- 1Faculty of Environmental Sciences and Natural Resource Management, Norwegian University of Life Sciences, Ås, Norway
- 2N2 Applied, Asker, Norway
Plasma-based nitrogen fixation has recently been shown to be applicable in the domain of manure management, as it has the ability to reduce ammoniacal nitrogen losses and increase the nitrogen content of organic wastes, with air and electricity as the only input. In addition, the plasma treatment confers antimicrobial properties, which we hypothesize to be transferable to methanogenic archaea and hence prevent methane formation during manure storage – a major contributor to global anthropogenic greenhouse gas emissions. In this work we compared the methane formation from cow manure to the methane formation in nitrogen enriched cow manure, kept in two outdoor storage tanks for 70–80 summer days over three consecutive years. In all instances, the methane formation was eliminated completely. To investigate the cause of inhibition, a controlled incubation experiment was conducted to show that neither the acidification nor the addition of nitrate or nitrite, alone or in combination, could explain the inhibition of methanogenesis and denitrification that occurred in plasma treated cow manure at moderate pH.
1 Introduction
The potent greenhouse gas methane (CH4) has received recent attention for its short lifespan in the atmosphere (IEA, 2022). Compared to the 120-year half-life of carbon dioxide (CO2), CH4 has an atmospheric lifespan of only 12 years (Lynch et al., 2020). The combination of its potency and short lifespan results in a time-dependent global warming potential (GWP) where the GWP over a 100-year period (GWP100) will be 27 times higher than that of CO2, whereas a 20-year time-horizon (GWP20) will result in a GWP of 81 CO2 equivalents. Consequently, measures that significantly reduce CH4 emissions will have an impact on the rate of global warming, particularly in the short term. The Intergovernmental Panel on Climate Change (IPCC, 2018) estimate that a 40–45% reduction of CH4 emissions can limit the global temperature rise to 1.5°C above pre-industrial level if implemented by 2030. The resultant delay in global warming may thus leave sufficient time for the complex task of decarbonizing power production, transportation, and other fossil fuel-dependent industries, and increase the probability of limiting global warming to 1.5°C throughout the 21st century.
Of global methane emissions, close to one third stems from agriculture, whereof the livestock sector is the main contributor. Methane emissions from livestock are dominated by enteric fermentation in ruminants and are estimated to account for 70% of global agricultural CH4 emissions, followed by manure management (7%) (Kuylenstierna et al., 2021). However, there are regional differences in the distribution of sources: in livestock-intense regions, where liquid manure is stored for prolonged periods, such as California, CH4 emissions from manure management equal those from enteric fermentation (CARB, 2020). Identifying and implementing measures that reduce CH4 emissions from manure management is therefore central to the 1.5°C target, as these measures must counteract a growing global demand for milk and meat (OECD-FAO, 2022).
Anaerobic digestion of livestock manure (AD) has been presented as a sensible mitigatory measure, as it captures the CH4 emitted from livestock manure and uses it to replace fossil gas. However, AD will often lead to increased methane production downstream (Balde et al., 2016; Rodhe et al., 2018) and exacerbated ammonia (NH3) emissions (Holly et al., 2017). An alternate or complimentary approach is acidification of livestock manure, commonly with sulfuric acid (H2SO4), to reduce the slurry pH and hence the ammonia volatilization and methanogenic activity (Hou et al., 2017). Retaining the effect during storage and at field application does however lead to a sulphur dose that often exceeds crop needs and increases the risk of soil acidification and sulphate leaching (Lamers et al., 1998).
A novel approach to manure management, that can work alone or in conjunction with the above technologies, is plasma treatment. In this process, a strong electric field ionises air, leading to the formation of a reactive nitrogen gas (NOx). The reactive gas is absorbed in livestock manure as nitrogen oxyanions (NOx−) resulting in a nitrogen enriched organic fertiliser (NEO), containing more plant available nitrogen at a lower pH, thus reducing NH3 volatilisation (Ingels and Graves, 2015). Since crops require more nitrogen than sulfur, the nutrient balance is improved, which in turn reduces the risk of soil acidification. The treatment is energy intense but electricity-based and is therefore suitable for integration with intermittent renewable energy.
In addition to the electricity-based nitrogen production and reduced losses of NH3, the plasma treatment has been shown to have antimicrobial effects (Hiis et al., 2023). This is important, as microbial activity in NEO would lead to denitrification and the subsequent loss of the plasma-generated nitrate (NO3−) and nitrite (NO2−). In this work we hypothesise that the inhibitory effect is transferable to methanogenic archaea. We measured the impact on CH4 emissions during the summer months over three consecutive years, in an outdoor storage facility, to approximate a farm scenario. To elucidate the mechanism behind the inhibition of CH4 emissions, we also conducted a controlled laboratory experiment investigating the effect of acidification (by H2SO4) and NO2− alone and in combination to imitate the effect of the plasma treatment.
2 Methodology
2.1 Farm-scenario experiment
Four outdoor monitoring tanks, each holding 2 m3 of slurry were used to estimate the CH4 emissions from untreated cow manure (UCM), which served as the non-plasma treated control, and nitrogen enriched organic fertiliser (NEO). All tanks were equipped with a lid that remained open, except during the 40-min measurement period when the lid was closed and gas samples were drawn at 10-min intervals to estimate CH4 emission. Figure 1 shows a schematic of the system. Continuous measurements of the pH and temperature in all tanks were conducted the first year.
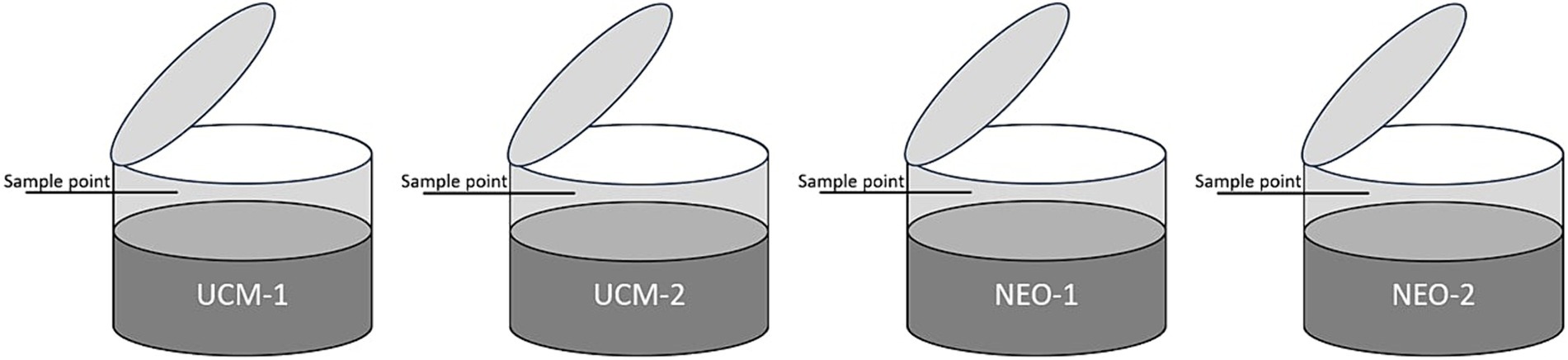
Figure 1. The four outdoor tanks had a retractable lid to ensure realistic exposure to the environment, except during the brief period of measurement. Samples were drawn through the sampling point with a syringe, transferred to a gas vial and the CH4 concentration was measured on a gas chromatograph.
The UCM originated from Skollenborg, Norway and was filtered with a screw press before being transferred to two of the tanks. The same filtered UCM was used as input material in the plasma process, which converts UCM to NEO. Figure 2 illustrates the plasma process and Table 1 shows the difference in chemical composition between the UCM and NEO (averaged over the three years). Each year, the tanks were drained before a new batch of UCM and NEO was transferred to the tanks, with approximately the same composition as the year prior.
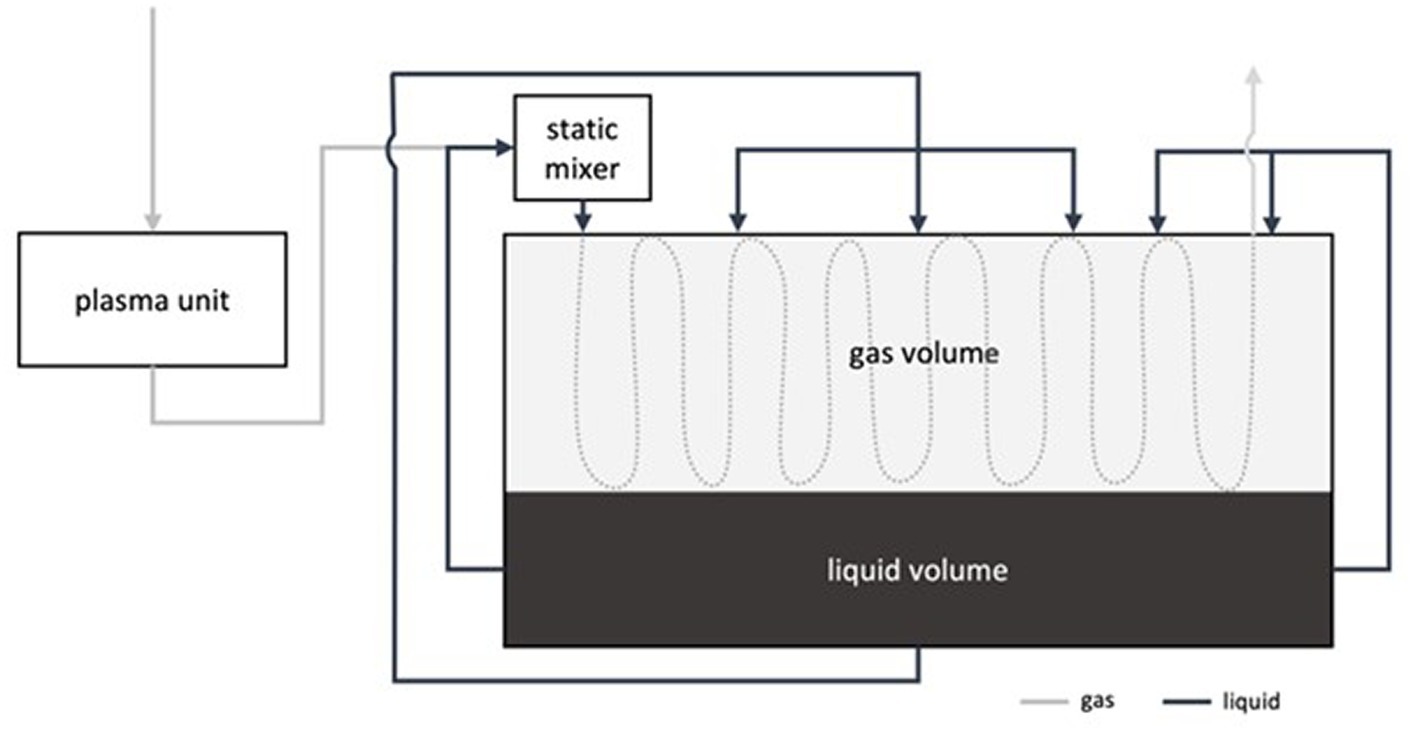
Figure 2. A schematic representation of the enrichment process. Air passes through the plasma generator, the resultant reactive nitrogen gas is subsequently absorbed in the organic substrate as NO3− and NO2−. The process is pH-controlled and produces material at a requested pH.
2.2 Laboratory experiment
In the laboratory experiment, the UCM was compared to UCM acidified with sulfuric acid (H2SO4) to pH 6.3 and pH 5.0 (UCMpH6.3 and UCMpH5.0) and to NEO at pH 6.3 and pH 5.0 (NEOpH6.3 and NEOpH5.0). Each UCM treatment was set up in nine gas tight serum-vials, the NEO treatment in three. Conditions in the vials were made anoxic by washing the bottles (repeated cycles of evacuation and He-filling while stirring the slurries) to accelerate methanogenesis. The vials were incubated at 30°C for 180 h in a water bath connected with a multi-column GC (Model 7890A, Agilent, Santa Clara, CA, USA) and a chemoluminescence NO analyser (Model 200 A, Advanced Pollution Instrumentation, San Diego, USA) via an autosampler and a peristaltic pump for repeated headspace analyses as described by Molstad et al. (2007). The methane concentrations in the headspace were measured six times a day.
Approximately halfway through the incubation, three vials from the UCM and UCMpH6.3 received a potassium nitrate (KNO3) dose resulting in a concentration of 1,000 mgNO3−- N/L, and another three vials received a potassium nitrate (KNO3) and potassium nitrite (KNO2) dose resulting in a concentration of 1,000 mgNO3−- N/L and 750 mgNO2−- N/L, which corresponds to the nitrogen oxyanion concentration in NEO. No CH4 formation was detected in the UCMpH5.0 vials. Therefore, no NO3− or NO2− was added to the UCMpH5.0 treatments. The setup aimed to determine whether pH, nitrate or nitrite, or a certain combination of the three, yielded a complete inhibition of methanogenesis or whether the inhibition is a result of particular properties of the plasma treatment.
3 Results and discussion
3.1 Farm-scenario experiment
Temperature readings suggested differences in microbial activity between the two treatments. As shown in Figure 3, the temperature at a slurry depth of 30 cm displayed higher peaks in UCM than in NEO, and more pronounced diurnal fluctuations, likely caused by higher microbial activity in the UCM that affected the slurry temperature, whereas air temperature was the main driver in NEO. However, the minima are lower in the UCM – resulting in comparable average temperature over the entire storage period.
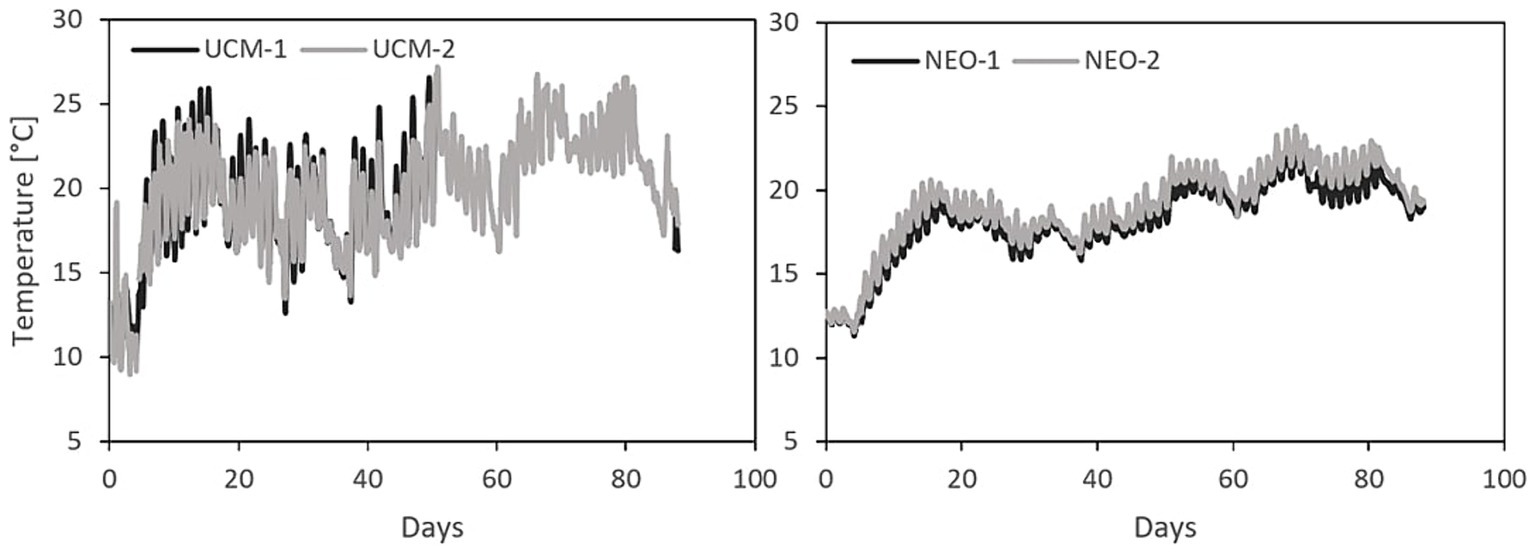
Figure 3. The temperature at 30 cm depth in all tanks, year 2 – ambient temperatures were used to determine the impact of temperature the following years.
The pH during storage also indicated a clear inhibition of microbial activity in NEO (Figure 4). The pH in NEO rose slowly from pH 5.3 to pH 5.9 throughout the 80 days of storage. The slow increase can be attributed to deprotonation of carbonates, combined with the reaction of NO2− with organic matter. The onset of microbial denitrification would have been associated with an abrupt pH increase and elevated temperatures as described by Hiis et al. (2023), suggesting an absence of denitrification in the stored NEO.
The apparent inhibition of microbial activity had a clear effect on the CH4 fluxes. Figure 5 shows the cumulative CH4 emissions for all tanks in the 2021–2023 measurement season, where the methane formation is completely inhibited by the nitrogen enrichment. A minimal yet consistent CH4 uptake is found in all NEO treatments.
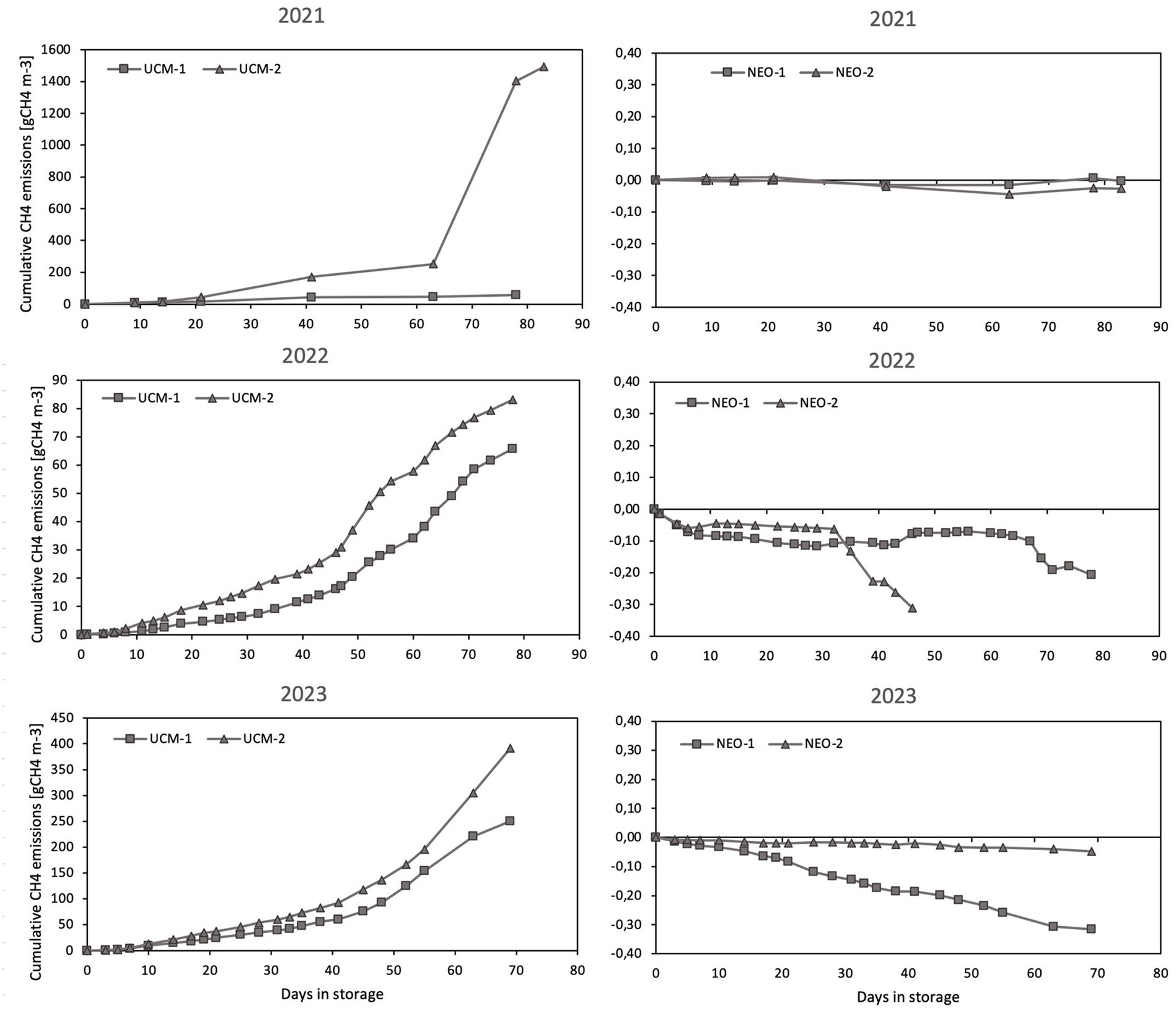
Figure 5. The cumulative CH4 emissions from all tanks over the three measurement periods. The tank lid-closing mechanism malfunctioned after 48 days in NEO-2 in 2022, which is why the measurements stop.
The low measurement frequency in 2022 did not yield an appropriate resolution of the fluxes, especially since the deviation in emissions between the two tanks in 2021 was large, mainly as a result of crust formation on UCM-1 and no crust formation on UCM-2. CH4 emissions were substantially higher in 2023 compared to 2022, a finding we currently have no explanation for, other than speculating that the annual temperature variations may have influenced the rate of CH4 formation. Despite emission-variation in the UCM, the reduction resulting from the plasma-based nitrogen enrichment was reliably above 100% throughout the storage periods. The complete emission reduction distinguishes the plasma-based nitrogen enrichment from acidification alone, which reports CH4 reductions in the range of 69 to 84% (Habtewold et al., 2018).
3.2 Laboratory experiment
The controlled incubation experiments demonstrated a pronounced effect of acidification and NO3− and NO2− addition on CH4 production, although their combinatory effect did not match that of the plasma treatment entirely. The unacidified UCM (pH 7.1) showed a steady methane formation, until either NO3− or NO3− + NO2− was added (Figure 6). The addition of NO3− + NO2− resulted in inhibition of methanogenesis throughout the incubation period, whereas the methane formation resumed in the NO3− treatment.
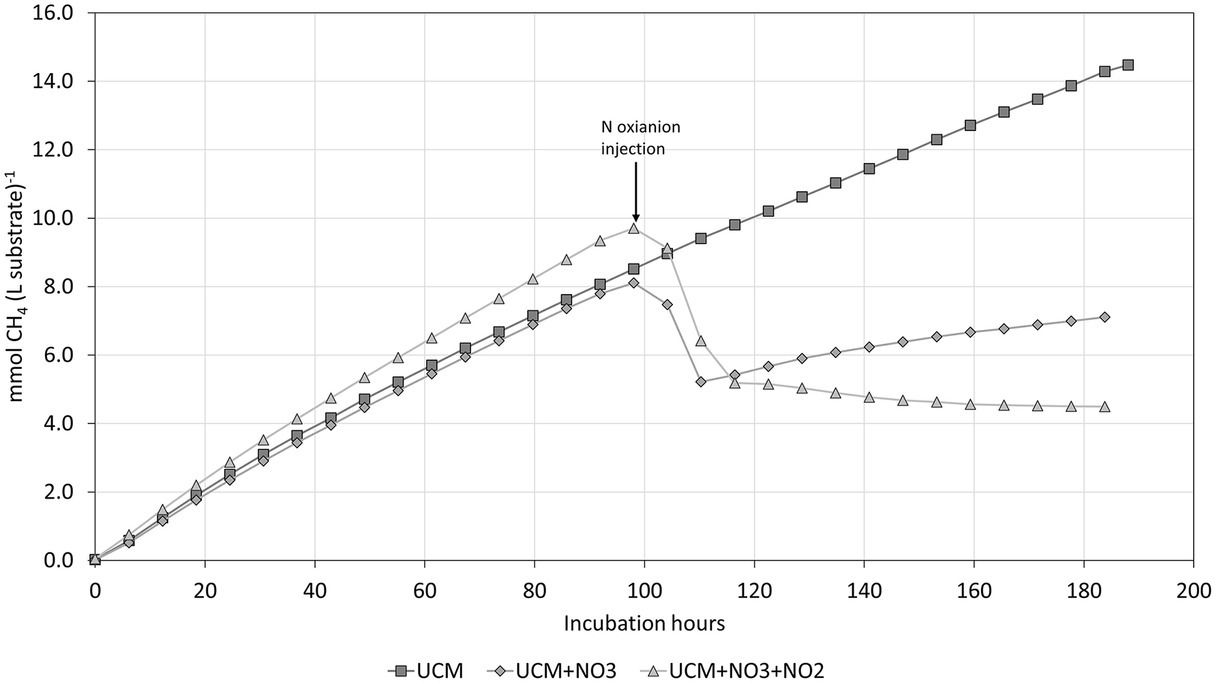
Figure 6. The cumulative CH4 formation in UCM, with and without elevated N oxyanion concentrations (injected after 98 h).
Both treatments where the nitrogen oxyanion concentration was artificially elevated resulted in denitrification, as was evident from N2 formation shown in Figure 7. The pronounced drop in CH4 concentration was unexpected; the increased formation of N2, N2O, NO and CO2 and could not entirely explain the reduced concentration. A potential explanation of the effect is denitrifying anaerobic methane oxidation (DAMO), as described by Wei et al. (2022), a thermodynamically favourable denitrification pathway where CH4 acts as electron donor and is oxidised to CO2.
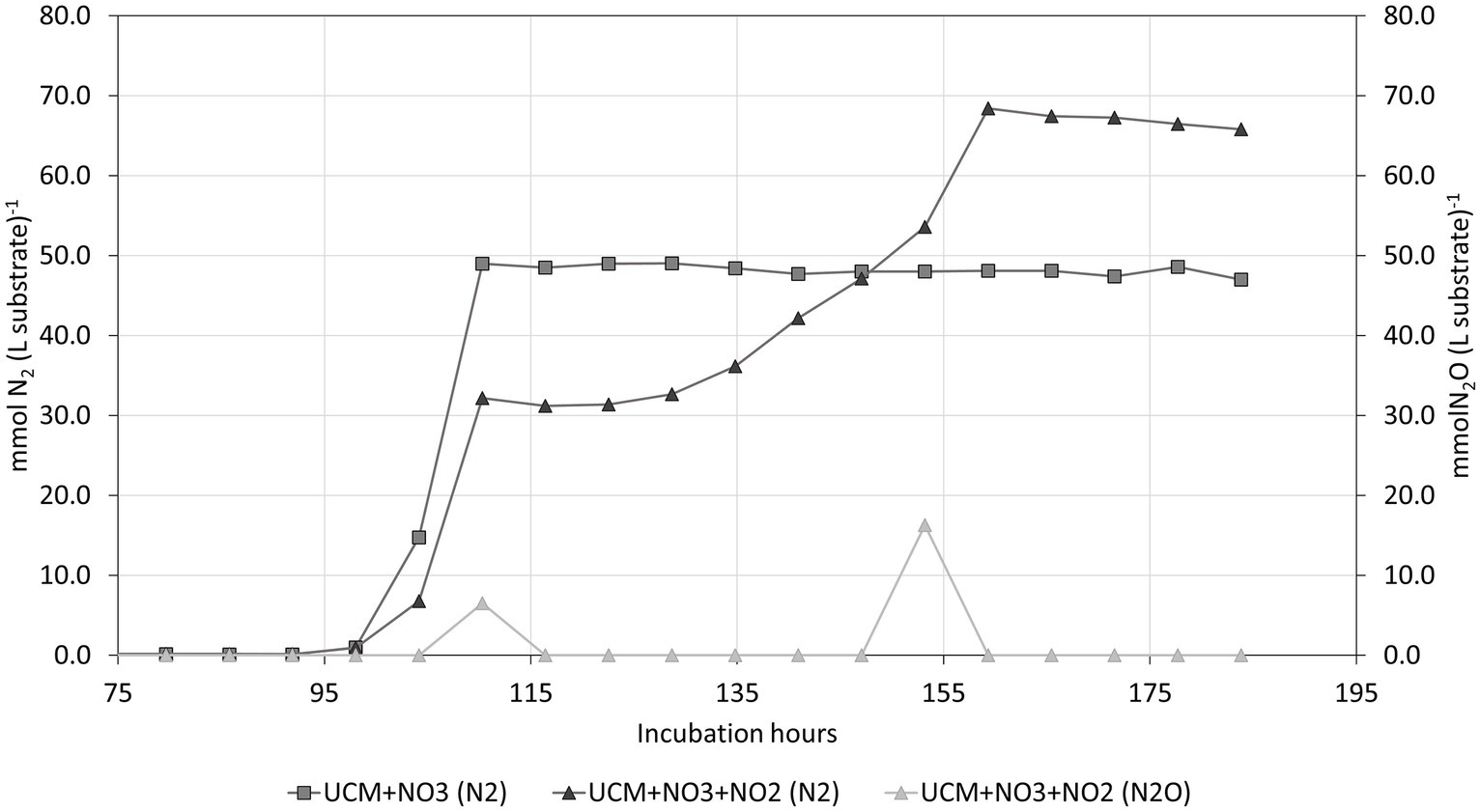
Figure 7. The N2 and N2O accumulation in the non-acidified treatments. All replicates displayed the same pattern, although shifted in time. Therefore, only one replicate of each treatment is displayed.
Figure 8 shows the impact of acidifying the UCM with H2SO4 to pH 6.3. Acidification alone resulted in a 45% reduction in CH4 accumulation. The nitrogen oxyanions were added in identical concentrations as in the prior experiment after 86 h. Both treatments resulted in complete inhibition of methanogenesis. NEO (UCM plasma treated to pH 6.3) showed a complete inhibition of methanogenesis throughout the experiment.
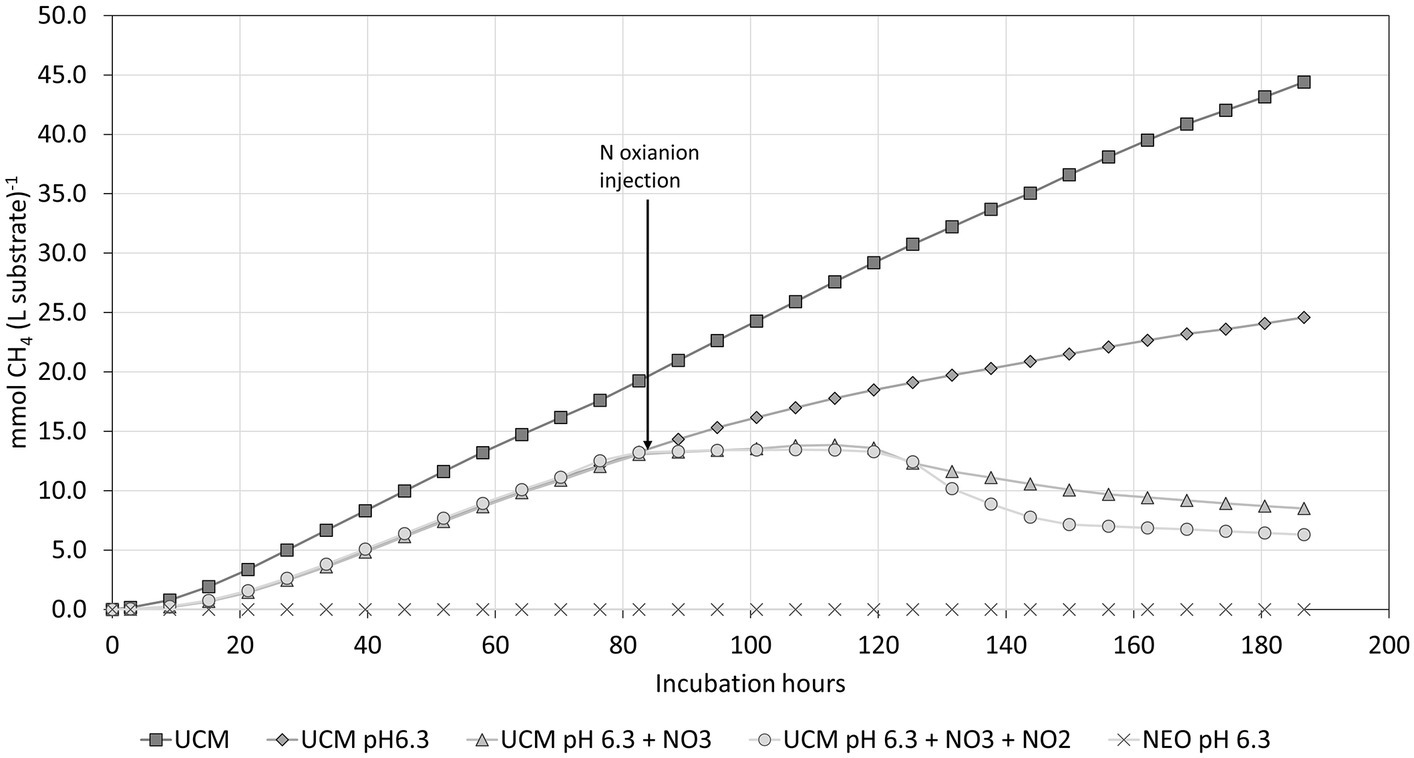
Figure 8. CH4 formation in the substrate acidified with H2SO4 to pH 6.3 compared to a non-acidified control (UCM). Nitrogen oxyanions were added after 86 h of incubation.
Both UCMpH6.3 + NO3 and UCMpH6.3 + NO3 + NO2 exhibited clear signs of denitrification. The pH at the end of the experiment was 8.1 and 8.2, which indicates that the alkalization occurred due to consumption of nitrogen oxyanions. In contrast, pH in NEO rose moderately from pH 6.3 to pH 6.5 and did not display the same N2O peaks as the comparable UCM treatments, as shown in Figure 9.
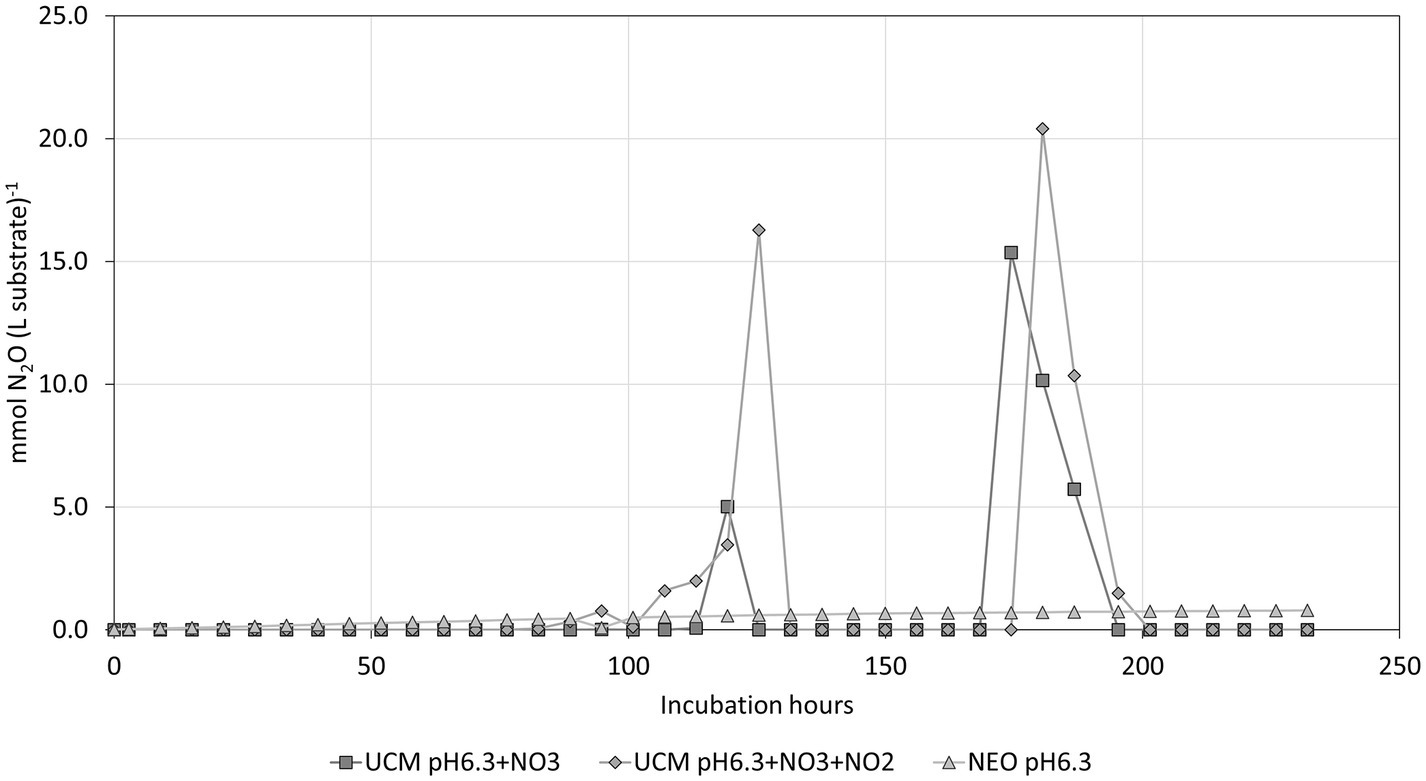
Figure 9. The N2O development show abrupt peaks during microbial denitrification compared to a low but steady N2O formation in NEO, which is likely a result of chemical interactions.
When acidifying the UCM to pH 5.0, no methane formation occurred over the duration of the experiment. However, the amount of H2SO4 required to reach this pH results in a sulphur content that often exceeds agronomic needs (5.4 kg 98%H2SO4 m−3). Targeting such low pH levels with sulfuric acidification should therefore be avoided.
The exact inhibitory mechanisms of plasma-based nitrogen enrichment cannot be determined by this set of experiments alone. However, some important observations can be made: the addition of nitrogen oxyanions suppresses CH4 formation, likely because of a shift to NO3− and NO2− as the favoured electron acceptors. Consequently, denitrifiers consume the nitrogen oxyanions whereafter methanogenesis is likely to resume (as in UCMNO3). Our results also indicate the occurrence of the DAMO process, as denitrification coincides with a reduction in the CH4 concentration that cannot be accounted for by dilution alone. Surprisingly, the NEO, even at a moderate pH of 6.3, did not result in the denitrification that occurred in its chemical imitations, suggesting that the plasma treatment itself has antimicrobial properties beyond the acidification and addition of nitrogen oxyanions. One hypothesis worthwhile exploring is that the plasma treatment occurs under aerobic conditions when the facultative anaerobic denitrifiers are more likely to respire oxygen and therefore cannot easily counter the elevated levels of undissociated nitrite (HNO2).
4 Conclusion
In this work we demonstrate that plasma-based nitrogen enrichment of cow manure inhibited methanogenesis completely in exposed outdoor storage tanks during the warm period of the year over three consecutive years. A laboratory experiment showed that the CH4 inhibition could be achieved by reducing the slurry pH below 5, or by amending the cow manure with nitrate and nitrite. However, the latter strategy resulted in rampant denitrification, which renders plasma-based nitrogen enrichment the only method by which both methanogenesis and denitrification can be avoided at moderately low pH.
Data availability statement
The raw data supporting the conclusions of this article will be made available by the authors, without undue reservation.
Author contributions
MN: Writing – original draft, Writing – review & editing. PD: Writing – original draft, Writing – review & editing.
Funding
The author(s) declare financial support was received for the research, authorship, and/or publication of this article. The authors acknowledge the financial support from the Norwegian Research Council (grant no. 309640).
Conflict of interest
The work was part of an industrial PhD where the MN works for a company that develops Plasma technology.
The remaining author declares that the research was conducted in the absence of any commercial or financial relationships that could be construed as a potential conflict of interest.
Publisher’s note
All claims expressed in this article are solely those of the authors and do not necessarily represent those of their affiliated organizations, or those of the publisher, the editors and the reviewers. Any product that may be evaluated in this article, or claim that may be made by its manufacturer, is not guaranteed or endorsed by the publisher.
References
Balde, H., Van der Zaag, A. C., Burtt, S. D., Wagner-Riddle, C., Crolla, A., Desjardins, R. L., et al. (2016). Methane emissions from digestate at an agricultural biogas plant. Bioresour. Technol. 216, 914–922. doi: 10.1016/j.biortech.2016.06.031
CARB (2020), California methane inventory – By IPCC category, CARB. Available at: https://ww2.arb.ca.gov/sites/default/files/classic/cc/inventory/ghg_inventory_ipcc_sum_2000-20ch4.pdf
Habtewold, J., Gordon, R., Sokolov, V., VanderZaag, A., Wagner-Riddle, C., and Dunfield, K. (2018). Reduction in methane emissions from acidified dairy slurry is related to inhibition of methanosarcina species. Front. Microbiol. 9:2806. doi: 10.3389/fmicb.2018.02806
Hiis, E., Nyvold, M., and Bakken, L. (2023). Inhibition of denitrification in nitrogen enriched organic fertiliser using plasma technology. Preprint. doi: 10.13140/RG.2.2.30579.50728
Holly, M. A., Larson, R. A., Powell, J. M., Ruark, M. D., and Aguirre-Villegas, H. (2017). Greenhouse gas and ammonia emissions from digested and separated dairy manure during storage and after land application. Agric. Ecosyst. Environ. 239, 410–419. doi: 10.1016/j.agee.2017.02.007
Hou, Y., Velthof, G. L., Lesschen, J. P., Staritsky, I. G., and Oenema, O. (2017). Nutrient recovery and emissions of ammonia, nitrous oxide, and methane from animal manure in Europe: effects of manure treatment technologies. Environ. Sci. Technol. 51, 375–383. doi: 10.1021/acs.est.6b04524
IEA (2022), Global methane tracker 2022, IEA, Paris. Available at: https://www.iea.org/reports/global-methane-tracker-2022, License: CC BY 4.0
Ingels, R., and Graves, D. B. (2015). Improving the efficiency of organic fertilizer and nitrogen use via air plasma and distributed renewable energy. Plasma Med. 5, 257–270. doi: 10.1615/PlasmaMed.2016015763
IPCC (2018). “Summary for policymakers” in Global warming of 1.5°C. An IPCC special report on the impacts of global warming of 1.5°C above pre-industrial levels and related global greenhouse gas emission pathways, in the context of strengthening the global response to the threat of climate change, sustainable development, and efforts to eradicate poverty. eds. V. Masson Delmotte, V. Masson Delmotte, P. Zhai, H. O. Portner, D. C. Roberts, and J. Skea, et al. (Geneva, Switzerland: World Meteorological Organisation)
Kuylenstierna, J. C., Michalopoulou, E., and Malley, C. (2021). Global methane assessment: Benefits and costs of mitigating methane emissions.
Lamers, L. P., Tomassen, H. B., and Roelofs, J. G. (1998). Sulfate-induced eutrophication and phytotoxicity in freshwater wetlands. Environ. Sci. Technol. 32, 199–205. doi: 10.1021/es970362f
Lynch, J., Cain, M., Pierrehumbert, R., and Allen, M. (2020). Demonstrating GWP*: a means of reporting warming-equivalent emissions that captures the contrasting impacts of short-and long-lived climate pollutants. Environ. Res. Lett. 15:044023. doi: 10.1088/1748-9326/ab6d7e
Molstad, L., Dörsch, P., and Bakken, L. R. (2007). Robotized incubation system for monitoring gases (O2, NO, N2O N2) in denitrifying cultures. J. Microbiol. Methods 71, 202–211. doi: 10.1016/j.mimet.2007.08.011
OECD-FAO (2022). OECD-FAO Agricultural Outlook 2022-2031, OECD Publishing, Paris. doi: 10.1787/f1b0b29c-en
Rodhe, L., Alverbäck, A., Ascue, J., Edström, M., Nordberg, Å., Pizzul, L., et al., (2018). Åtgärder för att minimera växthusgasutsläpp från lager med rötad och orötad gödsel. Retrieved from https://urn.kb.se/resolve?urn=urn:nbn:se:ri:diva-33858
Keywords: nitrogen fixation, plasma, methane emissions, sustainable livestock production, organic fertiliser
Citation: Nyvold M and Dörsch P (2024) Complete elimination of methane formation in stored livestock manure using plasma technology. Front. Sustain. Food Syst. 8:1370542. doi: 10.3389/fsufs.2024.1370542
Edited by:
Tom Misselbrook, Rothamsted Research, United KingdomReviewed by:
Nikolaos Vasileios Paranychianakis, Technical University of Crete, GreeceSven Gjedde Sommer, Aarhus University, Denmark
Copyright © 2024 Nyvold and Dörsch. This is an open-access article distributed under the terms of the Creative Commons Attribution License (CC BY). The use, distribution or reproduction in other forums is permitted, provided the original author(s) and the copyright owner(s) are credited and that the original publication in this journal is cited, in accordance with accepted academic practice. No use, distribution or reproduction is permitted which does not comply with these terms.
*Correspondence: Peter Dörsch, cGV0ZXIuZG9lcnNjaEBubWJ1Lm5v