minimum protein requirement of ~680 people. Therefore, the rate of protein production is relatively high. However, considering the high population density in urban zones, multiple PtP units would need to be distributed throughout the metropolitan area. The number and distribution density of mobile PtP units necessary to meet the protein demand of the three largest demand zones (S1–S3) are given in Table 3.

Table 3. The demand and distribution density of mobile PtP units at sites S1–S3 assuming conservative and optimistic design parameters.
Several hundred thousand people live within the urban zones around sites S1–S3. This would require deploying 700–5,800 mobile PtP units throughout the metropolitan areas at a distribution density of 5.4–11 units per km2. Planning the deployment, the operation, and the supply logistics for this number of PtP units in an urban setting would be exceedingly complex and unrealistic. However, our initial PtP unit design conservatively assumed a single PtP system per shipping container that occupies only 6.4% of the container space (total working volume ≈ 5 m3). Under optimistic assumptions, however, the container dimensions could theoretically accommodate a total working volume of 50 m3. With this configuration, and assuming the auxiliary equipment would fit into the remaining 27 m3 of space, the number of required PtP units could be reduced by a factor of 10 (i.e., ~70–580 containers) to satisfy the protein demand of these urban areas.
Deploying mobile PtP units for disaster relief may be a viable strategy considering these more optimistic values. The deployment logistics to get the PtP units to the at-risk areas is also feasible. For instance, ocean barges could deliver thousands of PtP shipping containers to a central seaport. Then, the containers could be transferred from the seaport to dry ports by train or vehicular transport. There are dry shipping ports in Mekele and additional ports 75 km from Addis Ababa (Dilipan, 2022). Railways have already connected the seaports in Djibouti to Mekele and Addis Ababa, and more railways are under construction (The Ethiopian Messenger, 2017). From there, short-distance transportation using lorries could be used to transport the PtP units to the designated sites. This transport scheme would allow for relatively rapid disaster relief. Another advantage of a decentralized strategy is that it provides greater accessibility for citizens – people would not need to walk long distances to reach a PtP unit. However, feedstock supply logistics would be more complex than the centralized deployment approach. Also, operating this large number of PtP units would require many trained technicians, resulting in high logistical effort and labor expenses.
Considering the scenario with multiple reactors in a single container, one PtP unit would require ~216 kg of anhydrous ammonia (l) per day as a nitrogen source. At an equivalent volume of 0.3 m3, the anhydrous ammonia (liq.) would occupy a relatively small amount of space within the PtP container and be adequate for short-term disaster relief (e.g., 1–2 weeks). On the other hand, sustained operation over long periods would require periodic resupplying of nitrogen. This relatively high N demand is expected because the protein product is a nitrogen-rich macronutrient with 16% N content (w/w). However, PtP is a contained system, so a large proportion of the supplied N is recovered in the final product, with minimal losses along the processing line, leading to an N-utilization efficiency of >34%. About a third of the N is lost at the RNA reduction stage, while moderate amounts are lost in the unrecovered liquid effluents. Nitrogen is highly conserved in the 1st-Stage bioreactor because the biocatalyst (C. ljungdahlii) is retained. Here, N supplementation is only required to cover cell replacement and the N deficit in the recycled water streams. Overall, the N-utilization efficiency of the PtP system is considerably higher than plant-and animal-based protein production (26 and 7%, respectively) (Pikaar et al., 2017) and would emit minimal reactive nitrogen into the environment.
The estimated water demand of a mobile PtP unit would be ~6,400 L per day (~26 L per kg protein), two-thirds of which is consumed by the electrolyzer. The remaining one-third of the water is required for bioreactor operation (e.g., media inputs). However, ~85% of this water is conserved within the PtP system due to internal water recovery and recycling with only minor losses along the processing chain. For example, the separated water from biomass centrifugation/drying can be collected and reused as media diluents in the 1st-and 2nd-Stage bioreactors. About 14% of the water losses occur as unrecoverable moisture in the downstream processing effluents. Finally, a small amount of moisture (~5%, w/w) is present in the final dry powder, constituting a minor loss. Therefore, PtP should not excessively burden the local water supply during drought.
The primary advantage of a centralized, industrial-scale PtP facility is its production capacity. An industrial-scale facility with a working volume of 1,000 m3 could produce an estimated 7.6 tons of protein per day. Four facilities of this scale would be sufficient to meet the protein demand of all individuals in the urban area of Mekele at site S1. However, industrial-scale facilities involve extended start-up periods, making them less feasible for immediate disaster relief. For example, the typical start-up period for an industrial-scale corn-to-ethanol plant is 30–60 days (Sandor, 2019). While bioprocess kinetics depend on many factors, including bioreactor design, operating conditions, and inoculum source (Angenent et al., 2022; Posmanik et al., 2020), pre-inoculated and preconditioned mobile PtP units with much shorter retention times are technically conceivable, which would provide more rapid disaster relief. Another considerable advantage of mobile PtP technology is the ability to generate protein on-site in areas suffering acute food insecurity, reducing dependence on food delivery supply chains. Thus, mobile PtP systems could also be used to complement conventional food aid efforts.
3.4 Protein yields from PtP outperform conventional agriculture
Conventional agriculture exerts pressure on the planet, using finite resources such as land, water, fossil energy, and nutrients. These agricultural practices generate considerable GHG emissions, accounting for about a quarter of all anthropogenic emissions (Poore and Nemecek, 2018). Detrimental consequences for the environment are becoming increasingly visible, and this damage is impacting all socio-economic sectors. Agriculture is particularly susceptible to climate variability and extreme weather, especially drought events. Consequently, agricultural yields of major crops are expected to decline (Zhao et al., 2017). Decreased agricultural yields would, in turn, require additional agricultural expansion and resource inputs to keep up with the increasing food demand of a growing world population. Agricultural expansion would then exacerbate emissions and further drive climate change. Thus, the current food production system would be trapped in a positive feedback loop of keeping up with food demand and exacerbating climate change (Harvey and Pilgrim, 2011). PtP technology offers an alternative.
For every ton of vegetal biomass, PtP provides approximately 390% more protein than the direct consumption of plants (Figure 7, primary axis). This disparity in protein yield underscores the remarkable efficiency of microbes in converting substrates into protein. An additional comparison with animal-based protein further highlights this advantage. Here, PtP would yield approximately 22 times more protein than animal protein, considering the same amount of biomass. In the worst-case scenario, PtP is 44% less efficient than plant-based protein but still 225% more efficient than animal-based protein. Moreover, the amount of available biomass for protein production relative to the local population varies considerably between the study sites of Mekele (S1), Addis Ababa (S2), and Hawasa (S3). Addis Ababa (S2) has relatively less available biomass for protein production to supply its population compared to Mekele (S1) and Hawasa (S3) (Figure 7, secondary axis). Thus, Addis Ababa would benefit more from PtP than the other sites. Here, under the best-case scenario, each ton of crop biomass allocated to PtP would provide protein to 0.016% of Addis Ababa’s population, which is 3.2 times more than plant-based protein and 16 times more than animal-based protein. In Mekele (S1) and Hawasa (S3), the per capita biomass availability is a magnitude greater than in Addis Ababa (S2). Here, each ton of crop biomass allocated to PtP would provide protein to 0.135% of Mekele’s population and 0.113% of Hawasa’s population. Finally, the per capita biomass availability can be several magnitudes higher in rural populations, suggesting mobile PtP systems would be particularly advantageous for these communities during food shortages.
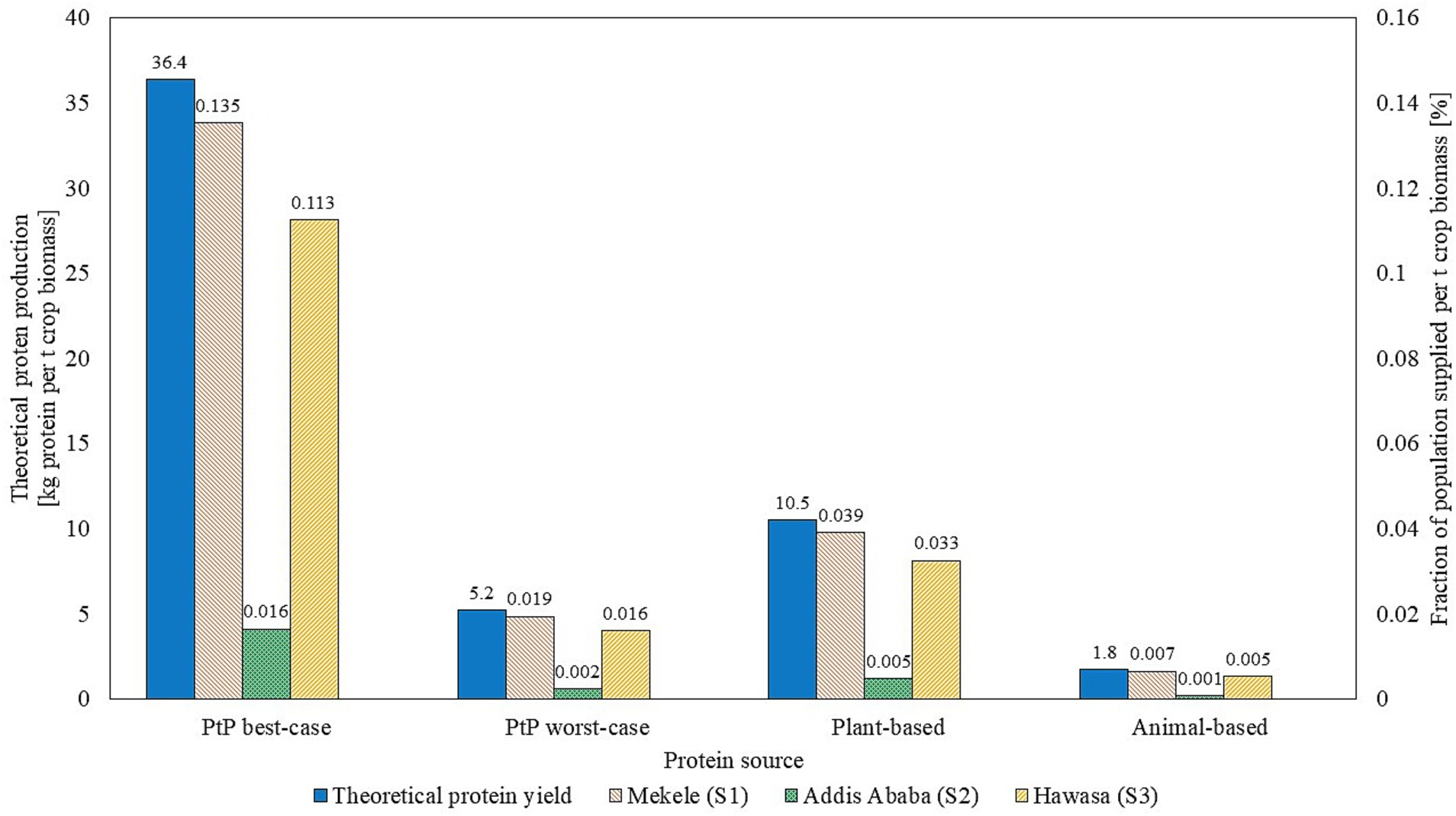
Figure 7. PtP protein yields in a best-case and a worst-case scenario compared to the protein yield of plants and animals from the same quantity of crop biomass on the primary axis. The secondary axis shows the fraction of daily protein demand of the urban areas’ population near the potential sites supplied per t of crop biomass in the best-case, worst-case, and plant-and animal-based scenarios.
Alongside protein quantity, it is also important to consider the digestibility and nutritional value of the protein. The amino acid profile of yeast and other SCPs is generally richer in essential amino acids than soy meal and other plant protein sources (Jach et al., 2022 Øverland et al., 2010). Moreover, plant-based diets often lack sufficient levels of vitamin B3 (Shepon et al., 2018). In contrast, B vitamins occur at relatively high levels in yeasts (Jach et al., 2022). For example, Schmitz et al. (2024) recently applied a two-stage bioprocess similar to the PtP process described here, but with the goal of producing vitamins (power-to-vitamins), in addition to protein. The researchers were able to grow yeast biomass containing vitamin B9 (folate) at high levels (6.7 mg per 100 g) (Schmitz et al., 2024). The recommended dietary intake of vitamin B9 is 400 μg·d−1 (Herbert, 1987). So, besides providing higher protein yields from equivalent substrate quantities, PtP should offer nutritional benefits on par with or superior to plant-and animal-based protein sources.
These results highlight the potential of PtP technology. PtP can salvage protein and essential nutrients from crops that are otherwise lost during a drought. The results also advocate leveraging healthy crops for PtP production. However, dedicating arable land for PtP production is not necessarily sustainable because it may not satisfy the population’s social needs. Traditional culinary uses of local vegetables and meat underpin the cultural identity and values of the Ethiopian people and the economy. Microbial-based foods may be an inadequate or unaccepted substitute. Therefore, PtP may better serve societal needs by valorizing readily available waste materials (Matassa et al., 2020), such as dead crops, crop residues, or inedible vegetal biomass grown on marginal land, to complement the local food system, rather than replace it. Still, the issue of land and material ownership rights would need to be addressed with some form of compensation. Despite the loss of biomass due to drought or as waste, these crops or residues are taken from arable land owned by farmers that required effort and expenditure to cultivate. Therefore, the farmers should be provided monetary compensation (e.g., flat rate payments, tax incentives) to supply their crops to PtP production.
4 Outlook and conclusions
Analysis of Ethiopia’s drought vulnerability revealed many food-insecure areas nationwide. Further indexing identified three highly at-risk sites, signifying the optimal locations for PtP deployment in a drought scenario. According to the model, the protein yields generated by these PtP plants would be sufficient to sustain the protein requirements of the local population for extended periods, suggesting PtP could be a practical disaster relief strategy. Moreover, PtP could be deployed using a centralized approach, with dedicated large-scale infrastructure, or a decentralized approach, with mobile, small-scale PtP units. While each strategy offered unique advantages, decentralized PtP units emerged as the preferable choice due to their rapid deployment capability and short start-up period.
PtP’s independence from climate and its resource-use efficiency make it a promising technology for ordinary use beyond disaster relief. In arid areas where water is already scarce, PtP could generate protein more effectively than crops that require large amounts of water for irrigation. At the same time, PtP would require less land and use nutrients more efficiently. Also, industrial CO2 sources and dry organic waste could additionally serve as PtP feedstock, increasing the protein supply considerably, especially near metropolitan areas. Therefore, having a dedicated PtP infrastructure would be advantageous because it would contribute to a steady food supply and make the population more resilient in all scenarios.
Most of the potential PtP locations predicted by the model cluster in areas with high populations and population densities. In a drought scenario, people in more urban areas may have greater access to nutritional supplies than people in more rural areas. Individuals in urban areas also may have more financial resources to access food if there are shortages due to drought. Given that the mobile units provide sufficient protein to small groups of individuals, future studies could investigate strategies for deploying mobile PtP units to aid rural communities specifically.
In the end, successful PtP implementation can depend on subjective factors such as consumer acceptance. PtP is a novel and unfamiliar food source that has not become normalized in the standard diet anywhere in the world, let alone in Ethiopia. If PtP fails to gain acceptance among the local population, deploying PtP in at-risk areas becomes impractical. However, it is reasonable to assume people would be amenable to PtP during food shortages and crisis situations. Also, the use of yeast should help foster consumer acceptance. In conclusion, and considering the predicted increase in drought severity due to climate change, PtP technology holds promise as a potential measure to address localized food insecurities, especially when implemented alongside other food aid programs.
Data availability statement
Publicly available datasets were analyzed in this study. This data can be found at: Landcover: - Link to the ESA web viewer of the landcover products http://maps.elie.ucl.ac.be/CCI/viewer/index.php - Link where the data can be downloaded http://maps.elie.ucl.ac.be/CCI/viewer/download.php - Link on how to cite the data https://www.esa-landcover-cci.org/?q=node/199 Population density: https://hub.worldpop.org/geodata/summary?id=46094 Population Count: https://hub.worldpop.org/geodata/summary?id=26183 Vegetation Health Index https://www.star.nesdis.noaa.gov/pub/corp/scsb/wguo/data/Blended_VH_4km/geo_TIFF/ Agricultural data meher season 2020/2021 https://www.statsethiopia.gov.et/our-survey-reports/.
Author contributions
MB: Conceptualization, Formal analysis, Investigation, Visualization, Writing – original draft. HN: Validation, Writing – review & editing. LA: Conceptualization, Supervision, Writing – review & editing. CZ: Conceptualization, Resources, Supervision, Writing – review & editing. JU: Conceptualization, Formal analysis, Funding acquisition, Project administration, Supervision, Writing – review & editing.
Funding
The author(s) declare that financial support was received for the research, authorship, and/or publication of this article. J.U. declares financial support was provided, in part, by the Agricultural Experiment Station at the University of Georgia. L.A. was supported, in part, by the Alexander von Humboldt Foundation in the framework of the Alexander von Humboldt Professorship, and the Novo Nordisk Foundation CO2 Research Center with grant number NNF21SA0072700, and the Novo Nordisk Foundation CO2 Research Center (CORC) with grant number NNF21SA0072700, and is published under the number CORC_24_36.
Conflict of interest
The authors declare that the research was conducted in the absence of any commercial or financial relationships that could be construed as a potential conflict of interest.
Publisher’s note
All claims expressed in this article are solely those of the authors and do not necessarily represent those of their affiliated organizations, or those of the publisher, the editors and the reviewers. Any product that may be evaluated in this article, or claim that may be made by its manufacturer, is not guaranteed or endorsed by the publisher.
Supplementary material
The Supplementary material for this article can be found online at: https://www.frontiersin.org/articles/10.3389/fsufs.2024.1429171/full#supplementary-material
References
Anderson, W., Cook, B. I., Slinski, K., Schwarzwald, K., McNally, A., and Funk, C. (2023). Multiyear La Niña events and multiseason drought in the horn of Africa. J. Hydrometeorol. 24, 119–131. doi: 10.1175/jhm-d-22-0043.1
Angenent, L. T., Usack, J. G., Sun, T., Fink, C., Molitor, B., Labatut, R., et al. (2022). “Upgrading anaerobic digestion within the energy economy – the methane platform” in Resource recovery from water: Principles and application. ed. I. Pikaar (London: IWA Publishing), 141–158.
Asfaw, S., Shiferaw, B., Simtowe, F., and Lipper, L. (2012). Impact of modern agricultural technologies on smallholder welfare: evidence from Tanzania and Ethiopia. Food Policy 37, 283–295. doi: 10.1016/j.foodpol.2012.02.013
Asfaw, A., Simane, B., Hassen, A., and Bantider, A. (2018). Variability and time series trend analysis of rainfall and temperature in northcentral Ethiopia: a case study in Woleka sub-basin. Weather Climate Extremes 19, 29–41. doi: 10.1016/j.wace.2017.12.002
Ault, T. R. (2020). On the essentials of drought in a changing climate. Science 368, 256–260. doi: 10.1126/science.aaz5492
Beltrando, G., and Camberlin, P. (1993). Interannual variability of rainfall in the eastern horn of Africa and indicators of atmospheric circulation. Int. J. Climatol. 13, 533–546. doi: 10.1002/joc.3370130505
Bratosin, B. C., Darjan, S., and Vodnar, D. C. (2021). Single cell protein: a potential substitute in human and animal nutrition. Sustain. For. 13:9284. doi: 10.3390/su13169284
Brinkhoff, T. City population. Available at: https://www.citypopulation.de/en/ethiopia/admin/ (Accessed November 17, 2023).
Chou, C., Chiang, J. C. H., Lan, C. W., Chung, C. H., Liao, Y. C., and Lee, C. J. (2013). Increase in the range between wet and dry season precipitation. Nat. Geosci. 6, 263–267. doi: 10.1038/ngeo1744
Chou, C., Neelin, J. D., Chen, C. A., and Tu, J. Y. (2009). Evaluating the “rich-get-richer” mechanism in tropical precipitation change under global warming. J. Clim. 22, 1982–2005. doi: 10.1175/2008JCLI2471.1
CSA . (2021). Agricultural sample survey 2020/2021 (2013 E.C): report on area and production of major crops (private peasant holdings, meher season). Available at: https://www.statsethiopia.gov.et/our-survey-reports/ (Accessed November 09, 2023).
Destaw, F., and Fenta, M. M. (2021). Climate change adaptation strategies and their predictors amongst rural farmers in Ambassel District, Northern Ethiopia. Jàmbá 13:974. doi: 10.4102/jamba.v13i1.974
Dilipan, T. (2022). 8 major ports in Ethiopia. Available at: https://www.marineinsight.com/know-more/8-major-ports-in-ethiopia/#Modjo_Dry_port (Accessed November 9, 2023).
Dong, Q., Wang, W., Kunkel, K. E., Shao, Q., Xing, W., and Wei, J. (2020). Heterogeneous response of global precipitation concentration to global warming. Int. J. Climatol. 41, E2347–E2359. doi: 10.1002/joc.6851
Dorosh, P., and Rashid, S. (2013). Food and agriculture in Ethiopia: Progress and policy challenges. Philadelphia, Pennsylvania: University of Pennsylvania Press.
Edwards, B., Gray, M., and Hunter, B. (2019). The social and economic impacts of drought. Aust. J. Soc. Issues 54, 22–31. doi: 10.1002/ajs4.52
Elzopy, K. A., Chaturvedi, A. K., Chandran, K. M., Gopinath, G., and Surendran, U. (2021). Trend analysis of long-term rainfall and temperature data for Ethiopia. S. Afr. Geogr. J. 103, 381–394. doi: 10.1080/03736245.2020.1835699
ESA . (2017). Land cover CCI product user guide version 2. Available at: https://maps.elie.ucl.ac.be/CCI/viewer/download/ESACCI-LC-Ph2-PUGv2_2.0.pdf (Accessed November 9, 2023).
ESS . (2023). Population size by sex zone and Wereda July 2023. https://www.statsethiopia.gov.et/population-projection/ (Accessed May 7, 2024).
Fazzini, M., Bisci, C., and Billi, P. (2015). “The climate of Ethiopia” in Landscapes and Landforms of Ethiopia. ed. P. Billi (Dordrecht: Springer), 65–87.
Ficklin, D. L., Null, S. E., Abatzoglou, J. T., Novick, K. A., and Myers, D. T. (2022). Hydrological intensification will increase the complexity of water resource management. Earths Future 10:e2021EF002487. doi: 10.1029/2021EF002487
Gecho, Y. (2017). Rural farm households’ income diversification: the case of Wolaita zone, Southern Ethiopia. SS 6:45. doi: 10.11648/j.ss.20170602.12
Harvey, M., and Pilgrim, S. (2011). The new competition for land: food, energy, and climate change. Food Policy 36, S40–S51. doi: 10.1016/j.foodpol.2010.11.009
Herbert, V. (1987). Folate, fact sheet for health professionals. Available at: https://ods.od.nih.gov/factsheets/Folate-HealthProfessional/ (Accessed October 1, 2023).
Hoque, M. A.-A., Pradhan, B., Ahmed, N., and Sohel, M. S. I. (2021). Agricultural drought risk assessment of northern New South Wales, Australia using geospatial techniques. Sci. Total Environ. 756:143600. doi: 10.1016/j.scitotenv.2020.143600
Hu, X., Kerckhof, F.-M., Ghesquière, J., Bernaerts, K., Boeckx, P., Clauwaert, P., et al. (2020). Microbial protein out of thin air: fixation of nitrogen gas by an autotrophic hydrogen-oxidizing bacterial enrichment. Environ. Sci. Technol. 54, 3609–3617. doi: 10.1021/acs.est.9b06755
Humpenöder, F., Bodirsky, B. L., Weindl, I., Lotze-Campen, H., Linder, T., and Popp, A. (2022). Projected environmental benefits of replacing beef with microbial protein. Nature 605, 90–96. doi: 10.1038/s41586-022-04629-w
Jach, M. E., Serefko, A., Ziaja, M., and Kieliszek, M. (2022). Yeast protein as an easily accessible food source. Metabolites 12:63. doi: 10.3390/metabo12010063
Jaramillo, S., Graterol, E., and Pulver, E. (2020). Sustainable transformation of Rainfed to irrigated agriculture through water harvesting and smart crop management practices. Front. Sustain. Food Syst. 4:437086. doi: 10.3389/fsufs.2020.437086
Matassa, S., Papirio, S., Pikaar, I., Hülsen, T., Leijenhorst, E., Esposito, G., et al. (2020). Upcycling of biowaste carbon and nutrients in line with consumer confidence: the “full gas” route to single cell protein. Green Chem. 22, 4912–4929. doi: 10.1039/D0GC01382J
Mengistu, D. D., Degaga, D. T., and Tsehay, A. S. (2021). Analyzing the contribution of crop diversification in improving household food security among wheat dominated rural households in Sinana district, bale zone, Ethiopia. Agric. Food Secur. 10, 1–15. doi: 10.1186/s40066-020-00280-8
Meza, I., Siebert, S., Döll, P., Kusche, J., Herbert, C., Eyshi Rezaei, E., et al. (2020). Global-scale drought risk assessment for agricultural systems. Nat. Hazards Earth Syst. Sci. 20, 695–712. doi: 10.5194/nhess-20-695-2020
Mishra, A., Ntihuga, J. N., Molitor, B., and Angenent, L. T. (2020). Power-to-protein: carbon fixation with renewable electric power to feed the world. Joule 4, 1142–1147. doi: 10.1016/j.joule.2020.04.008
Moges, D. M., and Bhat, H. G. (2021). Climate change and its implications for rainfed agriculture in Ethiopia. J. Water Climate Change 12, 1229–1244. doi: 10.2166/wcc.2020.058
Mohamed, A. A. (2017). Food security situation in Ethiopia: a review study. Int. J. Health Econ. Policy 2, 86–96. doi: 10.11648/j.hep.20170203.11
Molitor, B., Mishra, A., and Angenent, L. T. (2019). Power-to-protein: converting renewable electric power and carbon dioxide into single cell protein with a two-stage bioprocess. Energy Environ. Sci. 12, 3515–3521. doi: 10.1039/C9EE02381J
Naumann, G., Cammalleri, C., Mentaschi, L., and Feyen, L. (2021). Increased economic drought impacts in Europe with anthropogenic warming. Nat. Clim. Chang. 11, 485–491. doi: 10.1038/s41558-021-01044-3
NOAA . (2022). STAR - global vegetation health products: introduction. Available at: https://www.star.nesdis.noaa.gov/smcd/emb/vci/VH/index.php (Accessed November 9, 2023).
Noureldeen, N., Mao, K., Mohmmed, A., Yuan, Z., and Yang, Y. (2020). Spatiotemporal drought assessment over Sahelian countries from 1985 to 2015. J. Meteorol. Res. 34, 760–774. doi: 10.1007/s13351-020-9178-7
Orimoloye, I. R., Belle, J. A., Orimoloye, Y. M., Olusola, A. O., and Ololade, O. O. (2022). Drought: a common environmental disaster. Atmos. 13:111. doi: 10.3390/atmos13010111
Øverland, M., Tauson, A.-H., Shearer, K., and Skrede, A. (2010). Evaluation of methane-utilising bacteria products as feed ingredients for monogastric animals. Arch. Anim. Nutr. 64, 171–189. doi: 10.1080/17450391003691534
Pikaar, I., Matassa, S., Rabaey, K., Bodirsky, B. L., Popp, A., Herrero, M., et al. (2017). Microbes and the next nitrogen revolution. Environ. Sci. Technol. 51, 7297–7303. doi: 10.1021/acs.est.7b00916
Poore, J., and Nemecek, T. (2018). Reducing food's environmental impacts through producers and consumers. Science 360, 987–992. doi: 10.1126/science.aaq0216
Posmanik, R., Kim, A. H., Labatut, R. A., Usack, J. G., and Angenent, L. T. (2020). Granular sludge is a preferable inoculum for the biochemical methane potential assay for two complex substrates. Bioresour. Technol. 309:123359. doi: 10.1016/j.biortech.2020.123359
Ritala, A., Häkkinen, S. T., Toivari, M., and Wiebe, M. G. (2017). Single cell protein-state-of-the-art, industrial landscape and patents 2001-2016. Frontiers in microbiology 8:2009. doi: 10.3389/fmicb.2017.02009
Salazar-López, N. J., Barco-Mendoza, G. A., Zuñiga-Martínez, B. S., Domínguez-Avila, J. A., Robles-Sánchez, R. M., Ochoa, M. A. V., et al. (2022). Single-cell protein production as a strategy to reincorporate food waste and agro by-products back into the processing chain. Bioengineering 9:623. doi: 10.3390/bioengineering9110623
Sandor, D. (2019). Corn ethanol industry process data. Available at: https://www.nrel.gov/docs/fy09osti/45152.pdf (Accessed November 9, 2023).
Schmitz, L. M., Kreitli, N., Obermaier, L., Weber, N., Rychlik, M., and Angenent, L. T. (2024). Power-to-vitamins: producing folate (vitamin B 9) from renewable electric power and CO 2 with a microbial protein system. Trends in Biotechnology. doi: 10.1101/2024.02.22.581687
Seager, R., Naik, N., and Vecchi, G. A. (2010). Thermodynamic and dynamic mechanisms for large-scale changes in the hydrological cycle in response to global warming. J. Clim. 23, 4651–4668. doi: 10.1175/2010JCLI3655.1
Shepon, A., Eshel, G., Noor, E., and Milo, R. (2018). The opportunity cost of animal based diets exceeds all food losses. Proc. Natl. Acad. Sci. USA 115, 3804–3809. doi: 10.1073/pnas.1713820115
Sillman, J., Uusitalo, V., Ruuskanen, V., Ojala, L., Kahiluoto, H., Soukka, R., et al. (2020). A life cycle environmental sustainability analysis of microbial protein production via power-to-food approaches. Int. J. Life Cycle Assess. 25, 2190–2203. doi: 10.1007/s11367-020-01771-3
Sohnesen, T. P. (2020). Two sides to same drought: measurement and impact of Ethiopia’s 2015 historical drought. EconDisCliCha 4, 83–101. doi: 10.1007/s41885-019-00048-w
Teshome, A., and Zhang, J. (2019). Increase of extreme drought over Ethiopia under climate warming. Adv. Meteorol. 2019, 1–18. doi: 10.1155/2019/5235429
The Ethiopian Messenger . (2017). Railway development in Ethiopia. Available at: https://ethiopianembassy.be/railway-development-in-ethiopia/ (Accessed December 7, 2023).
Tom, B., Jeremy, F. A., Shohei, N., Mekonnen, B. B., Pierella, P., Nataliya, M., et al. (2020). Ethiopia Poverty Assessment - Harnessing Continued Growth for Accelerated Poverty Reduction (English). Washington, D.C.: World Bank Group. Available at: http://documents.worldbank.org/curated/en/992661585805283077/Ethiopia-Poverty-Assessment-Harnessing-Continued-Growth-for-Accelerated-Poverty-Reduction
Trnka, M., Hlavinka, P., Možný, M., Semerádová, D., Štěpánek, P., Balek, J., et al. (2020). Czech drought monitor system for monitoring and forecasting of agricultural drought and drought impacts. Nat. Resour. Sch. 40, 5941–5958. doi: 10.1002/joc.6557
UN . (2024). Demographic indicators by region, subregion and country, annually for 1950-2100: estimates, 1950-2023. Available at: https://population.un.org/wpp/Download/Standard/MostUsed/ (Accessed September 6, 2024).
USAID . Agriculture and food security in Ethiopia. Available at: https://www.usaid.gov/ethiopia/agriculture-and-food-security (Accessed September 8, 2024).
Usman, M. A., and Callo-Concha, D. (2021). Does market access improve dietary diversity and food security? Evidence from southwestern Ethiopian smallholder coffee producers. Agric. Econ. 9, 1–21. doi: 10.1186/s40100-021-00190-8
Wang, X. (2022). Managing land carrying capacity: key to achieving sustainable production Systems for Food Security. Land 11:484. doi: 10.3390/land11040484
Wilhite, D. A., and Glantz, M. H. (1985). Understanding: the drought phenomenon: the role of definitions. Water Int. 10, 111–120. doi: 10.1080/02508068508686328
WorldPop (2020). Individual countries 1km UN adjusted population density (2000–2020) : University of Southampton. Available at: https://hub.worldpop.org/geodata/summary?id=46094
Yang, R., Chen, Z., Hu, P., Zhang, S., and Luo, G. (2022). Two-stage fermentation enhanced single-cell protein production by Yarrowia lipolytica from food waste. Bioresour. Technol. 361:127677. doi: 10.1016/j.biortech.2022.127677
Keywords: food crisis, single-cell protein, extreme weather, sustainable food production, disaster relief, microbial protein
Citation: Buchner M, Nguyen HH, Angenent LT, Zarfl C and Usack JG (2024) Deployment of power-to-protein technology in Ethiopia to provide drought-related emergency relief and mitigate food insecurity. Front. Sustain. Food Syst. 8:1429171. doi: 10.3389/fsufs.2024.1429171
Edited by:
Sara M. Oliveira, International Iberian Nanotechnology Laboratory (INL), PortugalReviewed by:
Ahmed Hamad, Benha University, EgyptBudiastuti Kurniasih, Gadjah Mada University, Indonesia
Copyright © 2024 Buchner, Nguyen, Angenent, Zarfl and Usack. This is an open-access article distributed under the terms of the Creative Commons Attribution License (CC BY). The use, distribution or reproduction in other forums is permitted, provided the original author(s) and the copyright owner(s) are credited and that the original publication in this journal is cited, in accordance with accepted academic practice. No use, distribution or reproduction is permitted which does not comply with these terms.
*Correspondence: J. G. Usack, am9zZXBoLnVzYWNrQHVnYS5lZHU=