- 1Life Cycle Management, Sustainable Society, IVL Swedish Environmental Research Institute, Stockholm, Sweden
- 2Department of Sustainable Development, Environmental Science and Engineering, KTH Royal Institute of Technology, Stockholm, Sweden
- 3Department of Agricultural and Food Sciences, Alma Mater Studiorum, University of Bologna, Bologna, Italy
The number of vertical farms has been expanding rapidly in recent years to provide more resilient and sustainable global food provisioning closer to consumers. However, there is a lack of empirical evidence on whether vertical farms can provide sustainable sourcing of food. The purpose of this study is to assess the environmental performance of a modular cabinet vertical farm producing lettuce and basil on-site at the end-user. To assess the environmental performance of this system, a life cycle assessment (LCA) was conducted to determine the total impact of producing lettuce and basil on-site, i.e., a kitchen for an office cafeteria, and compare to conventional sourcing. The results from this study suggest that the modular vertical farm can provide crops with environmental impacts comparable to or less than conventionally sourced options. GHG emissions ranged from 0.78–1.18 kg CO2-eq per kg lettuce and from 1.45–2.12 kg CO2-eq per kg basil from on-site production. The ranges suggest that the environmental performance is sensitive to methodological choices and life cycle inventory (LCI) data choices. These include how to treat the infrastructure for the modular cabinet, as it is often rented as a growing-service system, in addition to the LCI data choices related to the source of electricity. In conclusion, under local conditions (i.e., Stockholm, Sweden) the modular vertical farm can produce lettuce with equivalent emissions and quality to imported lettuce, despite its high energy requirement. The findings and knowledge from this study add to the growing body of literature on vertical farming, providing empirical evidence on the sustainability of an on-site commercial cabinet-based vertical farm. Such information can be used for comparisons and validation of claims in the industry, and to provide empirical evidence to this developing field.
1 Introduction
Finding solutions for more resilient food production techniques closer to urban surroundings with lower vulnerability to supply-chain shocks is becoming increasingly crucial as urban populations continue to grow (Benke and Tomkins, 2017; O’Sullivan et al., 2020; Pulighe and Lupia, 2020). This necessity has led to the development and expansion of novel agricultural production systems to bring food production closer to end consumers, including vertical farming (van Delden et al., 2021; Blom et al., 2022; Casey et al., 2022). In the context of this research, the term vertical farm refers to an indoor crop production system comprised of multiple levels. Within these layers, the environmental conditions for plant growth are carefully managed, and the plants are nurtured using artificial lighting, e.g., light emitting diodes (LEDs) (Butturini and Marcelis, 2020; Kozai and Niu, 2020; van Delden et al., 2021). These include a large variety of production systems, ranging from large-scale warehouses, farms in basements of buildings, and modular systems, such as container farms and modular-cabinet farms in retail settings (Butturini and Marcelis, 2020; Martin and Bustamante, 2021; van Delden et al., 2021).
In recent years vertical farms have grown dramatically, garnering significant interest and funding from all over the world due to their potential to extend seasonal availability, reduce resource demand, secure food supplies, and ease pressure on agricultural land (Despommier, 2011; Thomaier et al., 2014; Graamans et al., 2018; van Delden et al., 2021; Martin and Bustamante, 2022). Nonetheless, vertical farms are still a new concept for food supply chains and are a growing area of research (Orsini et al., 2020; van Delden et al., 2021; Martin et al., 2023b,c). Much of the attention in the field has been placed on large commercial systems (Martin and Bustamante, 2021). Despite this, small-scale modular systems, such as in-store growing systems, and vertical farms connected to cafeterias and kitchens have grown in popularity and number in recent years worldwide due to the need to expand offerings, business models, and ways of connecting with customers (Bustamante, 2020; Butturini and Marcelis, 2020; Martin and Bustamante, 2021). Several vertical farms have received sizable investments for the development of modular systems in recent years (see, e.g., Jürkenbeck et al., 2019; Butturini and Marcelis, 2020; Renmark, 2021; InFarm, 2021).
These smaller systems are typically installed in an existing space, where the vertical farm provides customized solutions for the needs of the customer, whether the plants are used for consumption, e.g., in restaurants or cafes, or for resale, e.g., in food retailers. This introduces a new business model, where the vertical farming company often retains ownership and control of the infrastructure while the operation and farming are provided as a service, i.e., ‘growing as a service’ (Martin and Bustamante, 2021). Therefore, in this study, we refer to these developing modular systems as growing-service systems (GSS), as they are naturally connected to the concept of product-service systems (PSS) which are oriented toward achieving both economic and environmental outcomes (Tukker, 2004; Martin and Bustamante, 2021). Increasingly, the opportunity that these systems provide compared to conventional supply chains based on centralized production is being explored (Tukker, 2004; Mont et al., 2014; Geissdoerfer et al., 2018).
Vertical farming is frequently advocated in the literature as a sustainable method of supplying food (Despommier, 2011; Benke and Tomkins, 2017; Martin and Bustamante, 2022). However, there are very few analyses of the environmental effects of vertical farms in the scholarly literature (Orsini et al., 2020; Martin and Orsini, 2023; Martin et al., 2023b). There have been few cases where the environmental sustainability of vertical farming has been thoroughly investigated (Dorr et al., 2021; Martin et al., 2022; Weidner et al., 2022; Martin and Orsini, 2023; Martin et al., 2023b). Nevertheless, some recent evaluations of vertical farming systems, including container farms, have been carried out to provide empirical evidence on the impacts of commercial systems (Blom et al., 2022; Casey et al., 2022). However, the majority of research on vertical farms is devoted to theoretical evaluations to compare their effectiveness to other systems like open-field agriculture and greenhouses. There are also no studies of modular vertical farming systems, such as those in-store or connected to the kitchen systems, available in the literature, although investigations related to the business models and practices for these new approaches have been studied in Martin and Bustamante (2021) and recently in Drottberger and Langendahl (2023).
Empirical data on the viability of real commercial scenarios is lacking in the literature. While some of this can be attributed to the novelty of these systems, there needs to be more proof to support vertical farming’s promises of resource efficiency and reduced environmental impacts (Martin and Orsini, 2023). Furthermore, the environmental performance of vertical farms could also vary depending on the context, closely tied to local circumstances like the available energy systems (Graamans et al., 2018; Martin et al., 2022; Weidner et al., 2022; Martin and Orsini, 2023), sizes, locations within or outside the urban environment. Evaluations of the impacts of vertical farms compared with conventionally sourced produce are also important to highlight and compare to find the potential hotspots, drawbacks, or benefits of such systems.
The present study aims to assess the environmental performance of lettuce and basil production from a case study modular-cabinet vertical farm. Thereafter, the objectives are to (1) highlight the hotspots of the system, (2) compare vertically farmed produce with conventionally sourced produce, and (3) analyze the sensitivity to methodological choices and data in the life cycle assessment.
2 Methodology
The following sections outline the modular-cabinet vertical farm assessed in this study, a description of the methodology used to assess the environmental performance, how this was compared to conventionally sourced produce, and analyses conducted.
2.1 Modular-cabinet vertical farm (GrowOff)
The study evaluated the modular-cabinet vertical farm, hereafter referred to as GrowOff, developed by Grönska Stadsodling in Stockholm, Sweden (see Figure 1). Further details about the size and specifications are provided in Supplementary material.
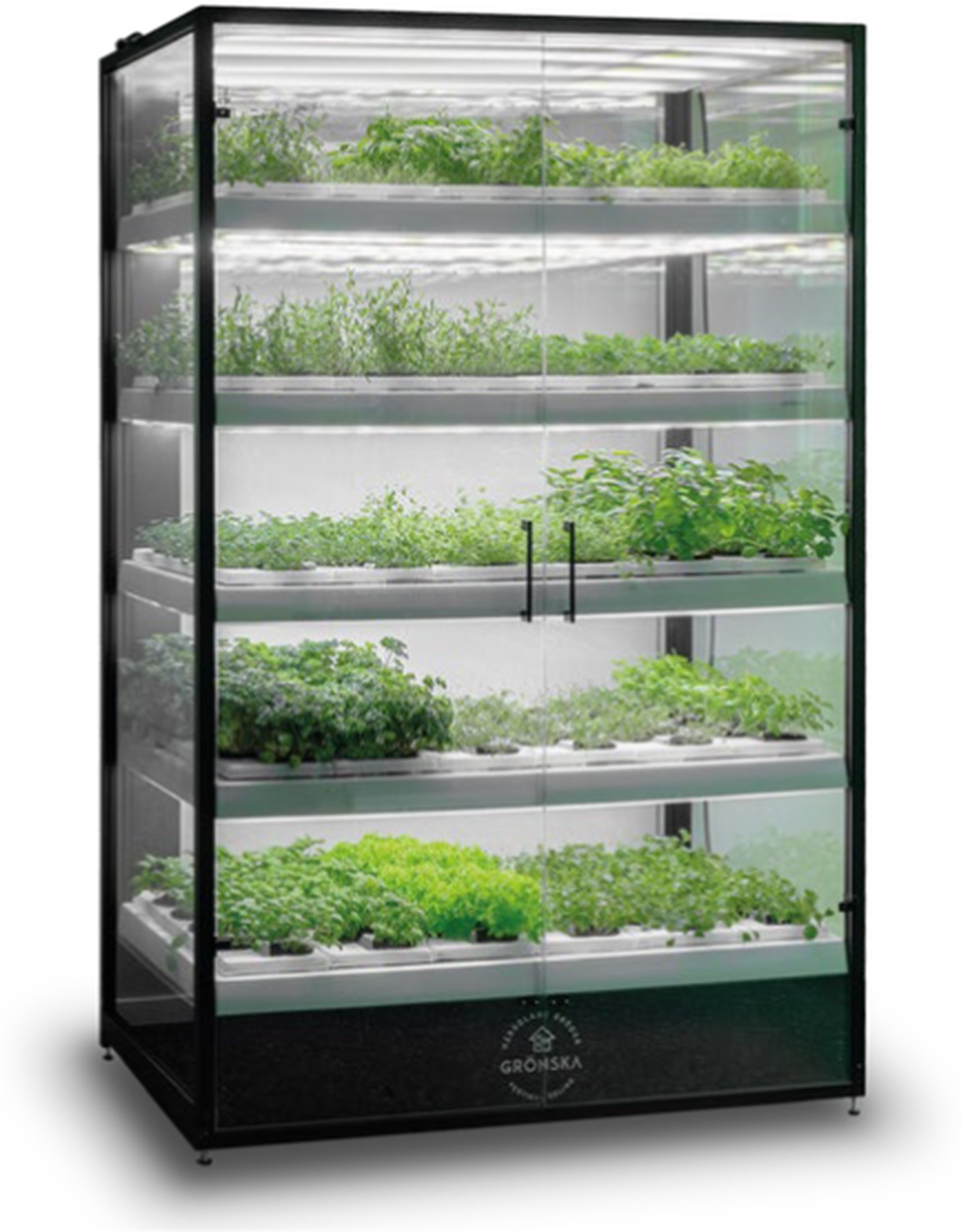
Figure 1. Depiction of the modular vertical farming system studied in this article, namely the GrowOff module. Permission from Grönska Stadsodling (www.gronska.org).
The GrowOff farm was designed to be employed in different environments to produce crops grown hydroponically and are currently in place around Sweden in cafeterias, restaurants, and supermarkets. The case was selected to provide a life cycle analysis to evaluate the viability of vertical farming in smaller systems, such as cabinet-based vertical farms, while also taking into account the general absence of comparable studies in the literature. It also provides further analysis of the environmental implications of new business models, such as growing service-systems (GSS), highlighted recently in a previous study (Martin and Bustamante, 2021).
Growing service-system models are an interesting system to review, as they are generally modular and have a high degree of autonomy. As depicted in Figure 2, the GrowOff farm is connected to a cloud-based server and application. From the application the end-user, and Grönska (the provider), can monitor and control the growing conditions in the farm. Using this information, and keeping track of inventory locally, the delivery of new consumables and seeded trays is performed once the inventory becomes low. Material, including seeded trays, nutrients, and pH control are sent to the end user once every few months. However, the application can monitor whether there is a need to send more of any material or consumables, and if there are any requirements for maintenance. Furthermore, the application can also provide feedback to the end-user on harvest periods and any other important insights. All the information can also be used to further optimize production based on its location and context. More about such growing-service systems, also referred to as farming as a service, can be found in Martin and Bustamante (2021).
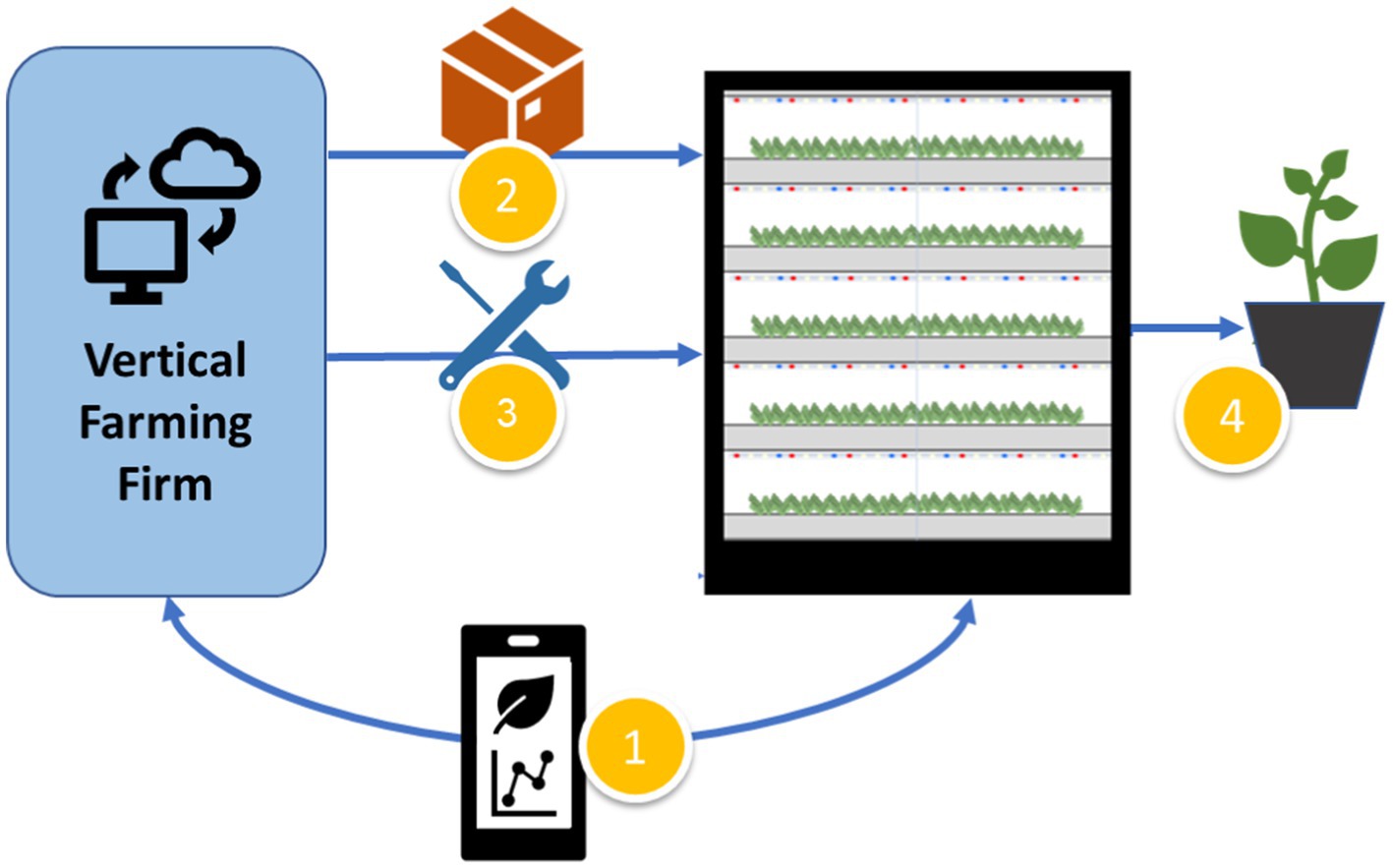
Figure 2. Review of the GrowOff farm system, based on Martin and Bustamante (2021). The farm and vertical farming firm are connected through (1) cloud-based application to monitor and provide feedback for (2) consumables and other inputs, (3) possible maintenance and optimization, and finally (4) information about the cultivation and final harvesting periods.
2.2 Life cycle assessment and life cycle inventory
Life cycle assessment was employed to study the environmental impacts of producing crops in this system and to compare them with other conventionally sourced products. The functional unit employed for the environmental assessment is 1 kg of edible produce available at the point of use. In this study, this includes both lettuce and basil, available to the end user, and are analyzed separately in this study. Data for this study were collected in collaboration with the producer and provider, Grönska, based on data for annual production of lettuce (Lactuca sativa-Crispinet) and basil (Ocimum basilicum-Genovese) from their own testing facility. In this study, it is assumed that the GrowOff farm is connected to a kitchen at a cafeteria, where the products are used directly, and thus no additional packaging and transportation are needed. This assumption is made as the primary markets for the GrowOff farm in Sweden are in such environments.
The study was conducted using a cradle-to-grave perspective, including the GrowOff farm infrastructure, cultivation, material inputs, energy, and transportation of materials and infrastructure. Wastes and waste handling from the GrowOff farm and its use in the cafeteria are also included in the assessment. However, waste handling for the infrastructure is not included as the vertical farm infrastructure ownership adds to the complexity of allocating its impacts between the user and producer. Nonetheless, a sensitivity to show the influence this would have on the overall results is also included in the analysis section. A depiction of the system boundaries of the study is illustrated in Figure 3.
OpenLCA v1.10.3 and Excel were used to conduct the life cycle assessment. The life cycle impact assessment (LCIA) method employed in this study was the Environmental Footprint v. 3.0 method, as it provides a robust method for exploring the implications of products and services in the European market. The impact categories included in this study are Global warming potential (measured in kg CO2-eq and denoted GHG), Acidification Potential (measured in molc H+ eq and denoted Acid.), Freshwater Eutrophication (measured in kg P-eq and denoted Fresh. Eutroph.), Freshwater Ecotoxicity (measured in CTUe and denoted Ecotox. Fresh.), Land Use (measured in Pt and denoted LU), Resource Depletion, Fossils (measured in MJ and denoted RU Fossils), Resource Depletion-Materials and Metals (measured in kg Sb-eq and denoted RU min. & met.), and Water Use (measured in m3 and denoted WU). These impact categories were chosen as they are used in previous assessments of food system sustainability analyses and highlight important regional, global, and resource implications pertinent to environmental concerns in Sweden (see, e.g., Joint Research Centre, Institute for Environment and Sustainability, 2010; SEPA, 2015; Martin and Brandão, 2017; Martin et al., 2022). Additionally, such impact categories are frequently used in studies of horticultural systems, allowing for comparisons, as seen in previous studies in the field (see, e.g., Bartzas et al., 2015; Dias et al., 2017; De Pascale et al., 2018; Martin et al., 2022).
Life cycle inventory (LCI) data to match all material, energy, processes, and infrastructure was obtained from Ecoinvent v. 3.8 (Ecoinvent, 2022). Details and assumptions for the production of lettuce and basil with the GrowOff farm are provided in the subsequent sections and further details are provided in Supplementary material, including a listing of all LCI datasets employed in the study (see Supplementary Table S2).
2.3 Scenarios
2.3.1 GrowOff-Lettuce
The baseline scenario GrowOff-lettuce is based on the annual production of lettuce in the GrowOff farm. This includes all infrastructure, material inputs, production outputs, wastes, transportation, and energy consumption. The annual production of lettuce assumed in the GrowOff-Lettuce scenario is roughly 2,404 lettuce plants, weighing 100 g each, producing a total output of 240 kg annually. The harvest time for lettuce is roughly 41 days with a planting density of 62 lettuce plants per m2 (see Table 1 for further details).
2.3.2 GrowOff-Basil
The GrowOff-Basil Scenario is similar to the GrowOff-Lettuce scenario above. The production outputs are different as the basil cycle is shorter, roughly 30 days, and has a planting density of roughly 69 basil plants per m2. As such, the total output is instead roughly 3,600 pots of basil. This amounts to roughly 144 kg of edible basil annually, assuming each pot is grown to contain roughly 40 g of edible basil. Furthermore, less water and fertilizer are required compared to lettuce although with an increased number of pots, the weight of the pots, growing media, and weight is increased while the weight of seeds and fertilizer are reduced. Further details are provided in Supplementary Table S1.
2.3.3 Energy system scenarios
Several scenarios are also included to test the influence of the choice of electric sourcing, as the GrowOff farms can be placed in different locations, and each end-user may have different electricity sourcing or certifications which can affect the environmental performance of the produce. The Baseline scenarios (GrowOff-Lettuce and GrowOff-Basil) are based on the average Swedish Electricity Mix, which includes hydro (39%), nuclear (41%), wind (10%), combined heat and power (9%) and Solar (less than 1%) (Swedish Energy Agency [SEA], 2021). As such, the study can be sensitive to the choice of electricity employed. The Swedish electricity mix was compared with datasets for different sourcing. This included sourcing only from hydropower, labeled ‘Hydro,’ and a mix including 50% of wind and 50% hydropower sourced electricity, labeled ‘Hydro/Wind’.
2.3.4 Infrastructure
The GrowOff farms are either purchased or leased as growing-service systems which are product-service system offerings (Martin and Bustamante, 2021). In the case of leasing a unit, the vertical farming company, Grönska, owns the infrastructure, and it is used by end-users for the production of the crops. As such, the method by which infrastructure impacts are allocated to the end-user and final products may be sensitive. In this study, in the baseline scenarios, GrowOff-Lettuce and GrowOff-Basil, all infrastructure impacts are allocated to the end user. However, for the scenarios labeled additionally with ‘Split Infra’, only 50% of the infrastructure impacts are allocated to the end user to fairly distribute the impacts between the user and the producer if the unit is leased. As such this can affect the environmental performance of the produce from the GrowOff farm.
2.4 Conventional supply chains
To compare the results of the GrowOff farm’s produce with conventional supplies, the sourcing of the lettuce and basil production from conventional production methods was included. Based on previous research and data on lettuce imports, it was assumed that lettuce was sourced from greenhouses and conventional open-field production in Spain, Italy, and the Netherlands (BAMA, 2023; Martin et al., 2023b), yet sourced from Sweden during the summer, which is assumed to be produced in greenhouses and open fields. LCI data for lettuce production in Italy, i.e., in greenhouses was obtained from Fusi et al. (2016). Data for lettuce production in Spanish open-fields was obtained from Casey et al. (2022). Blom et al. (2022) datasets for greenhouse production in the Netherlands were also employed. For this study, these datasets were remodeled in order to provide comparable results using the same LCIA methods and associated impact categories. For Swedish lettuce production, LCI data provided by the Agribalyse v. 3.1 (Agribalyse, 2022) database based on conditions in France were employed for open fields and polytunnel lettuce production. Additionally, transportation logistics were also modeled as a separate process to allow for the lettuce to be available at the end-user, in this case a cafeteria or restaurant. For all conventionally produced lettuce, it is assumed that the lettuce is transported from its origin to Helsingborg, Sweden where it is processed. This includes cutting, washing, and repackaging for distribution to end users in packaged lettuce bags (BAMA, 2023). For this study, a distance of 500 km was chosen, assuming the lettuce will be used in the Stockholm region. Supplementary material contain additional information on the modeling of conventionally supplied lettuce.
For basil it was assumed that conventional sources of basil include open-field production in Italy and greenhouse production in Sweden as basil is often sold as potted plants or cut bundles in packaging in Sweden. All data for farm-to-gate production impacts are developed based on conventional production available for basil in the scientific literature (see, e.g., Martin et al., 2023a) and from Agribalyse LCI datasets specifically for basil. Additionally, data from the gray literature, such as reports and carbon footprint figures are employed for comparisons, although limited to the GHG emissions, as LCA data for herbs is generally absent in the scientific literature. All transportation for basil is also included, both from primary production to the wholesaler and finally to the end-user.
3 Results and analysis
The following sections provide results from the environmental life cycle assessment of the GrowOff farm producing both lettuce and basil separately. This is done by first presenting the impacts of the baseline scenario. Thereafter, the implications of different scenarios to highlight the influence of data sources and methodological considerations are analyzed. Finally, a comparison to conventionally supplied lettuce and basil is provided.
3.1 GrowOff-Lettuce scenarios
The contribution of various processes to the baseline, GrowOff-Lettuce, scenario’s environmental impacts is illustrated in Figure 4. As indicated, electricity contributes to the greatest share of the environmental consequences in essentially all environmental impact categories, except for the Resource Use-Minerals and Metals category where the infrastructure dominates.
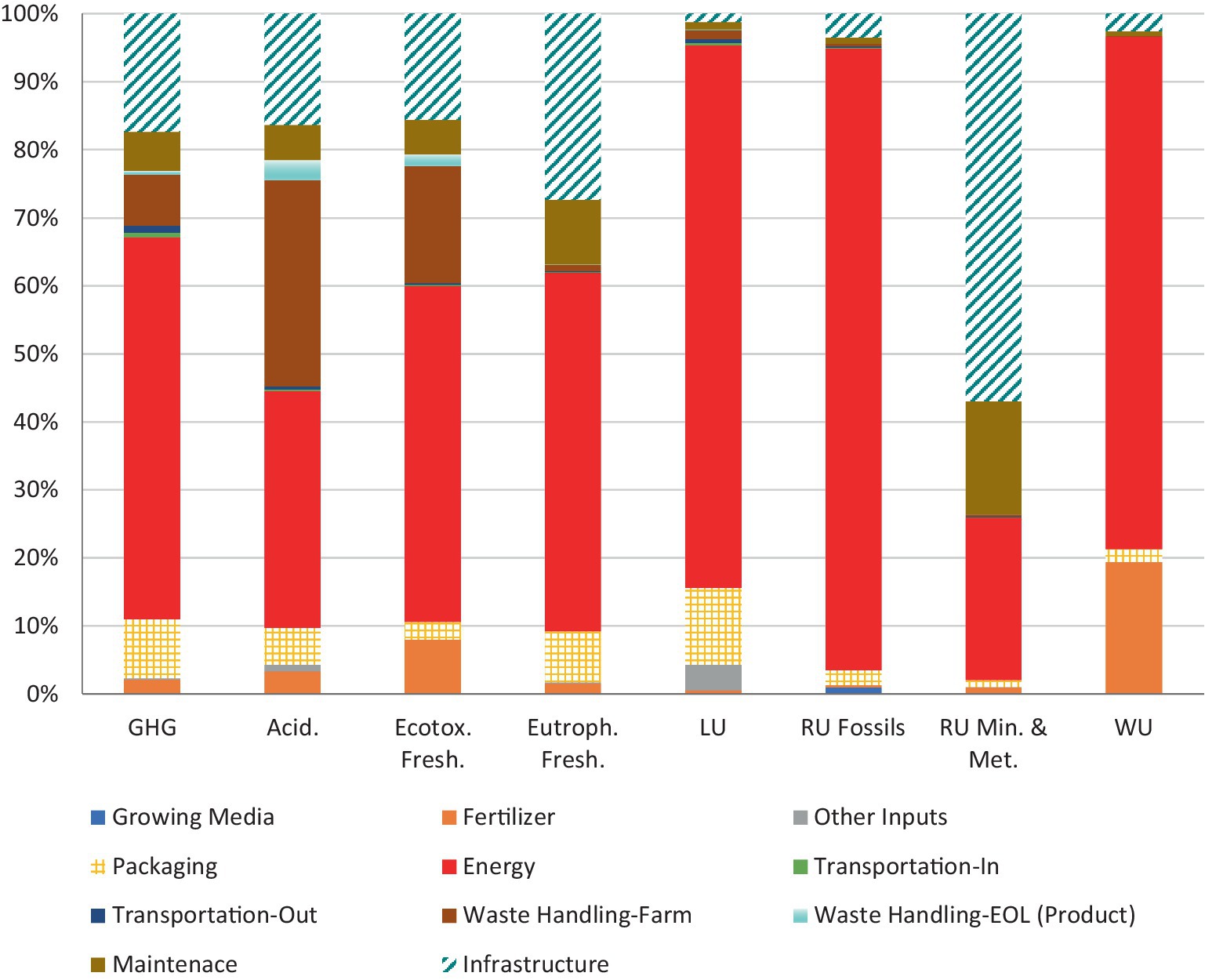
Figure 4. Contribution of different processes to the environmental impacts of 1 kg of edible lettuce for the GrowOff-Lettuce scenario (GHG, greenhouse gasses; Acid., acidification; Ecotox. Fresh., ecotoxicity freshwater; Eutroph. Fresh., eutrophication freshwater; LU, land use; RU Fossils, resource use fossils; RU Min. & Metals, resource use-minerals and metals; WU, water use).
Other processes contributing largely to the impact categories include infrastructure, waste handling, maintenance, and fertilizers. For the Resource Use (RU Minerals and Metals) impact category, infrastructure contributed to the largest share of impacts, where over 80% of the contribution to resource use was found to be attributable to the metals and electronics required for the infrastructure. Table 2 provides the impacts per kg of lettuce produced for further details.
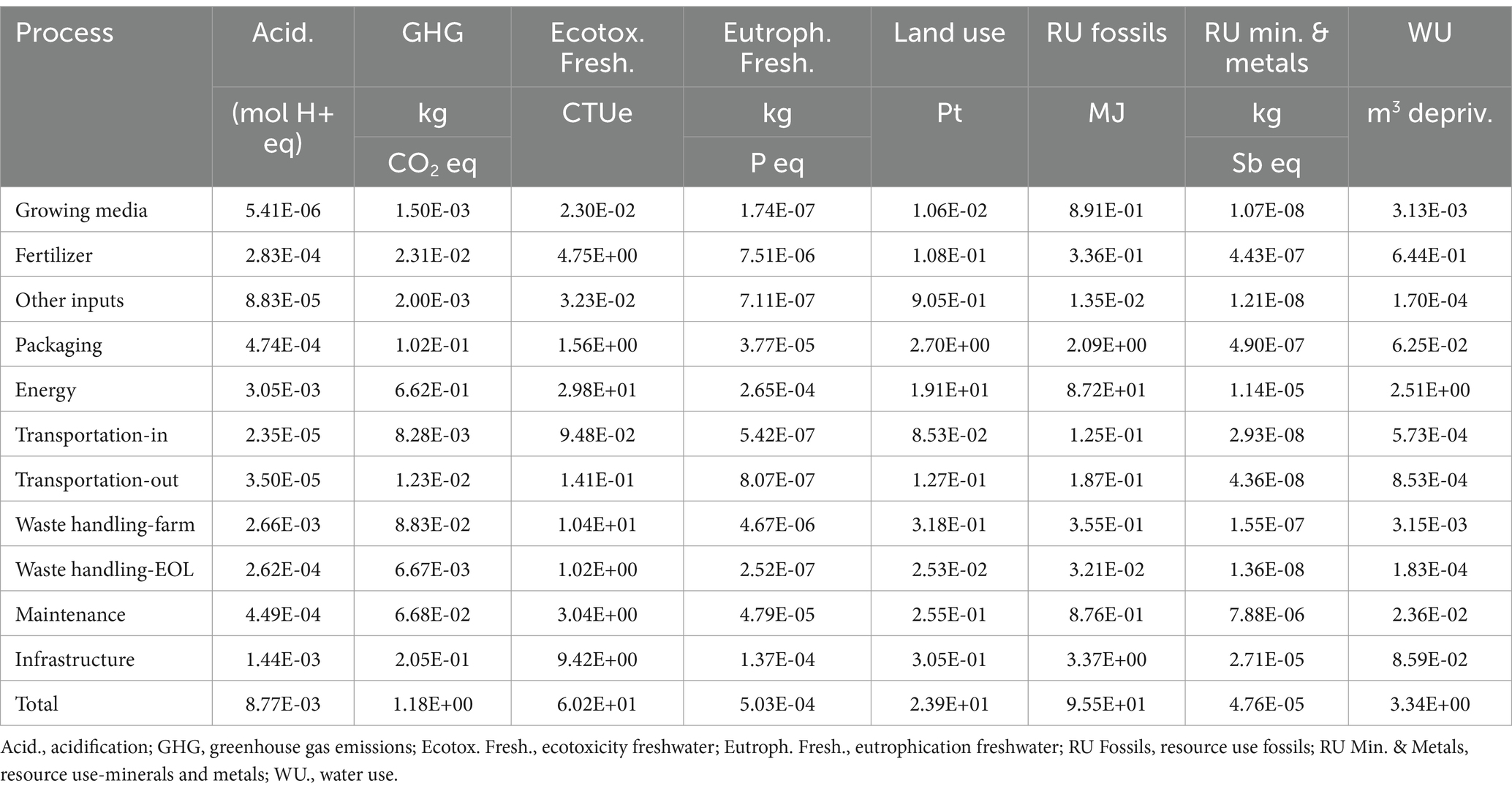
Table 2. Analysis of the contribution of different processes and inputs to the life cycle impacts of 1 kg of edible lettuce for the GrowOff-Lettuce scenario.
For the GrowOff-Lettuce scenario, the GHG emissions associated with lettuce production from the GrowOff farm are roughly 1.18 kg CO2-eq per kg edible lettuce. It can also be denoted that roughly 3.3 m3 of water is deprived through the production of 1 kg of lettuce, which is primarily due to the contribution of water depleted from electricity. It can also be denoted that the results for water use are for the impact category Water depletion. As such, the results suggest that roughly 3 L of water is deprived through the production of 1 g of lettuce, which is primarily due to the contribution of water depleted from the electricity conversion process (hydropower). Once again this is for the entire life cycle water depletion for all processes to produce the lettuce. It should be noted that this is large in comparison to the farm-based use of water of roughly 0.014 L per g of lettuce (resulting in a Water Use Efficiency of about 71.4 g of fresh biomass per liter of water consumed).
3.1.1 Sensitivity to electricity sourcing and infrastructure for lettuce
Figure 5 provides a review of the sensitivity of the choice of electricity sourcing and the methodological choice for the allocation of infrastructure impacts, both separately and combined. As illustrated, the electricity impacts are reduced largely through the choice of either 100% hydro or a mix of hydro and wind power. Accordingly, employing a mix of hydro and wind power would lower the GHG emissions to roughly 0.87 kg CO2-eq per kg of lettuce.
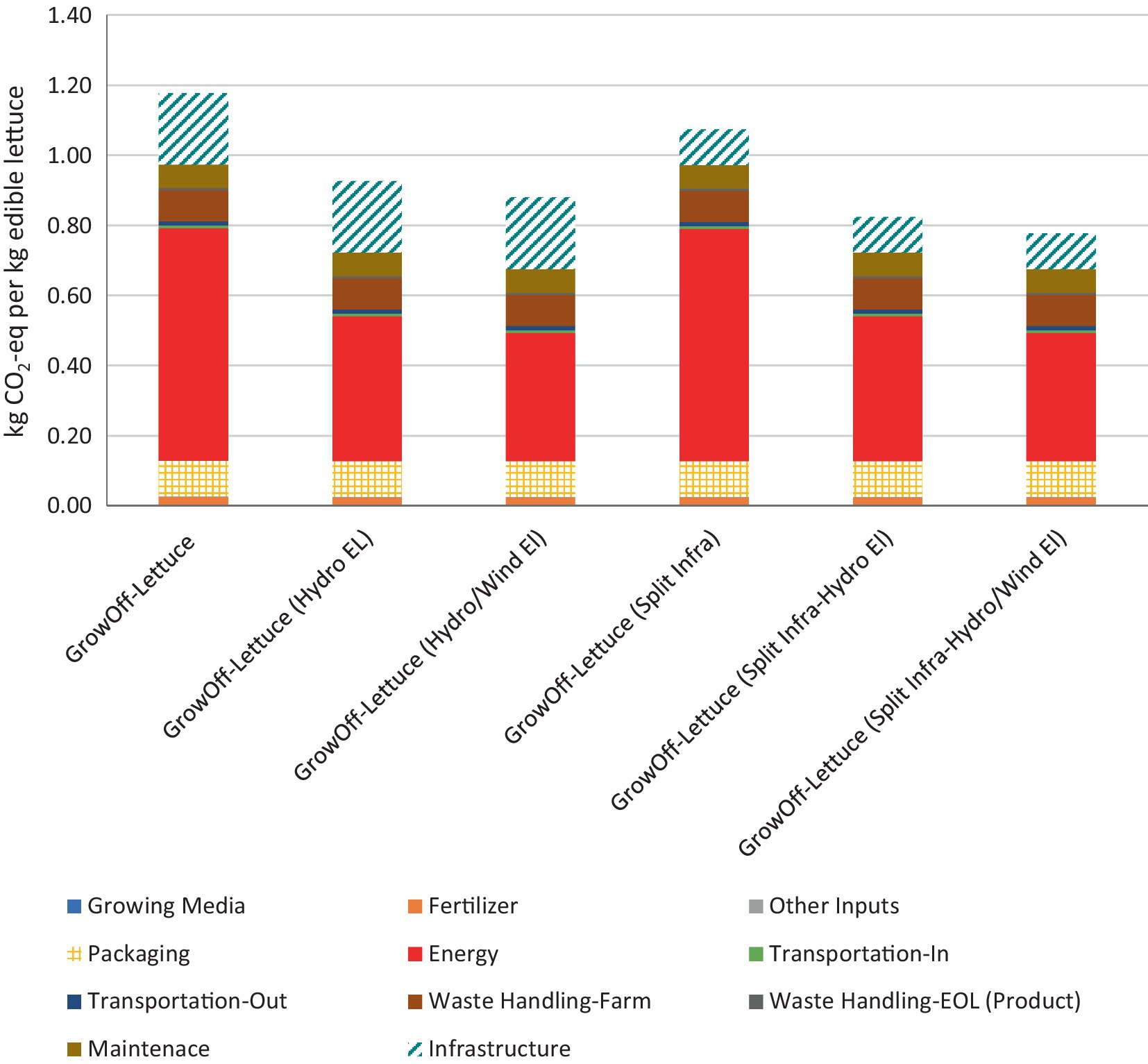
Figure 5. Illustration of the influence of the selection of energy sourcing and infrastructure allocation for 1 kg of edible lettuce for GHG emissions (shown in kg CO2-eq per kg edible lettuce).
Furthermore, by allocating only 50% of the infrastructure impacts, the emissions are also reduced. The GrowOff-Lettuce (Split Infra) scenario had GHG impacts of roughly 1.07 kg CO2-eq per kg of lettuce. If combined with a renewable electricity mix, i.e., a mix of hydro and wind power electricity, the GHG emissions are further reduced to 0.77 kg CO2-eq per kg of lettuce.
As Table 3 outlines, the renewable-based electricity scenarios reduced nearly all environmental impacts, although an increase in water use was highlighted due to the higher inclusion of hydropower electricity. Furthermore, nearly all scenarios have lower land use impacts compared to the GrowOff-Lettuce scenario. This was found to result from a large share of land use impacts originating from biomass employed for combined heat and power plants, which dominated the land use category due to the use of wood chips and other forest residues as fuel in the GrowOff-Lettuce scenario which employed the Swedish mix. The ‘Split Infra’ scenarios had lower environmental impacts in all categories, with notably less resource use of minerals and metals due to less allocation of the infrastructure. Finally, for scenarios including a mix of hydro and wind power scenarios, a slight increase in resource use-minerals and metals, was found due largely to an increase in infrastructure for wind power.
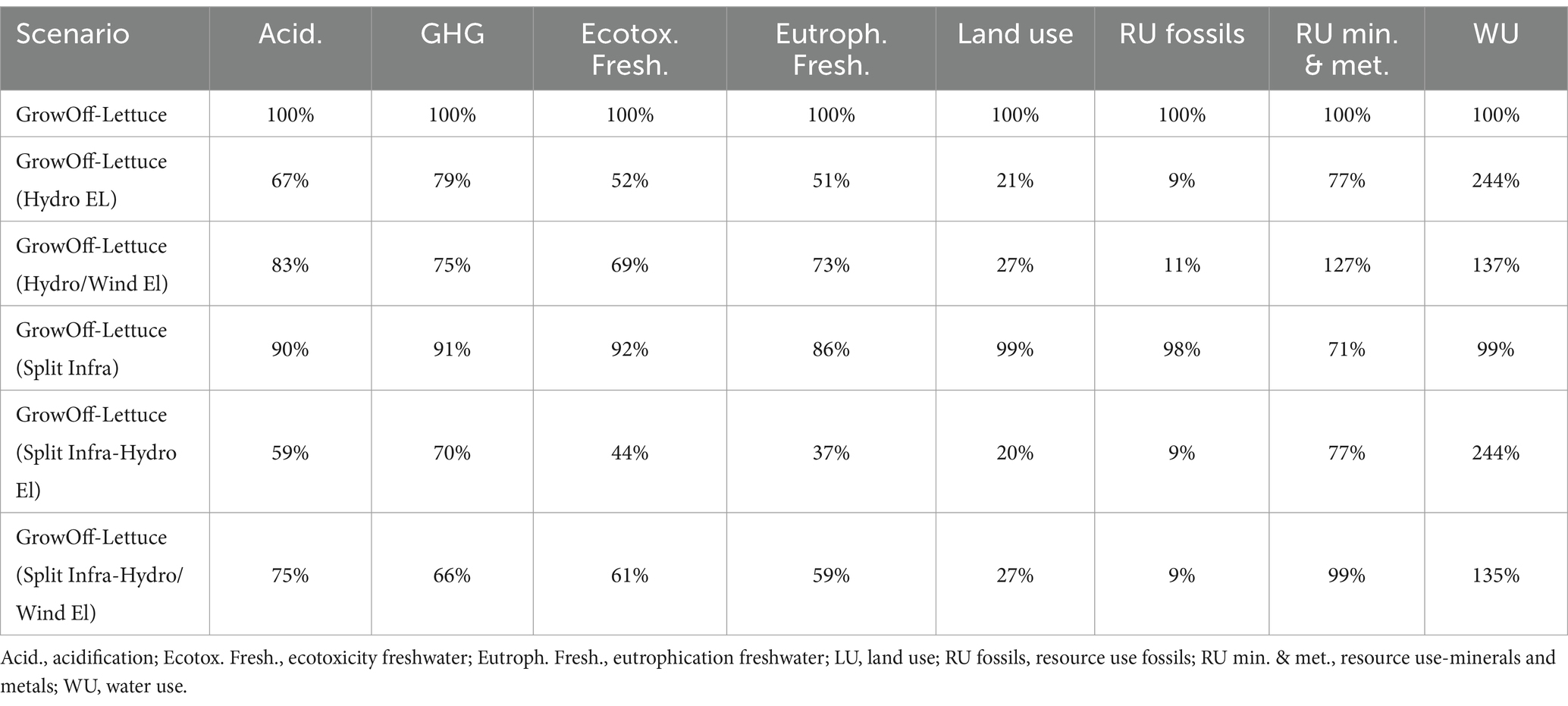
Table 3. Sensitivity to the choice of electricity system employed and allocation of infrastructure impacts on the different environmental impacts for the lettuce production (shown % change compared to the Baseline results).
3.1.2 Comparison to conventional lettuce sources
As shown in Figure 6, the GrowOff produces lettuce with lower GHG emissions than most conventionally sourced lettuce. The highest and lowest impacts for the GrowOff, i.e., GrowOff-Lettuce and GrowOff-Lettuce (Split-Infra Hydro/Wind) scenarios were compared with other alternatives. For example, while the production from imported lettuce from field-based production systems are much lower, their distribution impacts from both importing and domestic distribution largely increase the impacts from these sources. As such, all imported lettuce was found to have higher impacts than the GrowOff. However, although only seasonally available, Swedish-produced lettuce was found to have equal or lower emissions than the GrowOff, depending on which scenario is compared. For example, the impacts for the Swedish greenhouse production were similar to the GrowOff-Lettuce scenario. However, the field produced, both in open-field and polytunnels, had lower impacts than the GrowOff-Lettuce. However, compared to the GrowOff-Lettuce (Split Infra-Hydro/Wind El) scenario, the Swedish-sourced lettuce scenarios were all higher.
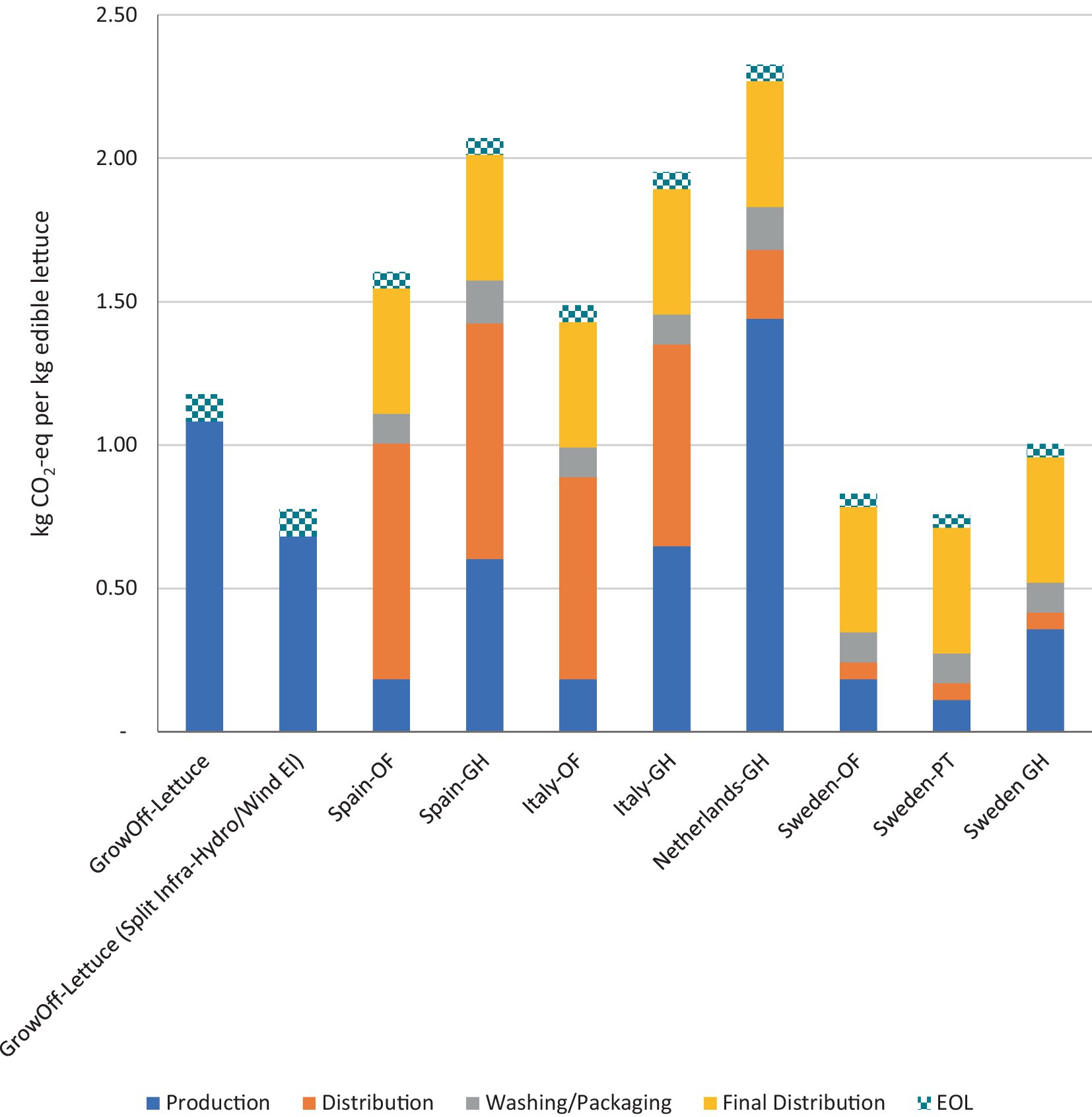
Figure 6. Comparison of the GHG emissions for the GrowOff farm lettuce versus conventionally sourced lettuce (shown in kg CO2-eq per kg edible lettuce). OF, open field; GH, greenhouse; PT, polytunnel.
3.2 GrowOff-Basil
As illustrated in Figure 7, the impacts of the different inputs and processes for the Baseline-Basil scenario are similar to those in the Baseline-Lettuce scenario. However, the contribution of the water and fertilizers is reduced, due to the reduction in demand for water and fertilizer for basil production.
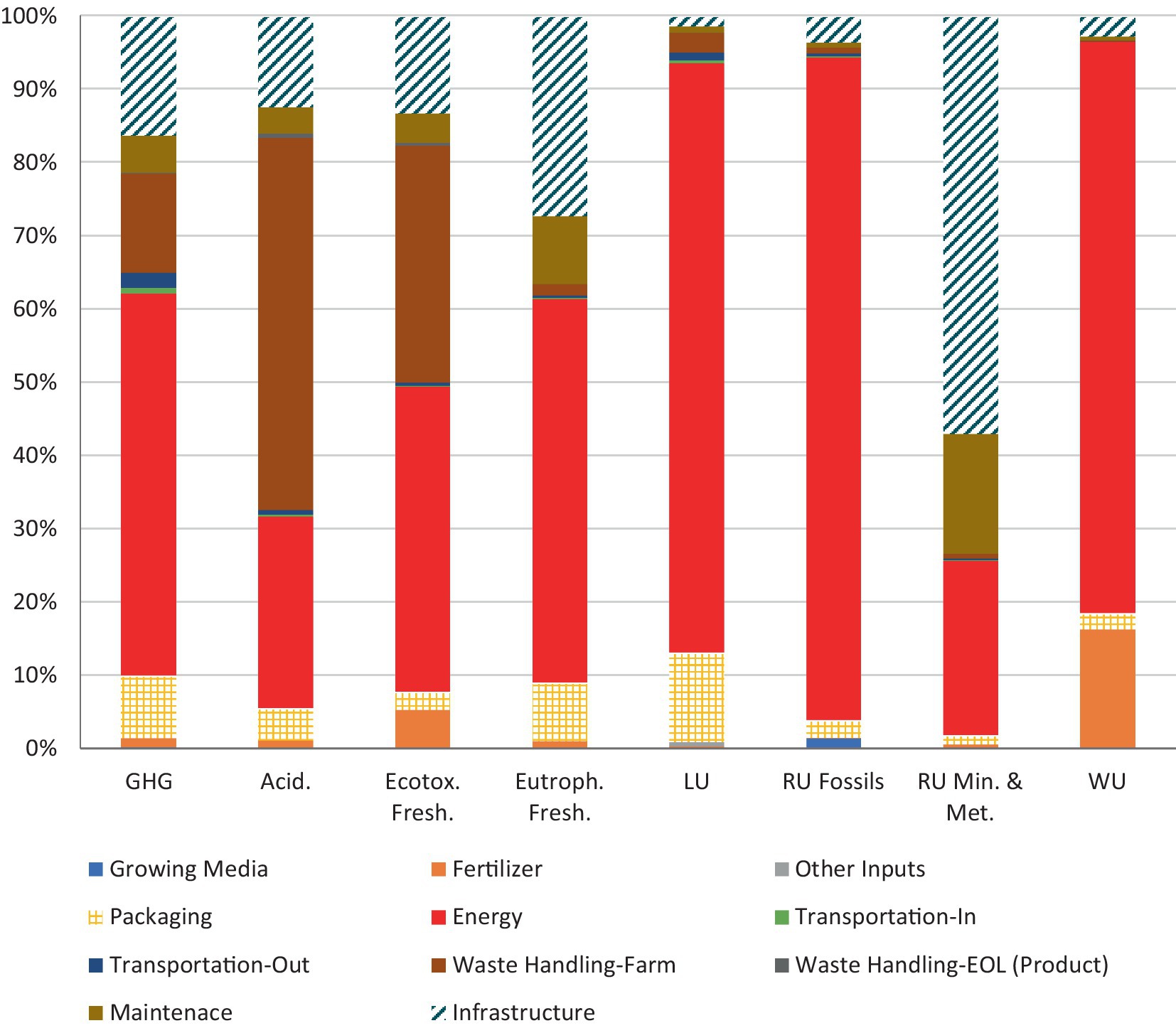
Figure 7. Contribution of different processes to the environmental impacts of 1 kg of edible basil for the GrowOff-Basil scenario (GHG, greenhouse gases; acid., acidification; Ecotox. Fresh., ecotoxicity freshwater; Eutroph. Fresh., eutrophication freshwater; LU, land use; RU fossils, resource use fossils; RU min. & met., resource use-minerals and metals; WU, water use).
Table 4 provides a breakdown of the different impacts per kg of basil produced. As shown the GHG emissions are roughly 2.12 kg CO2-eq per kg of edible basil. It can also be denoted that roughly 5.4 m3 of water is deprived through the production of 1 kg of edible basil, which is primarily due to the contribution of water depleted from electricity. As such the GHG emissions and the water deprivation are roughly double those compared to lettuce, resulting in a water use efficiency of roughly 20 L per kg of edible basil (or 50 g of edible basil biomass per liter of water consumed).
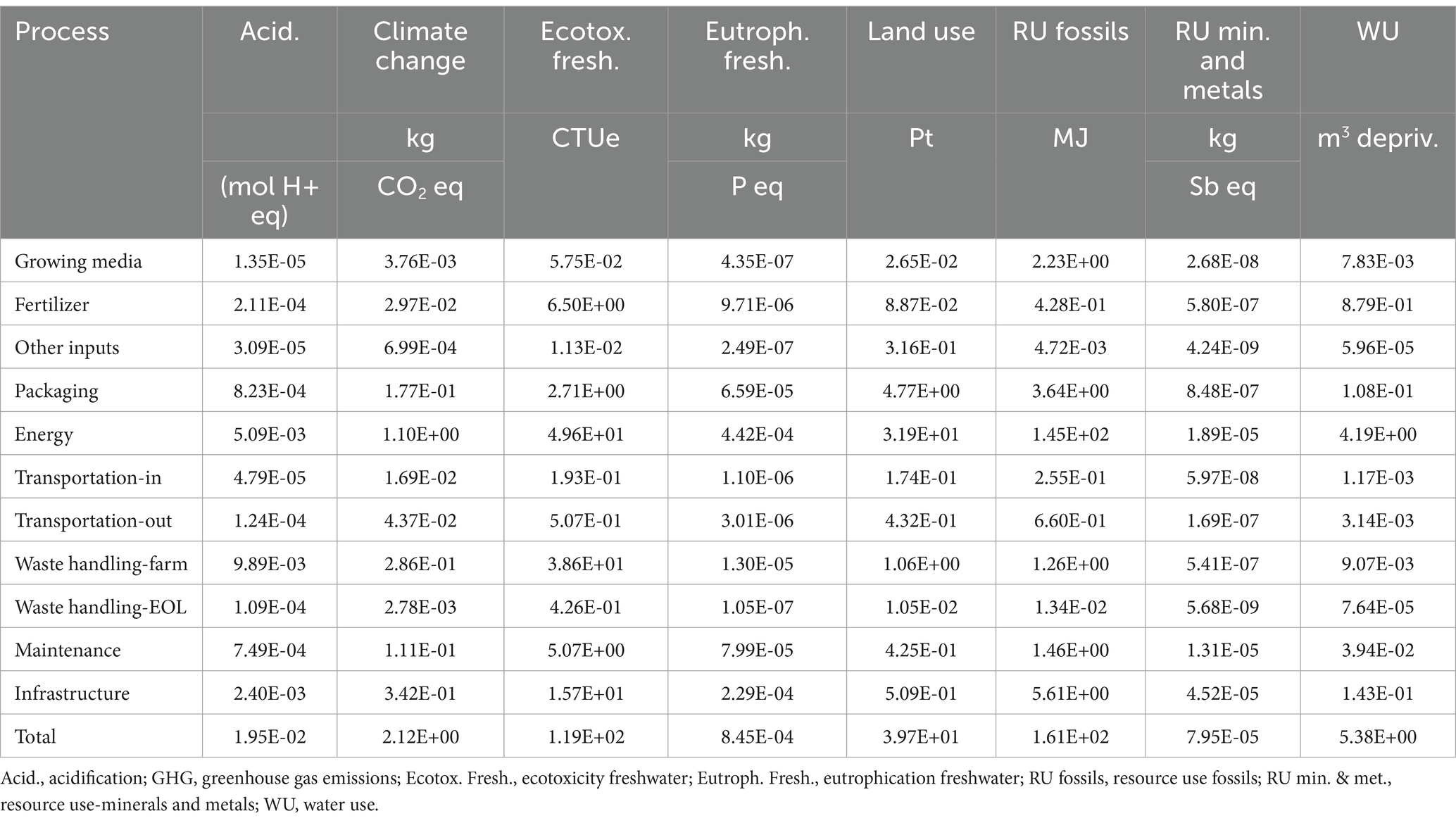
Table 4. Analysis of the contribution of different processes and inputs to the life cycle impacts of 1 kg of edible basil for the GrowOff-Basil scenario.
3.2.1 Sensitivity to methodological choices (electricity supply and infrastructure) for basil
Figure 8 provides a review of the sensitivity of electricity sourcing for basil GHG emissions. As demonstrated, electricity sourcing has a large influence on the results. Compared to the baseline scenario GrowOff-Basil, all scenarios employing only renewable energy were shown to have lower GHG emissions. While the GrowOff-Basil scenario has GHG emissions of roughly 2.12 kg CO2−eq/kg edible lettuce, the GrowOff-Basil-Hydro and GrowOff-Basil (Hydro/Wind) further reduce GHG emissions to roughly 1.68 and 1.60 kg CO2−eq per kg edible basil, respectively.
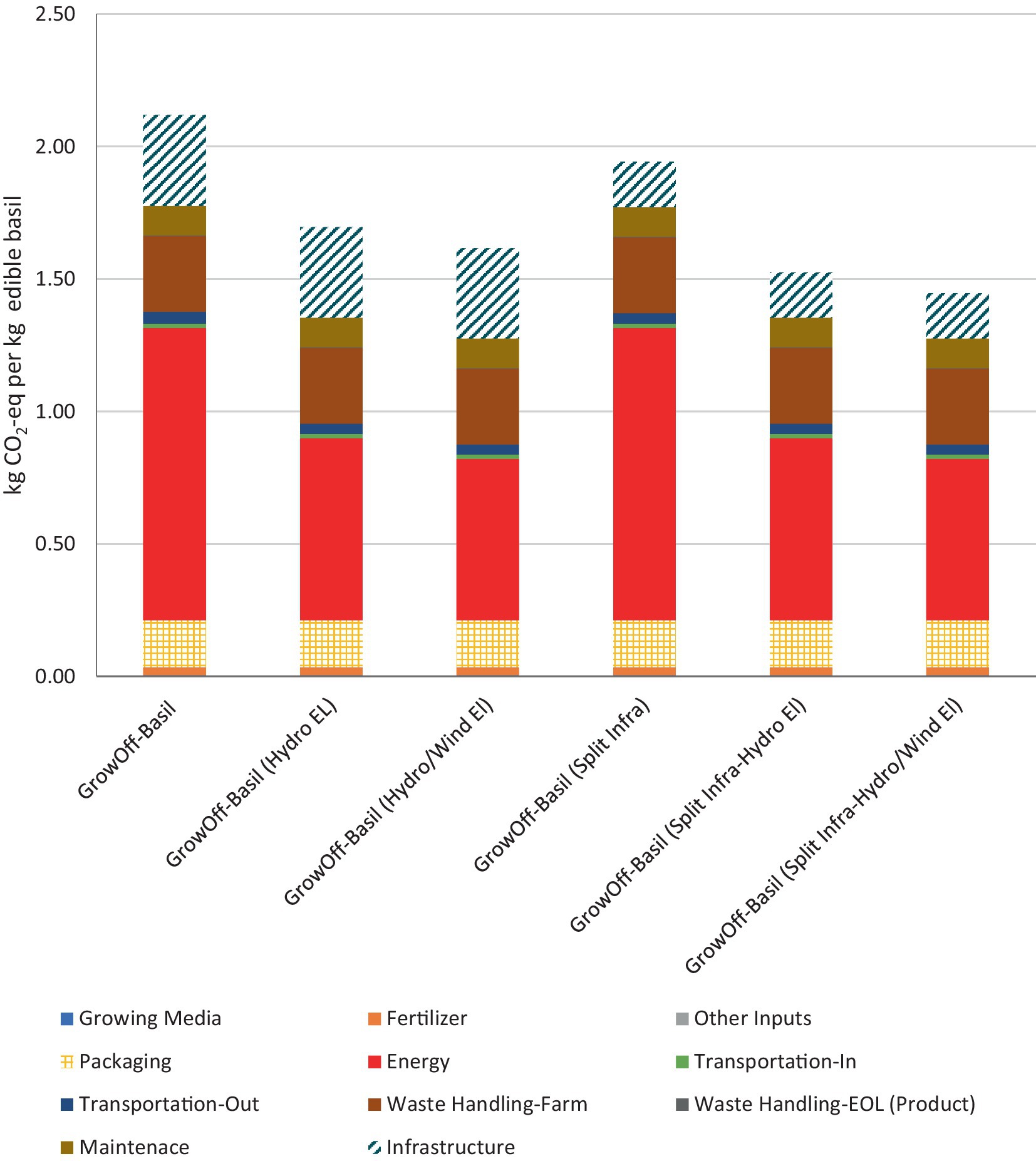
Figure 8. Illustration of the influence of the selection of energy sourcing and infrastructure allocation for 1 kg of edible basil.
Once again, also for basil, allocating only 50% of the infrastructure impacts results in reduced emissions. The GrowOff-Basil (Split Infra) scenario had GHG impacts of roughly 1.94 kg CO2-eq per kg of edible basil. Splitting the infrastructure and employing a mix of hydro and wind power electricity, the GHG emissions were reduced to 1.45 kg CO2-eq per kg of edible basil. As shown in Table 5, this also lowers environmental impacts in all categories, with a reduction of roughly 30% in resource use-minerals and metals, due to less allocation of the infrastructure. Once again for all renewable-based electricity scenarios, nearly all environmental impact categories show reductions, except for water use, due to an increase in hydropower-based electricity.
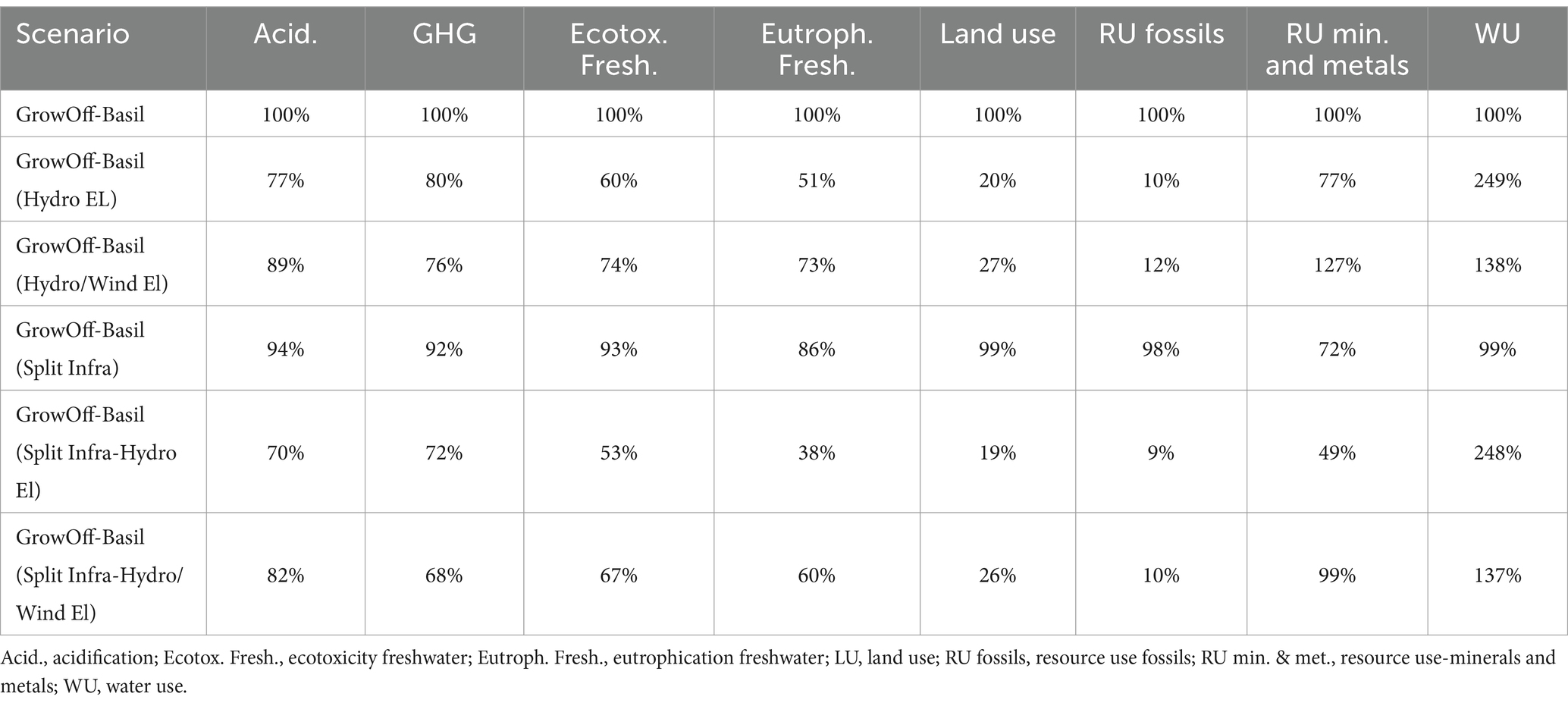
Table 5. Sensitivity to the choice of electricity system employed and allocation of infrastructure impacts on the different environmental impacts for the basil production (shown % change compared to the baseline results).
3.2.2 Comparison to conventional basil sources
Figure 9 provides a comparison of the environmental impacts of basil produced with the GrowOff farm compared to conventional sourcing. For the comparison, the categories featuring the highest and lowest impacts, i.e., the GrowOff-Basil and GrowOff-Basil Split-Infra Hydro/Wind scenarios, were compared with other alternatives available in the literature. The impacts from the GrowOff farm were lower than those on basil produced in Italy, employing data from Agribalyse for open-field production of basil, based on French conditions. Additionally, the impacts from the GrowOff farm were lower compared to basil produced in greenhouses in Sweden, based on data from Martin et al. (2023a) on potted basil from a large greenhouse producer near Stockholm.
3.3 Sensitivity to location and inclusion of infrastructure waste handling
To test the implications of the location of the GrowOff modular vertical farm, different locations were included. These included Gothenburg and Luleå with distances of 500 and 1,000 km, respectively. For each location, logistics for shipping the consumables to the end-user roughly six times per year, in addition to shipping the GrowOff modular vertical farm to these locations, was also included.
As shown in Table 6, there is no significant increase in overall emissions. However, given the logistics required and shipping of the GrowOff farm, increases in the GHG emissions were evident for the process ‘Transportation-In’ for shipping the GrowOff infrastructure and the ‘Transportation-Out’ which includes the palleted consumables. These increases were not large, and the total GHG emissions for lettuce produced in Luleå, Gothenburg, and Stockholm were 1.20, 1.19 and 1.18 kg CO2-eq per kg edible lettuce, respectively.

Table 6. Influence of the distance for shipping consumables for the GrowOff farm, depicting the contribution for the Transportation-in and Transportation-out processes for 1 kg of edible lettuce for the three different locations in Sweden, i.e., Stockholm, Gothenburg, Luleå.
As the waste handling of the infrastructure was not included in the main analysis, as suggested in the methodology, a sensitivity to its inclusion was also analyzed. The baseline scenario GrowOff-Lettuce and the scenario with the impacts of the infrastructure are split, i.e., GrowOff-Lettuce-Split Infra were used to show this sensitivity. As seen in Table 7, including the end-of-life treatment of the infrastructure had only a minor impact on the overall emissions, roughly adding 3.8 and 2.1% to the overall impacts in the GrowOff-Lettuce and GrowOff Lettuce-Split Infra scenarios, respectively.

Table 7. Influence of including EOL for the Growoff-Lettuce scenarios with and without a split of the infrastructure impacts.
4 Discussion
4.1 Environmental performance and sensitivity to data and methodological choices
The results from this study suggest that the GrowOff farm can provide crops with environmental impacts comparable to or lower than conventionally sourced options. For lettuce, the GHG emissions ranged from roughly 0.78–1.18 kg CO2-eq per kg edible lettuce compared to 0.75–2.07 kg CO2-eq per kg edible lettuce for conventional sourcing. Similar findings were seen for the basil, where the GHG emissions ranged from roughly 1.45–2.12 kg CO2-eq per kg basil compared to conventional sourcing with GHG emissions ranging from 2.9–6.2 kg CO2-eq per kg basil. For lettuce, the results were in line with the literature on lettuce production in vertical farms in Sweden (see, e.g., Martin et al., 2023b). For basil, the results demonstrated in the GrowOff farm were lower than average results for a conventional greenhouse producer in Sweden from a previous study (see Martin et al., 2023a). Furthermore, compared to a domestic carbon accounting platform, the emissions are nearly a third lower (CarbonCloud, 2022). This was shown, however, to be sensitive to methodological choices for the environmental life cycle assessment, specifically the allocation of infrastructure and electricity sourcing.
The environmental implications for both the lettuce and basil production were primarily attributable to electricity. In this study, the contribution of the electricity source to the overall GHG emissions ranged from 42 to 62% of the total GHG emissions for the lettuce and 38–58% for the basil. As highlighted in the scenarios, by employing different energy sources it is possible to deduce that the results are highly sensitive to the source of electricity. Choosing a more renewable mix of power, such as wind or hydropower has a significant impact on the overall impacts, despite the large share of renewables in the Swedish electricity mix. These results are in line with findings in recent research by Casey et al. (2022) and Blom et al. (2022) for small vertical farms employing primarily renewable energy. In these studies, employing the regional electricity mix, i.e., from the Netherlands and the U.K., respectively, produced much larger GHG emissions, roughly 8 kg CO2-eq per kg lettuce. Furthermore, for comparisons to open-field, tunnel production, and imported greenhouse products, the overall GHG emissions were largely related to the distribution distance and methods of production. Similar findings have also been highlighted in Martin et al. (2023a).
However, not only the sourcing of the electricity, but the datasets employed for the electricity source can also have an influence on the overall environmental performance. The data employed in this study are from the Ecoinvent database, which may be higher than those figures reported by energy utility companies. For example, in the Environmental Product Declarations (EPDs) from Vattenfall (a regional energy utility company), the GHG emissions for wind power are 15.5 g CO2-eq per kWh and 6.9 g CO2-eq/kWh from hydropower (EPD, 2021, 2022). Corresponding Ecoinvent datasets for wind power range from 4 to 49 g CO2-eq/kWh for hydropower to 14–24 g CO2-eq/kWh for wind. This choice can reduce the impacts, providing evidence to the end-users of the importance of their electricity sourcing for the impacts from the GrowOff system and its produce. Similar claims have been made in prior studies of hydroponic greenhouses (Dias et al., 2017; Graamans et al., 2018) and vertical farms (Martin and Molin, 2019; Weidner et al., 2022). Nonetheless, the sourcing of electricity is not without controversy. End-user firms may have good intentions by purchasing electricity with certificates of origins as identified by Brander et al. (2018), although it is not certain that certificates ensure that electricity is produced from their claimed origins or lead to changes in the electricity mix of a given region. In this study, the default electricity mix was assumed to be Swedish. Martin and Molin (2019) show that the choice of electricity system is sensitive. For example, in a recent study of a hydroponic system in Sweden, the choice of hydropower-based electricity led to large performance improvements over other similar systems due primarily to this choice where the GHG emissions per kWh were significantly lower than those employed in this study (Milestad et al., 2020).
It was illustrated in this study that infrastructure represents a major contributing factor to many of the environmental impact categories. Nonetheless, it is also sensitive to assumptions and methodological considerations employed. For example, in this study, a number of assumptions for the lifetime of different structures and machines can influence the overall impact of the system. Furthermore, the geographical location of the GrowOff farm could be studied in greater detail, as its proximity to potential maintenance providers can also influence its overall environmental impacts (see, e.g., Martin et al., 2021). As there are GrowOff farms situated from the far north of Sweden to the south, the delivery of consumables and maintenance can influence the impacts of the system, especially in countries (such as Sweden) that have large distances between population centers. Although maintenance was not studied, the sensitivity analysis results suggest that the location of the GrowOff farm in Sweden can influence the environmental performance. Once again, to reduce the impacts of the consumables, the provider ships consumables only six times per year with enough seeded trays, fertilizers, and pH regulators required. Finally, a sensitivity to the inclusion of the waste handling of the infrastructure was also included. As highlighted, this inclusion had only a minor influence on the overall impacts, although this can also be sensitive to the lifetime choices as mentioned previously.
In the target country (Sweden) the availability and sourcing of lettuce and basil may vary seasonally. As shown in Martin et al. (2023a), the impacts from, e.g., greenhouse production can also vary significantly during the year, as energy demands for lighting and heating the greenhouse greatly influence the environmental performance of the produce. As such, there can be a variation of the environmental impacts throughout the year for conventionally sourced products. As such, it should also be considered that across the year, local alternatives to the produce from the GrowOff farm may provide lower environmental impacts in specific months. However, this was not studied in this article, although future research could address such concerns, especially if the sourcing of the conventional produce is documented for, e.g., a restaurant or cafeteria.
4.2 Modular GSS solutions and benefits of hyper-localization
Many firms developing GSS systems highlight the environmental sustainability of their offerings, in particular positive connotations relating to resource efficiency and reduced toxicity as a result of both the lack of pesticides and the closed environment. These framings are particularly common among urban agricultural actors and have been found as major drivers for consumer acceptance of high-tech vertical farming solutions (Specht et al., 2014; Coyle and Ellison, 2017; Jürkenbeck et al., 2019). As suggested by Martin and Bustamante (2021), while such information is important for firms highlighting the benefits of modular growing systems, supporting evidence is often lacking. Thus, the results from this study provide concrete empirical evidence of the sustainability of modular vertical farming systems. In doing so, it also contributes to the product-service system literature, which often assumes but rarely quantifies the potential environmental benefits of such systems (Lindahl et al., 2014; Salazar et al., 2015; Bocken et al., 2018).
While the results of the study show advantages in the environmental performance of the GrowOff farm’s produce compared to conventional sourcing, the benefits of on-site production may not be fully captured. Since GSS systems are closed production systems working independently from seasonality and climate, there is potential to have further positive effects on food system issues such as food loss and waste. Although transportation and distribution have been observed as not having a large impact in terms of GHG emission compared to the production stage, cultivating on-site may reduce the transportation distance of conventional supply chains, decreasing the time frame between harvest at the farm and customers purchasing or consumption. The absence of food transportation also means that less food may be wasted because of incorrect storage practices (van Delden et al., 2021). This is particularly valid for fresh plants rich in water content such as lettuce and basil which, for this reason, may benefit from a strategy where production meets local consumption (Kozai and Niu, 2020). This adds nuance to the debate around centralized or distributed food production, where traditionally the belief in industrialized food supply chains is that centralized food processing creates less waste overall than distributed food processing (Parfitt et al., 2010).
The prospect of growing a wider selection of fresh vegetables locally, on-site, and avoiding transportation could offer not just environmental benefits but also improved quality. This includes the possibility of enhanced flavor and nutritional benefits, providing differentiation opportunities from conventionally produced varieties, which often need to be optimized for transportation resistance which may create trade-offs with both taste and nutrition (Bogomolova et al., 2016; Harada and Whitlow, 2020; Renmark, 2021). While GSS producers often tout the freshness and beneficial nutritional aspects of locally produced crops (Martin and Bustamante, 2021), the need for further studies remains. A large body of literature has reviewed the potential effects on quality and nutrition by adjusting process elements such as lighting or nutrients in vertical farms (Selma et al., 2012; Nicole et al., 2019; Sharathkumar et al., 2020). Evidence also suggests that LEDs may positively influence the quality and bioactive compounds composition of crops due to the possibility of fine tuning the light spectrum according to the selected light wavelengths to apply. For instance, several studies have studied the influence of LEDs on specialized metabolites production in different cultivars of basil, with significant increases in bioactive compounds with specific light wavelengths (Bantis et al., 2016; Hosseini et al., 2018; Pennisi et al., 2019, 2020; Lin et al., 2021; Appolloni et al., 2022). Therefore, growing indoors in such modular-distributed vertical farms allows for these systems to potentially enhance the biochemical compositions, health-promoting effect, and taste of fresh produce, although further studies are needed to validate if these modular vertical farms can produce such benefits.
4.3 Limitations and future research
While this study provides empirical evidence based on data provided for the GrowOff farm in the context of Sweden, i.e., Stockholm, the results may not be generalizable to any other location. The results suggest the logistics did not show a large influence on the results. However, depending on the location, the electricity mix may also influence the results although this was not analyzed in this study beyond the sensitivity. For example, in Sweden, there are four electricity markets with varying production capacities (see, e.g., Papageorgiou et al., 2020). Furthermore, comparative assessments were also provided for lettuce and basil production for conventional sourcing. For each GrowOff user, the conventional sourcing may vary, both in the location and seasonality. Once again for Sweden, which imports a large share of its food, comparisons to conventional supplies in other regions may not show the same benefits from such modular cabinet vertical farms.
There are no available studies on similar small scale, i.e., modular cabinet vertical farms, despite their proliferation worldwide (Butturini and Marcelis, 2020; Martin and Bustamante, 2021). As the GrowOff has been significantly modified and optimized from its introduction in 2018, a more longitudinal approach could be employed to study the change in such systems over time. This could provide the companies with evidence of their work with optimization and toward more sustainable and resource-efficient production systems (Martin et al., 2023a).
Additionally, this study focused only on the environmental performance of the GSS systems. Future studies could further explore questions related to business model development and the opportunities and challenges for achieving both economic and sustainability-oriented outcomes. To do so, an additional understanding of the benefits of a cloud-based system and extensive data collection would be essential, along with the interaction and value creation between the provider and users. These aspects could be connected to the PSS literature, which often explores these dynamics, e.g., how the provider maintaining control of the system and maintenance may reduce risks for the user (Tukker and Tischner, 2006; Lingegård, 2020). Further exploring the context and the potential of this to influence the sustainability of PSS systems is also important as it is currently lacking (Martin et al., 2021). However, as these systems also rely on new business models for the end-users, social and economic sustainability should also be explored. Therefore, to better understand the potential impacts, more research could focus on the implications of such growing service systems from a three-pillar sustainability perspective.
5 Conclusion
In this study, a life cycle assessment was performed for a cabinet-based vertical farm located in Stockholm, Sweden, producing either lettuce or basil. From the life cycle assessment, it was found that the modular vertical farm produced lettuce with GHG emissions of roughly 0.78–1.18 kg CO2-eq kg−1 lettuce. The lower value was associated with the impacts of the infrastructure being partially allocated to the system as it is rented and with a more renewable energy mix, i.e., a mix of hydro and wind power electricity. The results also suggest that the vertical farm can produce basil with GHG emissions of roughly 1.45–2.12 kg CO2-eq per kg basil. Once again, the lower values are associated with renewable electricity sourcing and only partial allocation of the infrastructure impacts.
The largest share of all environmental impacts stems from the electricity use for the cabinet farm in nearly all impact categories. Additionally, the cabinet infrastructure, transportation involved for consumables, waste handling, and the growing media were also of importance. Comparisons were also made to conventionally sourced lettuce and basil. The results indicate that the cabinet vertical farm produced lettuce and basil with lower GHG emissions. As highlighted, methodological choices affected the results and comparisons. This primarily includes electricity sourcing, data, cabinet infrastructure allocation, and LCI data for comparisons to conventional lettuce and basil sourcing. Furthermore, while this study analyzes a cabinet-based vertical farm, which is a prevalent form of modular vertical farm worldwide, the context and region of vertical farm assessments may vary. As such, the findings of this study cannot be applied to other regions and may be specific to the Swedish context due primarily to the large share of renewable energy in the electricity mix.
Results and knowledge from this study provide insights into the environmental implications of vertical farming and add to the developing literature on the sustainability of controlled environment agriculture by studying the environmental performance of a commercial cabinet-based vertical farm from a Swedish context. It also provides empirical evidence on the sustainability and potential of product-service systems business models for vertical farming and retail stakeholders. While the research provides empirical evidence for one vertical farm, further evidence is needed in this novel and expanding field, especially for modular vertical farms, which are prevalent throughout the world. Finally, it is suggested that future research should focus on the viability, optimization, and improvement possibilities for the GrowOff farms, and the quality of products produced in such systems compared to conventionally sourced alternatives.
Data availability statement
The raw data supporting the conclusions of this article will be made available by the authors, without undue reservation.
Author contributions
MM: Writing – review & editing, Writing – original draft, Visualization, Validation, Supervision, Software, Resources, Project administration, Methodology, Investigation, Funding acquisition, Formal analysis, Data curation, Conceptualization. MB: Writing – review & editing, Writing – original draft, Validation, Methodology, Formal analysis, Conceptualization. IZ: Validation, Software, Project administration, Methodology, Investigation, Writing – review & editing, Writing – original draft. FO: Writing – review & editing, Writing – original draft, Validation, Methodology, Investigation, Formal analysis, Data curation.
Funding
The author(s) declare that financial support was received for the research, authorship, and/or publication of this article. The research leading to this publication has also received funding from the Italian Ministry of Education and Research (MUR), within the call for Research Projects of National Interest (PRIN), within the project “VFARM - Sustainable Vertical Farming” (Project code: 2020ELWM82, CUP:J33C20002350001).
Acknowledgments
We would like to express our gratitude to Natalie, Robin and Jeremie at Grönska Stadsodling for their cooperation and access to data for completing this article.
Conflict of interest
The authors declare that the research was conducted in the absence of any commercial or financial relationships that could be construed as a potential conflict of interest.
The author(s) declared that they were an editorial board member of Frontiers, at the time of submission. This had no impact on the peer review process and the final decision.
Publisher’s note
All claims expressed in this article are solely those of the authors and do not necessarily represent those of their affiliated organizations, or those of the publisher, the editors and the reviewers. Any product that may be evaluated in this article, or claim that may be made by its manufacturer, is not guaranteed or endorsed by the publisher.
Supplementary material
The Supplementary material for this article can be found online at: https://www.frontiersin.org/articles/10.3389/fsufs.2024.1403580/full#supplementary-material
References
Appolloni, E., Pennisi, G., Zauli, I., Carotti, L., Paucek, I., Quaini, S., et al. (2022). Beyond vegetables: effects of indoor LED light on specialized metabolite biosynthesis in medicinal and aromatic plants, edible flowers, and microgreens. J. Sci. Food Agric. 102, 472–487. doi: 10.1002/jsfa.11513
BAMA (2023). Personal communication with BAMA Nordic, subject: supply country for mixed salad bags. Helsingborg, Sweden.
Bantis, F., Ouzounis, T., and Radoglou, K. (2016). Artificial LED lighting enhances growth characteristics and total phenolic content of Ocimum basilicum, but variably affects transplant success. Sci. Hortic. 198, 277–283. doi: 10.1016/j.scienta.2015.11.014
Bartzas, G., Zaharaki, D., and Komnitsas, K. (2015). Life cycle assessment of open field and greenhouse cultivation of lettuce and barley. Inf. Process Agric. 2, 191–207. doi: 10.1016/j.inpa.2015.10.001
Benke, K., and Tomkins, B. (2017). Future food-production systems: vertical farming and controlled-environment agriculture. Sustain.: Sci. Pract. Policy 13, 13–26. doi: 10.1080/15487733.2017.1394054
Blom, T., Jenkins, A., Pulselli, R. M., and van den Dobbelsteen, A. A. J. F. (2022). The embodied carbon emissions of lettuce production in vertical farming, greenhouse horticulture, and open-field farming in the Netherlands. J. Clean. Prod. 377:134443. doi: 10.1016/j.jclepro.2022.134443
Bocken, N. M., Schuit, C. S., and Kraaijenhagen, C. (2018). Experimenting with a circular business model: lessons from eight cases. Environ. Innov. Soc. Trans. 28, 79–95. doi: 10.1016/j.eist.2018.02.001
Bogomolova, S., Loch, A., Lockshin, L., and Buckley, J. (2016). Consumer factors associated with purchasing local versus global value chain foods. Renew. Agric. Food Syst. 33, 33–46. doi: 10.1017/s1742170516000375
Brander, M., Gillenwater, M., and Ascui, F. (2018). Creative accounting: a critical perspective on the market-based method for reporting purchased electricity (scope 2) emissions. Energ Policy 112, 29–33. doi: 10.1016/j.enpol.2017.09.051
Bustamante, M. J. (2020). Using sustainability-oriented process innovation to shape product markets. Int. J. Innov. Manag. 24:2040001. doi: 10.1142/S1363919620400010
Butturini, M., and Marcelis, L. F. M. (2020). “Chapter 4 – vertical farming in Europe: present status and outlook” in plant factory. eds. T. Kozai, G. Niu, and M. Takagaki. 2nd ed (Academic Press), 77–91.
CarbonCloud (2022). Climate hub: herbs in pot, fresh, greenhouse grown (Sweden). Available at: https://apps.carboncloud.com/climatehub/product-reports/id/156551543712 (accessed December 03, 2023).
Casey, L., Freeman, B., Francis, K., Brychkova, G., McKeown, P., Spillane, C., et al. (2022). Comparative environmental footprints of lettuce supplied by hydroponic controlled-environment agriculture and field-based supply chains. J. Clean. Prod. 369:133214. doi: 10.1016/j.jclepro.2022.133214
Coyle, B. D., and Ellison, B. (2017). Will consumers find vertically farmed produce “out of reach?”. Choices 32, 1–8. doi: 10.22004/ag.econ.253382
De Pascale, S., Rouphael, Y., Gallardo, M., and Thompson, R. B. (2018). Water and fertilization management of vegetables: state of art and future challenges. Eur. J. Hortic. Sci. 83, 306–318. doi: 10.17660/eJHS.2018/83.5.4
Despommier, D. (2011). The vertical farm: controlled environment agriculture carried out in tall buildings would create greater food safety and security for large urban populations. J. Verbr. Lebensm. 6, 233–236. doi: 10.1007/s00003-010-0654-3
Dias, G. M., Ayer, N. W., Khosla, S., Van Acker, R., Young, S. B., Whitney, S., et al. (2017). Life cycle perspectives on the sustainability of Ontario greenhouse tomato production: benchmarking and improvement opportunities. J. Clean. Prod. 140, 831–839. doi: 10.1016/j.jclepro.2016.06.039
Dorr, E., Goldstein, B., Horvath, A., Aubry, C., and Gabrielle, B. (2021). Environmental impacts and resource use of urban agriculture: a systematic review and meta-analysis. Environ. Res. Lett. 16:093002. doi: 10.1088/1748-9326/ac1a39
Drottberger, A., and Langendahl, P. A. (2023). Farming-as-a-service initiative in the making: insights from emerging proto-practices in Sweden. Smart Agric. Technol. 6:100368. doi: 10.1016/j.atech.2023.100368
EPD (2021) Electricity from Vattenfall’s Nordic hydropower. Environmental product declaration (EPD) S-P-00088. Stockholm, Sweden.
EPD (2022) Electricity from Vattenfall’s wind farms. Environmental product declaration (EPD) S-P-01435. Stockholm, Sweden.
Fusi, A., Castellani, V., Bacenetti, J., Cocetta, G., Fiala, M., and Guidetti, R. (2016). The environmental impact of the production of fresh cut salad: a case study in Italy. Int. J. Life Cyc. Asses. 21, 162–175. doi: 10.1007/s11367-015-1019-z
Geissdoerfer, M., Vladimirova, D., and Evans, S. (2018). Sustainable business model innovation: a review. J. Clean. Prod. 198, 401–416. doi: 10.1016/j.jclepro.2018.06.240
Graamans, L., Baeza, E., van den Dobbelsteen, A., Tsafaras, I., and Stanghellini, C. (2018). Plant factories versus greenhouses: comparison of resource use efficiency. Agric. Syst. 160, 31–43. doi: 10.1016/j.agsy.2017.11.003
Harada, Y., and Whitlow, T. H. (2020). Urban rooftop agriculture: challenges to science and practice. Front. Sustain. Food Syst. 4:00076. doi: 10.3389/fsufs.2020.00076
Hosseini, A., Zare Mehrjerdi, M., and Aliniaeifard, S. (2018). Alteration of bioactive compounds in two varieties of basil (Ocimum basilicum) grown under different light spectra. J. Essent. Oil-Bear. Plants 21, 913–923. doi: 10.1080/0972060X.2018.1526126
InFarm (2021). InFarm Homepage. Available at: www.infarm.com (accessed August 10, 2021)
Joint Research Centre, Institute for Environment and Sustainability (2010). “International reference life cycle data system (ILCD) handbook” in General guide for life cycle assessment – detailed guidance (Ispra, Italy: Publications Office of the European Union).
Jürkenbeck, K., Heumann, A., and Spiller, A. (2019). Sustainability matters: consumer acceptance of different vertical farming systems. Sustain. For. 11:4052. doi: 10.3390/su11154052
Kozai, T., and Niu, G. (2020). “Role of the plant factory with artificial lighting (PFAL) in urban areas” in Plant factory. Eds. Kozai, T., Niu, Takagaki, M. (London, England: Academic Press), 7–34.
Lin, K. H., Huang, M. Y., and Hsu, M. H. (2021). Morphological and physiological response in green and purple basil plants (Ocimum basilicum) under different proportions of red, green, and blue LED lightings. Sci. Hortic. 275:109677. doi: 10.1016/j.scienta.2020.109677
Lindahl, M., Sundin, E., and Sakao, T. (2014). Environmental and economic benefits of integrated product service offerings quantified with real business cases. J. Clean. Prod. 64, 288–296. doi: 10.1016/j.jclepro.2013.07.047
Lingegård, S. (2020). Product service systems: business models toward a circular economy, in Handbook of the Circular Economy, eds M. Brandao, D. Lazarevic, and G. Finnveden (Cheltenham: Edward Elgar Publishing), 61–73. doi: 10.4337/9781788972727.00013
Martin, M., Bengtsson, E., Carotti, L., Orrestig, K., and Orsini, F. (2023a). Environmental assessment of greenhouse herb production: a case of longitudinal improvement options in Sweden. Resour. Conserv. Recycl. 193:106948. doi: 10.1016/j.resconrec.2023.106948
Martin, M., and Brandão, M. (2017). Evaluating the environmental consequences of Swedish food consumption and dietary choices. Sustain. For. 9:2227. doi: 10.3390/su9122227
Martin, M., and Bustamante, M. J. (2021). Growing-service systems: new business models for modular urban-vertical farming. Front. Sustain. Food Syst. 5:787281. doi: 10.3389/fsufs.2021.787281
Martin, M., and Bustamante, M. J. (2022). Creating expectations and controversies for urban-vertical farming. Acta Hortic. 1356, 17–24. doi: 10.17660/ActaHortic.2022.1356.3
Martin, M., Elnour, M., and Siñol, A. C. (2023b). Environmental life cycle assessment of a large-scale commercial vertical farm. Sustain. Prod. Consum. 40, 182–193. doi: 10.1016/j.spc.2023.06.020
Martin, M., Heiska, M., and Björklund, A. (2021). Environmental assessment of a product-service system for renting electric-powered tools. J. Clean. Prod. 281:125245. doi: 10.1016/j.jclepro.2020.125245
Martin, M., and Molin, E. (2019). Environmental assessment of an urban vertical hydroponic farming system in Sweden. Sustainability 11:4124. doi: 10.3390/su11154124
Martin, M., and Orsini, F. (2023). “Life cycle assessment of indoor vertical farms” in Advances in plant factories: new technologies in indoor vertical farming. eds. T. Kozai and E. Hayashi (Cambridge, England: Burleigh and Dodds).
Martin, M., Orsini, F., Appolloni, E., Rufí-Salís, M., Tonini, P., Pennisi, G., et al. (2023c). “State-of-the-art on the environmental footprint of urban farming” in Commercial urban agriculture: current status and future perspectives. eds. J. Monzini and F. Orsini (Italy: Food and Agricultural Organisation Rome).
Martin, M., Weidner, T., and Gullström, C. (2022). Estimating the potential of building integration and regional synergies to improve the environmental performance of urban vertical farming. Front. Sustain. Food Syst. 6:849304. doi: 10.3389/fsufs.2022.849304
Milestad, R., Carlsson-Kanyama, A., and Schaffer, C. (2020). The högdalen urban farm: a real case assessment of sustainability attributes. Food Secur. 12, 1461–1475. doi: 10.1007/s12571-020-01045-8
Mont, O., Neuvonen, A., and Lähteenoja, S. (2014). Sustainable lifestyles 2050: stakeholder visions, emerging practices and future research. J. Clean. Prod. 63, 24–32. doi: 10.1016/j.jclepro.2013.09.007
Nicole, C. C. S., Mooren, J., Pereira Terra, A. T., Larsen, D. H., Woltering, E. J., Marcelis, L. F. M., et al. (2019). Effects of LED lighting recipes on postharvest quality of leafy vegetables grown in a vertical farm. Acta Hortic. 1256, 481–488. doi: 10.17660/ActaHortic.2019.1256.68
O’Sullivan, C. A., McIntyre, C. L., Dry, I. B., Hani, S. M., Hochman, Z., and Bonnett, G. D. (2020). Vertical farms bear fruit. Nat. Biotechnol. 38, 160–162. doi: 10.1038/s41587-019-0400-z
Orsini, F., Pennisi, G., Zulfiqar, F., and Gianquinto, G. (2020). Sustainable use of resources in plant factories with artificial lighting (PFALs). Eur.J.Hortic.Sci. 85, 297–309. doi: 10.17660/eJHS.2020/85.5.1
Papageorgiou, A., Ashok, A., Hashemi Farzad, T., and Sundberg, C. (2020). Climate change impact of integrating a solar microgrid system into the Swedish electricity grid. Appl. Energ. 268:114981. doi: 10.1016/j.apenergy.2020.114981
Parfitt, J., Barthel, M., and Macnaughton, S. (2010). Food waste within food supply chains: quantification and potential for change to 2050. Philos. Trans. R. Soc. B: Biol. Sci. 365, 3065–3081. doi: 10.1098/rstb.2010.0126
Pennisi, G., Blasioli, S., Cellini, A., Maia, L., Crepaldi, A., Braschi, I., et al. (2019). Unraveling the role of red:blue LED lights on resource use efficiency and nutritional properties of indoor grown sweet basil. Front. Plant Sci. 10:305. doi: 10.3389/fpls.2019.00305
Pennisi, G., Pistillo, A., Orsini, F., Cellini, A., Spinelli, F., Nicola, S., et al. (2020). Optimal light intensity for sustainable water and energy use in indoor cultivation of lettuce and basil under red and blue LEDs. Sci. Hortic. 272:109508. doi: 10.1016/j.scienta.2020.109508
Pulighe, G., and Lupia, F. (2020). Food first: COVID-19 outbreak and cities lockdown a booster for a wider vision on urban agriculture. Sustain. For. 12:5012. doi: 10.3390/su12125012
Renmark, A. (2021). Hajpad trend växer – men är den hållbar? Dagligvarunytt, 7 May 2021, Nr. 5. Available at: https://www.dagligvarunytt.se/marknadsnytt/innovation/hajpad-odlingstrend-vaxer-i-butiken-som-bake-off-for-kryddgront/ (accessed November 03, 2022).
Salazar, C., Lelah, A., and Brissaud, D. (2015). Eco-designing product service systems by degrading functions while maintaining user satisfaction. J. Clean. Prod. 87, 452–462. doi: 10.1016/j.jclepro.2014.10.031
Selma, M. V., Luna, M. C., Martínez-Sánchez, A., Tudela, J. A., Beltrán, D., Baixauli, C., et al. (2012). Sensory quality, bioactive constituents and microbiological quality of green and red fresh-cut lettuces (Lactuca sativa L.) are influenced by soil and soilless agricultural production systems. Postharv. Bio. Techn. 63, 16–24. doi: 10.1016/j.postharvbio.2011.08.002
SEPA (2015). Swedish environmental objectives. Stockholm, Sweden: Swedish Environmental Protection Agency (SEPA).
Sharathkumar, M., Heuvelink, E., and Marcelis, L. F. M. (2020). Vertical farming: moving from genetic to environmental modification. Trends Plant Sci. 25, 724–727. doi: 10.1016/j.tplants.2020.05.012
Specht, K., Siebert, R., Opitz, I., Freisinger, U., Sawicka, M., Werner, A., et al. (2014). Urban agriculture of the future: an overview of sustainability aspects of food production in and on buildings. Agric. Hum. Values 31, 33–51. doi: 10.1007/s10460-013-9448-4
Swedish Energy Agency [SEA] . Energy in Sweden 2020: an overview. (2021). Available at: https://energimyndigheten.a-w2m.se/System/TemplateView.aspx?p=Arkitektkopia&id=fa46fcb27d264766b0bf84982a07fed6&q=2020:1&lstqty=1 (accessed February 12, 2022).
Thomaier, S., Specht, K., Henckel, D., Dierich, A., Siebert, R., Freisinger, U. B., et al. (2014). Farming in and on urban buildings: present practice and specific novelties of zero-acreage farming (ZFarming). Renew. Agric. Food Syst. 30, 43–54. doi: 10.1017/S1742170514000143
Tukker, A. (2004). Eight types of product–service system: eight ways to sustainability? Experiences from SusProNet. Bus. Strat. Env. 13, 246–260. doi: 10.1002/bse.414
Tukker, A., and Tischner, U. (2006). Product-services as a research field: past, present and future. Reflections from a decade of research. J. Clean. Prod. 14, 1552–1556. doi: 10.1016/j.jclepro.2006.01.022
van Delden, S. H., SharathKumar, M., Butturini, M., Graamans, L. J. A., Heuvelink, E., Kacira, M., et al. (2021). Current status and future challenges in implementing and upscaling vertical farming systems. Nat. Food 2, 944–956. doi: 10.1038/s43016-021-00402-w
Keywords: vertical farm, life cycle assessment (LCA), sustainability, controlled environment agriculture (CEA), product service system
Citation: Martin M, Bustamante MJ, Zauli I and Orsini F (2024) Environmental life cycle assessment of an on-site modular cabinet vertical farm. Front. Sustain. Food Syst. 8:1403580. doi: 10.3389/fsufs.2024.1403580
Edited by:
Jagadeesh Yeluripati, The James Hutton Institute, United KingdomReviewed by:
Frances Sandison, The James Hutton Institute, United KingdomToyoki Kozai, Japan Plant Factory Association, Japan
Copyright © 2024 Martin, Bustamante, Zauli and Orsini. This is an open-access article distributed under the terms of the Creative Commons Attribution License (CC BY). The use, distribution or reproduction in other forums is permitted, provided the original author(s) and the copyright owner(s) are credited and that the original publication in this journal is cited, in accordance with accepted academic practice. No use, distribution or reproduction is permitted which does not comply with these terms.
*Correspondence: Michael Martin, bWljaGFlbC5tYXJ0aW5AaXZsLnNl