- 1Department of Agronomy, Faculty of Agriculture, Universitas Gadjah Mada, Yogyakarta, Indonesia
- 2Department of Soil Science, Faculty of Agriculture, Universitas Gadjah Mada, Yogyakarta, Indonesia
- 3Agrotechnology Innovation Centre, Universitas Gadjah Mada, Yogyakarta, Indonesia
Introduction: Addressing the global demand for rice production necessitates innovative approaches to enhance upland rice yield in rainfed agroecosystems, considering the challenges posed by increasing population, limited land fertility, low productivity, and water availability.
Methods: In this study, our study investigated the impact of biochar and organic fertilizer on ten promising rice lines (G1 – G10) and two control (G11 – G12) cultivars under rainfed conditions. The experimental design used a split-plot design with four soil amendments as main plots, namely control, organic fertilizer, biochar, and biochar + organic fertilizer and 12 rice genotypes as subplot.
Results: The absolute attainable yield gaps, differentiating organic and control (GAP1), biochar + organic and control (GAP2), and biochar and control (GAP3), ranged from 1.5 to 3.7 or increased of 91–580%, 0.8 to 3.5 (72–560%), and 0.6 to 2.58 tons/ha (58–472%), respectively. Notably, G2 + organic exhibited the highest positive absolute yield gap, ranging from 1.1 to 5.38 tons/ha, based on the yield gap matrix. Furthermore, genotype main effect plus genotype-environment interaction (GGE) biplot analysis identified G2 as the most promising rice line, displaying superior yield performance for cultivation in biochar and organic amended soils.
Discussion: These findings provide valuable insights for farmers, governments, and stakeholders, offering a roadmap to optimize rainfed areas for rice production, serving as practical guidance to enhance overall rice productivity in rainfed agroecosystems.
1 Introduction
Meeting the anticipated food demand resulting from Indonesia’s population growth, which is expected to exceed 120% by 2050 (Alexandratos and Bruinsma, 2012; Rozi et al., 2023), rice production must be significantly increased. Being the primary food for nearly 80% of the population, with an average consumption of 1.6 kg per capita per week (Sitaresmi et al., 2023). the nation faces a potential threat to food security due to an imbalance between consumption and production growth. Despite such high consumption levels, rice production has witnessed a decline from 59.2 million tons in 2018 to 54.7 million tons in 2022 (Statistics Indonesia, 2018; Khasanah and Astuti, 2022). Various factors, including genotypes, water availability, soil fertility, and farmers’ skills, and climate change contribute to this production decline (Chen et al., 2008; Ansari et al., 2023). Addressing this gap requires identifying optimal agricultural practices for rice cultivation, focusing on field management and genotypes to enhance rice yield (Senguttuvel et al., 2021).
Fostering the development and optimization of rice cultivation in Indonesia’s rainfed agroecosystem holds promise as a solution to increase yield amidst various pedologic, climatic, and hydrologic challenges. While rainfed areas constitute 30% of the total agricultural land in Indonesia, the average rice yield in these regions is approximately 3.7 tons/ha lower than that in paddy fields, which typically yield 5 tons/ha (Statistics Indonesia, 2021). Rainfed areas encompass lands outside the irrigated zone solely reliant on rainfall for irrigation (Devendra, 2012). A critical constraint in rainfed agriculture is the availability and sustainability of water, significantly impacting crop growth and yield (Rockström et al., 2010). Globally, rainfed crops exhibit a yield reduction of around 50% compared to irrigated conditions (Jaramillo et al., 2020). Additionally, research conducted in rainfed areas in various countries has documented yield decreases of 6 t/ha in China (Terjung et al., 1985), 0.7 tons/ha in Thailand (Sacklokham et al., 2020), and 0.5 to 4.3 tons/ha in India (Kumar et al., 2021). Addressing these challenges is imperative for sustainable and enhanced paddy production in rainfed agroecosystems.
To address the numerous challenges faced by rainfed areas, the application of soil amendments, specifically through biochar and organic fertilizer, emerges as a viable strategy to optimize rice growth while maintaining soil water availability (Głąb et al., 2020; Ansari et al., 2023). Biochar, primarily derived from the pyrolysis process involving the combustion of biomass or organic material under limited oxygen conditions and low temperatures (≤700°C), plays a crucial role in enhancing soil water retention and reduce nitrous oxide emission (Mukhtar et al., 2023; Rassaei, 2023, 2024). Physically, biochar is highly porous, thus its application to soil is considered to improve a range of soil physical and chemical properties including soil moisture content, plant available water content (PAWC) (Hardie et al., 2014), water retention capacity and nutritional status of rhizosphere (nitrogen, phosphorous, and potassium) (Ghassemi-Golezani et al., 2023). Moreover, this is achieved by reducing soil bulk density (Abel et al., 2013; Da Silva Mendes et al., 2021), increasing soil pore volume (Obia et al., 2016; An et al., 2022), and promoting soil aggregation (Herath et al., 2013; Islam et al., 2021). Specifically, rice husk biochar (RHB) has a great quantity of macropores (75–100 μm) and its application to soil enhances the addition of soil pore sized 6 to 45 μm (Lu et al., 2014). With the more water sufficiency, it can avoid suppressing leaf expansion and stomatal conductance thereby leads to maximize photosynthetic rate (Tardieu et al., 2014). Biologically, large amount of porosity and surface properties of biochar provides a suitable environment for soil microbial growth and reproduction, protecting beneficial soil microorganisms (Warnock et al., 2010). Some of the microorganisms inluenced by amendments of biochar including nitrogen-fixing bacteria (Kim et al., 2007), gram-positive bacteria, and actinomycetes (Purakayastha et al., 2019), arbuscular mycorrhizal colonization (Solaiman et al., 2010). Chemically, biochar addition can increase the soil organic matter content (Zygourakis, 2017) through promoting polymerization of small organic molecules through surface catalytic activity (Liang et al., 2010). In addition, biochar also increased the availability and reduced the leaching of nitrogen in the soil (Güereña et al., 2013), absorbing NH3 to reduce nitrogen loss and improve utilization of nitrogen (Taghizadeh-Toosi et al., 2012). Meanwhile, Cation exchange capacity (CEC) also increased along with the addition of biochar. Soil with a high CEC is easy to adsorb NH4+, K+, Ca2+, and Mg2+, which can effectively improve the usage of nutrient ions and reduce the leaching of nutrients (Zhang et al., 2021). Structurally, the acidic aromatic carbon on the surface of biochar is oxidized to form abundant functional groups (–OH, –COOH), enhancing the adsorption capacity of cations and increasing CEC (Atkinson et al., 2010). In rice cultivation, the application of rice husk biochar (RHB) influences both vegetative and generative aspects, enhancing tiller number, root dry weight (Sang et al., 2018), panicle count, grain yield (Barus, 2016), 1,000-grain weight, and filled grain (Mishra et al., 2017). In addition to biochar, organic fertilizer proves beneficial in augmenting soil fertility and crop productivity. Biologically, organic fertilizer fosters increased soil microbial activity, as evidenced by elevated urease and sucrase activity, along with an enhanced soil respiration rate (LI et al., 2018). This is further reflected in higher soil microbial biomass carbon, soil microbial biomass nitrogen, and soil enzyme activity (Ren et al., 2019). From a chemical soil perspective, organic fertilizer stabilizes organic matter (Houot et al., 2009; Chen et al., 2022), augments nutrient levels, thereby promoting plant growth and yield (Zraibi et al., 2015; Ye et al., 2020). Regarding soil water retention, organic fertilizer indirectly influences an increase in soil water content by enhancing porosity and pore distribution (Lal, 2020). Incorporating these soil amendments presents a comprehensive approach to mitigate constraints in rainfed areas and optimize rice cultivation.
The yield gap analysis serves a functional role in quantifying the disparity between the average agricultural and potential crop yield under optimal conditions, considering factors such as sufficient water and nutrition, or the yield achievable through economic practices (EY) with optimal management (Evans and Fischer, 1999). This analysis is a powerful method not only identifies factors limiting current farm yields but also forms the basis for recommending improved agricultural practices to close the gap (Van Ittersum et al., 2013). Besides yields gap analysis (YGA), GEI (genotype-by environment interaction) is an important issue in crop breeding and production (Kang, 2004). Cultivar evaluation and mega-environment identification are among the most important objectives of multi-environment trials (Yan et al., 2000). A GGE-biplot graph can describe visual information related on the evaluation of genotype, environment, and their interactions and it has been widely used in various crops (Yan et al., 2007). The objectives of this research are twofold: (i) to investige, quantify, and evaluate the impact of biochar and organic fertilizer on rice yield under rainfed conditions and attainable yield (Yatt), actual yield (Ya), and yield gap (Yg) of different paddy genotypes with various soil amendments; (ii) to select suitable genotypes in each soil amendment trial. Notably, there is a gap in the literature regarding yield gap analysis and GGE biplot application in paddy cultivation, specifically in rainfed areas of Indonesia. Firstly, Indonesia faces significant challenges in rice production due to its reliance on rainfed agriculture, which is highly susceptible to climate variability and other environmental factors. Through applying advanced analytical techniques such as yield gap analysis and GGE biplot, this study has the potential to provide valuable insights into understanding the productivity constraints and identifying opportunities for improving rice yield under rainfed conditions. Furthermore, addressing this research gap is important for informing evidence-based decision-making by policymakers, agricultural practitioners, and other stakeholders involved in rice cultivation in Indonesia. The findings of this study could offer practical recommendations for optimizing resource use, enhancing crop management practices, and mitigating yield gaps in rainfed paddy cultivation. This study can inform the recommendation of precise agronomic management practices involving different soil amendments and genotypes to benefit farmers, researchers, and other stakeholders.
2 Materials and methods
2.1 Study site
The experiment was conducted in Playen District, Gunung Kidul Regency, The Special Region of Yogyakarta (75o6’30” S to 75o9’0”S and 110o28’30″ E to 110o32’0″ E) (Figure 1), spanning from November 2021 to April 2022 (refer to Table S2 in Supplementary material). Geographically, the area exhibited an average air temperature of 25.54°C and a relative humidity (RH) of 83.90%. The soil type identified was Lithic Haplusterts, classified as vertisol according to USDA standards (Alam et al., 2020). Physically, the soil texture in the field was predominantly clay with markedly slow drainage (0.001 cm hour−1). The soil possessed a water-holding capacity (WHC) of 40.36% and a total porosity of 38.64%. Chemically, the soil exhibited a cation exchange capacity (CEC) of 60.22 cmol (+) kg−1 (extremely high), a soil pH (H₂O) of 8.4 (alkaline), and a soil organic carbon (SOC) content of 1.80 (low). Additionally, total nitrogen (TN) content was 0.09% (extremely low), phosphorus availability (P) was 14 ppm (medium), and potassium availability (K) was 0.24 cmol (+) kg−1 (low). The soil also contained high levels of available of calcium (Ca) at 24.52 cmol (+) kg−1, magnesium (Mg) at 2.23 cmol (+) kg−1, and sodium (Na) at 0.85 cmol (+) kg−1 (Suryanto et al., 2022). Historically, in the experimental site, the previous crops were maize (Zea mays) cultivated from April to June and was fallowed until September because of low rainfall. Meanwhile, the rice cultivation is started in October to March because it coincides with the start of the rainy season.
2.2 Design of experiments and treatment application
In this research, soil amendment involved the use of locally harvested RHB, and organic fertilizer derived from milk sewage. The rationale behind choosing these specific soil amendments lies in their unique properties and their potential to address specific soil fertility constraints and improve crop productivity in rainfed paddy cultivation (Rassaei, 2022). Rice husk biochar is known for its ability to improve soil structure, enhance nutrient retention, and promote microbial activity, thereby increasing soil fertility and supporting healthier plant growth. On the other hand, organic fertilizer derived from milk sewage provides essential nutrients to the soil, improves soil organic matter content, and enhances soil microbial diversity, all of which contribute to improved soil fertility and crop yield. The RHB was produced through the kiln method (Kong and Sii, 2020), employing modified iron plates equipped with chimneys and shutters. Laboratory analysis revealed the chemical composition of the rice husk biochar, indicating a pH (H₂O) of 8.02, carbon (C) content of 34.60%, hydrogen (H) content of 4.23%, nitrogen (N) content of 0.47%, and oxygen (O) content of 31.70% (Kastono et al., 2022). On the other hand, the organic fertilizer was sourced from milk sewage generated by the Agrotechnology Innovation Centre at Universitas Gadjah Mada. Laboratory analysis of the milk sewage organic fertilizer indicated the presence of 44.90% organic carbon (C-organic), 5.86% total nitrogen (N), 9.96% phosphorus pentoxide (P₂O₅), and 0.17% potassium oxide (K₂O) (Taryono, 2022). The experimental design used a split-plot (Figure 2) with three replications and the randomization used R studio software package agricolae (de Mendiburu, 2021). The main factor was organic soil amendment consisted of control (without organic matter and soil amendment), organic fertilizer (compost sourced from milk sewage) with a dose of 20 tons/ha, RHB (20 tons/ha), and a combination of organic fertilizer (10 tons/ha) and biochar (10 tons/ha). The subplot was 12 genotypes of rice consisted of 10 promising rice lines sourced from Universitas Gadjah Mada, Indonesia (G1 - G10) and 2 rice cultivars as control sourced from the Indonesian Center for Rice Research (ICRR), West Java, Indonesia (G11 and G12). The split-plot design was chosen for its ability to efficiently address the complex interaction between the main treatment factors (such as soil amendments) and the subplot treatment factors (such as different rice varieties or planting densities) (Kowalski and Potcner, 2003). The land preparation was started 1 week before sowing the seed by agitating the soil surface using hoe and then applied biochar and organic fertilizer to soil in each plot with designated dosages based on the experimental design. Meanwhile, the seeds were soaked in the water 12 h before planting and were sown directly into the field with distance of 20 × 20 cm. Additionally, chemical fertilizers were utilized in accordance with local farming practices, employing varying dosages and products. Specifically, urea, SP36, ZA, and KCL were applied at rates of 300 kg/ha, 150 kg/ha, 100 kg/ha, and 150 kg/ha, respectively and it applied 3 times, namely 14 days after planting (Urea, ZA, SP36, KCl), 30 days after planting (Urea) and 60 days after planting (Urea, ZA, SP-36, and KCL). Pesticides were employed to mitigate pest and fungal infestations, including Plenum (containing pimetrozin as the active ingredient) for controlling Nilaparvata lugens, Prevathon (with klorantraniliprol as the active ingredient) for caterpillar control, Nordox to manage blast (Pyricularia orizae), and Bacterial Leaf Blight (Xanthomonas oryzae). Furthermore, irrigation in the field relied entirely on rainfall. Consequently, the planting schedule was synchronized with the onset of the wet season, commencing in November 2021. Moreover, monthly precipitations in Gunung Kidul from October 2021 to April 2022 were 145.7 mm, 344.8 mm, 409.9 mm, 199 mm, 188 mm, 403 mm, and 243 mm, respectively (Statistics Indonesia, 2023).
2.3 Data collection
For the calculation of yield components, rice yield observations were conducted using a digital scale during harvesting after the grains had been dried to a moisture content of 14%. The rice grain were weighed using a ACIS digital scale and grain moisture meter LDS-1G was used to measure the moisture content, following the methodology outlined by the International Rice Research Institute (IRRI, 2015). Statistically, the grain yield was measured by selecting harvested crops from a plot area of approximately 5 m2 per plot. The obtained values were then converted into yield per hectare (ha) according to the standards outlined by IRRI (Gomez, 1972).
2.4 Statistical analysis
The data was required to be normally distributed with homogeneity variance assumptions. The normal distribution had a Q-Q plot and homogeneous variance with a residual vs. value graph (Welham et al., 1990). Comparisons of response variable was conducted using ANOVA (p < 0.05) and followed by the Scott-Knott test (p < 0.05) (Scott and Knott, 1974). ANOVA checks the impact of one or more factors by comparing the means of different samples and Scott-Knott test is statistical post-hoc analysis of grouping means, which distinguishes results without ambiguity (Bhering et al., 2008). The interaction between rice genotypes with organic matter and soil amendement was visualized using the GGE-biplot technique (Yan et al., 2007). The GGE biplot technique can be used to determine: (1) Which-won-where patttern in genotype and environment, (2) Average environment coordination (AEC) based on environment focused scaling of the mean value and stability of genotype, (3) Ranking of entries based on both mean and instability, and (4) Discriminativeness vs. representativeness. To assess the yield improvement achieved by each treatment, a yield gap analysis was conducted. The yield gap represents the difference in yield between genotypes in various treatments and is denoted by the symbol of the absolute attainable yield gap (Yga). In this research, the yield gap can be calculated using the equation proposed by Senthilkumar (2022):
Where Yga was the absolute attainable yield gap (t ha−1); Yatt was the economically attainable/exploitable yield (t ha−1) using 3 different soil amendments, namely biochar, organic, and biochar + organic treatment; the actual yield of control (Yac) was the yield of paddy (t ha−1) with none of the additional soil amendments (Senthilkumar, 2022). Basically, yield actual (Yac) was the farmers’ agriculture practice in a rainfed agroecosystem. Local farmers have followed these practices for a long time.
Specifically, to know the differences across the treatments, the absolute yield gap between each treatment was analyzed. GAP1 (Yatto – Yac) was the absolute attainable yield gap between organic yield and control yield. GAP2 (Yattb + o – Yac) was the absolute attainable yield gap between biochar + organic yield and control yield. GAP3 (Yattb – Yac) was the absolute attainable yield gap between biochar yield and control yield. The data was analyzed using SAS 9.4 (Federer and King, 2006) and Rstudio software with metan (Olivoto and Lúcio, 2020), car (Fox and Weisberg, 2019), ggplot2 packages (Wickham, 2016).
3 Results
3.1 The yield of genotypes and yield improvement due to soil amendment on rainfed agroecosystem
The research findings indicate that the yield performance across various combinations of soil amendments and genotypes yielded mixed results. Graph show that all selected linear models had normally distributed data due to the points are on the line (Figure 3A) and homogeneous variance using a fitted value plot revealed that selected linear models had homogeneous because the points on the graph spread without a pattern (Figure 3B). The ANOVA analysis revealed significant impacts of soil amendments, genotype (G), and genotype × soil amendment (GEI) on grain yields (refer to Table S1 in Supplementary material). Notably, GEI exhibited a particularly high influence on grain yield (p < 0.01). Moreover, based on the contributions to variations in grain yield represented by the total sum of squares, the genotype factor emerged as the most significant influencer, followed closely by soil amendment. Specifically, the value of partial eta-square (ηp2) value of soil amendments, genotypes, and interaction indicates a large effect (ηp2 > 0.13) with value 0.74, 0.76, and 0.43, respectively.
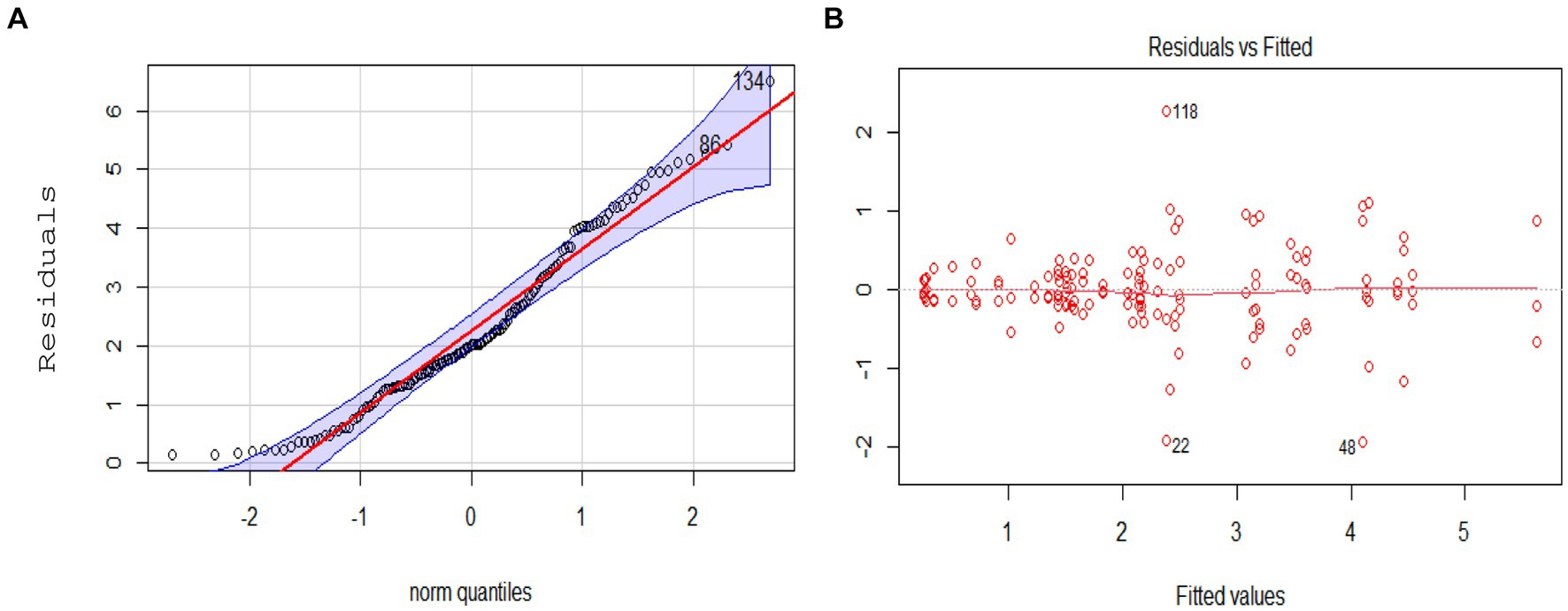
Figure 3. (A) Q–Q plot to evaluate the assumption of normally distributed variance; (B) Fitted value plot (residual against fitted value).
Figure 4 illustrates the yield and absolute yield gap of each genotype in different soil amendments within rainfed agroecosystems. Significance levels are denoted by lowercase letters above the bars, indicating differences identified through the Scott-Knott grouping test. In general, most genotypes exhibited improved yields with the addition of biochar and organic fertilizer compared to the control. The best interaction was observed in G2 with organic treatment, displaying the highest yield at 5.63 tons/ha, surpassing other interactions (see Figure 3). Conversely, when clustered by genotypes, some of the lowest performances were observed in the control (without soil amendment) across most genotype interactions. Specifically, the interaction of control × G6 demonstrated the lowest yield, with a value of 0.25 tons/ha (see Figure 3). Further analysis revealed significant differences (p < 0.05) in yield values between organic and control, as well as between biochar + organic and control for each genotype. Meanwhile, biochar exhibited significantly different yields than the control for 10 genotypes, excluding G4 and G7. These results underscore the impact of soil amendments and genotype interactions on grain yield, providing valuable insights for optimizing agricultural practices in rainfed agroecosystems.
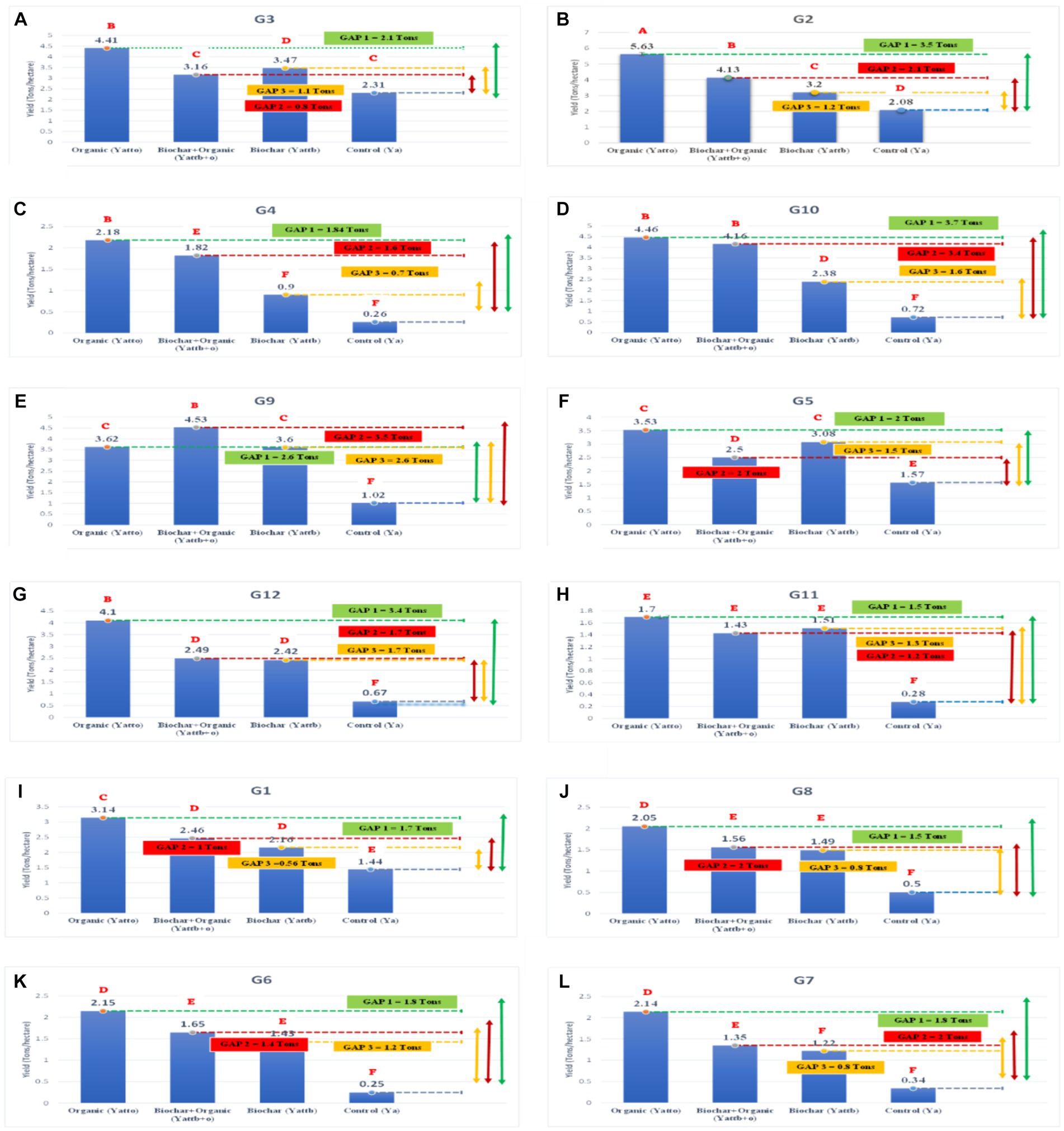
Figure 4. The yield and absolute yield gap of among genotypes (A) G3, (B) G2, (C) G4, (D) G10, (E) G9, (F) G5, (G) G12, (H) G11, (I) G1, (J) G8, (K) G6, (L) G7 in different soil amendment in rainfed agroecosystem. Different type lowercase letters above the bars indicated significantly different (p < 0.05, Scott-Knott grouping test).
Moving on to Figure 5, it displays the absolute attainable yield gap between treatments, subdivided into GAP1 (Yatto organic – Yac), GAP2 (Yattbiochar + organic – Yac), and GAP3 (Yatt biochar – Yac). Each subfigure corresponds to a specific comparison, aiding in the assessment of yield differences across treatments. Based on the yield gap analysis presented in Figure 5, it is evident that organic treatments consistently yielded the highest grain yields compared to biochar, biochar + organic, and the control, with the exception of G9. Specifically, the absolute attainable yield gap (GAP1) between organic and control was observed across various genotypes, revealing substantial improvements. For instance, GAP1 values were as follows for different genotypes: G1 (1.7 tons), G2 (3.55 tons), G3 (2.1 tons), G4 (1.92 tons), G5 (1.96 tons), G6 (1.90 tons), G7 (1.8 tons), G8 (1.55 tons), G9 (2.6 tons), G10 (3.7 tons), G11 (1.4 tons), and G12 (3.43 tons) (refer to Figure 3). The overall range for GAP1, representing the absolute attainable or exploitable yield gap between organic (Yatto) and control (Ya), was found to be in the range of 1.5–3.7 tons across different genotypes or increasing of 91–580% than control.
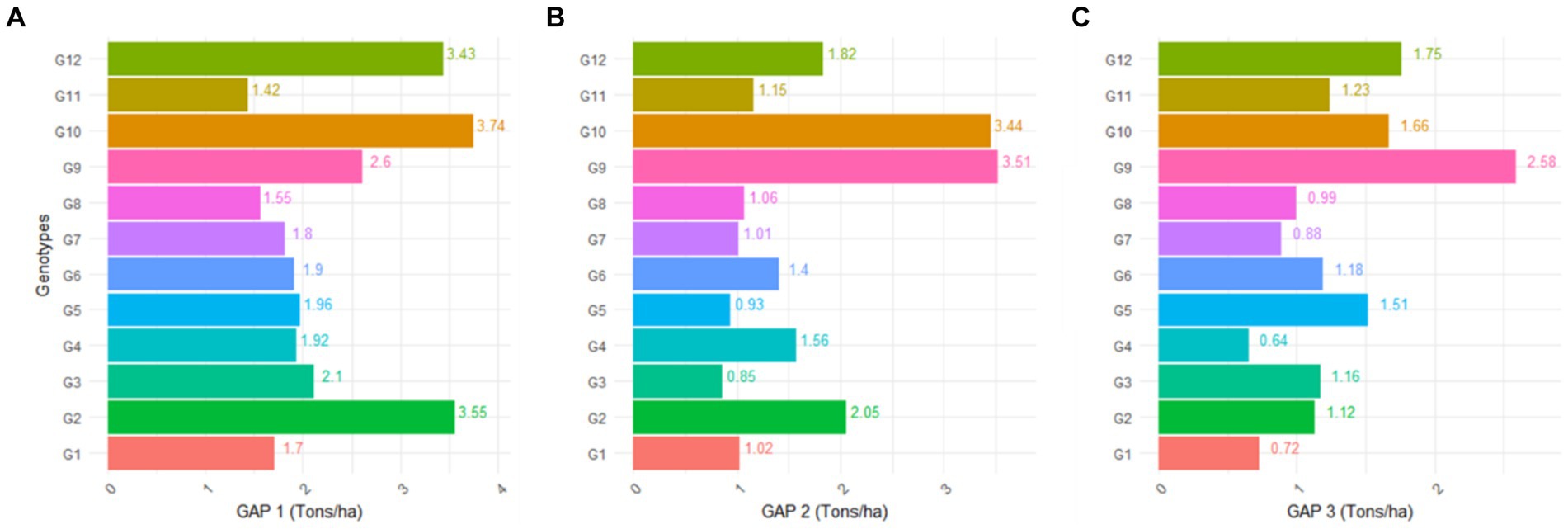
Figure 5. (A) The absolute attainable yield gap between each treatment was analyzed. GAP1 (Yatto organic – Yac); (B) GAP2 (Yattbiochar + organic – Yac); and (C) GAP3 (Yatt biochar – Yac).
The addition of biochar + organic also exerted a notable impact on paddy yield, surpassing the yield in the control for several genotypes. The yield of all genotypes demonstrated an increase with the application of this treatment. Specifically, among the different genotypes, the absolute attainable yield gap (GAP2) between biochar + organic and control was observed as follows: G1 (1.02 tons), G2 (2.05 tons), G3 (0.85 tons), G4 (1.56 tons), G5 (0.93 tons), G6 (1.4 tons), G7 (1 ton), G8 (1.06 tons), G9 (3.51 tons), G10 (3.44 tons), G11 (1.15 tons), and G12 (1.82 tons). Importantly, the yield differences between biochar + organic and control were found to be statistically significant (p < 0.05) for all genotypes. Consequently, the range for GAP2, representing the absolute attainable or exploitable yield gap between biochar + organic (Yattb + o) and control (Ya), was in the range of 0.8–3.5 tons across different genotypes or increasing of around 72–560%. Biochar demonstrated a positive impact on paddy yield compared to the control in select genotypes. The attainable yield gap (GAP3) between biochar and control (Yatt biochar - Yac) for different genotypes was observed as follows: G1 (0.71 tons), G2 (1.12 tons), G3 (1.16 tons), G4 (0.64 tons), G5 (1.51 tons), G6 (1.18 tons), G7 (0.88 tons), G8 (0.99 tons), G9 (2.58 tons), G10 (1.66 tons), G11 (1.23 tons), and G12 (1.75 tons). The overall range for GAP3, representing the absolute attainable or exploitable yield gap between biochar (Yattb) and control (Ya), was found to be in the range of 0.6–2.58 tons across different genotypes or increasing around 58–472%. Furthermore, the yield differences between biochar and control were statistically significant (p < 0.05) in G6, G1, G8, G12, G5, G9, G10, G3, and G2. These results highlight the effectiveness of biochar in enhancing paddy yield in specific genotypes within rainfed agroecosystems.
A comprehensive view of the absolute yield gap for all combination treatments (soil amendment and genotype) can be observed in the absolute yield gap matrix (refer to Figure 6). Figure 6 presents the absolute yield gap matrix across environments (soil amendments) and genotypes. This matrix offers a comprehensive overview of yield gaps, facilitating comparisons between different combinations of soil amendments and genotypes. Specifically, the least yield gap was approximately 0.01 tons per hectare, while the largest gap reached 5.3 tons/ha. The most substantial yield gap was identified in the comparison between organic x G2 and control x G6, registering a value of 5.3 tons/ha. Analyzing all combinations of genotype and soil amendments based on the absolute yield gap matrix, G2 with organic soil amendments emerged as the most favorable combination. It exhibited a positive yield gap, averaging around 1.1–5.38 tons/ha, surpassing other combinations (refer to Figure 6). The second-best combination was biochar + organic and G9, with an average yield gap of approximately 0.07–4.28 tons/ha compared to others. Conversely, control × G6 and G4 were identified as the least favorable combinations, yielding around 0.25 tons per hectare and 0.28 tons/ha, respectively. This assessment is attributed to their negative absolute yield gap when compared with all other treatments.
3.2 Determination of genotypes that suitable in rainfed agroecosystem
To assess the information pertaining to the evaluation of genotype, environment, and their interactions, the GGE Biplot methodology was employed, presenting visual parameters for these indicators. The GGE-biplot in this research was displayed with four types, namely discriminative versus representative, mean versus stability performance, which-won-where pattern, and ranking genotypes (refer to Figure 5). Mathematically, the GGE biplot characterizes the singular values for the first principal component (PC1) and the second principal component (PC2) through the contribution of diversity (total eigenvalues) obtained from the Singular Value Decomposition (SVD) of environment-centered or environment-standardized genotype-by-environment data (GED). The total variation in genotype (G) and genotype-by-environment interaction (GxE) was captured by PC1 and PC2, accounting for 93.33% of the total G + GxE variation (PC1 + PC2). The PC1 score represents the yield of the lines, with PC1 > 0 indicating high-yield lines and PC1 < 0 indicating low-yield lines. On the other hand, the PC2 score reflects stability, with scores approaching zero indicating stable lines and vice versa. In short, genotypes G2, G3, G1, G5, G12, G9, and G10 can be classified as high-yield genotypes, as their PC1 values are greater than zero or their average yields surpass the overall average for all genotypes. Conversely, G4, G6, G7, G8, and G11 are categorized as low-yield genotypes due to their PC1 values being less than zero.
The “which-won-where” aspect of the GGE Biplot comprises an irregular polygon and a set of lines drawn from the biplot origin, intersecting each side at right angles (refer to Figure 7C). This representation reveals that G2 and G3 emerged as the most suitable genotypes in biochar and organic environments, demonstrating higher yields than others in these conditions. Additionally, G9 exhibited greater suitability in a biochar + organic environment, displaying the highest yield compared to other genotypes in that specific setting. Conversely, G4, G6, G7, G8, and G11 demonstrated poor performance across all environments, as indicated by the polygon vertices where no environmental indicators fall within that sector. This highlights the inadequacy of these genotypes in adapting to diverse environments and their suboptimal performance in comparison to other genotypes. The assessment of mean performance and stability in the GGE Biplot (refer to Figure 7B) is valuable in evaluating an ideal genotype, which should ideally exhibit both high mean performance and high stability within a mega-environment. The arrow sign on the Average Environment Coordinate (AEC) abscissa provides insight into the ranking of genotypes in increasing order. Based on the rank orders, G2, G3, and G9 emerged as the top three genotypes, displaying the highest mean performance. In contrast, G3 and G10 were identified as two of the least stable genotypes. Moreover, G2, G11, G12, G7, and G8 were recognized as the most stable genotypes in this research. This determination is based on their shorter lines near zero, indicating a higher level of stability across different environments.
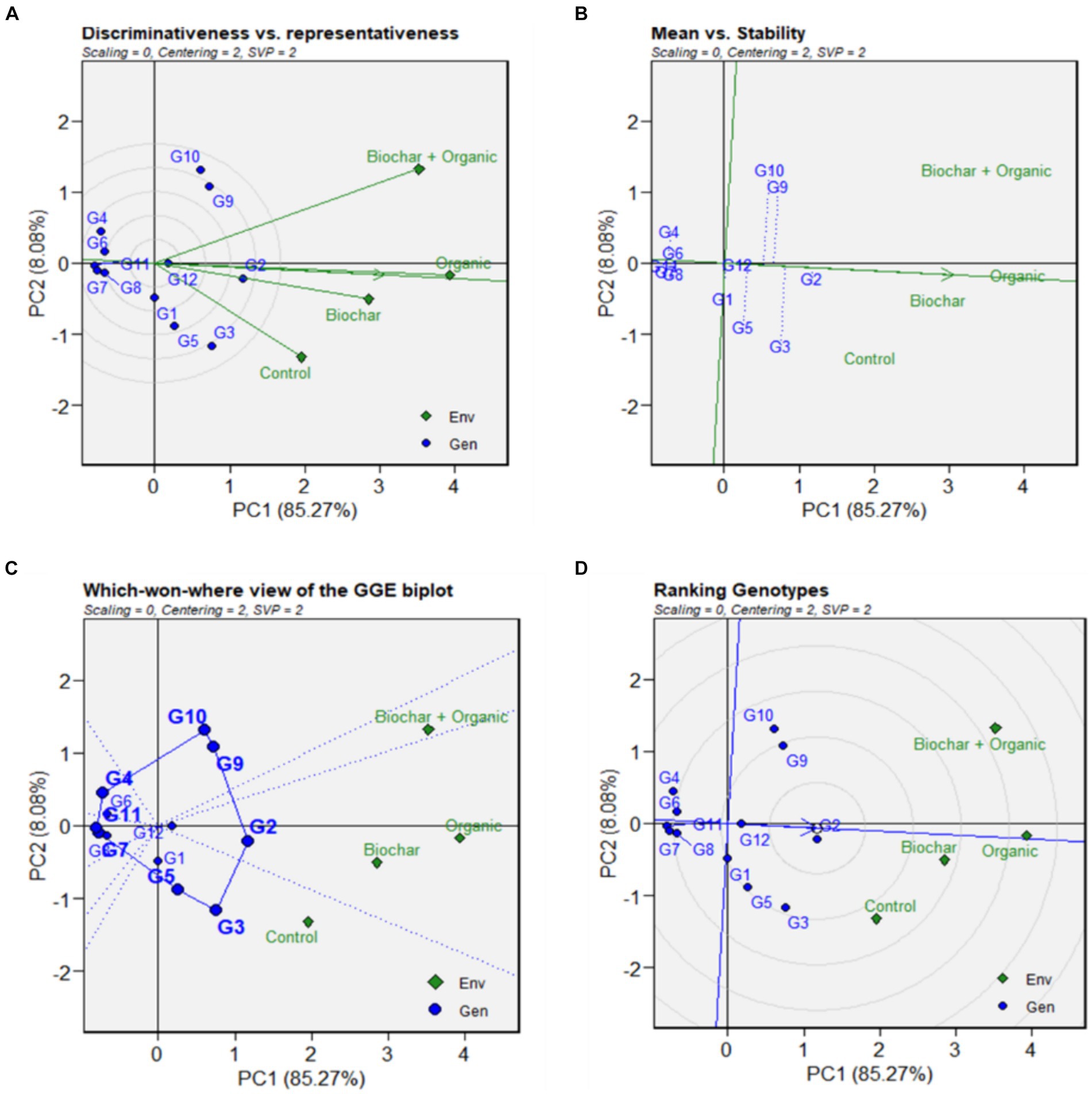
Figure 7. (A) GGE-Biplot visualization consisting of discriminative versus representative; (B) mean versus stability performance; (C) which-won-where pattern, and (D) ranking genotypes.
The Discriminating Power vs. Representativeness analysis of GGE (refer to Figure 7A), or the evaluation of test environments, proves effective in identifying superior genotypes for mega-environments. In this context, biochar emerged as the most favorable environment for selecting superior genotypes, evident from its small angles with the AEC abscissa. On the other hand, biochar + organic and organic environments, characterized by long vectors and large angles with the AEC abscissa, were deemed suitable for eliminating unstable genotypes. Furthermore, based on the correlation, biochar and organic exhibited a stronger positive correlation compared to other environments due to the acute angle between them. This positive correlation implies that biochar and organic environments provided more similar information about the yield of genotypes. Consequently, this analysis aids in discerning environments that are better suited for identifying superior genotypes and those suitable for assessing stability in genotype performance. In this study, our study presented the ranking of genotypes based on the arrowed Average Environment Coordinate (AEC) abscissa and various concentric circles. The results revealed that some genotypes were positioned closer to the center of the concentric circles, while others were relatively distant from the origin of the circles. Notably, among the genotypes, G2 held a central position near the center of the concentric circles, followed by G12, G3, G5, G1, and G9 (refer to Figure 6). Conversely, G4, G6, G7, and G8 were situated farther from the center of the concentric circles. In this context, G2 in the research can be considered as an exemplary cultivar., representing the ideal genotype with the highest yield and stability compared to other cultivars. This ranking provides valuable insights for selecting genotypes that exhibit a desirable balance of high mean performance and stability across different environments.
4 Discussion
Soil modification through the application of biochar and organic fertilizer emerges as a viable alternative to enhance paddy yield in rainfed conditions (Rassaei, 2022, 2024). Rainfed areas typically include critical zones vulnerable to water insufficiency, posing challenges to the growth and yield of plants. Cultivating paddy in such lands can detrimentally impact growth, development, and overall yield (Boonwichai et al., 2019). The observed improvement in paddy yield in this research can be attributed to the addition of biochar and organic fertilizer. The distinct values of absolute yield gap for each interaction highlight the significant influence of soil amendment management on paddy yield performance. The range values of GAP1, GAP2, and GAP3, representing the absolute attainable yield gaps, are within the ranges of 1.55–3.74 tons, 0.85–3.51 tons, and 0.64–2.58 tons, respectively (refer to Figures 2, 3). This underscores the substantial impact of soil amendment practices on enhancing paddy yield in rainfed agroecosystems.
4.1 Absolute yield gap using organic fertilizer and biochar in tested genotypes
The yield variations observed across all genotypes underscore the impact of different soil amendments. Notably, the application of organic fertilizer consistently yielded the highest response in most genotypes, suggesting that organic amendments may enhance genotypes’ ability to reach their yield potential more effectively than other amendments. Additionally, the addition of organic fertilizer significantly differed (p < 0.05) from the control in all genotypes (refer to Figure 4). Among all combinations, organic fertilizer demonstrated the highest yield for genotypes G1, G2, G3, G4, G5, G6, G7, G8, G10, G11, and G12, with values ranging from approximately 3.14 tons/ha to 5.63 tons/ha (refer to Figures 4, 5). Moreover, the absolute attainable yield gap with organic fertilizer exhibited the highest values for these genotypes (refer to Figure 5). The influence of organic fertilizer in improving yield is further highlighted by its longer vector in the GGE biplot analysis, indicating its higher impact compared to other environments (refer to Figure 7). Previous research has also shown yield improvements in paddy using organic fertilizer. For instance, the use of Chromolaena odorata (siam weed) compost (SWC) at 10 tons/ha resulted in the highest upland rice yield of 2.97 tons/ha, a 91.75% increase compared to the control without SWC (Suryanto et al., 2020a). Similarly, the application of livestock waste fertilizer (sewage sludge) increased plant productivity by up to 300%, reaching 6 tons/ha, compared to the control without sewage sludge, which yielded 2 tons/ha (Jatav et al., 2022). Considering genotype characteristics, previous studies have suggested that the yield performance of certain genotypes, such as G3 (GM 2), G2 (GM 28), and G11 (Inpari 33), is influenced by soil moisture content (Suryanto et al., 2020b). These genotypes have been observed to exhibit varying degrees of resilience and adaptability to environmental conditions, particularly in rainfed agroecosystems. In the current research, G2 emerged as particularly noteworthy, demonstrating remarkable suitability for organic environments. Notably, G2 displayed the highest yield and stability compared to other genotypes, as evidenced by its consistent performance across different soil amendments (refer to Figures 7B,D). This robust performance underscores the importance of genotype selection and adaptation to specific environmental conditions, highlighting the potential for optimizing agricultural productivity through targeted breeding programs and crop management strategies.
In this research, the utilization of organic fertilizer in the soil significantly improved the yield (p < 0.05). The positive impact of organic fertilizer on soil and crops is attributed to various mechanisms observed in previous studies. The application of organic fertilizer has been shown to enhance soil quality by increasing soil organic matter and influencing soil physical and chemical properties through mineral decomposition (Assefa and Tadesse, 2019). Additionally, (Pandey and Shukla, 2006) demonstrated that organic fertilizer application can increase soil moisture content by more than 3% compared to control conditions. Research by (Pagliai et al., 1981) indicated that applying 50 tons of sewage sludge organic fertilizer per hectare could significantly increase soil porosity by more than 50%, thereby enhancing the soil’s water retention capacity. Moreover, organic fertilizer has been found to positively impact plant water status and proline content. Ye et al. (2022) reported a 94.20% increase in the relative water content of pear plants with the use of organic fertilizer compared to conditions without organic fertilizer. In terms of proline content, (Alinezhad et al., 2013) demonstrated that organic fertilizer application reduced proline content in drought-stressed barley plants by 30%. Additionally, (Duo et al., 2018) showed that nano compost and microbial inoculation under severe drought stress conditions could decrease proline content by more than 20% in Festuca arundinacea. Overall, the findings highlight the multifaceted benefits of organic fertilizer, including its positive impact on soil properties, moisture retention, and plant water status, ultimately contributing to improved crop yield.
The yield increased with the addition of biochar + organic fertilizer combinations. The absolute attainable yield (GAP2) values for G1 to G12 were approximately 1.02 to 3.51 tons/ha. Overall, the absolute attainable yield with biochar + organic fertilizer was lower than organic fertilizer alone, indicating that the lower dosage of organic fertilizer in combination with biochar had a lesser impact on yield. Biochar primarily functions in nutrient transformation rather than providing nutrients to the soil (DeLuca et al., 2015). Research by (Schulz and Glaser, 2012) demonstrated that compost yielded 10% more tomato biomass compared to biochar when applied in the same amount (5% of soil weight). Moreover, the yield of genotypes with the addition of biochar alone improved, although to a lesser extent compared to organic and biochar + organic treatments. The yield increase (GAP3) for G1 to G12 ranged from 0.72 to 2.58 tons/ha compared to the control (Figure 3C). Previous research has also demonstrated increased paddy yield with the application of biochar. Singh et al. (2018) reported that 10 tons/ha of RHB can increase tiller number and yield by over 50% in nutrient-poor agricultural soils. Additionally, (Dong et al., 2015) observed that rice straw biochar enhances paddy yield in waterlogged conditions.
The mechanism through which biochar improves soil conditions and enhances crop yield in rainfed areas involves physical, chemical, and biological amendment indicators, as observed in previous research. Biochar, produced through the pyrolysis process from carbon-based feedstock, plays a crucial role in the soil ecosystem. Biologically, its application fosters a conducive environment for soil microbial communities (Zhu et al., 2017). The two most commonly biological communities of mycorrhizal fungi arbuscular (AM) and ectomycorrhizal (EM) are often positively affected by biochar presence (Warnock et al., 2007). Specifically, Arbuscular mycorrhizal fungi (AMF) have demonstrated that AMF can improve the growth of host plants by promoting nutrient and water uptake to alleviate in drought stress condition (Bowles et al., 2018). Furthermore, these microbes can explore soil pores with the root hair to access water and nutrient sources (Li et al., 2019). On physically effects, biochar addition can reduce the overall tensile strength of the soil which therefore make root nutrient mining more effective, as well as allow seeds to germinate more easily as well as invertebrates to move through the soil easier (Lehmann et al., 2011). Moreover, biochar positively influences soil water retention due to its unique structure, characterized by a high internal surface area and numerous pores (Ghodake et al., 2021). Studies by Varela Milla et al. (2013) demonstrated that the addition of 0.5 kg of biochar per cubic meter of soil increased soil moisture content by 5%. Additionally, Wang et al. (2019) found that adding 1 gram of walnut shell biochar to 1 kilogram of soil increased water field capacity by approximately 20% in sandy loam soil. On chemical properties on soil, biochar can improve the electrochemical properties of the roots and thereby increase nutrient absorption by crops. Electrochemical properties of roots in the form of zeta potential and cation exchange capacity play an important role in the absorption of nutrients by plants and adding 25 g biochar to 1 kg of soil can amend electrochemical properties of roots, nutrients absorption, and growth parameters of safflower and mint (Farhangi-Abriz and Ghassemi-Golezani, 2023). The positive effects of biochar on water retention in soil also extend to plant water status and proline levels under drought stress conditions Akhtar et al. (2014) reported that increased water holding capacity (WHC) in soil due to biochar application enhanced leaf relative water content (RWC) in tomatoes subjected to deficit irrigation. Moreover, the application of biochar led to a reduction in proline content in various plants, including Quercus castaneifolia seedlings (Zoghi et al., 2019), Rosmarinus officinalis (Kasmaei et al., 2019), and perennial ryegrass (Lolium perenne L.) (Safari et al., 2023).
In the context of national production, the combination of specific genotypes and soil amendments demonstrated in this study holds significant promise for implementation in rainfed areas. Particularly noteworthy is the potential for these combinations to surpass the average national yield of 3.7 tons/ha, as reported by Statistics Indonesia (2021). This suggests that the findings of our research have practical implications for enhancing agricultural productivity and sustainability at the national level. Notably, combinations such as G2 + organic, G3 + organic, G10 + organic, G9 + biochar + organic, and G12 + organic exhibited yields surpassing the national average (Figure 2). Among these, G2 + organic emerged as the most favorable combination, displaying both high yield and stability across environments (Figures 4, 6, 7B,D). While G9 and G10 exhibited high yields, their stability warrants further investigation over the long term (Figure 5B). The yield gap analysis conducted in this research emphasizes the potential of soil management technologies in enhancing paddy productivity in rainfed areas. Moreover, organic treatment consistently resulted in the highest yields across genotypes compared to other treatments, except for G9. Conversely, the control treatment, representing conventional farmer practices without soil amendments, showed the lowest yields. This underscores the importance of incorporating soil amendments in rainfed agroecosystems to maintain ecological functions and enhance paddy yields over the long term. The long-term use of chemical fertilizers by farmers, leading to decreased soil organic matter, soil organism populations, soil compaction, and increased soil acidity, likely contributed to reduced yields (Pahalvi et al., 2021). Through identifying genotypes and soil amendments that can outperform the national average yield, our study offers valuable insights for policymakers, agricultural practitioners, and stakeholders seeking to improve agricultural outcomes in rainfed agroecosystems. Moreover, the adoption of these optimized combinations has the potential to contribute to food security, economic development, and environmental sustainability efforts on a broader scale. However, further validation and field trials are warranted to confirm the scalability and replicability of these findings across diverse agroecological contexts and farming systems. Through continued research and collaborative efforts through exploring additional soil amendments, assessing the long-term effects of different treatments, and investigating interactions between soil, genotype, using inhibitors, and environmental factors can leverage the findings of this study to advance sustainable agricultural practices and address the challenges of food production in rainfed areas more effectively (Yoshida and Horie, 2010; Yoshida et al., 2011; Lin et al., 2021, 2022a,b).
Developing paddy cultivation in rainfed areas presents numerous challenges associated with climatic variability (Boer et al., 2004; Naylor et al., 2007). The growth of paddy crops is inherently dependent on dynamic rainfall patterns, which vary annually and significantly impact yield outcomes (Ansari et al., 2021, 2023). Furthermore, it is essential to recognize that in this study, factors such as water evaporation and transpiration were not explicitly accounted for, which could provide valuable additional data to support yield assessments. Moving forward, it is imperative to incorporate comprehensive variables related to climatic conditions and soil water dynamics, including soil relative humidity, water evaporation, and transpiration rates (Ansari et al., 2019). Through integrating these factors into our analyses, this research can achieve a more precise and comprehensive understanding of paddy yield dynamics in rainfed agroecosystems (Alam et al., 2022).
The current study reveals several evident limitations that warrant discussion. Firstly, our focus on paddy yield measurements, while informative, represents a partial examination of crop performance. Regrettably, our analysis did not extend to encompassing crucial aspects of growth and physiology, such as leaf area, proline levels, chlorophyll content, and other physiological parameters. This oversight is noteworthy considering existing research that highlights correlations between these physiological factors and yield outcomes. Thus, the absence of such comprehensive physiological assessments in our study may restrict the depth of understanding regarding the mechanisms driving yield variations in response to soil amendment treatments. Secondly, the limitations of our study are compounded by constraints in water measurement conditions. Water dynamics, including absorption rates, crop water content, evaporation rates, and transpiration rates, exert significant influences on paddy growth and yield. However, our research did not thoroughly investigate these parameters, potentially overlooking critical factors affecting crop performance. Recognizing the importance of water management in agricultural productivity, future investigations should strive to incorporate more comprehensive assessments of morphological and physiological parameters of paddy plants, along with detailed analyses of soil water conditions. Through addressing these limitations and broadening the scope of inquiry, future research endeavors can contribute to a more nuanced understanding of the complex interactions between soil amendments, water dynamics, and crop performance in rainfed agroecosystems. Despite these limitations, our findings offer valuable insights into the potential of soil amendments to address the challenges of low productivity in rainfed conditions. Specifically, our research highlights the efficacy of biochar and organic fertilizer as alternative solutions for enhancing paddy yield in rainfed environments characterized by numerous agronomic and climatic constraints. By exploring innovative approaches such as soil amendment strategies, we can effectively mitigate the adverse impacts of climatic variability and optimize agricultural productivity in rainfed areas. These findings contribute to the ongoing dialog surrounding sustainable agriculture practices and offer tangible solutions to enhance food security and livelihoods in rainfed regions. The application of biochar and organic fertilizer has demonstrated promising outcomes in enhancing paddy yields within rainfed agricultural systems. However, it is essential to recognize that the effectiveness of these treatments may vary significantly across different environmental contexts. This variability stems from a complex interplay of integrated factors, including soil fertility, climatic conditions (especially rainfall intensity), and the genetic characteristics of paddy varieties. Consequently, it is imperative to conduct further comprehensive research across diverse environmental settings to fully understand the nuanced effects of these soil amendments by conducting studies in various environments to elucidate how different soil and climatic conditions interact with treatment applications, thereby refining our understanding of optimal agricultural practices for sustainable paddy cultivation.
5 Conclusion
In conclusion, our research underscores the substantial positive impact of soil amendments, particularly organic and biochar + organic treatments, on enhancing paddy yield in rainfed agroecosystems. Through rigorous analysis, we have identified significant absolute attainable yield gaps across different treatments, ranging from 1.5 to 3.7 tons/ha or an increase of 91 to 580% for GAP1, 0.8 to 3.5 tons/ha (72 to 560%) for GAP2, and 0.6 to 2.58 tons/ha (58 to 472%) for GAP3. These findings highlight the remarkable potential of targeted soil management strategies to substantially increase paddy yield in rainfed conditions. Of particular note is the outstanding performance of G2 with organic fertilizer amendments, which demonstrated a positive absolute yield gap and an average yield improvement of 1.1 to 5.38 tons/ha compared to other treatments. This underscores the effectiveness of organic amendments in boosting productivity and underscores the importance of selecting appropriate genotypes for specific soil environments. Moreover, our GGE biplot analysis reinforces these results by emphasizing the suitability of certain genotypes for specific soil environments. Notably, G2 and G3 excel in biochar and organic environments, respectively, while G9 shows exceptional performance in biochar + organic conditions. By evaluating genotypes based on both mean performance and stability, we have identified G2 as the top-performing genotype, providing valuable insights into cultivar selection for rainfed conditions. Overall, our study underscores the critical importance of sustainable soil management practices in optimizing paddy yield for long-term agricultural productivity in rainfed areas. Through leveraging the positive outcomes observed in this research, we can further advance our understanding and implementation of sustainable agricultural strategies, ultimately contributing to enhanced food security and livelihoods in rainfed regions. Additionally, future research efforts should explore additional soil amendments, investigate genotype-environment interactions in more detail, adding the substance to reduce GHG emissions, and assess the long-term sustainability of implemented soil management practices to continuously improve agricultural productivity in rainfed areas.
Data availability statement
The original contributions presented in the study are included in the article/Supplementary material, further inquiries can be directed to the corresponding author.
Author contributions
YS: Conceptualization, Formal analysis, Investigation, Writing – original draft, Writing – review & editing. BK: Methodology, Supervision, Writing – review & editing, Validation. TA: Data curation, Investigation, Validation, Writing – review & editing. SH: Data curation, Formal analysis, Investigation, Methodology, Writing – review & editing. Supriyanta: Data curation, Investigation, Methodology, Supervision, Writing – review & editing. AA: Data curation, Investigation, Software, Supervision, Validation, Writing – review & editing. Taryono: Conceptualization, Funding acquisition, Supervision, Writing – review & editing.
Funding
The author(s) declare that financial support was received for the research, authorship, and/or publication of this article. The authors thank the Agrotechnology Innovation Center of Universitas Gadjah Mada, which has provided facilities to support this research. This research is supported by Universitas Gadjah Mada through Final Project Recognition Grant Universitas Gadjah Mada Number 5075/UN1.P.II/Dit-Lit/PT.01.01/2023.
Conflict of interest
The authors declare that the research was conducted in the absence of any commercial or financial relationships that could be construed as a potential conflict of interest.
Publisher’s note
All claims expressed in this article are solely those of the authors and do not necessarily represent those of their affiliated organizations, or those of the publisher, the editors and the reviewers. Any product that may be evaluated in this article, or claim that may be made by its manufacturer, is not guaranteed or endorsed by the publisher.
Supplementary material
The Supplementary material for this article can be found online at: https://www.frontiersin.org/articles/10.3389/fsufs.2024.1384530/full#supplementary-material
References
Abel, S., Peters, A., Trinks, S., Schonsky, H., Facklam, M., and Wessolek, G. (2013). Impact of biochar and hydrochar addition on water retention and water repellency of sandy soil. Geoderma 202-203, 183–191. doi: 10.1016/J.GEODERMA.2013.03.003
Akhtar, S. S., Li, G., Andersen, M. N., and Liu, F. (2014). Biochar enhances yield and quality of tomato under reduced irrigation. Agric. Water Manag. 138, 37–44. doi: 10.1016/j.agwat.2014.02.016
Alam, T., Suryanto, P., Handayani, S., Kastono, D., and Kurniasih, B. (2020). Optimizing application of biochar, compost and nitrogen fertilizer in soybean intercropping with kayu putih (Melaleuca cajuputi). Rev. Bras. Ciênc. Solo 44, 1–17. doi: 10.36783/18069657rbcs20200003
Alam, T., Suryanto, P., Handayani, S., Supriyanta, S., Wulandari, R. A., Anshari, A., et al. (2022). Soil quality measurement for sustainable soybean yield agroforestry system under different crop rotation models. Biodiversitas J. Biol. Divers. 23, 6155–6163. doi: 10.13057/biodiv/d231209
Alinezhad, S., Sinaki, J. M., Zarei, M., and Abadi, M. B. F. (2013). Effects of organic fertilizers and drought stress on physiological traits in barley. Int. J. Agron. Plant Product. 4, 300–306
An, N., Zhang, L., Liu, Y., Shen, S., Li, N., Wu, Z., et al. (2022). Biochar application with reduced chemical fertilizers improves soil pore structure and rice productivity. Chemosphere 298:134304. doi: 10.1016/j.chemosphere.2022.134304
Ansari, A., Kato, T., and Fitriah, A. (2019). Simulating streamflow through the SWAT model in the Keduang sub-watershed, Wonogiri regency, Indonesia. AgriTECH 39, 60–69. doi: 10.22146/agritech.29737
Ansari, A., Lin, Y.-P., and Lur, H.-S. (2021). Evaluating and adapting climate change impacts on Rice production in Indonesia: a case study of the Keduang subwatershed Central Java. Environments 8:117. doi: 10.3390/environments8110117
Ansari, A., Pranesti, A., Telaumbanua, M., Alam, T., Taryono, T., Wulandari, R. A., et al. (2023). Evaluating the effect of climate change on rice production in Indonesia using multimodelling approach. Heliyon 9:e19639. doi: 10.1016/j.heliyon.2023.e19639
Assefa, S., and Tadesse, S. (2019). The principal role of organic fertilizer on soil properties and agricultural productivity-a review. Agric. Res. Tech: Open Access J 22:556192. doi: 10.19080/ARTOAJ.2019.22.556192
Atkinson, C. J., Fitzgerald, J. D., and Hipps, N. A. (2010). Potential mechanisms for achieving agricultural benefits from biochar application to temperate soils: a review. In Plant Soil 1:337. doi: 10.1007/s11104-010-0464-5
Barus, J. (2016). Utilization of crops residues as compost and biochar for improving soil physical properties and upland rice productivity. J. Degrad. Mining Lands Manag. 3, 631–637. doi: 10.15243/jdmlm.2016.034.631
Bhering, L. L., Cruz, C. D., De Vasconcelos, E. S., Ferreira, A., and De Resende, M. F. R. (2008). Alternative methodology for Scott-Knott test. Crop Breed. Appl. Biotechnol. 8, 9–16. doi: 10.12702/1984-7033.v08n01a02
Boer, R., Faqih, A., Naylor, R. L., Battisti, D. S., Vimont, D. J., Falcon, W. P., et al. (2004). Current and future rainfall variability in Indonesia. An integrated assessment of climate change impacts, adaptation and vulnerability in watershed areas and communities in Southeast Asia. Report from AIACC project no AS21. Int. START Secret. 104:47
Boonwichai, S., Shrestha, S., Babel, M. S., Weesakul, S., and Datta, A. (2019). Evaluation of climate change impacts and adaptation strategies on rainfed rice production in Songkhram River basin, Thailand. Sci. Total Environ. 652, 189–201. doi: 10.1016/j.scitotenv.2018.10.201
Bowles, T. M., Jackson, L. E., and Cavagnaro, T. R. (2018). Mycorrhizal fungi enhance plant nutrient acquisition and modulate nitrogen loss with variable water regimes. Glob. Chang. Biol. 24, e171–e182. doi: 10.1111/gcb.13884
Chen, S., Zeng, F., Pao, Z., and Zhang, G. (2008). Characterization of high-yield performance as affected by genotype and environment in rice. J Zhejiang Univ Sci B 9, 363–370. doi: 10.1631/jzus.B0710603
Chen, M., Zhang, S., Liu, L., Liu, J., and Ding, X. (2022). Organic fertilization increased soil organic carbon stability and sequestration by improving aggregate stability and iron oxide transformation in saline-alkaline soil. Plant Soil 474, 233–249. doi: 10.1007/s11104-022-05326-3
Da Silva Mendes, J., Fernandes, J. D., Chaves, L. H. G., Guerra, H. O. C., Tito, G. A., and de Brito Chaves, I. (2021). Chemical and physical changes of soil amended with biochar. Water Air Soil Pollut. 232, 1–13. doi: 10.1007/s11270-021-05289-8
de Mendiburu, F. (2021). Agricolae: R package version 1.3–5. Vienna, Austria: Statistical Procedures for Agricultural Research.
DeLuca, T. H., Gundale, M. J., MacKenzie, M. D., and Jones, D. L. (2015). Biochar effects on soil nutrient transformations. Biochar. Environ. Manag. Sci. Technol. Implement. 2, 421–454. doi: 10.4324/9781849770552
Devendra, C. (2012). Rainfed areas and animal agriculture in Asia: the wanting agenda for transforming productivity growth and rural poverty. Asian Australas. J. Anim. Sci. 25, 122–142. doi: 10.5713/ajas.2011.r.09
Dong, D., Feng, Q., Mcgrouther, K., Yang, M., Wang, H., and Wu, W. (2015). Effects of biochar amendment on rice growth and nitrogen retention in a waterlogged paddy field. J. Soils Sediments 15, 153–162. doi: 10.1007/s11368-014-0984-3
Duo, L. A., Liu, C. X., and Zhao, S. L. (2018). Alleviation of drought stress in turfgrass by the combined application of nano-compost and microbes from compost. Russ. J. Plant Physiol. 65, 419–426. doi: 10.1134/S102144371803010X
Evans, L. T., and Fischer, R. A. (1999). Yield potential: its definition, measurement, and significance. Crop Sci. 39, 1544–1551. doi: 10.2135/cropsci1999.3961544x
Farhangi-Abriz, S., and Ghassemi-Golezani, K. (2023). Improving electrochemical characteristics of plant roots by biochar is an efficient mechanism in increasing cations uptake by plants. Chemosphere 313:137365. doi: 10.1016/j.chemosphere.2022.137365
Federer, W. T., and King, F. (2006). Variations on Split plot and Split block experiment designs. Variations on Split Plot and Split Block Experiment Designs. doi: 10.1002/0470108584
Fox, J., and Weisberg, S. (2019). An {R} companion to applied regression, third edition. Sage: Thousand Oaks CA.
Ghassemi-Golezani, K., Rahimzadeh, S., and Farhangi-Abriz, S. (2023). Utilization of biochar in rainfed farming systems: a meta-analysis. Bioresour. Technol. Rep. 22:101436. doi: 10.1016/j.biteb.2023.101436
Ghodake, G. S., Shinde, S. K., Kadam, A. A., Saratale, R. G., Saratale, G. D., Kumar, M., et al. (2021). Review on biomass feedstocks, pyrolysis mechanism and physicochemical properties of biochar: state-of-the-art framework to speed up vision of circular bioeconomy. J. Clean. Prod. 297:126645. doi: 10.1016/j.jclepro.2021.126645
Głąb, T., Żabiński, A., Sadowska, U., Gondek, K., Kopeć, M., Mierzwa-Hersztek, M., et al. (2020). Fertilization effects of compost produced from maize, sewage sludge and biochar on soil water retention and chemical properties. Soil Tillage Res. 197:104493. doi: 10.1016/j.still.2019.104493
Güereña, D., Lehmann, J., Hanley, K., Enders, A., Hyland, C., and Riha, S. (2013). Nitrogen dynamics following field application of biochar in a temperate north American maize-based production system. Plant Soil 365, 239–254. doi: 10.1007/s11104-012-1383-4
Hardie, M., Clothier, B., Bound, S., Oliver, G., and Close, D. (2014). Does biochar influence soil physical properties and soil water availability? Plant Soil 376, 347–361. doi: 10.1007/s11104-013-1980-x
Herath, H. M. S. K., Camps-Arbestain, M., and Hedley, M. (2013). Effect of biochar on soil physical properties in two contrasting soils: An Alfisol and an Andisol. Geoderma 209-210, 188–197. doi: 10.1016/J.GEODERMA.2013.06.016
Houot, S., Cambier, P., Benoit, P., Bodineau, G., Deschamps, M., Jaulin, A., et al. (2009). Effet d’apports de composts sur la disponibilité de micropolluants métalliques et organiques dans un sol cultivé. Étude et Gestion Des Sols 16, 255–274.
IRRI (2015). Steps to successful Rice production. Los Baños, Philippines: International Rice Research Institute.
Islam, M. U., Jiang, F., Guo, Z., and Peng, X. (2021). Does biochar application improve soil aggregation? A meta-analysis. Soil Tillage Res. 209:104926. doi: 10.1016/j.still.2020.104926
Jaramillo, S., Graterol, E., and Pulver, E. (2020). Sustainable transformation of rainfed to irrigated agriculture through water harvesting and smart crop management practices. Front. Sustain. Food Syst. 4:437086. doi: 10.3389/fsufs.2020.437086
Jatav, S. S., Singh, S. K., Parihar, M., Alsuhaibani, A. M., Gaber, A., and Hossain, A. (2022). Application of sewage sludge in a rice (Oryza sativa L.)-wheat (Triticum aestivum L.) system influences the growth, yield, quality and heavy metals accumulation of rice and wheat in the northern Gangetic alluvial plain. Life 12:484. doi: 10.3390/life12040484
Kang, M. S. (2004). Breeding: genotype-by-environment interaction. Encyclopedia Plant Crop Sci., 218–221. doi: 10.1081/E-EPCS-120010525
Kasmaei, L. S., Yasrebi, J., Zarei, M., Ronaghi, A., Ghasemi, R., Saharkhiz, M. J., et al. (2019). Impacts of PGPR, compost and biochar of Azolla on dry matter yield, nutrient uptake, physiological parameters and essential oil of Rosmarinus officinalis L. J. Cultiv. Plants/Journal Für Kulturpflanzen 71, 3–13. doi: 10.5073/JfK.2019.01.01
Kastono, D., Suryanto, P., Rogomulyo, R., Handayani, S., Supriyanta, W. M. H., and Alam, T. (2022). Differences in biochar sources for controlled nitrogen loss in a hybrid maize agroforestry system with Melaleuca cajuputi. J. Eng. Tech. Sci. 54, 220109–220134. doi: 10.5614/j.eng.technol.sci.2022.54.1.9
Khasanah, I. N., and Astuti, K. (2022). Luas Panen dan Produksi Padi di Indonesia 2022. Indonesia: Badan Pusat Statistik.
Kim, J. S., Sparovek, G., Longo, R. M., De Melo, W. J., and Crowley, D. (2007). Bacterial diversity of terra preta and pristine forest soil from the Western Amazon. Soil Biol. Biochem. 39, 684–690. doi: 10.1016/j.soilbio.2006.08.010
Kong, K. K., and Sii, H. S. (2020). Design and construction of mobile biochar kiln for small farmers. IOP Conf. Ser. Mater. Sci. Eng. 788:12075. doi: 10.1088/1757-899X/788/1/012075
Kowalski, S. M., and Potcner, K. J. (2003). How to recognize a Split-plot experiment. Qual. Prog. 36, 60–66.
Kumar, A., Raman, A., Yadav, S., Verulkar, S. B., Mandal, N. P., Singh, O. N., et al. (2021). Genetic gain for rice yield in rainfed environments in India. Field Crop Res. 260:107977. doi: 10.1016/j.fcr.2020.107977
Lal, R. (2020). Soil organic matter content and crop yield. J. Soil Water Conserv. 75, 27A–32A. doi: 10.2489/jswc.75.2.27A
Lehmann, J., Rillig, M. C., Thies, J., Masiello, C. A., Hockaday, W. C., and Crowley, D. (2011). Biochar effects on soil biota - a review. Soil Biol. Biochem. 43. doi: 10.1016/j.soilbio.2011.04.022
Li, J., Meng, B., Chai, H., Yang, X., Song, W., Li, S., et al. (2019). Arbuscular mycorrhizal fungi alleviate drought stress in C3 (Leymus chinensis) and C4 (hemarthria altissima) grasses via altering antioxidant enzyme activities and photosynthesis. Front. Plant Sci. 10, 1–12. doi: 10.3389/fpls.2019.00499
Li, C., Yun, S., and Zhang, L. (2018). Effects of long-term organic fertilization on soil microbiologic characteristics, yield and sustainable production of winter wheat. J. Integr. Agric. 17, 210–219. doi: 10.1016/S2095-3119(17)61740-4
Liang, B., Lehmann, J., Sohi, S. P., Thies, J. E., O’Neill, B., Trujillo, L., et al. (2010). Black carbon affects the cycling of non-black carbon in soil. Org. Geochem. 41, 206–213. doi: 10.1016/j.orggeochem.2009.09.007
Lin, Y. P., Ansari, A., Cheng, L. C., Lin, C. M., Wunderlich, R. F., Cao, T. N. D., et al. (2021). Measuring responses of dicyandiamide-, 3,4-dimethylpyrazole phosphate-, and allylthiourea-induced nitrification inhibition to soil abiotic and biotic factors. Int. J. Environ. Res. Public Health 18, 1–12. doi: 10.3390/ijerph18137130
Lin, Y.-P., Ansari, A., Ngoc-Dan Cao, T., Shiau, Y.-J., Lur, H.-S., Muzaffar, A., et al. (2022a). Using inhibitors to trade greenhouse gas emission for ammonia losses in paddy soil: a zero-sum game. Environ. Technol. Innov. 28:102547. doi: 10.1016/j.eti.2022.102547
Lin, Y.-P., Ansari, A., Wunderlich, R. F., Lur, H.-S., Ngoc-Dan Cao, T., and Mukhtar, H. (2022b). Assessing the influence of environmental niche segregation in ammonia oxidizers on N2O fluxes from soil and sediments. Chemosphere 289:133049. doi: 10.1016/j.chemosphere.2021.133049
Lu, S. G., Sun, F. F., and Zong, Y. T. (2014). Effect of rice husk biochar and coal fly ash on some physical properties of expansive clayey soil (vertisol). Catena 114, 37–44. doi: 10.1016/j.catena.2013.10.014
Mishra, A., Taing, K., Hall, M. W., and Shinogi, Y. (2017). Effects of rice husk and rice husk charcoal on soil physicochemical properties, rice growth and yield. Agric. Sci. 8, 1014–1032. doi: 10.4236/as.2017.89074
Mukhtar, H., Ansari, A., Ngoc-Dan Cao, T., Wunderlich, R. F., and Lin, Y. P. (2023). Thermodynamic sensitivity of ammonia oxidizers-driven N2O fluxes under oxic-suboxic realms. Chemosphere 334:138872. doi: 10.1016/j.chemosphere.2023.138872
Naylor, R. L., Battisti, D. S., Vimont, D. J., Falcon, W. P., and Burke, M. B. (2007). Assessing risks of climate variability and climate change for Indonesian rice agriculture. Proc. Natl. Acad. Sci. USA 104, 7752–7757. doi: 10.1073/pnas.0701825104
Obia, A., Mulder, J., Martinsen, V., Cornelissen, G., and Børresen, T. (2016). In situ effects of biochar on aggregation, water retention and porosity in light-textured tropical soils. Soil Tillage Res. 155, 35–44. doi: 10.1016/J.STILL.2015.08.002
Olivoto, T., and Lúcio, A. D. C. (2020). Metan: an R package for multi-environment trial analysis. Methods Ecol. Evol. 11, 783–789. doi: 10.1111/2041-210X.13384
Pagliai, M., Guidi, G., La Marca, M., Giachetti, M., and Lucamante, G. (1981). Effects of sewage sludges and composts on soil porosity and aggregation. Wiley Online Library.
Pahalvi, H. N., Rafiya, L., Rashid, S., Nisar, B., and Kamili, A. N. (2021). Chemical fertilizers and their impact on soil health: ecofriendly tools for reclamation of degraded soil environs. Microbiota Biofertiliz. 2, 1–20
Pandey, C., and Shukla, S. (2006). Effects of composted yard waste on water movement in sandy soil. Compost Sci. Util. 14, 252–259. doi: 10.1080/1065657X.2006.10702293
Purakayastha, T. J., Bera, T., Bhaduri, D., Sarkar, B., Mandal, S., Wade, P., et al. (2019). A review on biochar modulated soil condition improvements and nutrient dynamics concerning crop yields: pathways to climate change mitigation and global food security. Chemosphere 227, 345–365. doi: 10.1016/j.chemosphere.2019.03.170
Rassaei, F. (2022). Effect of two different sources of organic amendment on soil characteristics and chemical forms of cadmium. Agrochimica: Int. J. Plant Chem. Soil Sci. Plant Nutr. 66, 277–293. doi: 10.12871/00021857202244
Rassaei, F. (2023). Nitrous oxide emissions from rice paddy: impacts of rice straw and water management. Environ. Prog. Sustain. Energy 42:e14066. doi: 10.1002/ep.14066
Rassaei, F. (2024). Rice yield and carbon dioxide emissions in a paddy soil: a comparison of biochar and polystyrene microplastics. Environ. Prog. Sustain. Energy 43:e14217. doi: 10.1002/ep.14217
Ren, F., Sun, N., Xu, M., Zhang, X., Wu, L., and Xu, M. (2019). Changes in soil microbial biomass with manure application in cropping systems: a meta-analysis. Soil Tillage Res. 194:104291. doi: 10.1016/j.still.2019.06.008
Rockström, J., Karlberg, L., Wani, S. P., Barron, J., Hatibu, N., Oweis, T., et al. (2010). Managing water in rainfed agriculture—the need for a paradigm shift. Agric. Water Manag. 97, 543–550. doi: 10.1016/j.agwat.2009.09.009
Rozi, F., Santoso, A. B., Mahendri, I. G. A. P., Hutapea, R. T. P., Wamaer, D., Siagian, V., et al. (2023). Indonesian market demand patterns for food commodity sources of carbohydrates in facing the global food crisis. Heliyon 9:e16809. doi: 10.1016/j.heliyon.2023.e16809
Sacklokham, S., Chialue, L., and Yang, F. (2020). Rainfed and irrigated rice farming on the Savannakhet plain. White Gold, 151–168. doi: 10.1007/978-981-15-0998-8_7
Safari, S., Nazari, F., Vafaee, Y., and Teixeira da Silva, J. A. (2023). Impact of rice husk biochar on drought stress tolerance in perennial ryegrass (Lolium perenne L.). J. Plant Growth Regul. 42, 810–826. doi: 10.1007/s00344-022-10588-3
Sang, D. A., Bakar, R. A., Ahmad, S. H., and Rahim, K. A. (2018). Infuences of rice husk biochar (RHB) on rice growth performance and fertilizer nitrogen recovery up to maximum tillering stage. J. Wetlands Environ. Manag. 6, 32–44. doi: 10.20527/jwem.v6i1.150
Schulz, H., and Glaser, B. (2012). Effects of biochar compared to organic and inorganic fertilizers on soil quality and plant growth in a greenhouse experiment. J. Plant Nutr. Soil Sci. 175, 410–422. doi: 10.1002/jpln.201100143
Scott, A. J., and Knott, M. (1974). A cluster analysis method for grouping means in the analysis of variance. Biometrics 30, 507–512. doi: 10.2307/2529204
Senguttuvel, P., Sravanraju, N., Jaldhani, V., Divya, B., Beulah, P., Nagaraju, P., et al. (2021). Evaluation of genotype by environment interaction and adaptability in lowland irrigated rice hybrids for grain yield under high temperature. Sci. Rep. 11:15825. doi: 10.1038/s41598-021-95264-4
Senthilkumar, K. (2022). Closing rice yield gaps in Africa requires integration of good agricultural practices. Field Crop Res. 285:108591. doi: 10.1016/j.fcr.2022.108591
Singh, C., Tiwari, S., Gupta, V. K., and Singh, J. S. (2018). The effect of rice husk biochar on soil nutrient status, microbial biomass and paddy productivity of nutrient poor agriculture soils. CATENA. 171, 485–493. doi: 10.1016/j.catena.2018.07.042
Sitaresmi, T., Hairmansis, A., Widyastuti, Y., Rachmawati, S. U., Wibowo, B. P., Widiastuti, M. L., et al. (2023). Advances in the development of rice varieties with better nutritional quality in Indonesia. J. Agric. Food Res. 12:100602. doi: 10.1016/j.jafr.2023.100602
Solaiman, Z. M., Blackwell, P., Abbott, L. K., and Storer, P. (2010). Direct and residual effect of biochar application on mycorrhizal root colonisation, growth and nutrition of wheat. Aust. J. Soil Res. 48, 546–547. doi: 10.1071/SR10002
Statistics Indonesia (2018). Executive summary 2018 harvested area and Rice production in Indonesia. Statistics Indonesia: Badan Pusat Statistik.
Statistics Indonesia (2021). The 2021 analysis of Paddy productivity in Indonesia (the results of crop cutting survey). Statistics Indonesia: Badan Pusat Statistik.
Suryanto, P., Faridah, E., Nurjanto, H. H., Putra, E. T. S., Kastono, D., Handayani, S., et al. (2022). Short-term effect of in situ biochar briquettes on nitrogen loss in hybrid rice grown in an agroforestry system for three years. Agronomy 12:564. doi: 10.3390/agronomy12030564
Suryanto, P., Kurniasih, B., Faridah, E., Nurjanto, H. H., Rogomulyo, R., Handayani, S., et al. (2020a). Influence of furrow with organic material and chromolaena odorata compost on upland rice productivity in an agroforestry system with melaleuca cajuputi. Biodiversitas 21, 780–791. doi: 10.13057/biodiv/d210246
Suryanto, P., Taryono, S., Kastono, D., Putra, E. T. S., Handayani, S., Widyawan, M. H., et al. (2020b). Assessment of soil quality parameters and yield of rice cultivars in Melaleuca cajuputi agroforestry system. Biodiversitas J. Biol. Diver. 21, 3463–3470. doi: 10.13057/biodiv/d210807
Taghizadeh-Toosi, A., Clough, T. J., Sherlock, R. R., and Condron, L. M. (2012). Biochar adsorbed ammonia is bioavailable. Plant Soil 350, 57–69. doi: 10.1007/s11104-011-0870-3
Tardieu, F., Parent, B., Caldeira, C. F., and Welcker, C. (2014). Genetic and physiological controls of growth under water deficit. Plant Physiol. 164, 1628–1635. doi: 10.1104/pp.113.233353
Taryono, T. (2022). Tanggapan Kacang Hijau (Vigna Radiata (L.) R. Wilczek) terhadap Pemberian Mikoriza dan Kompos Lumpur Pengolahan Limbah Susu. Vegetalika 12, 183–197. doi: 10.22146/veg.78653
Terjung, W. H., Hayes, J. T., Ji, H., Todhunter, P. E., and O’Rourke, P. A. (1985). Potential paddy rice yields for rainfed and irrigated agriculture in China and Korea. Ann. Assoc. Am. Geogr. 75, 83–101. doi: 10.1111/j.1467-8306.1985.tb00060.x
Van Ittersum, M. K., Cassman, K. G., Grassini, P., Wolf, J., Tittonell, P., and Hochman, Z. (2013). Yield gap analysis with local to global relevance—a review. Field Crop Res. 143, 4–17. doi: 10.1016/j.fcr.2012.09.009
Varela Milla, O., Rivera, E. B., Huang, W.-J., Chien, C., and Wang, Y.-M. (2013). Agronomic properties and characterization of rice husk and wood biochars and their effect on the growth of water spinach in a field test. J. Soil Sci. Plant Nutr. 13, 251–266. doi: 10.4067/s0718-95162013005000022
Wang, D., Li, C., Parikh, S. J., and Scow, K. M. (2019). Impact of biochar on water retention of two agricultural soils–a multi-scale analysis. Geoderma 340, 185–191. doi: 10.1016/j.geoderma.2019.01.012
Warnock, D. D., Lehmann, J., Kuyper, T. W., and Rillig, M. C. (2007). Mycorrhizal responses to biochar in soil - concepts and mechanisms. In Plant and soil (Vol. 300, doi: 10.1007/s11104-007-9391-5
Warnock, D. D., Mummey, D. L., McBride, B., Major, J., Lehmann, J., and Rillig, M. C. (2010). Influences of non-herbaceous biochar on arbuscular mycorrhizal fungal abundances in roots and soils: results from growth-chamber and field experiments. Appl. Soil Ecol. 46, 450–456. doi: 10.1016/j.apsoil.2010.09.002
Welham, S. J., Gezan, S. A., Clark, S. J., and Mead, A. (1990). Statistical methods in biology: Design and analysis of experiments and regression. Lowa: CRC Press Ames.
Yan, W., Hunt, L. A., Sheng, Q., and Szlavnics, Z. (2000). Cultivar evaluation and mega-environment investigation based on the GGE biplot. Crop Sci. 40, 597–605. doi: 10.2135/cropsci2000.403597x
Yan, W., Kang, M. S., Ma, B., Woods, S., and Cornelius, P. L. (2007). GGE Biplot vs. AMMI analysis of genotype-by-environment data. Crop Sci. 47, 643–653. doi: 10.2135/CROPSCI2006.06.0374
Ye, S., Peng, B., and Liu, T. (2022). Effects of organic fertilizers on growth characteristics and fruit quality in pear-jujube in the loess plateau. Sci. Rep. 12:13372. doi: 10.1038/s41598-022-17342-5
Ye, L., Zhao, X., Bao, E., Li, J., Zou, Z., and Cao, K. (2020). Bio-organic fertilizer with reduced rates of chemical fertilization improves soil fertility and enhances tomato yield and quality. Sci. Rep. 10:177. doi: 10.1038/s41598-019-56954-2
Yoshida, H., and Horie, T. (2010). A model for simulating plant N accumulation, growth and yield of diverse rice genotypes grown under different soil and climatic conditions. Field Crop Res. 117, 122–130. doi: 10.1016/j.fcr.2010.02.007
Yoshida, H., Horie, T., Nakazono, K., Ohno, H., and Nakagawa, H. (2011). Simulation of the effects of genotype and N availability on rice growth and yield response to an elevated atmospheric CO2 concentration. Field Crop Res. 124, 433–440. doi: 10.1016/j.fcr.2011.07.016
Zhang, Y., Wang, J., and Feng, Y. (2021). The effects of biochar addition on soil physicochemical properties: a review. In Catena 202:105284. doi: 10.1016/j.catena.2021.105284
Zhu, X., Chen, B., Zhu, L., and Xing, B. (2017). Effects and mechanisms of biochar-microbe interactions in soil improvement and pollution remediation: a review. Environ. Poll. 227, 98–115. doi: 10.1016/j.envpol.2017.04.032
Zoghi, Z., Hosseini, S. M., Kouchaksaraei, M. T., Kooch, Y., and Guidi, L. (2019). The effect of biochar amendment on the growth, morphology and physiology of Quercus castaneifolia seedlings under water-deficit stress. Eur. J. For. Res. 138, 967–979. doi: 10.1007/s10342-019-01217-y
Zraibi, L., Chaabane, K., Berrichi, A., Sbaa, M., Badaoui, M., Zarhloule, Y., et al. (2015). Assessment of the agronomic value of the sludge compost for the wastewater treatment plant from Nador city. J. Mater. Environ. Sci. 6, 2975–2985
Keywords: biochar, organic fertilizer, soil management, upland rice, rainfed agroecosystem, yield gap
Citation: Santosa YT, Kurniasih B, Alam T, Handayani S, Supriyanta, Ansari A and Taryono (2024) Investigating the dynamics of upland rice (Oryza sativa L.) in rainfed agroecosystems: an in-depth analysis of yield gap and strategic exploration for enhanced production. Front. Sustain. Food Syst. 8:1384530. doi: 10.3389/fsufs.2024.1384530
Edited by:
Tibebu Kassawmar, Addis Ababa University, EthiopiaReviewed by:
Salar Farhangi-Abriz, Cotton Research Institute of Iran, IranFarzad Rassaei, Islamic Azad University, Isfahan, Iran
Copyright © 2024 Santosa, Kurniasih, Alam, Handayani, Supriyanta, Ansari and Taryono. This is an open-access article distributed under the terms of the Creative Commons Attribution License (CC BY). The use, distribution or reproduction in other forums is permitted, provided the original author(s) and the copyright owner(s) are credited and that the original publication in this journal is cited, in accordance with accepted academic practice. No use, distribution or reproduction is permitted which does not comply with these terms.
*Correspondence: Taryono, dGFyaW9ubzYwQHVnbS5hYy5pZA==