- Earth and Life Institute - Applied Microbiology (ELIM), Université Catholique de Louvain (UCLouvain), Louvain-la-Neuve, Belgium
Currently, it seems inconceivable to dispute the major role of microorganisms in human health or insects with endosymbionts. Although microbial endophytes were discovered long ago, little is known about the roles of plant-associated microorganisms. Some endophytes are horizontally transmitted, whereas others are seed-borne; together, they influence plant health. Beneficial endophytes can promote plant growth and yield by increasing plant resistance to biotic and abiotic stresses. Recently, the tools available to study the phytobiome have much improved, opening doors for a better understanding of the fascinating interactions taking place at the plant level. This review redefines the conceptual framework for “endophyte” and “endophytome,” focusing on the intricate dynamics of bacterial endophytomes. Systematically examining the formation pathways and profiling endophytes allows for a comprehensive exploration of the intricate dynamics governing plant-microbe interactions. Additionally, the assessment of how endophytomes are influenced by both biotic and abiotic factors provides essential insights into the adaptability and resilience of plant-associated microorganisms. Our comprehensive analysis integrates genomic insights with environmental considerations, offering a nuanced perspective on the functional roles of bacterial endophytomes. Therefore, a new, inclusive definition is essential to accurately represent the complexity of interactions within the plant microbiome as well as having the whole picture of associated concepts.
Introduction
Plants naturally host distinct microbial communities outside and inside their tissues in the phyllosphere (i.e., air-plant interface), rhizosphere (i.e., root-soil interface), and endosphere (i.e., internal tissues of the plant). Microorganisms associated with the endosphere, known as endophytes, directly influence plant metabolism and plant cells. Several studies have highlighted the benefits of endophytes in improving plant health (Harman et al., 2021) by revealing their ability to enhance nutrient uptake, disease resistance, stress tolerance, conserve water, and promote soil health, which contributes to reducing environmental impacts, increasing productivity, and long-term sustainability in agriculture (Hardoim et al., 2008; Compant et al., 2010; Hardoim et al., 2015). Therefore, harnessing these natural benefits as biocontrol agents can reduce environmental impacts of pest management, offer targeted pests and disease control, and enhance agricultural system resilience.
In endophyte studies, conventional methods often entail isolating bacteria from culture media after surface disinfection, followed by reinoculation into plants. A critical issue with this approach is its heavy reliance on cultivating endophytes in artificial culture media, despite the majority of the microbial diversity in natural environments remaining unculturable. Additionally, the surface disinfection step used to isolate endophytes introduces biases limiting the recovered isolates’ diversity. Therefore, the isolated strains might not accurately represent the full diversity of plant-associated endophytes. Furthermore, reinoculation of endophytes from one plant into another of the same species, as practiced in the conventional approach, disrupts natural host-specific interactions between endophytes and their host plants. These knowledge gaps hamper our ability to understand the ecological significance and functional potential of endophytes fully (Hardoim et al., 2015; Compant et al., 2019; Papik et al., 2020).
Given these limitations, there is an urgent need for a timely review and re-evaluation of the conventional methods employed in endophyte research. Emerging techniques such as metagenomics, metatranscriptomics, and single-cell sequencing can provide valuable insights into the diversity, functionality, and dynamics of endophytes without the constraints of cultivation-based methods. A more effective approach would be directly examining the endophytic communities to evaluate how abiotic and biotic stressors influence their dynamics and evolution. Rapid progress in sequencing techniques has enabled the in situ study of these endophytic communities, thereby advancing the understanding of their functional roles in plants. Although progress has been made on plant endophytes, there is still a long way to go to achieve what has been done so far on human microbiota (Gilbert et al., 2018).
Here, we discuss the multifaceted nature of plant-endophyte interactions, redefine the concept of endophytes, explore the formation of the bacterial endophytome, examine the existence of a bacterial endophytome profile, analyze the factors driving variation in endophyte communities. This will provide a conclusion and future perspectives on the topic and highlight how understanding the interactions between plants and endophytes can contribute to sustainable agriculture.
Plant-endophyte multifaceted relations
Back to the roots—defining endophyte
The term ‘endophyte’ is derived from the Greek words ‘endon’ meaning ‘within’ and ‘phyton’ meaning ‘plant’. The term was coined by De Bary et al. (1866) referring to the presence of ‘bacteria’ living inside a fern called Ophioglossum. At that time, the term ‘bacteria’ was used to encompass all living organisms, including those living inside a plant.
Endophytes have been defined thereafter in different ways, not always including the full range of living organisms able to reside within their host plants. Petrini (1961) proposed restricting the definition to ‘all organisms inhabiting plant organs that, at some time in their life, can colonize internal plant tissues without causing apparent harm to the host’. Several other definitions have been proposed, often restricting endophytes to a group of organisms (e.g., fungi or bacteria), symbiotic and/or commensal microorganisms, or facultative and/or mandatory microorganisms (Cabral et al., 1993; Quadt-Hallmann et al., 1997; Rosenblueth and Martínez-Romero, 2006; Hardoim et al., 2015; de Medeiros Azevedo et al., 2021).
Recent technological advances, facilitated by metagenomic studies and drastic reduction in sequencing costs, have provided a much better view of the diversity and the type of microorganisms associated with plants, with emerging terms like ‘phytobiome’ referring to the collective community of microorganisms, including bacteria, fungi, archaea, viruses, and other microbes, that interact with plants within a specific environment. It encompasses a plant-associated microbiome that includes both endophytic and rhizospheric microorganisms. The term ‘holobiome’ is defined as the collective assemblage of all organisms, including plants, animals, and microorganisms, as well as their genetic material, that coexist within a specific ecological niche or habitat. Redefining the term ‘endophytome’ with regard to these newly defined terms is also necessary for the precision, clarity, and elucidation of the complex interactions and synergistic relationships between organisms, as well as their combined contributions to ecosystem functioning, health, and resilience.
Several plant-associated microbes may sometimes cause disease but stay associated with their host plants simply as commensals. For example, Xylella fastidiosa has been associated with more than 600 asymptomatic host plants (Delbianco et al., 2021). Similarly, Clavibacter and Curtobacterium are often associated with asymptomatic plants, although some species are dangerous plant pathogens (Eichenlaub and Gartemann, 2011; Bulgari et al., 2014). Using a metagenomic approach to search for plant viruses has revealed the presence of numerous viral sequences and viruses that do not cause diseases (Roossinck, 2010). Some plant pathogens, individuals of the same species, or even strains, are also found in alternate hosts, where they do not cause diseases (Salvaudon et al., 2005). What drives the shift of such organisms along the ‘parasite–mutualist continuum’ has yet to be explained (Drew et al., 2021). Dependent on the host-microorganism association, excluding the ‘pathogen’ from the endophyte definition is impossible considering these examples.
To fully capture the complexity and diversity of endophytic organisms and their relationships with plants and elucidate the complex network of interactions and dependencies in endophytic microbial populations in plants, there is an urgent need to return to the original definition proposed by De Bary et al. (1866). As our understanding of endophytes continues to evolve, it is essential to develop a comprehensive and inclusive definition encompassing all aspects of their ecology and biology.
Based on the definition of the human microbiome, we propose to define the “endophytome” as a collective community of endophytes, including bacteria, fungi, archaea, viruses, and other microbes, that inhabit plants as obligatory or facultative organisms that are vertically or horizontally transmitted. The endophytome is composed of endophytes that may interact dynamically with plants through (Kogel et al., 2006; Buée et al., 2009; Agler et al., 2016; Berendsen et al., 2018; Papik et al., 2020; Swanson et al., 2022; Figure 1):
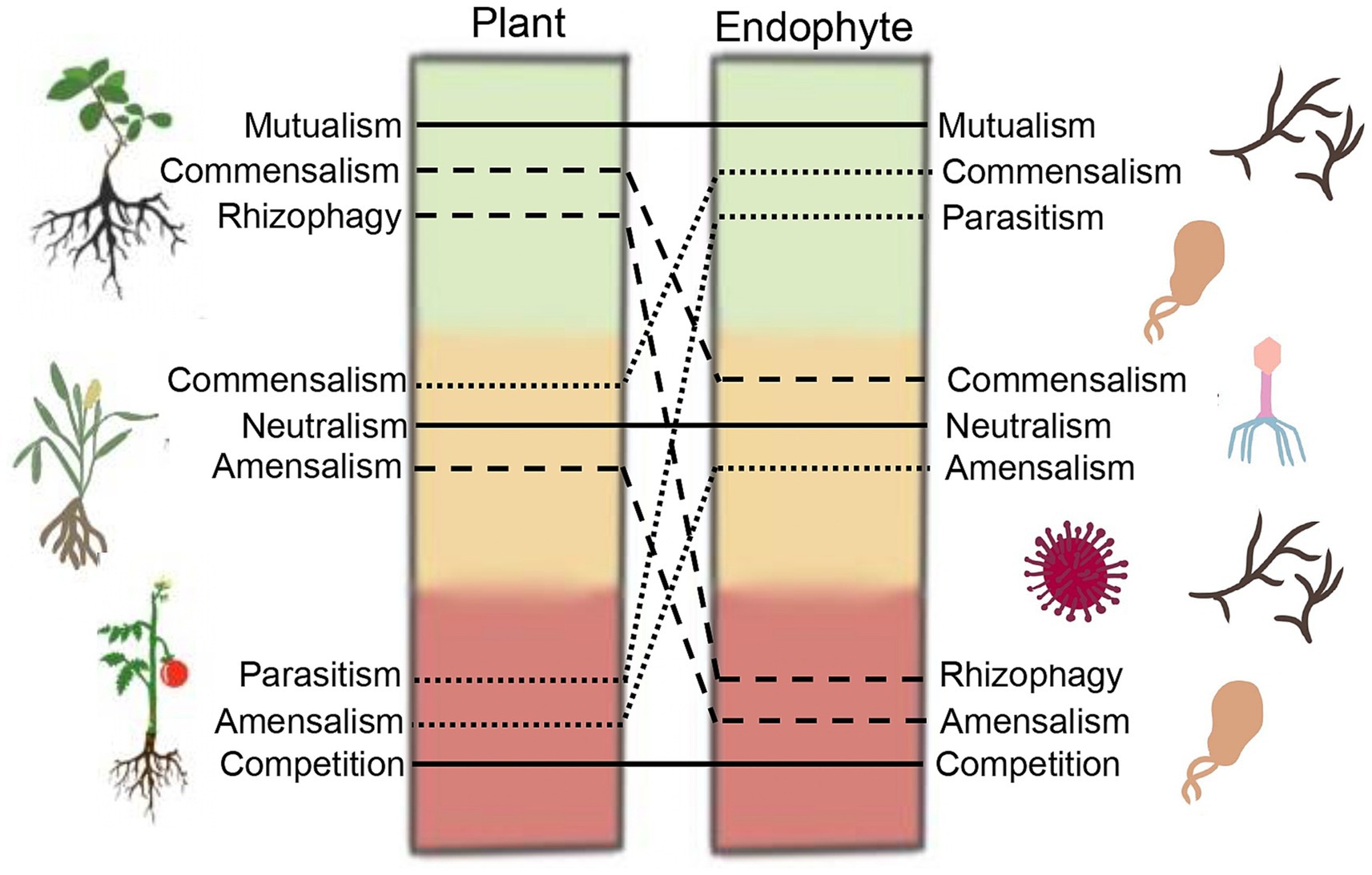
Figure 1. Theoretical framing of interactions between endophytes and host plants. Some interactions are more evident than others and therefore more studied (e.g., mutualism). Others are more complex to represent or even name (e.g., neutralism). Mutualism—mutually beneficial interaction between endophyte and its host plant. Both partners derive advantages and receive reciprocal benefits. Parasitism/“rhizophagy”—only one actor benefits. This can lead to plant disease or plants can benefit microorganisms without providing any advantages. Commensalism—beneficial for one agent and safe for another. Neutralism—no influence on either of the partners. Competition—negatively affects both partners. Amensalism—one organism is negatively affected or inhibited, while the other organism remains unaffected. A given endophyte may develop different interactions with its host during its life cycle. The two central rectangles show the effect of the interaction on the plant (left) and the endophyte (right). Green, yellow or red represent beneficial, neutral or harmful effects of the interaction. Each line represents a type of interaction.
Mutualism—mutually beneficial relationships or interactions between endophytes and their host plants, in which both partners derive advantages and receive reciprocal benefits. Example: Arbuscular mycorrhizae fungi (from Glomeromycota phylum) form structures called arbuscules inside the plant root cells, facilitating carbohydrates exchange and plant ability to absorb water and nutrients enhancement (Stürmer and Kemmelmeier, 2021). Recognized as a prominent endophyte model, the strain Gluconacetobacter diazotrophicus PAL5 employs a multifaceted strategy to bolster plant growth. Its repertoire includes biological nitrogen fixation, the synthesis of siderophores and antimicrobial substances, and the facilitation of mineral nutrient solubilization (Saravanan et al., 2008; De Oliveira et al., 2016). PAL5 demonstrates remarkable resilience, exhibiting improved plant growth even under challenging conditions such as salt, drought, and low nitrogen stress (De Oliveira et al., 2016; Filgueiras et al., 2020; Tufail et al., 2021). Studies have elucidated the bacterium’s adaptive responses to salt stress, involving morphological adjustments, cellular viability modifications, and significant proteomic alterations. The pivotal role of the DegP protease in stress tolerance has been underscored, shedding light on molecular mechanisms crucial for bacterial adaptation to environmental pressures (Leandro et al., 2021). Furthermore, PAL5’s contribution to plant colonization, particularly in rice roots, has highlighted the indispensable roles of superoxide dismutase and glutathione reductase. These enzymes play critical roles in defending the bacterium against oxidative stress, thereby fostering successful symbiosis with host plants and enhancing overall stress tolerance and health (Alquéres et al., 2013). Availability of nitrogen often limiting plant growth, intensive research on bacteria fixing nitrogen was conducted (Lima et al., 1987; Boddey, 1995). Among those bacteria, Azoarcus sp. strain BH72 was often used as a model (Hurek and Reinhold-Hurek, 2003). Azoarcus is a diazotrophic endophyte usually colonizing rice or grass roots mainly studied for its ability to fix nitrogen, its roots colonization process (Hurek and Reinhold-Hurek, 2003; Reinhold-Hurek and Hurek, 2007; Krause et al., 2017; Chen et al., 2020; Plucani do Amaral et al., 2023).
Parasitism/ “rhizophagy”—where only one actor benefits. This interaction can either lead to plant disease or the plant can benefit from the microorganisms without providing any advantages. Example: (1) Rhizophagy is a mechanism where plant can internalize and digest nutrients from symbiotic microbes (bacteria and fungi; White et al., 2018). (2) The genus Xanthomonas often are plant associated bacteria, collectively causing diseases on hundreds of host plants, including crops and ornamental plants (Mansfield et al., 2012; Peduzzi et al., 2022).
Commensalism—where it is beneficial for one agent and safe for the other. Example: (1) Fungal endophyte Epichloë residing within the tissues of grass can produce secondary metabolites, such as alkaloids beneficial for plant host (Bastias et al., 2017). (2) The protist Polymyxa betae, is a potential vector of sugar beet viruses. When free of virus, it alleviates plant defense response, playing hide-and-seek with sugar beet and allowing for their mutual development (Desoignies and Legreve, 2011; Decroës et al., 2022).
Competition—where both partners are negatively affected by the interaction. Example: Xylella fastidiosa is a bacterium that colonizes the xylem vessels, the water-carrying channels in the plant. It depends on the plant for its nutrition and survival. When it rapidly kills the host plant, the source of nutrients on which the bacterium depends is rapidly exhausted. This can lead to a significant halt or slowdown in bacterial growth (Landa et al., 2022).
Neutralism—where the interaction does not influence either partners.
Amensalism—where one organism is negatively affected or inhibited, whereas the other remains unaffected.
These last two interactions are difficult to illustrate concerning the endophytome due to lack of knowledge and should only be considered as theoretical. As endophytes are present within the plant for at least part of their cycle, it would be difficult to imagine that no benefits would result from these interactions.
A given endophyte may develop different interactions with its host during its life cycle and a “healthy” endophytome refers to microbiome presence in absence of any disease and shows a large degree of diversity that may be shaped by the host plant (genera, species), the stage of development, and the environment. The endophytome can also be characterized as a functional core or a complement of metabolic and other molecular functions that are performed by the microbiome with a plant part but are not necessary provided by the same organisms in different species/ individual as seen in human microbiome (Lloyd-Price et al., 2016).
The following sections focus on bacterial endophytomes. Our focus will now shift toward unraveling the entry pathways of bacterial endophytes and exploring whether transmission occurs through vertical or horizontal pathways. We will explore the drivers of the diversity and composition of bacterial endophytes. By unraveling the mystery of the bacterial endophytome, we aimed to gain deeper insights into its functional roles and potential applications in agriculture and ecosystem management.
Where does bacterial endophytome come from?
The endophytome comprises seed-transmitted microorganisms and microorganisms from the environment. Endophytes colonize the host vertically from parent to offspring, mainly via seed or vegetative propagation, horizontally through the environment, or in mixed modes (Bright and Bulgheresi, 2010; Figure 2).
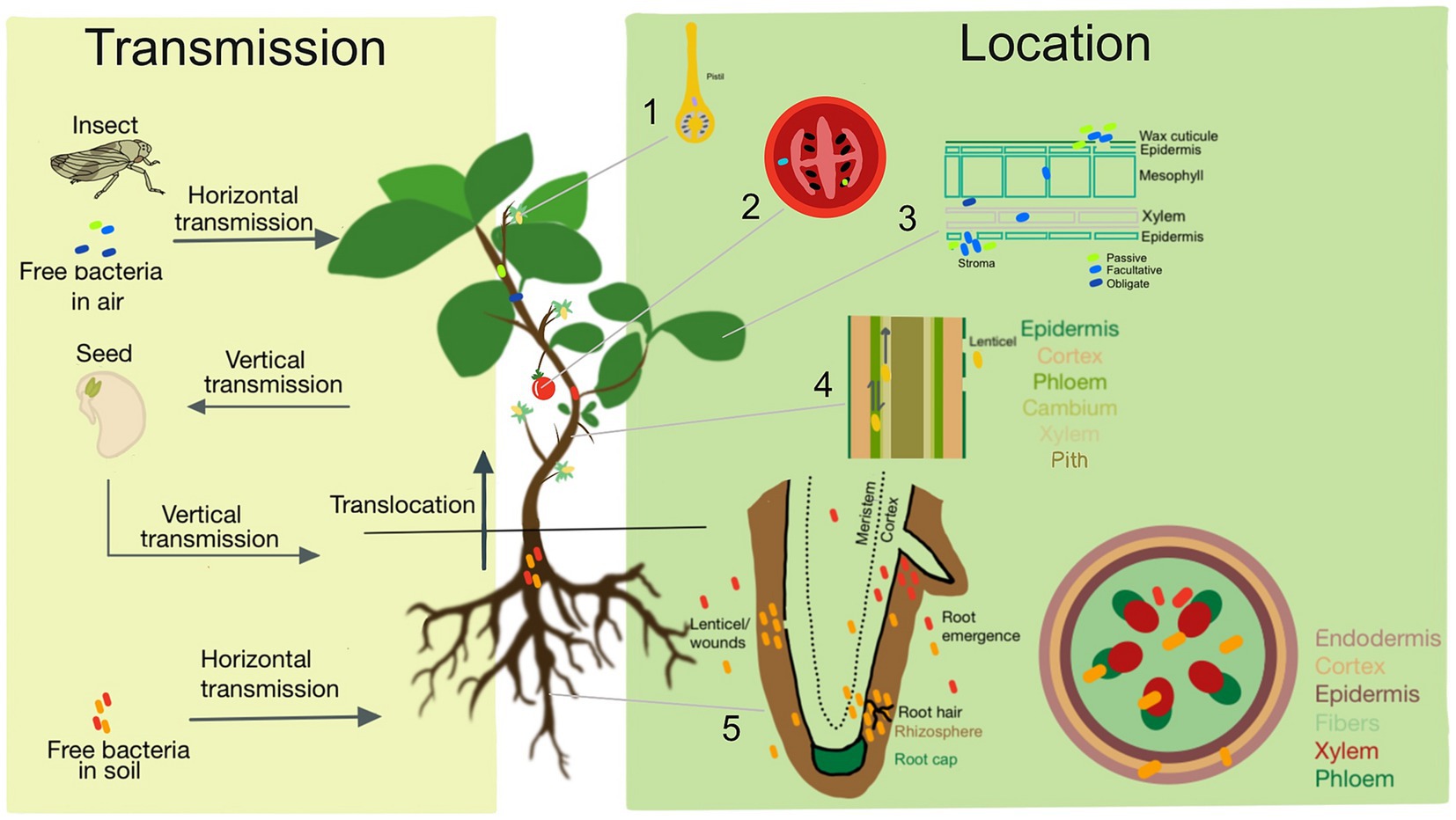
Figure 2. Types of endophyte transmission and endophyte location in plants. The gray arrows indicate different types of transmission of endophytes in the host plant: horizontally and vertically. The endophytes can move through translocation to reach different locations within the plant: the anthosphere (1), the carposphere (2), the phyllosphere (3), the caulosphere (4), and the rhizosphere (5).
Vertical pathway: This pathway involves the transmission of bacteria through seeds or vegetative propagation (Cankar et al., 2005; Mastretta et al., 2009; dos Santos et al., 2018). Endophytes present in the seeds can be acquired from pollen, flowers, fruits, or the plant’s soil. Bacteria reach the reproductive organs from the seed via xylem vessels or the shoot apical meristem and differentiate into reproductive organs (Frank et al., 2017). Most vertically transferred bacteria must spend their entire lives inside the host, on which they depend to survive. Vertical transmission has been identified as a factor that helps align the interests of mutualists, suggesting that symbiotic endophytes transferred vertically are mutualists and likely provide an indispensable function (Herre et al., 1999). Indeed, seed endophytes have been shown to benefit their hosts by releasing seeds from dormancy, facilitating germination, protecting against pathogens, and promoting the growth of seedlings (Puente et al., 2009). Typical members include the genera Acinetobacter, Bacillus, Micrococcus, Paenibacillus, Pantoea, and Pseudomonas (Truyens et al., 2015).
Horizontal pathway: Horizontal transfer is mainly realized by the soil-to-root route because roots are considered the main entry point for microorganisms. Indeed, bacteria can penetrate the endodermis, the innermost layer of the root cortex, through root hair channels, cracks in the epidermis, cell-to-cell junctions, or through root cap cells that detach as the roots grow (Hardoim et al., 2008). Once bacteria reach the endodermis, they must pass through the Casparian strip, a cell wall thickening that prevents the free diffusion of molecules and ions between the root cortex and vascular tissues. Studies suggest that some bacteria can pass through the Casparian strip by inducing the expression of endodermal transporters, such as aquaporins, which allow bacteria to cross the endodermal barrier (Bulgarelli et al., 2013). Additionally, some bacteria secrete cell wall-degrading enzymes or manipulate host cell signaling pathways to facilitate their entry into the endodermis. Once in the endodermis, endophytes mainly move into the xylem vascular system, allowing systemic colonization of internal plant compartments (James et al., 2002). They can colonize xylem vessels and perforation plates between these vessels (James et al., 2002; Compant et al., 2005). In addition to this main transport route, other endophytes also colonize intercellular spaces (Hardoim et al., 2015). This pathway can be considered passive or active (Hardoim et al., 2008) depending on the penetration mode within the plant. An active penetration pathway can be mediated by the attachment of bacterial endophytes to plant cells using their flagellum and the secretion of metabolites that facilitate the penetration process (mostly exopolysaccharides [EPS] and cell wall degrading enzyme; Kandel et al., 2017; Pinski et al., 2019; Ullah et al., 2019). To some extent, root exudates and rhizodeposits can attract certain types of microorganisms in planta (Hallmann et al., 1997; Germida et al., 1998; Sessitsch et al., 2002; Cocking, 2003; Compant et al., 2010; Hardoim et al., 2011; dos Santos et al., 2018). Bacterial endophytes can also be horizontally transmitted to the phyllosphere level (Redford et al., 2010; Kembel et al., 2014; dos Santos et al., 2018) from dust, rainwater, or surrounding pollinators. Hydathodes, lenticels, and stomata are the primary gateways for endophytes in these environments (Compant et al., 2010; Hardoim et al., 2011; Frank et al., 2017). Plants can close or open their stomata upon contact with different microbial antigens (Gimenez-Ibanez et al., 2017; Melotto et al., 2017), which suggests that the plant immune system plays a role in the selection of microbes entering the endosphere (Fesel and Zuccaro, 2016). However, some foliar endophytes also control stomatal opening (Rho et al., 2018). Plant- and sap-feeding insects can be endophyte vectors. These insects have piercing–sucking mouthparts and can directly puncture the phloem and/or xylem cells that transmit the endophytes (Frank et al., 2017). Finally, only compatible endophyte-plant interactions can successfully colonize the endosphere, which explains the reduced number of endophytes compared with rhizospheric bacteria (Hardoim et al., 2011; Khare et al., 2018). An analysis of all sequenced 16S rRNA gene available in the International Nucleotide Sequence Database Collaboration identified four phyla containing more than 96% sequenced endophytic prokaryotes: Proteobacteria (54%), Actinobacteria (20%), Firmicutes (16%), and Bacteroidetes (6%). Most endophytic bacteria belong to the class Gammaproteobacteria, including Acinetobacter, Enterobacter, Pantoea, Pseudomonas, Stenotrophomonas, and Serratia (Hardoim et al., 2011).
Toward an endophytome profile?
The endophytome comprises various endophytes characterized by genomic differences compared with free-living microorganisms. However, these differences can be difficult to describe because some are facultative (Del Orozco-Mosqueda et al., 2021). Ali et al. (2014) were the first to compare the genomes of endophytes and non-endophytes, thereby identifying the genes involved in secretion and transport activities, polymer degradation or modification, detoxification, and maintenance of redox potential. They suggested that these functions might play a role in endophytic behavior, allowing them to colonize plants.
This review presents the functional characteristics of endophytic bacteria and their involvement in plant colonization and propagation. The soil-to-root colonization steps were divided into: (1) detecting root exudates, (2) motility toward the plant, (3) adhering to root surfaces, (4) penetrating the root surface, and (5) colonizing the plant’s internal parts. Each of these stages is mediated by a variety of biomolecules that influence the expression of both bacterial and colonized plant genes (Pinski et al., 2019; Figure 3).
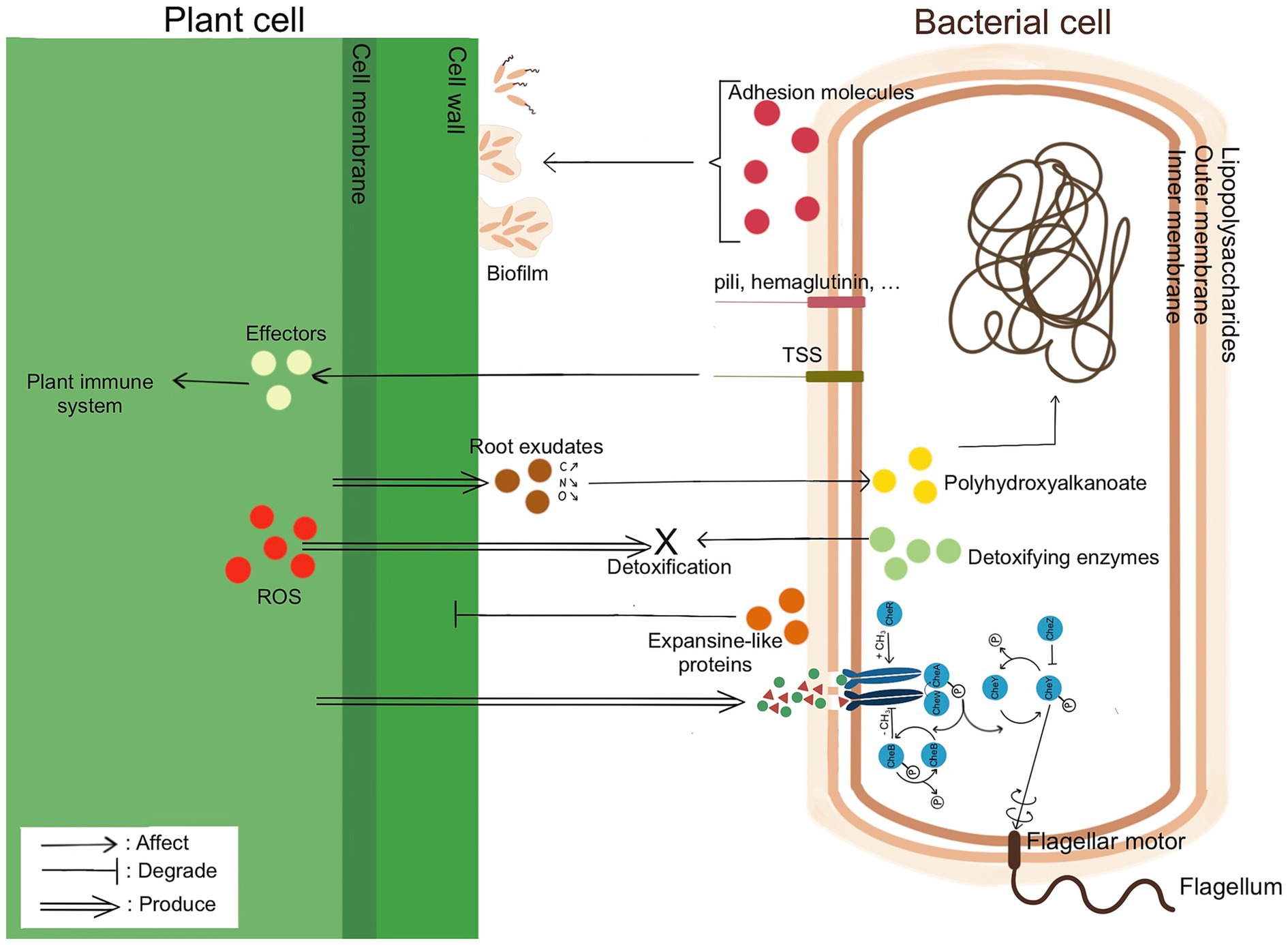
Figure 3. Principal drivers involved in bacterial endophyte colonization of plant host. Adhesion molecules () and hemagglutinin, curli protein, pili IV, and pili I (
) facilitate the attachment of endophytic microorganisms to the plant’s surfaces, promoting biofilm formation and providing a stable environment for colonization, nutrient exchange, and interactions within the plant tissues. Type 3 and 6 secretion systems (
) produce effectors (
), which play a role in manipulating the host plant’s cellular processes, suppressing immune responses, and promoting colonization. Plant cell wall degrading enzymes (
) enable endophytes to break down and penetrate the plant cell wall, facilitating colonization. Expansin-like proteins (
) promote cell wall loosening and expansion, allowing endophytes to access nutrients and establish symbiotic interactions within plant tissues. Detoxifying enzymes (
) can neutralize reactive oxygen species (
) generated by plants as a defense response and metabolize phytoalexins, aiding in the plant’s protection against oxidative damage and enhancing its resistance against pathogens or stressors. Root exudates (
) influence the production of polyhydroxyalkanoate (
), which affects the expression of specific genes involved in the colonization process, nutrient acquisition, and establishment of interactions with the host plant. Attractants (
) or repellents (
) play a role in endophyte motility through the action of methyl-accepting chemotaxis proteins (
) and chemoreceptors (
), which detect the presence of specific chemical signals released by plants, guiding endophytes toward attractive compounds or repelling them from harmful substances. The motility of endophytes is influenced by the differential expression and activity of methyl-accepting chemotaxis proteins and chemoreceptors, allowing them to navigate toward favorable environments or avoid hostile conditions within the plant.
Colonization by endophytic bacteria starts with the chemotaxis of free-living bacteria toward the roots, aided by methyl-accepting chemotaxis proteins (MCPs). These transmembrane sensors detect molecules and direct bacteria toward attractants or away from repellents (Balsanelli et al., 2016). The involvement of MCPs in plant colonization was demonstrated using inactivation mutants of Herbaspirillum seropedicae SmR1 and Azospirillum brasilense Sp7. In SmR1, one MCP is required to sense the rhizosphere and direct bacteria toward host-secreted compounds. In Sp7, the inactivation of another MCP results in impaired chemotaxis and colonization of plant roots (Greer-Phillips et al., 2004).
At the root surface, bacteria can attach through biofilms, which contain water, proteins, polysaccharides, eDNA, RNA, and ions. Mutants in various species, such as G. diazotrophicus PAL, have shown that other polymers, including EPS, glucomannan, and cellulose, also affect attachment and colonization. Adhesion is mediated by the flagella, pili, curli, and hemagglutinins. The upregulation of genes encoding filamentous hemagglutinin proteins suggests their involvement in root attachment (Barak et al., 2005; Williams et al., 2008; Monteiro et al., 2012; Ainelo et al., 2017). Bacterial surface components, particularly lipopolysaccharides (LPS), play crucial roles in early attachment and colonization. Mutations in rfbB or rfbC, which are involved in rhamnose biosynthesis, reduce the attachment to and colonization of maize roots by H. seropedicae SmR1, as well as the bacterium’s robustness against detergents, antibiotics, and phytohormones. Similarly, mutations in the rfbD gene of A. brasilense impair attachment and colonization efficiency. O-antigen ligase, involved in O-antigen biosynthesis, was upregulated during colonization, indicating its involvement in plant-endophyte interactions. Bacterial LPS binds to maize root lectin proteins leading to agglutination, which is impaired when the O-antigen ligase is inactive, resulting in reduced attachment and colonization efficiency (Jofré et al., 2004; Balsanelli et al., 2013).
After attachment to the root surface, bacterial endophytes enter the plant through natural openings or wounds or by producing plant cell wall-degrading enzymes (e.g., pectinase or cellulase) that facilitate entry and spread within plants. For example, Azoarcus sp. BH72 requires endoglucanase for rice root colonization and spreads to the aboveground parts, whereas Bacillus mycoides EC18 upregulates hydrolases and chitin-binding proteins in response to root exudates (Badri and Vivanco, 2009; Reinhold-Hurek and Hurek, 2011; Baetz and Martinoia, 2014).
During colonization, specific endophytic genes, such as genes encoding membrane-associated proteins belonging to the resistance nodulation and cell division (RND) efflux systems, actively contribute to successful establishment. Among them, the Membrane Fusion Protein (MFP) subunit of the RND family efflux systems has been shown to have a significant role in the successful colonization of the host plant, as observed in the case of the endophyte Erwinia amylovora in apple trees (Taghavi et al., 2010). Detoxification mechanisms such as glutathione synthesis and reductase-related genes have been identified as crucial factors for competent endophytes, as they protect their host against oxidative stress induced after host infection (Compant et al., 2010). Thus, these genes play a potential role in the protective response of endophytic bacteria to oxidative stress resulting from the infection of plant hosts (Alquéres et al., 2013).
After entering the plant, certain endophytes spread systemically throughout the plant, eventually reaching the flowers, fruits, and seeds through the xylem vessels of their host plants, taking advantage of their flagella and the plant’s transpiration stream (Compant et al., 2005).
Transport proteins, including various genes associated with the major facilitator superfamily (MFS) transporter system, play a crucial role in the endophytic lifestyle by facilitating the uptake of plant-synthesized nutrients. These proteins are essential for acquiring nutrients found internally within plants or released into the rhizosphere. Their primary function involves the exchange of diverse carbohydrates and amino acids, enabling efficient nutrient transfer between the endophyte and its host plant (Taghavi et al., 2010; Ali et al., 2014). Certain endophytic genes appear responsible for bacterial nutrition within the plant, including non-plant-cell-wall-breaking hydrolases found in the genomes of plant growth-promoting bacterial endophytes, such as Enterobacter spp. 638 and Serratia proteamaculans 568 (Taghavi et al., 2010). The widespread presence of these enzymes among different endophytes suggests their potential involvement in the versatile utilization of sugars, which could be a valuable characteristic of proficient endophytes.
Endophytic bacteria possess various secretion systems that differentiate them from free-living bacteria. Among these, the type VI secretion system (T6SS) appears to be involved in utilizing plant carbon sources (Del Orozco-Mosqueda et al., 2021). Bacterial endophytes commonly exhibit type I and II secretion systems (Reinhold-Hurek and Hurek, 2011), whereas type III and type IV secretion systems are predominantly found in pathogenic bacteria and are typically absent in endophytes (Downie, 2010). The type V secretion system, also known as an autotransporter, is primarily observed in endophytes. Additionally, T6SS may benefit plant-microbe interactions (Mattinen et al., 2008) and have been detected in certain bacterial endophytes (Reinhold-Hurek and Hurek, 2011).
Some groups of genes have been identified as conserved and potentially responsible for endophytic behavior, including various transcriptional regulators involved in metabolic adaptation and quorum sensing, such as AraC, FrmR, AsnC, LrgB, LysR, DeoR, and WrbA (Table 1). Many of these regulators play a role in modulating carbohydrate metabolism, which is crucial when cells enter the stationary phase of growth. The presence of these regulatory proteins suggests their likely function, specifically in the non-invasive breakdown of the plant cell wall, which occurs when endophytes meet plant hosts during infection. Additionally, some of these transcriptional regulators are involved in communication pathways that are vital for altering the behavior of an organism when it adapts to its physiology (Ali et al., 2014). The gene encoding a lysine biosynthesis enzyme, specifically diaminopimelate decarboxylase, along with the gene encoding a lysine exporter protein, may also be involved in the transition of the bacterium from a free-living state in the soil to an endophytic state within the plant (Maddocks and Oyston, 2008; Ali et al., 2014).
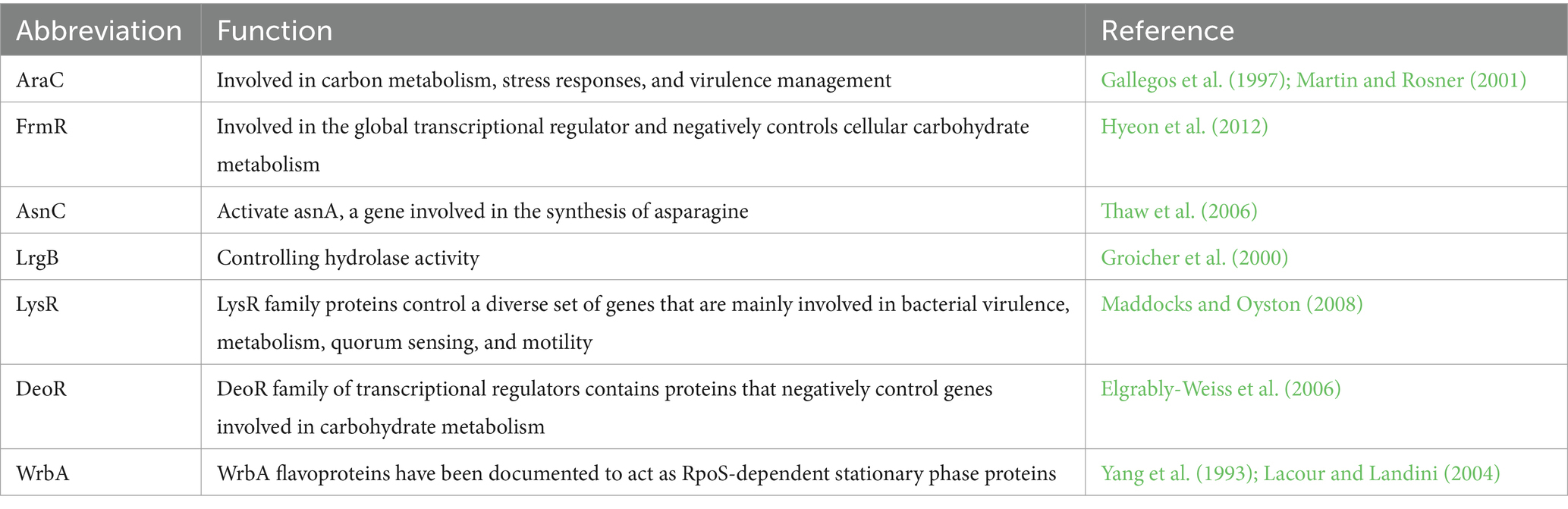
Table 1. Conserved classes of genes responsible for endophytic behavior, including various transcriptional regulators.
Endophyte community variation drivers
A holobiont is the assemblage of a host, its microbiome, and any other organism that contributes to the functioning of the host and forms an ecological niche through mutual interconnections (Vandenkoornhuyse et al., 2015). The evolutionary history of the holobiont may be shaped significantly by the response of microorganisms to environmental changes, as well as the host organism itself (Occhipinti, 2013; Vandenkoornhuyse et al., 2015; Rosenberg and Zilber-Rosenberg, 2016; Hassani et al., 2018). Endophytic bacteria are typically defined as individual microorganisms rather than communities, whereas plants are influenced mainly by microbial communities. A microbial community can be defined as an assemblage of co-occurring and potentially interacting microbes present in a defined habitat in space and time (Compant et al., 2010). In this section, we review the effects of biotic and abiotic stresses on these communities and their effects on plants.
During the colonization of the endosphere, osmotic pressure, oxygen availability, carbon sources, and pH of the environment change, and bacteria must quickly adapt (Singh et al., 2021). Soil type and plant development stage appear to be the most important drivers of bacterial endophytic communities, whose composition is influenced by biotic factors and abiotic factors (Figures 2, 4).
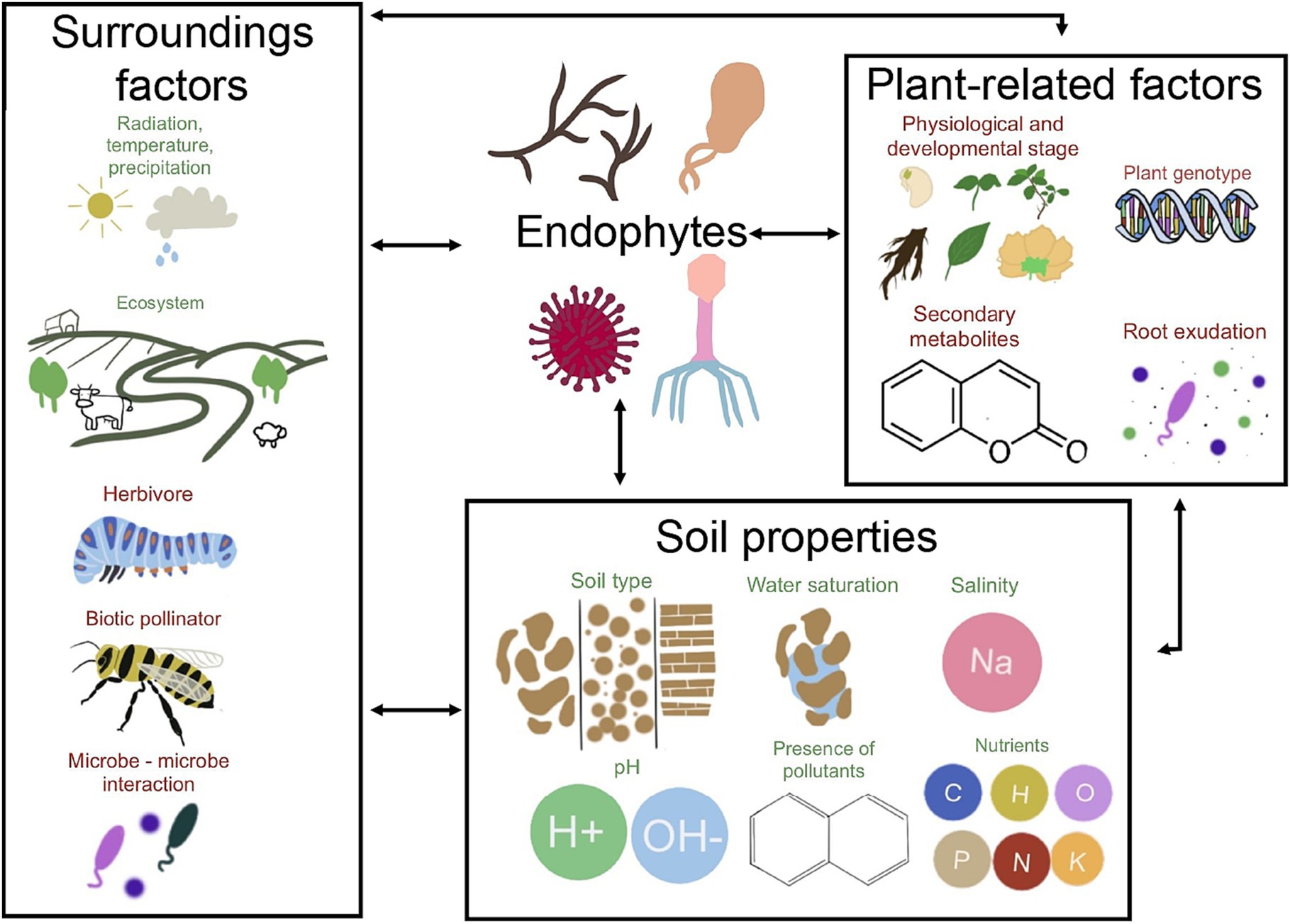
Figure 4. Drivers of endophyte community variation, categorized into biotic (red) and abiotic (green) factors. These drivers are further divided into three main categories: surroundings factors such as radiation, temperature, precipitation, ecosystem, herbivores, biotic pollinator or microbe-microbe interaction; plant-related factors such as physiological and developmental stage, plant genotype, secondary metabolites or root exudation; and soil properties such as the presence of pollutants, soil type, water saturation, nutrient availability, pH or salinity. Understanding the interplay between these drivers is crucial for comprehending the dynamics of endophyte communities and their responses to different ecological conditions.
Biotic factors include (1) plant genotype (Hardoim et al., 2011; Yu et al., 2015; Ding and Melcher, 2016), developmental, and physiological stage (Jin et al., 2014; Yang et al., 2017); (2) composition of the root exudate and secondary metabolites (Aulakh et al., 2001; Shaw et al., 2006; Zhang et al., 2006; Dennis et al., 2010; Philippot et al., 2013; Vandenkoornhuyse et al., 2015; Henning et al., 2016; Musilova et al., 2016; Sasse et al., 2018); (3) biotic pollination; and (4) microbe-microbe interactions (Vandenkoornhuyse et al., 2015; Rosenberg and Zilber-Rosenberg, 2016).
Plant genotype, developmental and physiological stage—Plant genotype is one of the main factors. Research on bacterial endophyte communities is facilitated by high-throughput sequencing techniques, especially for root and phyllosphere microbiota. Next-generation sequencing (NGS) has revealed differences in the taxonomic composition of phyllosphere bacterial endophyte communities depending on the nature of the host plant species. Therefore, the core seed microbiome depends on the host genotype (Saikkonen et al., 2012). In addition to this genotypic effect, different plant organs harbor distinctive bacterial endophytes, suggesting that plants play a role in structuring endophytic communities (Jin et al., 2014; Yang et al., 2017). These variations in bacterial endophyte communities can also be explained by different environmental sources or by their ability to colonize different niches in planta. The examples are listed in Table 2.
Root exudation—Plants can control variations in bacterial endophyte communities through root exudation. Plant exudate photosynthesis-derived compounds in the soil control the composition of microbial communities (Dennis et al., 2010; Philippot et al., 2013). Root exudate composition changes with plant species, cultivars, and developmental stages (Aulakh et al., 2001). For example, variation in phenolic compounds drives interactions between bacteria and plants (Shaw et al., 2006). Beneficial microbes are drawn to the root exudates because of their metabolites. Rhizospheric organisms use carbohydrates, lipids, amino acids, phenolics, phytosiderophores, and flavonoids as carbon sources (Badri and Vivanco, 2009). These metabolites modulate gene expression patterns in microorganisms to modify bacteria-host interactions (Mark et al., 2005; Yi et al., 2017). Due to the limited number of studies focusing on its impact on the composition of the bacterial endophyte community, the mechanisms underlying this influence are still poorly understood. Examples of the effects of root exudation on endophytic communities are listed in Table 2.
Plant secondary metabolites—Plant secondary metabolites (PSM), such as luteolin, quercetin, daidzein, and steviol glycosides (Papik et al., 2020), are another one of the most important factors influencing the structure and function of the endophyte community (Musilova et al., 2016; Sasse et al., 2018). PSM are a potential carbon source for rhizosphere and endophyte communities. They exhibit antimicrobial activity and induce the selection of microorganisms in the rhizosphere (Musilova et al., 2016). The more PSM are present in the rhizosphere, the higher the probability of microorganisms being capable of degrading pollutants because PSM can serve as primary substrates and/or enzyme inducers for the growth of microorganisms (Singer et al., 2003, 2004). Secondary metabolites can influence gene expression, modulate host immunity, alter primary and secondary metabolism, and morphology of the host plant, which can result in plant growth promotion, increased stress tolerance, or reduced rates of herbivory during endophyte colonization (Zhang et al., 2006; Henning et al., 2016). Microorganisms found in the soil are primarily influenced by the rhizosphere, which constitutes the first plant-influenced habitat they encounter. Plant metabolism profoundly affects the thin layer of soil surrounding the roots through the release of oxygen and secretion of exudates, including carbon-rich molecules and antimicrobial compounds. Consequently, the rhizospheres between roots and soil are highly dynamic environments, resulting in differentiated microbial rhizosphere and endosphere communities (Vandenkoornhuyse et al., 2015).
Microbe-microbe interactions—Microbe-microbe interactions play an important role in endophyte variation. Interspecies interactions can affect the diversity and productivity of communities. Even if often described to be rare, positive interactions between microorganisms of soil microbiome often occur in coculture primarily as parasistisms (18%), commensalisms (12%) and mutualisms (5%; Kehe et al., 2021). Microorganisms and host plants form a mutually inclusive ecosystem, which has recently been discussed regarding their mutual interconnections (Occhipinti, 2013; Vandenkoornhuyse et al., 2015). Endophytic bacteria can antagonize phytopathogens (Hu et al., 2009; Fürnkranz et al., 2012; Khalaf and Raizada, 2018; Zhao et al., 2018). Fungi and bacteria can interact in the endosphere, which is crucial for the development of endophytic communities. Identifying the major endophytic taxa of certain crops and clarifying their unique roles in plants would contribute significantly to developing the aforementioned agricultural applications of endophytes and deepen our knowledge of the microbial composition in the endosphere. Some examples of microbe-microbe interactions are listed in Table 2.
Abiotic factors influencing endophytic communities include (1) radiation and temperature (Redman et al., 2002; Ju et al., 2006; Nissinen et al., 2012); (2) precipitation and moisture (Rolli et al., 2015; Ullah et al., 2019); (3) soil type (Buyer et al., 1999; Hinsinger et al., 2009; Polivkova et al., 2018); (4) pH (Hayman and Tavares, 1985; Wang et al., 1993; Anyango et al., 1995; Lafay and Burdon, 1998; Cornelissen et al., 2011); (5) nutrients (Hermans et al., 2017); and (6) presence of pollutants (Vergani et al., 2017; Yergeau et al., 2018).
Radiation/temperature—Radiation and temperature affect endophytic communities, mostly by influencing host plant colonization (Redman et al., 2002; Ju et al., 2006; Nissinen et al., 2012). An important increase in temperature decreases shoot endophytic density because of reduced endophytic colonization (Pillay and Nowak, 1997). In contrast, a slight increase in temperature (+ 5°C) in temperate forests results in higher endophytic bacterial population (Rahman et al., 2021). It has been shown that climate change treatments, represented by elevated temperatures and/or increased CO2 concentrations shift leaf-associated bacterial communities (Ren et al., 2015) and could impact bacterial functions such N2 fixation (Nissinen et al., 2012; Rahman et al., 2021; Table 3).
Water and oxygen availability—precipitation, and soil moisture are also important factors affecting endophyte diversity. One common perturbation is drought stress, which impacts root bacterial communities directly by changing moisture availability and indirectly by altering soil chemistry and plant phenotypes. Many putative causes of endophytic bacterial community shifts have been hypothesized (Table 2). First, naturally selected bacteria are directly resistant to drought stress. Some physiological mechanisms improve drought tolerance, including sporulation and thick cell walls, which are primarily found in bacteria belonging to gram-positive phyla, such as Actinobacteria and Bacilli (Cherif et al., 2015; Tocheva et al., 2016). Differences in survival could also be explained by the ability to produce and accumulate osmolytes, such as amino acids (proline, glutamine, and glycine betaine) and carbohydrates (trehalose and ectoine). Gram-negative bacteria produce osmolytes only when they are constitutively produced (Schimel et al., 2007). Second, slow-growing microbes could be selected according to the nutrient-poor (less labile organic carbon and nitrogen) but oxygen-rich characteristics of drought environments. These characteristics favor oligotrophic bacteria with high metabolic activity, mostly gram-positive bacteria (Curiel Yuste et al., 2014; Hartmann et al., 2017). The third hypothesis is that drought stress may induce the production of various compounds by bacteria, thereby affecting community stability. A high antibiotic content was found in drought environments, probably produced by drought stress-tolerant bacteria that outcompete other bacteria for limited resources (Bouskill et al., 2016). Taxa selected by drought stress and plants can be in the rhizosphere or endosphere and improve drought stress resistance in plants by maintaining host functions and fitness (Rolli et al., 2015; Ullah et al., 2019). Several studies have reported a correlation between drought stress resistance in plants and a shift in their microbiome in response to stress (Naylor et al., 2017; Santos-Medellín et al., 2017; Fitzpatrick et al., 2018). Flooding also affects endophytic communities, favoring the growth of anaerobic and aerotolerant bacteria. In this environment, endophytic bacteria may play an essential role in fixing nitrogen as reported in rice (Ferrando and Fernández Scavino, 2015). Indeed, as oxygen irreversibly inhibits nitrogenase, N2 fixers are favored in oxygen-poor environment (Smercina et al., 2019; Table 3).
Soil type—Soil type is described by Hinsinger et al. (2009) as one of the major abiotic drivers of endophyte community diversity. Multiple studies have shown that soil type strongly influence the endophytic bacterial and fungal communities (Buyer et al., 1999; Polivkova et al., 2018; Hou et al., 2019; Adeleke et al., 2022). Soil fertility, physicochemical properties, pH, porosity, water and oxygen content and soil organic carbon are interlinked to influence soil microbial density and activity (Rahman et al., 2021). The primary features of the soil, such as water-holding capacity, cation-exchange capacity, hydraulic conductivity, oxygen diffusion and humidity directly or indirectly influence soil bacterial communities and, indirectly influence endophytic bacterial communities. The relationship between the soil characteristics and bacterial taxonomic groups reflects the ecological functions of these organisms (Hermans et al., 2017). For example, soil with a high clay content has smaller interparticle spaces and, therefore, a lower oxygen content, which favors nitrogen-fixing bacteria.
pH—pH has been shown to affect microbial communities in the rhizosphere, but its impact also extends to the endosphere (Hayman and Tavares, 1985; Wang et al., 1993; Anyango et al., 1995; Lafay and Burdon, 1998; Cornelissen et al., 2011). A slight change in pH promotes the growth of more adapted species by lowering the original community (Rahman et al., 2021; Table 3).
Pollutants—The presence of pollutants in the soil selects microorganisms capable of using and/or degrading them as sources of carbon and energy (Vergani et al., 2017; Yergeau et al., 2018). Heavy metals, naturally present in soils but also used in fertilizers, pesticides, and wastewater irrigation, explained a portion of the variability in the abundance of bacterial phyla and classes (Table 3).
Nutrients—Nutrients are essential for bacterial survival. The carbon-nitrogen ratio and the level of P may influence soil bacterial populations (Hermans et al., 2017).
Conclusion and future perspectives
This review aims to establish a refined and enhanced understanding of endophytes and endophytomes, considering the rapid technological advancements that have deepened our understanding of this concept. Additionally, we extensively examined the profile, colonization pathway, and various drivers responsible for the diversity and variation observed in the bacterial endophytome.
The urgent need for sustainable agriculture arises from the imperative to ensure global food security, mitigate environmental degradation, and build resilience in the face of climate change. Embracing sustainable practices is not merely an option but an essential strategy for safeguarding the well-being of present and future generations (Espinosa-Leal et al., 2018; Raza et al., 2019; Qaim, 2020; Delitte et al., 2021). The colonization of plants, animals, and humans by countless microorganisms has sparked a significant shift in the study of the effects of these microorganisms on the biology and well-being of their hosts (Drew et al., 2021). Numerous cases of microbial evolution causing transition across the ‘parasite-mutualist continuum’ have been reported both in human and plant health and will further emerge through research. For example, a healthy gut microbiome is now considered crucial for human growth, development, and immune system establishment, underscoring the importance of maintaining balance for optimal health (Kartjito et al., 2023). Recent data show that early-life gut microbiome development can protect against diseases linked to an imbalanced gut microbiome in later stages of life (Barone et al., 2023; Sabino et al., 2023). These findings support the idea that targeted therapies can restore the gut microbiome during infancy, potentially promoting long-term infant health (Charbonneau et al., 2017). Thus, modifying early colonization or addressing early-life gut dysbiosis may be strategies for enhancing well-being (Kapourchali and Cresci, 2020). The importance of microbiota in animal health has also been demonstrated in numerous studies, for example, in honeybees, where the genetic modification of a core gut bacterium improves resistance to viral infection (Leonard et al., 2020). In the future, engineering a microbiome or symbiont community via direct genetic modification of key transition loci in microbiome members could be used to improve human health. Future therapeutic and prophylactic modalities may be used to treat cancer and obesity (Sekirov et al., 2010).
In plant health, endophytes play a crucial role in alleviating both abiotic and biotic stresses. They enhance plant tolerance to adverse environmental conditions such as drought, salinity, and extreme temperatures. They stimulate the production of stress-related compounds and regulate plant hormone levels, thereby improving water and nutrient uptake efficiencies. Additionally, endophytes promote root development and enhance plant defense mechanisms against abiotic stressors (Khare et al., 2018; Ullah et al., 2019; Nadarajah, 2020; Singh et al., 2022). In response to biotic stress, endophytes shield against pathogens and herbivores by producing antimicrobial compounds, inducing systemic resistance, and activating plant defense pathways. These symbiotic microorganisms confer resistance to a wide range of pathogens, including bacteria, fungi, and nematodes, thereby ensuring plant health and productivity in the face of biotic challenges (Hardoim et al., 2008; Bulgarelli et al., 2013; Compant et al., 2019; Papik et al., 2020; Oukala et al., 2021). Overall, endophytes hold great promise for enhancing plant resilience and mitigating the negative impacts of abiotic and biotic stresses.
Drawing parallel to the human microbiota, there is a growing trend toward exploring endophytes in a similar manner. However, using a definition that targets only beneficial endophytes has prevented research from advancing as quickly as in the medical field. Moreover, studies of endophytes have been plagued by several limitations: (1) properties are studied in a single species or within closely related genotypes, but rarely across a taxonomically wide range of species; (2) the environmental conditions in which plant-endophyte interactions are investigated are often similar; (3) consortia are not often explored and bacterial and fungal endophytes are often considered separately (Elasri et al., 2001; Andreote et al., 2009; Hardoim et al., 2015). Consequently, our understanding of the modes of action of endophytes and their use in agriculture is limited.
The multiple interactions between endophytome, plants and environmental factors highlighted in this review reveals that endophytes need to be studied in terms of their endophytome as a community of microorganisms that share a common space. Using new technologies available in metagenomics, we argue that the dynamics of evolution of endophytic communities in plants during plant development and in response to biotic and abiotic stresses need to be explored using metatranscriptomics, metaproteomics, and metabolomics. Until now, the adoption of these technologies has been limited owing to their high cost and the complexity of the experimental and analytical procedures involved. However, the rapid evolution of technologies and price and cost decrease of these technologies promise for providing a more comprehensive perspective of the microbiome (Liu et al., 2021). This knowledge will allow the underlying mechanisms to be elucidated and products derived from well-adapted endophytes to be developed. Understanding the intricacies of endophytic communities can pave the way for sustainable agricultural practices.
Author contributions
SL: Writing – original draft, Writing – review & editing. LP: Writing – original draft, Writing – review & editing. CB: Writing – review & editing. AL: Writing – review & editing.
Funding
The author(s) declare that financial support was received for the research, authorship, and/or publication of this article. LP was supported by the Foundation for Training in Industrial and Agricultural Research (FRIA, FNRS). SL was supported by Redebel and the Walloon Region (BIOSTIMTEST project, convention N°DGO6-8270).
Conflict of interest
The authors declare that the research was conducted in the absence of any commercial or financial relationships that could be construed as a potential conflict of interest.
Publisher’s note
All claims expressed in this article are solely those of the authors and do not necessarily represent those of their affiliated organizations, or those of the publisher, the editors and the reviewers. Any product that may be evaluated in this article, or claim that may be made by its manufacturer, is not guaranteed or endorsed by the publisher.
Supplementary material
The Supplementary material for this article can be found online at: https://www.frontiersin.org/articles/10.3389/fsufs.2024.1378436/full#supplementary-material
References
Adeleke, B. S., Fadiji, A. E., Ayilara, M. S., Igiehon, O. N., Nwachukwu, B. C., and Babalola, O. O. (2022). Strategies to enhance the use of endophytes as bioinoculants in agriculture. Horticulturae 8:498–551. doi: 10.3390/horticulturae8060498
Agler, MT, Ruhe, J, Kroll, S, Morhenn, C, Kim, ST, et al. (2016). Microbial Hub Taxa Link Host and Abiotic Factors to Plant Microbiome Variation. PLOS Biology. 14:e1002352. doi: 10.1371/journal.pbio.1002352
Ainelo, H., Lahesaare, A., Teppo, A., Kivisaar, M., and Teras, R. (2017). The promoter region of lapA and its transcriptional regulation by Fis in Pseudomonas putida. PLoS One 12:e0185482. doi: 10.1371/journal.pone.0185482
Ali, S., Duan, J., Charles, T. C., and Glick, B. R. (2014). A bioinformatics approach to the determination of genes involved in endophytic behavior in Burkholderia spp. J. Theor. Biol. 343, 193–198. doi: 10.1016/j.jtbi.2013.10.007
Alquéres, S., Meneses, C., Rouws, L., Rothballer, M., Baldani, I., Schmid, M., et al. (2013). The bacterial superoxide dismutase and glutathione reductase are crucial for endophytic colonization of rice roots by Gluconacetobacter diazotrophicus PAL5. Mol. Plant-Microbe Interact. 26, 937–945. doi: 10.1094/MPMI-12-12-0286-R
Andreote, F. D., De Araújo, W. L., De Azevedo, J. L., Van Elsas, J. D., Da Rocha, U. N., and Van Overbeek, L. S. (2009). Endophytic colonization of potato (Solanum tuberosum L.) by a novel competent bacterial endophyte, Pseudomonas putida strain P9, and its effect on associated bacterial communities. Appl. Environ. Microbiol. 75, 3396–3406. doi: 10.1128/AEM.00491-09
Anyango, B., Wilson, K. J., Beynon, J. L., and Giller, K. E. (1995). Diversity of rhizobia nodulating Phaseolus vulgaris L. in two Kenyan soils with contrasting pHs. Appl. Environ. Microbiol. 61, 4016–4021. doi: 10.1128/aem.61.11.4016-4021.1995
Aulakh, M. S., Wassmann, R., Bueno, C., Kreuzwieser, J., and Rennenberg, H. (2001). Erratum: characterization of root exudates at different growth stages of fen rice (Oryza sativa L.) cultivars (plant biology 3 (139-148)). Plant Biol. 3:298. doi: 10.1055/s-2001-15205
Badri, D. V., and Vivanco, J. M. (2009). Regulation and function of root exudates. Plant Cell Environ. 32, 666–681. doi: 10.1111/j.1365-3040.2009.01926.x
Baetz, U., and Martinoia, E. (2014). Root exudates: the hidden part of plant defense. Trends Plant Sci. 19, 90–98. doi: 10.1016/j.tplants.2013.11.006
Balachandar, D., Sandhiya, G. S., Sugitha, T. C. K., and Kumar, K. (2006). Flavonoids and growth hormones influence endophytic colonization and in planta nitrogen fixation by a diazotrophic Serratia sp. in rice. World J. Microbiol. Biotechnol. 22, 707–712. doi: 10.1007/s11274-005-9094-0
Balsanelli, E., Tadra-Sfeir, M. Z., Faoro, H., Pankievicz, V. C., de Baura, V. A., Pedrosa, F. O., et al. (2016). Molecular adaptations of Herbaspirillum seropedicae during colonization of the maize rhizosphere. Environ. Microbiol. 18, 2343–2356. doi: 10.1111/1462-2920.12887
Balsanelli, E., Tuleski, T. R., de Baura, V. A., Yates, M. G., Chubatsu, L. S., de Oliveira Pedrosa, F., et al. (2013). Maize root lectins mediate the interaction with Herbaspirillum seropedicae via N-acetyl glucosamine residues of lipopolysaccharides. PLoS One 8:e77001. doi: 10.1371/journal.pone.0077001
Barak, J. D., Gorski, L., Naraghi-Arani, P., and Charkowski, A. O. (2005). Salmonella enterica virulence genes are required for bacterial attachment to plant tissue. Appl. Environ. Microbiol. 71, 5685–5691. doi: 10.1128/AEM.71.10.5685-5691.2005
Barone, M., Ramayo-Caldas, Y., Estellé, J., Tambosco, K., Chadi, S., Maillard, F., et al. (2023). Gut barrier-microbiota imbalances in early life lead to higher sensitivity to inflammation in a murine model of C-section delivery. Microbiome 11, 1–24. doi: 10.1186/s40168-023-01584-0
Berendsen, RL, Vismans, G, Yu, K, Song, Y, de Jonge, R, Burgman, WP, et al. (2018). Disease-induced assemblage of a plant-beneficial bacterial consortium. ISME J. 12, 1496–1507. doi: 10.1038/s41396-018-0093-1
Bastias, D. A., Martínez-Ghersa, M. A., Ballaré, C. L., and Gundel, P. E. (2017). Epichloë fungal endophytes and plant defenses: not just alkaloids. Trends Plant Sci. 22, 939–948. doi: 10.1016/j.tplants.2017.08.005
Boddey, R. M. (1995). Biological nitrogen fixation in sugar cane: a key to energetically viable biofuel production. CRC Crit Rev Plant Sci 14, 263–279. doi: 10.1080/07352689509701929
Bouskill, N. J., Wood, T. E., Baran, R., Ye, Z., Bowen, B. P., Lim, H. C., et al. (2016). Belowground response to drought in a tropical forest soil. I. Changes in microbial functional potential and metabolism. Front. Microbiol. 7, 1–11. doi: 10.3389/fmicb.2016.00525
Bright, M., and Bulgheresi, S. (2010). A complex journey: transmission of microbial symbionts. Nat. Rev. Microbiol. 8, 218–230. doi: 10.1038/nrmicro2262
Buée, M, Reich, M, Murat, C, Morin, E, Nilsson, RH, Uroz, S, et al. (2009). 454 Pyrosequencing analyses of forest soils reveal an unexpectedly high fungal diversity. New Phytol. 184, 449–456. doi: 10.1111/j.1469-8137.2009.03003.x
Bulgarelli, D., Schlaeppi, K., Spaepen, S., Van Themaat, E. V. L., and Schulze-Lefert, P. (2013). Structure and functions of the bacterial microbiota of plants. Annu. Rev. Plant Biol. 64, 807–838. doi: 10.1146/annurev-arplant-050312-120106
Bulgari, D., Minio, A., Casati, P., Quaglino, F., Delledonne, M., and Bianco, P. A. (2014). Curtobacterium sp. genome sequencing underlines plant growth promotion-related traits. Genome Announc. 2:e00592–14. doi: 10.1128/genomeA.00592-14
Buyer, J. S., Roberts, D. P., and Russek-Cohen, E. (1999). Microbial community structure and function in the spermosphere as affected by soil and seed type. Can. J. Microbiol. 45, 138–144. doi: 10.1139/w98-227
Cabral, D., Stone, J. K., and Carroll, G. C. (1993). The internal mycobiota of Juncus spp.: microscopic and cultural observations of infection patterns. Mycol. Res. 97, 367–376. doi: 10.1016/S0953-7562(09)81140-4
Cankar, K., Kraigher, H., Ravnikar, M., and Rupnik, M. (2005). Bacterial endophytes from seeds of Norway spruce (Picea abies L. karst). FEMS Microbiol. Lett. 244, 341–345. doi: 10.1016/j.femsle.2005.02.008
Charbonneau, M. R., Donnell, D. O., Blanton, L. V., Totten, S. M., Davis, J. C. C., Barratt, M. J., et al. (2017). Growth in models of infant undernutrition. Cell 164, 859–871. doi: 10.1016/j.cell.2016.01.024.Sialylated
Chen, X., Marszałkowska, M., and Reinhold-Hurek, B. (2020). Jasmonic acid, not Salicyclic acid restricts endophytic root colonization of Rice. Front. Plant Sci. 10, 1–15. doi: 10.3389/fpls.2019.01758
Cherif, H., Marasco, R., Rolli, E., Ferjani, R., Fusi, M., Soussi, A., et al. (2015). Oasis desert farming selects environment-specific date palm root endophytic communities and cultivable bacteria that promote resistance to drought. Environ. Microbiol. Rep. 7, 668–678. doi: 10.1111/1758-2229.12304
Cocking, E. C. (2003). Endophytic colonization of plant roots by nitrogen-fixing bacteria. Plant Soil 252, 169–175. doi: 10.1023/A:1024106605806
Compant, S., Clément, C., and Sessitsch, A. (2010). Plant growth-promoting bacteria in the rhizo- and endosphere of plants: their role, colonization, mechanisms involved and prospects for utilization. Soil Biol. Biochem. 42, 669–678. doi: 10.1016/j.soilbio.2009.11.024
Compant, S., Duffy, B., Nowak, J., Clément, C., and Barka, E. A. (2005). Use of plant growth-promoting bacteria for biocontrol of plant diseases: principles, mechanisms of action, and future prospects. Appl. Environ. Microbiol. 71, 4951–4959. doi: 10.1128/AEM.71.9.4951-4959.2005
Compant, S., Samad, A., Faist, H., and Sessitsch, A. (2019). A review on the plant microbiome: ecology, functions, and emerging trends in microbial application. J. Adv. Res. 19, 29–37. doi: 10.1016/j.jare.2019.03.004
Cornelissen, J. H. C., Sibma, F., Van Logtestijn, R. S. P., Broekman, R. A., and Thompson, K. (2011). Leaf pH as a plant trait: species-driven rather than soil-driven variation. Funct. Ecol. 25, 449–455. doi: 10.1111/j.1365-2435.2010.01765.x
Curiel Yuste, J., Fernandez-Gonzalez, A. J., Fernandez-Lopez, M., Ogaya, R., Penuelas, J., Sardans, J., et al. (2014). Strong functional stability of soil microbial communities under semiarid Mediterranean conditions and subjected to long-term shifts in baseline precipitation. Soil Biol. Biochem. 69, 223–233. doi: 10.1016/j.soilbio.2013.10.045
De Bary, A., and Irmisch, TH., Pringsheim, N., and Sachs, J. (1866). Morphologie und Physiologie der Pilze, Flechten und Myxomyceten Hofmeister’s Handbook of Physiological Botany. Engelmann: Leipzig.
de Medeiros Azevedo, T., Aburjaile, F. F., Ferreira-Neto, J. R. C., Pandolfi, V., and Benko-Iseppon, A. M. (2021). The endophytome (plant-associated microbiome): methodological approaches, biological aspects, and biotech applications. World J. Microbiol. Biotechnol. 37:206–230. doi: 10.1007/s11274-021-03168-2
de Oliveira, M. V. V., Intorne, A. C., Vespoli, L. S., Madureira, H. C., Leandro, M. R., Pereira, T. N. S., et al. (2016). Differential effects of salinity and osmotic stress on the plant growth-promoting bacterium Gluconacetobacter diazotrophicus PAL5. Arch. Microbiol. 198, 287–294. doi: 10.1007/s00203-015-1176-2
Decroës, A., Mahillon, M., Genard, M., Lienard, C., Lima-Mendez, G., Gilmer, D., et al. (2022). Rhizomania: Hide and Seek of Polymyxa betae and the beet necrotic yellow vein virus with Beta vulgaris. Mol. Plant-Microbe Interact. 35, 989–1005. doi: 10.1094/MPMI-03-22-0063-R
Del Orozco-Mosqueda, M., Orozco-Mosqueda, M. C., and Santoyo, G. (2021). Plant-microbial endophytes interactions: scrutinizing their beneficial mechanisms from genomic explorations. Curr Plant Biol 25:100189. doi: 10.1016/j.cpb.2020.100189
Delbianco, A., Gibin, D., Pasinato, L., and Morelli, M. (2021). Update of the Xylella spp. host plant database – systematic literature search up to 31 December 2020. EFSA J. 19:6674. doi: 10.2903/j.efsa.2021.6674
Delitte, M., Caulier, S., Bragard, C., and Desoignies, N. (2021). Plant microbiota beyond farming practices: a review. Front Sustain Food Syst 5, 1–14. doi: 10.3389/fsufs.2021.624203
Dennis, P. G., Miller, A. J., and Hirsch, P. R. (2010). Are root exudates more important than other sources of rhizodeposits in structuring rhizosphere bacterial communities? FEMS Microbiol. Ecol. 72, 313–327. doi: 10.1111/j.1574-6941.2010.00860.x
Desoignies, N., and Legreve, A. (2011). In vitro dual culture of polymyxa betae in agrobacterium rhizogenes transformed sugar beet hairy roots in liquid media. J. Eukaryot. Microbiol. 58, 424–425. doi: 10.1111/j.1550-7408.2011.00563.x
Ding, T., and Melcher, U. (2016). Influences of plant species, season and location on leaf endophytic bacterial communities of non-cultivated plants. PLoS One 11, 1–13. doi: 10.1371/journal.pone.0150895
dos Santos, M. L., Berlitz, D. L., Wiest, S. L. F., Schünemann, R., Knaak, N., and Fiuza, L. M. (2018). Benefits associated with the interaction of endophytic Bacteria and plants. Braz. Arch. Biol. Technol. 61, 1–11. doi: 10.1590/1678-4324-2018160431
Downie, J. A. (2010). The roles of extracellular proteins, polysaccharides and signals in the interactions of rhizobia with legume roots. FEMS Microbiol. Rev. 34, 150–170. doi: 10.1111/j.1574-6976.2009.00205.x
Drew, G. C., Stevens, E. J., and King, K. C. (2021). Microbial evolution and transitions along the parasite–mutualist continuum. Nat. Rev. Microbiol. 19, 623–638. doi: 10.1038/s41579-021-00550-7
Egamberdieva, D., Wirth, S. J., Shurigin, V. V., Hashem, A., and Abd Allah, E. F. (2017). Endophytic bacteria improve plant growth, symbiotic performance of chickpea (Cicer arietinum L.) and induce suppression of root rot caused by fusarium solani under salt stress. Front. Microbiol. 8, 1–13. doi: 10.3389/fmicb.2017.01887
Eichenlaub, R., and Gartemann, K. H. (2011). The clavibacter michiganensis subspecies: molecular investigation of gram-positive bacterial plant pathogens. Annu. Rev. Phytopathol. 49, 445–464. doi: 10.1146/annurev-phyto-072910-095258
Elasri, M., Delorme, S., Lemanceau, P., Stewart, G., Laue, B., Glickmann, E., et al. (2001). Acyl-Homoserine lactone production is more common among plant-associated. Society 67, 1198–1209. doi: 10.1128/AEM.67.3.1198
Elgrably-Weiss, M., Schlosser-Silverman, E., Rosenshine, I., and Altuvia, S. (2006). DeoT, a DeoR-type transcriptional regulator of multiple target genes. FEMS Microbiol. Lett. 254, 141–148. doi: 10.1111/j.1574-6968.2005.00020.x
Espinosa-Leal, C. A., Puente-Garza, C. A., and García-Lara, S. (2018). In vitro plant tissue culture: means for production of biological active compounds. Planta 248, 1–18. doi: 10.1007/s00425-018-2910-1
Ferrando, L., and Fernández Scavino, A. (2015). Strong shift in the diazotrophic endophytic bacterial community inhabiting rice (Oryza sativa) plants after flooding. FEMS Microbiol. Ecol. 91:fiv104. doi: 10.1093/femsec/fiv104
Fesel, P. H., and Zuccaro, A. (2016). Dissecting endophytic lifestyle along the parasitism/mutualism continuum in Arabidopsis. Curr. Opin. Microbiol. 32, 103–112. doi: 10.1016/j.mib.2016.05.008
Filgueiras, L., Silva, R., Almeida, I., Vidal, M., Baldani, J. I., and Meneses, C. H. S. G. (2020). Gluconacetobacter diazotrophicus mitigates drought stress in Oryza sativa L. Plant Soil 451, 57–73. doi: 10.1007/s11104-019-04163-1
Fitzpatrick, C. R., Copeland, J., Wang, P. W., Guttman, D. S., Kotanen, P. M., and Johnson, M. T. J. (2018). Assembly and ecological function of the root microbiome across angiosperm plant species. Proc. Natl. Acad. Sci. USA 115, E1157–E1165. doi: 10.1073/pnas.1717617115
Francioli, D., Cid, G., Hajirezaei, M. R., and Kolb, S. (2022). Leaf bacterial microbiota response to flooding is controlled by plant phenology in wheat (Triticum aestivum L.). Sci. Rep. 12, 1–13. doi: 10.1038/s41598-022-15133-6
Frank, A. C., Guzmán, J. P. S., and Shay, J. E. (2017). Transmission of bacterial endophytes. Microorganisms 5:70–90. doi: 10.3390/microorganisms5040070
Fürnkranz, M., Lukesch, B., Müller, H., Huss, H., Grube, M., and Berg, G. (2012). Microbial diversity inside pumpkins: microhabitat-specific communities display a high antagonistic potential against Phytopathogens. Microb. Ecol. 63, 418–428. doi: 10.1007/s00248-011-9942-4
Gallegos, M. T., Schleif, R., Bairoch, A., Hofmann, K., and Ramos, J. L. (1997). Arac/XylS family of transcriptional regulators. Microbiol. Mol. Biol. Rev. 61, 393–410. doi: 10.1128/.61.4.393-410.1997
Germida, J. J., Siciliano, S. D., De Freitas, J. R., and Seib, A. M. (1998). Diversity of root-associated bacteria associated with field-grown canola (Brassica napus L.) and wheat (Triticum aestivum L.). FEMS Microbiol. Ecol. 26, 43–50. doi: 10.1016/S0168-6496(98)00020-8
Gilbert, J. A., Blaser, M. J., Caporaso, J. G., Jansson, J. K., Lynch, S. V., and Knight, R. (2018). Current understanding of the human microbiome. Nat. Med. 24, 392–400. doi: 10.1038/nm.4517
Gimenez-Ibanez, S., Boter, M., Ortigosa, A., García-Casado, G., Chini, A., Lewsey, M. G., et al. (2017). JAZ2 controls stomata dynamics during bacterial invasion. New Phytol. 213, 1378–1392. doi: 10.1111/nph.14354
Greer-Phillips, S. E., Stephens, B. B., and Alexandre, G. (2004). An energy taxis transducer promotes root colonization by Azospirillum brasilense. J. Bacteriol. 186, 6595–6604. doi: 10.1128/JB.186.19.6595-6604.2004
Groicher, K. H., Firek, B. A., Fujimoto, D. F., and Bayles, K. W. (2000). The Staphylococcus aureus lrgAB operon modulates murein hydrolase activity and penicillin tolerance. J. Bacteriol. 182, 1794–1801. doi: 10.1128/JB.182.7.1794-1801.2000
Hallmann, J., Quadt-Hallmann, A., Mahaffee, W. F., and Kloepper, J. W. (1997). Bacterial endophytes in agricultural crops. Can. J. Microbiol. 43, 895–914. doi: 10.1139/m97-131
Hardoim, P. R., Andreote, F. D., Reinhold-Hurek, B., Sessitsch, A., van Overbeek, L. S., and van Elsas, J. D. (2011). Rice root-associated bacteria: insights into community structures across 10 cultivars. FEMS Microbiology Ecology, 77, 154–164. doi: 10.1111/j.1574-6941.2011.01092.x
Hardoim, P. R., van Overbeek, L. S., Berg, G., Pirttilä, A. M., Compant, S., Campisano, A., et al. (2015). The hidden world within plants: ecological and evolutionary considerations for defining functioning of microbial endophytes. Microbiol. Mol. Biol. Rev. 79, 293–320.
Hardoim, P. R., van Overbeek, L. S., and Van Elsas, J. D. (2008). Properties of bacterial endophytes and their proposed role in plant growth. Trends Microbiol. 16, 463–471. doi: 10.1016/j.tim.2008.07.008
Harman, G., Khadka, R., Doni, F., and Uphoff, N. (2021). Benefits to plant health and productivity from enhancing plant microbial symbionts. Front. Plant Sci. 11:610065. doi: 10.3389/fpls.2020.610065
Hartmann, M., Brunner, I., Hagedorn, F., Bardgett, R. D., Stierli, B., Herzog, C., et al. (2017). A decade of irrigation transforms the soil microbiome of a semi-arid pine forest. Mol. Ecol. 26, 1190–1206. doi: 10.1111/mec.13995
Hassani, M. A., Durán, P., and Hacquard, S. (2018). Microbial interactions within the plant holobiont. Microbiome 6:58. doi: 10.1186/s40168-018-0445-0
Hayman, D. S., and Tavares, M. (1985). Plant growth responses to vesicular-arbuscular mycorrhiza xv. Influence of soil pH on the symbiotic efficiency of different endophytes. New Phytol. 100, 367–377.
Henning, J. A., Weston, D. J., Pelletier, D. A., Timm, C. M., Jawdy, S. S., and Classen, A. T. (2016). Root bacterial endophytes alter plant phenotype, but not physiology. PeerJ 2016, 1–20. doi: 10.7717/peerj.2606
Hermans, S. M., Buckley, H. L., Case, B. S., Curran-cournane, F., and Taylor, M. (2017). Bacteria as emerging indicators of soil condition Syrie. Appl. Environ. Microbiol. 83, 1–13. doi: 10.1128/AEM.02826-16
Herre, E. A., Knowlton, N., Mueller, U. G., and Rehner, S. A. (1999). Patterns of ecological transmission and evolutionary association. Trends Ecol. Evol. 14, 49–53.
Hinsinger, P., Bengough, A. G., Vetterlein, D., and Young, I. M. (2009). Rhizosphere: biophysics, biogeochemistry and ecological relevance. Plant Soil 321, 117–152. doi: 10.1007/s11104-008-9885-9
Hou, L., He, X., Li, X., Wang, S., and Zhao, L. (2019). Species composition and colonization of dark septate endophytes are affected by host plant species and soil depth in the mu us sandland, Northwest China. Fungal Ecol. 39, 276–284. doi: 10.1016/j.funeco.2019.01.001
Hu, X., Fang, Q., Li, S., Wu, J., and Chen, J. (2009). Isolation and characterization of endophytic and rhizosphere bacterial antagonists of soft rot pathogen from Pinellia ternata. FEMS Microbiol. Lett. 295, 10–16. doi: 10.1111/j.1574-6968.2009.01558.x
Hurek, T., and Reinhold-Hurek, B. (2003). Azoarcus sp. strain BH72 as a model for nitrogen-fixing grass endophytes. J. Biotechnol. 106, 169–178. doi: 10.1016/j.jbiotec.2003.07.010
Hyeon, J. E., Kang, D. H., Kim, Y. I., You, S. K., and Han, S. O. (2012). GntR-type transcriptional regulator pckr negatively regulates the expression of phosphoenolpyruvate Carboxykinase in Corynebacterium glutamicum. J. Bacteriol. 194, 2181–2188. doi: 10.1128/JB.06562-11
James, E. K., Gyaneshwar, P., Mathan, N., Barraquio, W. L., Reddy, P. M., Iannetta, P. P. M., et al. (2002). Infection and colonization of rice seedlings by the plant growth-promoting bacterium Herbaspirillum seropedicae Z67. Mol. Plant-Microbe Interact. 15, 894–906. doi: 10.1094/MPMI.2002.15.9.894
Jin, H., Yang, X. Y., Yan, Z. Q., Liu, Q., Li, X. Z., Chen, J. X., et al. (2014). Characterization of rhizosphere and endophytic bacterial communities from leaves, stems and roots of medicinal Stellera chamaejasme L. Syst. Appl. Microbiol. 37, 376–385. doi: 10.1016/j.syapm.2014.05.001
Jofré, E., Lagares, A., and Mori, G. (2004). Disruption of dTDP-rhamnose biosynthesis modifies lipopolysaccharide core, exopolysaccharide production, and root colonization in Azospirillum brasilense. FEMS Microbiol. Lett. 231, 267–275. doi: 10.1016/S0378-1097(04)00003-5
Johnston-Monje, D., and Raizada, M. N. (2011). Conservation and diversity of seed associated endophytes in Zea across boundaries of evolution, ethnography and ecology. PLoS One 6:e20396. doi: 10.1371/journal.pone.0020396
Ju, H. J., Hill, N. S., Abbott, T., and Ingram, K. T. (2006). Temperature influences on endophyte growth in tall fescue. Crop Sci. 46, 404–412. doi: 10.2135/cropsci2005.0282
Kandel, S. L., Joubert, P. M., and Doty, S. L. (2017). Bacterial endophyte colonization and distribution within plants. Microorganisms 5, 9–11. doi: 10.3390/microorganisms5040077
Kapourchali, F. R., and Cresci, G. A. M. (2020). Early-life gut microbiome—the importance of maternal and infant factors in its establishment. Nutr. Clin. Pract. 35, 386–405. doi: 10.1002/ncp.10490
Kartjito, M. S., Yosia, M., Wasito, E., Soloan, G., Agussalim, A. F., and Basrowi, R. W. (2023). Defining the relationship of gut microbiota, immunity, and cognition in early life—a narrative review. Nutrients 15:2642. doi: 10.3390/nu15122642
Kehe, J., Ortiz, A., Kulesa, A., Gore, J., Blainey, P. C., and Friedman, J. (2021). Positive interactions are common among culturable bacteria. Sci. Adv. 7, 1–10. doi: 10.1126/sciadv.abi7159
Kembel, S. W., O’Connor, T. K., Arnold, H. K., Hubbell, S. P., Wright, S. J., and Green, J. L. (2014). Relationships between phyllosphere bacterial communities and plant functional traits in a neotropical forest. Proc. Natl. Acad. Sci. USA 111, 13715–13720. doi: 10.1073/pnas.1216057111
Khalaf, E. M., and Raizada, M. N. (2018). Bacterial seed endophytes of domesticated cucurbits antagonize fungal and oomycete pathogens including powdery mildew. Front. Microbiol. 9, 1–18. doi: 10.3389/fmicb.2018.00042
Khare, E., Mishra, J., and Arora, N. K. (2018). Multifaceted interactions between endophytes and plant: developments and prospects. Front. Microbiol. 9:2732. doi: 10.3389/fmicb.2018.02732
Kogel, K. H., Franken, P., and Hückelhoven, R. (2006). Endophyte or parasite - what decides? Curr. Opin. Plant Biol. 9, 358–363. doi: 10.1016/j.pbi.2006.05.001
Krause, A., Julich, H., Mankar, M., and Reinhold-Hurek, B. (2017). The regulatory network controlling ethanol-induced expression of alcohol dehydrogenase in the endophyte azoarcus sp. strain BH72. Mol. Plant-Microbe Interact. 30, 778–785. doi: 10.1094/MPMI-01-17-0013-R
Lacour, S., and Landini, P. (2004). σS-dependent gene expression at the onset of stationary phase in Escherichia coli: function of σS-dependent genes and identification of their promoter sequences. J. Bacteriol. 186, 7186–7195. doi: 10.1128/JB.186.21.7186-7195.2004
Lafay, B., and Burdon, J. J. (1998). Molecular diversity of rhizobia occurring on native shrubby legumes in southeastern Australia. Appl. Environ. Microbiol. 64, 3989–3997. doi: 10.1128/aem.64.10.3989-3997.1998
Landa, B. B., Saponari, M., Feitosa-Junior, O. R., Giampetruzzi, A., Vieira, F. J. D., Mor, E., et al. (2022). Xylella fastidiosa ‘s relationships: the bacterium, the host plants, and the plant microbiome. New Phytol. 234, 1598–1605. doi: 10.1111/nph.18089
Leandro, M. R., Vespoli, L. S., Andrade, L. F., Soares, F. S., Boechat, A. L., Pimentel, V. R., et al. (2021). DegP protease is essential for tolerance to salt stress in the plant growth-promoting bacterium Gluconacetobacter diazotrophicus PAL5. Microbiol. Res. 243:126654. doi: 10.1016/j.micres.2020.126654
Leonard, S. P., Powell, J. E., Perutka, J., Geng, P., Heckmann, L. C., Horak, R. D., et al. (2020). Supplementary materials for engineered symbionts activate honey bee immunity and limit pathogens. Science 1979, 573–576. doi: 10.1126/science.aax9039.Engineered
Lima, E., Boddey, R. M., and Döbereiner, J. (1987). Quantification of biological nitrogen fixation associated with sugar cane using a 15N aided nitrogen balance. Soil Biol. Biochem. 19, 165–170. doi: 10.1016/0038-0717(87)90077-0
Liu, Y. X., Qin, Y., Chen, T., Lu, M., Qian, X., Guo, X., et al. (2021). A practical guide to amplicon and metagenomic analysis of microbiome data. Protein Cell 12, 315–330. doi: 10.1007/s13238-020-00724-8
Liu, Y., Zhu, A., Tan, H., Cao, L., and Zhang, R. (2019). Engineering banana endosphere microbiome to improve fusarium wilt resistance in banana. Microbiome 7, 1–15. doi: 10.1186/s40168-019-0690-x
Lloyd-Price, J., Abu-Ali, G., and Huttenhower, C. (2016). The healthy human microbiome. Genome Med. 8, 1–11. doi: 10.1186/s13073-016-0307-y
Macedo-Raygoza, G. M., Valdez-Salas, B., Prado, F. M., Prieto, K. R., Yamaguchi, L. F., Kato, M. J., et al. (2019). Enterobacter cloacae, an endophyte that establishes a nutrient-transfer Symbiosis with Banana plants and protects against the black Sigatoka pathogen. Front. Microbiol. 10, 1–15. doi: 10.3389/fmicb.2019.00804
Maddocks, S. E., and Oyston, P. C. F. (2008). Structure and function of the LysR-type transcriptional regulator (LTTR) family proteins. Microbiology (N Y) 154, 3609–3623. doi: 10.1099/mic.0.2008/022772-0
Mansfield, J., Genin, S., Magori, S., Citovsky, V., Sriariyanum, M., Ronald, P., et al. (2012). Top 10 plant pathogenic bacteria in molecular plant pathology. Mol. Plant Pathol. 13, 614–629. doi: 10.1111/j.1364-3703.2012.00804.x
Mark, G. L., Dow, J. M., Kiely, P. D., Higgins, H., Haynes, J., Baysse, C., et al. (2005). Transcriptome profiling of bacterial responses to root exudates identifies genes involved in microbe-plant interactions. Proc. Natl. Acad. Sci. USA 102, 17454–17459. doi: 10.1073/pnas.0506407102
Martin, R. G., and Rosner, J. L. (2001). The AraC transcriptional activators. Curr. Opin. Microbiol. 4, 132–137. doi: 10.1016/S1369-5274(00)00178-8
Mastretta, C., Taghavi, S., Van Der Lelie, D., Mengoni, A., Galardi, F., Gonnelli, C., et al. (2009). Endophytic bacteria from seeds of Nicotiana tabacum can reduce cadmium phytotoxicity. Int. J. Phytoremediation 11, 251–267. doi: 10.1080/15226510802432678
Mattinen, L., Somervuo, P., Nykyri, J., Nissinen, R., Kouvonen, P., Corthals, G., et al. (2008). Microarray profiling of host-extract-induced genes and characterization of the type VI secretion cluster in the potato pathogen Pectobacterium atrosepticum. Microbiology (N Y) 154, 2387–2396. doi: 10.1099/mic.0.2008/017582-0
Melotto, M., Zhang, L., Oblessuc, P. R., and He, S. Y. (2017). Stomatal defense a decade later. Plant Physiol. 174, 561–571. doi: 10.1104/pp.16.01853
Miller, R. W., and Eady, R. R. (1988). Molybdenum and vanadium nitrogenases of Azotobacter chroococcum. Low temperature favours N2 reduction by vanadium nitrogenase. Biochem. J. 256, 429–432. doi: 10.1042/bj2560429
Monteiro, R. A., Balsanelli, E., Tuleski, T., Faoro, H., Cruz, L. M., Wassem, R., et al. (2012). Genomic comparison of the endophyte Herbaspirillum seropedicae SmR1 and the phytopathogen Herbaspirillum rubrisubalbicans M1 by suppressive subtractive hybridization and partial genome sequencing. FEMS Microbiol. Ecol. 80, 441–451. doi: 10.1111/j.1574-6941.2012.01309.x
Musilova, L., Ridl, J., Polivkova, M., Macek, T., and Uhlik, O. (2016). Effects of secondary plant metabolites on microbial populations: changes in community structure and metabolic activity in contaminated environments. Int. J. Mol. Sci. 17:1205. doi: 10.3390/ijms17081205
Nadarajah, K. K. (2020). Ros homeostasis in abiotic stress tolerance in plants. Int. J. Mol. Sci. 21, 1–29. doi: 10.3390/ijms21155208
Naylor, D., Degraaf, S., Purdom, E., and Coleman-Derr, D. (2017). Drought and host selection influence bacterial community dynamics in the grass root microbiome. ISME J. 11, 2691–2704. doi: 10.1038/ismej.2017.118
Nissinen, R. M., Männistö, M. K., and van Elsas, J. D. (2012). Endophytic bacterial communities in three arctic plants from low arctic fell tundra are cold-adapted and host-plant specific. FEMS Microbiol. Ecol. 82, 510–522. doi: 10.1111/j.1574-6941.2012.01464.x
Occhipinti, A. (2013). Plant coevolution: evidences and new challenges. J. Plant Interact. 8, 188–196. doi: 10.1080/17429145.2013.816881
Oukala, N., Aissat, K., and Pastor, V. (2021). Bacterial endophytes: the hidden actor in plant immune responses against biotic stress. Plan. Theory 10:1012. doi: 10.3390/plants10051012
Papik, J., Folkmanova, M., Polivkova-Majorova, M., Suman, J., and Uhlik, O. (2020). The invisible life inside plants: deciphering the riddles of endophytic bacterial diversity. Biotechnol. Adv. 44:107614. doi: 10.1016/j.biotechadv.2020.107614
Peduzzi, C., Sagia, A., Burokienė, D., Nagy, I. K., Fischer-Le Saux, M., Portier, P., et al. (2022). Novel genetic traits in Xanthomonas unveiled by the complete genome sequencing of three clade-1 xanthomonads. Washington: American Society for Microbiology.
Petrini, O. (1961). “Fungal Endophytes of Tree Leaves” in Microbial Ecology of Leaves. ed. J. H. Andrews . (New York, NY: Springer), 77–88.
Philippot, L., Raaijmakers, J. M., Lemanceau, P., and Van Der Putten, W. H. (2013). Going back to the roots: the microbial ecology of the rhizosphere. Nat. Rev. Microbiol. 11, 789–799. doi: 10.1038/nrmicro3109
Pillay, V. K., and Nowak, J. (1997). Lnoculum density, temperature, and genotype effects on in vitro growth promotion and epiphytic and endophytic colonization of tomato (Lycopersicon esculentum L.) seedlings inoculated with a pseudomonad bacterium. Can. J. Microbiol. 43, 354–361.
Pinski, A., Betekhtin, A., Hupert-Kocurek, K., Mur, L. A. J., and Hasterok, R. (2019). Defining the genetic basis of plant–endophytic bacteria interactions. Int. J. Mol. Sci. 20:1947. doi: 10.3390/ijms20081947
Pires, C., Franco, A. R., Pereira, S. I. A., Henriques, I., Correia, A., Magan, N., et al. (2017). Metal(loid)-contaminated soils as a source of Culturable heterotrophic aerobic Bacteria for remediation applications. Geomicrobiol J. 34, 760–768. doi: 10.1080/01490451.2016.1261968
Plucani do Amaral, F., Wang, J., Williams, J., Tuleski, T. R., Joshi, T., Ferreira, M. A. R., et al. (2023). Mapping genetic variation in Arabidopsis in response to plant growth-promoting bacterium Azoarcus olearius DQS-4T. Microorganisms 11, 1–15. doi: 10.3390/microorganisms11020331
Polivkova, M., Suman, J., Strejcek, M., Kracmarova, M., Hradilova, M., Filipova, A., et al. (2018). Diversity of root-associated microbial populations of Tamarix parviflora cultivated under various conditions. Appl. Soil Ecol. 125, 264–272. doi: 10.1016/j.apsoil.2018.02.002
Puente, M. E., Li, C. Y., and Bashan, Y. (2009). Endophytic bacteria in cacti seeds can improve the development of cactus seedlings. Environ. Exp. Bot. 66, 402–408. doi: 10.1016/j.envexpbot.2009.04.007
Qaim, M. (2020). Role of new plant breeding Technologies for Food Security and Sustainable Agricultural Development. Appl. Econ. Perspect. Policy 42, 129–150. doi: 10.1002/aepp.13044
Quadt-Hallmann, A., Hallmann, J., and Kloepper, J. W. (1997). Bacterial endophytes in cotton: location and interaction with other plant-associated bacteria. Can. J. Microbiol. 43, 254–259. doi: 10.1139/m97-035
Rahman, N. S. N. A., Hamid, N. W. A., and Nadarajah, K. (2021). Effects of abiotic stress on soil microbiome. Int. J. Mol. Sci. 22:9036. doi: 10.3390/ijms22169036
Raza, A., Razzaq, A., Mehmood, S. S., Zou, X., Zhang, X., Lv, Y., et al. (2019). Impact of climate change on crops adaptation and strategies to tackle its outcome: a review. Plan. Theory 8:34. doi: 10.3390/plants8020034
Redford, A. J., Bowers, R. M., Knight, R., Linhart, Y., and Fierer, N. (2010). The ecology of the phyllosphere: geographic and phylogenetic variability in the distribution of bacteria on tree leaves. Environ. Microbiol. 12, 2885–2893. doi: 10.1111/j.1462-2920.2010.02258.x
Redman, R. S., Sheehan, K. B., Stout, R. G., Rodriguez, R. J., and Henson, J. M. (2002). Thermotolerance generated by plant/fungal Symbiosis. Science 298:1581. doi: 10.1126/science.1078055
Reinhold-Hurek, B., and Hurek, T. (2007). Chapter 9- endophytic assocations of Azoarcus spp. Associative and Endophytic Nitrogen-fixing Bacteria and Cyanobacterial Associations, 5, 191–207. doi: 10.1007/1-4020-3546-2
Reinhold-Hurek, B., and Hurek, T. (2011). Living inside plants: bacterial endophytes. Curr. Opin. Plant Biol. 14, 435–443. doi: 10.1016/j.pbi.2011.04.004
Ren, G., Zhang, H., Lin, X., Zhu, J., and Jia, Z. (2015). Response of leaf endophytic bacterial community to elevated CO2 at different growth stages of rice plant. Front. Microbiol. 6:855. doi: 10.3389/fmicb.2015.00855
Rho, H., van Epps, V., Wegley, N., Doty, S. L., and Kim, S. H. (2018). Salicaceae endophytes modulate stomatal behavior and increase water use efficiency in rice. Front. Plant Sci. 9, 1–15. doi: 10.3389/fpls.2018.00188
Rolli, E., Marasco, R., Vigani, G., Ettoumi, B., Mapelli, F., Deangelis, M. L., et al. (2015). Improved plant resistance to drought is promoted by the root-associated microbiome as a water stress-dependent trait. Environ. Microbiol. 17, 316–331. doi: 10.1111/1462-2920.12439
Rong, Y., Wang, Y., Guan, Y., Ma, J., Cai, Z., Yang, G., et al. (2017). Pyrosequencing reveals soil enzyme activities and bacterial communities impacted by graphene and its oxides. J. Agric. Food Chem. 65, 9191–9199. doi: 10.1021/acs.jafc.7b03646
Roossinck, M. J. (2010). Lifestyles of plant viruses. Philosophical Trans. Royal Society B: Biolog. Sci. 365, 1899–1905. doi: 10.1098/rstb.2010.0057
Rosenberg, E., and Zilber-Rosenberg, I. (2016). Microbes drive evolution of animals and plants: the hologenome concept. MBio 7:1395. doi: 10.1128/mBio.01395-15
Rosenblueth, M., and Martínez-Romero, E. (2006). Bacterial endophytes and their interactions with hosts. Mol. Plant-Microbe Interact. 19, 827–837. doi: 10.1094/MPMI-19-0827
Sabino, J., Tarassishin, L., Eisele, C., Hawkins, K., Barré, A., Nair, N., et al. (2023). Influence of early life factors, including breastmilk composition, on the microbiome of infants born to mothers with and without inflammatory bowel disease. J. Crohns Colitis 17, 1723–1732. doi: 10.1093/ecco-jcc/jjad096
Saikkonen, K., Wa, P. R., and Helander, M. (2012). Lasp-1 Regulates Podosome Function. PLoS One 7, 1–10. doi: 10.1371/Citation
Salvaudon, L., Héraudet, V., and Shykoff, J. A. (2005). Parasite-host fitness trade-offs change with parasite identity: genotype-specific interactions in a plant-pathogen system. Evolution (N Y) 59, 2518–2524. doi: 10.1111/j.0014-3820.2005.tb00965.x
Santos-Medellín, C., Edwards, J., Liechty, Z., Nguyen, B., and Sundaresan, V. (2017). Drought stress results in a compartment-specific restructuring of. MBio 8, 1–15. doi: 10.1128/mBio.00764-17
Saravanan, V. S., Madhaiyan, M., Osborne, J., Thangaraju, M., and Sa, T. M. (2008). Ecological occurrence of Gluconacetobacter diazotrophicus and nitrogen-fixing Acetobacteraceae members: their possible role in plant growth promotion. Microb. Ecol. 55, 130–140. doi: 10.1007/s00248-007-9258-6
Sasse, J., Martinoia, E., and Northen, T. (2018). Feed your friends: do Plant exudates shape the root microbiome? Trends Plant Sci. 23, 25–41. doi: 10.1016/j.tplants.2017.09.003
Schimel, J., Balser, T. C., and Wallenstein, M. (2007). Microbial stress-response physiology and its implications for ecosystem function. Ecology 88, 1386–1394. doi: 10.1890/06-0219
Sekirov, I., Russell, S. L., Antunes, C. M., and Finlay, B. B. (2010). Gut microbiota in health and disease. Physiol. Rev. 90, 859–904. doi: 10.1152/physrev.00045.2009
Sessitsch, A., Hardoim, P., Döring, J., Weilharter, A., Krause, A., Woyke, T., et al. (2012). Functional characteristics of an endophyte community colonizing Rice roots as revealed by metagenomic analysis. Mol. Plant-Microbe Interact. 25, 28–36. doi: 10.1094/MPMI-08-11-0204
Sessitsch, A., Reiter, B., Pfeifer, U., and Wilhelm, E. (2002). Cultivation-independent population analysis of bacterial endophytes in three potato varieties based on eubacterial and Actinomycetes-specific PCR of 16S rRNA genes. FEMS Microbiol. Ecol. 39, 23–32. doi: 10.1016/S0168-6496(01)00189-1
Shaw, L. J., Morris, P., and Hooker, J. E. (2006). Perception and modification of plant flavonoid signals by rhizosphere microorganisms. Environ. Microbiol. 8, 1867–1880. doi: 10.1111/j.1462-2920.2006.01141.x
Singer, A. C., Crowley, D. E., and Thompson, I. P. (2003). Secondary plant metabolites in phytoremediation and biotransformation. Trends Biotechnol. 21, 123–130. doi: 10.1016/S0167-7799(02)00041-0
Singer, A. C., Thompson, I. P., and Bailey, M. J. (2004). The tritrophic trinity: a source of pollutant-degrading enzymes and its implications for phytoremediation. Curr. Opin. Microbiol. 7, 239–244. doi: 10.1016/j.mib.2004.04.007
Singh, P., Chauhan, P. K., Upadhyay, S. K., Singh, R. K., Dwivedi, P., Wang, J., et al. (2022). Mechanistic insights and potential use of Siderophores producing microbes in rhizosphere for mitigation of stress in plants grown in degraded land. Front. Microbiol. 13:898979. doi: 10.3389/fmicb.2022.898979
Singh, P., Singh, R. K., Guo, D. J., Sharma, A., Singh, R. N., Li, D. P., et al. (2021). Whole genome analysis of sugarcane root-associated endophyte Pseudomonas aeruginosa B18—a plant growth-promoting bacterium with antagonistic potential against Sporisorium scitamineum. Front. Microbiol. 12, 1–21. doi: 10.3389/fmicb.2021.628376
Smercina, D. N., Evans, S. E., Friesen, M. L., and Tiemann, L. K. (2019). To fix or not to fix: controls on free-living nitrogen fixation in the rhizosphere. Appl. Environ. Microbiol. 85:e02546–18. doi: 10.1128/AEM.02546-18
Stürmer, S. L., and Kemmelmeier, K. (2021). The Glomeromycota in the Neotropics. Front. Microbiol. 11, 1–18. doi: 10.3389/fmicb.2020.553679
Swanson, E, Sbissi, I, Ktari, A, Cherif-Silini, H, Ghodhbane-Gtari, F, Tisa, LS, et al. (2022). Decrypting phytomicrobiome of the neurotoxic actinorhizal species, Coriaria myrtifolia, and dispersal boundary of Frankia cluster 2 in soil outward compatible host rhizosphere. Front. Microbiol. 13:1027317. doi: 10.3389/fmicb.2022.1027317
Taghavi, S., van der Lelie, D., Hoffman, A., Zhang, Y. B., Walla, M. D., Vangronsveld, J., et al. (2010). Genome sequence of the plant growth promoting endophytic bacterium Enterobacter sp. 638. PLoS Genet. 6:19. doi: 10.1371/journal.pgen.1000943
Thaw, P., Sedelnikova, S. E., Muranova, T., Wiese, S., Ayora, S., Alonso, J. C., et al. (2006). Structural insight into gene transcriptional regulation and effector binding by the Lrp/AsnC family. Nucleic Acids Res. 34, 1439–1449. doi: 10.1093/nar/gkl009
Tocheva, E. I., Ortega, D. R., and Jensen, G. J. (2016). Sporulation, bacterial cell envelopes and the origin of life. Nat. Rev. Microbiol. 14, 535–542. doi: 10.1038/nrmicro.2016.85
Truyens, S., Weyens, N., Cuypers, A., and Vangronsveld, J. (2015). Bacterial seed endophytes: genera, vertical transmission and interaction with plants. Environ. Microbiol. Rep. 7, 40–50. doi: 10.1111/1758-2229.12181
Tufail, M. A., Touceda-González, M., Pertot, I., and Ehlers, R. U. (2021). Gluconacetobacter diazotrophicus Pal5 enhances plant robustness status under the combination of moderate drought and low nitrogen stress in Zea mays L. Microorganisms 9:870. doi: 10.3390/microorganisms9040870
Ullah, A., Nisar, M., Ali, H., Hazrat, A., Hayat, K., Keerio, A. A., et al. (2019). Drought tolerance improvement in plants: an endophytic bacterial approach. Appl. Microbiol. Biotechnol. 103, 7385–7397. doi: 10.1007/s00253-019-10045-4
Vandenkoornhuyse, P., Quaiser, A., Duhamel, M., Le Van, A., and Dufresne, A. (2015). The importance of the microbiome of the plant holobiont. New Phytol. 206, 1196–1206. doi: 10.1111/nph.13312
Vergani, L., Mapelli, F., Marasco, R., Crotti, E., Fusi, M., Di Guardo, A., et al. (2017). Bacteria associated to plants naturally selected in a historical PCB polluted soil show potential to sustain natural attenuation. Front. Microbiol. 8, 1–13. doi: 10.3389/fmicb.2017.01385
Wang, Y., Li, C., Kou, Y., Wang, J., Tu, B., Li, H., et al. (2017). Soil pH is a major driver of soil diazotrophic community assembly in Qinghai-Tibet alpine meadows. Soil Biol. Biochem. 115, 547–555. doi: 10.1016/j.soilbio.2017.09.024
Wang, G. M., Stribley, D. P., Tinker, P. B., and Walker, C. (1993). Effects of pH on arbuscular mycorrhiza I. Field observations on the long-term liming experiments at Rothamsted and Woburn. New Phytol. 124, 465–472. doi: 10.1111/j.1469-8137.1993.tb03837.x
White, J. F., Kingsley, K. L., Verma, S. K., and Kowalski, K. P. (2018). Rhizophagy cycle: an oxidative process in plants for nutrient extraction from symbiotic microbes. Microorganisms 6:95. doi: 10.3390/microorganisms6030095
Williams, A., Wilkinson, A., Krehenbrink, M., Russo, D. M., Zorreguieta, A., and Downie, J. A. (2008). Glucomannan-mediated attachment of Rhizobium leguminosarum to pea root hairs is required for competitive nodule infection. J. Bacteriol. 190, 4706–4715. doi: 10.1128/JB.01694-07
Yang, R., Liu, P., and Ye, W. (2017). Illumina-based analysis of endophytic bacterial diversity of tree peony (Paeonia sect. Moutan) roots and leaves. Braz. J. Microbiol. 48, 695–705. doi: 10.1016/j.bjm.2017.02.009
Yang, W., Ni, L., and Somerville, R. L. (1993). A stationary-phase protein of Escherichia coli that affects the mode of association between the trp repressor protein and operator-bearing DNA. Proc. Natl. Acad. Sci. USA 90, 5796–5800. doi: 10.1073/pnas.90.12.5796
Yergeau, E., Tremblay, J., Joly, S., Labrecque, M., Maynard, C., Pitre, F. E., et al. (2018). Soil contamination alters the willow root and rhizosphere metatranscriptome and the root-rhizosphere interactome. ISME J. 12, 869–884. doi: 10.1038/s41396-017-0018-4
Yi, Y., de Jong, A., Frenzel, E., and Kuipers, O. P. (2017). Comparative transcriptomics of Bacillus mycoides root exudates reveals different genetic adaptation of endophytic and soil isolates. Front. Microbiol. 8, 1–14. doi: 10.3389/fmicb.2017.01487
Yu, X., Yang, J., Wang, E., Li, B., and Yuan, H. (2015). Effects of growth stage and fulvic acid on the diversity and dynamics of endophytic bacterial community in stevia rebaudiana bertoni leaves. Front. Microbiol. 6, 1–13. doi: 10.3389/fmicb.2015.00867
Zhang, Q., Acuña, J. J., Inostroza, N. G., Mora, M. L., Radic, S., Sadowsky, M. J., et al. (2019). Endophytic bacterial communities associated with roots and leaves of plants growing in Chilean extreme environments. Sci. Rep. 9, 1–12. doi: 10.1038/s41598-019-41160-x
Zhang, H. W., Song, Y. C., and Tan, R. X. (2006). Biology and chemistry of endophytes. Nat. Prod. Rep. 23, 753–771. doi: 10.1039/b609472b
Zhang, N., Wang, D., Liu, Y., Li, S., Shen, Q., and Zhang, R. (2014). Effects of different plant root exudates and their organic acid components on chemotaxis, biofilm formation and colonization by beneficial rhizosphere-associated bacterial strains. Plant Soil 374, 689–700. doi: 10.1007/s11104-013-1915-6
Keywords: endophytome, community structure, bacteria, plant-endophyte interactions, plant-microbe interactions, microbiome, phytobiome, dysbiosis
Citation: Lengrand S, Pesenti L, Bragard C and Legrève A (2024) Bacterial endophytome sources, profile and dynamics—a conceptual framework. Front. Sustain. Food Syst. 8:1378436. doi: 10.3389/fsufs.2024.1378436
Edited by:
Everlon Cid Rigobelo, São Paulo State University, BrazilReviewed by:
V. S. Saravanan, Pondicherry University, IndiaSwarnalee Dutta, Jeonbuk National University, Republic of Korea
Copyright © 2024 Lengrand, Pesenti, Bragard and Legrève. This is an open-access article distributed under the terms of the Creative Commons Attribution License (CC BY). The use, distribution or reproduction in other forums is permitted, provided the original author(s) and the copyright owner(s) are credited and that the original publication in this journal is cited, in accordance with accepted academic practice. No use, distribution or reproduction is permitted which does not comply with these terms.
*Correspondence: Anne Legrève, anne.legreve@uclouvain.be
†These authors have contributed equally to this work and share first authorship