- 1College of Engineering, Northeast Agricultural University, Harbin, China
- 2Guangzhou Institute of Energy Conversion, Chinese Academy of Sciences, Guangzhou, China
- 3Key Laboratory of Swine Facilities Engineering, Ministry of Agriculture, Harbin, China
- 4Department of Chemical Engineering, Waterloo Institute for Nanotechnology, University of Waterloo, Waterloo, ON, Canada
- 5Jiangsu Weiguang Biotechnology Co., Ltd., Zhenjiang, China
- 6Huzhou Key Laboratory of Medical and Environmental Applications Technologies, School of Life Sciences, Huzhou University, Huzhou, China
Introduction: Animal waste proteins have been increasing in the past decade, along with consumer demands. Their huge volume and the environmental issues caused by improper treatment probably pose a massive threat to human health. These animal waste proteins contain many valuable bioactive peptides and can be used not only as nutrient substances but also as primary functional ingredients in many industries, including agriculture, food, and pharmaceuticals. However, the advancement of the value-added application of animal waste proteins within the past 10 years has not been elucidated yet. In this regard, this paper scrutinized the studies on the applications of hydrolysates and peptides from animal waste proteins throughout the last decade, hoping to display a whole picture of their value-adding applications.
Methods: The Web of Science and Google Scholar were searched from January 1, 2013, to December 12, 2023. This review included field trials, in vitro and in vivo assays, and in silico analysis based on literature surveys or proteolysis simulation. The quality of the included studies was evaluated by Journal Citation Reports, and the rationality of the discussion of studies included.
Results: Numerous studies were performed on the application potential of hydrolysates and peptides of animal waste proteins in agricultural, food, and medicinal industries. Particularly, due to the nutritional value, safety, and especially competitive effects, the peptide with antioxidant, antimicrobial, antihypertensive, antidiabetic, or antithrombotic activities can be used as a primary functional ingredient in food and pharmaceuticals.
Discussion: These value-added applications of animal waste proteins could be a step towards sustainable animal by-products management, and simultaneously, open new avenues in the rapid development of nutraceuticals and pharmaceuticals. However, further studies on the bioavailability and structure-activity relationship are required to verify their therapeutic effects.
1 Introduction
Over the past few years, there has been a significant surge in the global intake of high-protein foods (Peydayesh et al., 2022). When the meat industry and slaughterhouses yield a tremendous amount of meat products, a copious supply of protein-rich by-products is also produced. These animal by-products are frequently considered to be low-value and therefore discarded (Chakrabarti et al., 2018; Etemadian et al., 2021; Yao et al., 2022; Yang et al., 2023). However, it is worth noting that these by-products contain a diverse range of highly valuable bioactive compounds. Recently, there has been a significant upswing in the research of bioactive peptides derived from animal waste proteins. These peptides have been discovered to possess distinctive properties and intricate compositions with exceptional potential in diverse industries, including but not limited to agriculture, nutraceuticals, pharmaceuticals, and cosmetics (Korhonen and Pihlanto, 2006; Giordano et al., 2018; Karami and Akbari-Adergani, 2019; Phadke et al., 2021). As a result, researchers are keenly exploring their applications in various fields, intending to meet the continuous need for protein and unlock their full potential (Owji et al., 2018; Chavez and Uchanski, 2021; Lee S. Y. et al., 2021; Lee S. et al., 2021; Madhu et al., 2022).
Animal waste proteins can be one of the best sources of bioactive peptides, which are crucial molecules and may exert physiological effects in life (Wadhwa and Bakshi, 2016; Peydayesh et al., 2022; Timorshina et al., 2022). Animal waste proteins are mainly obtained from by-products or unused parts of animals from slaughterhouses after their primary processing for food production, such as skin, bones, cartilage, tendons, organs, trimmings, and other components that are not used for human consumption (Mora et al., 2014; Zhao et al., 2021). Nowadays, engineers and researchers are working hard to give value to these waste proteins by converting them into functional ingredients and new valuable products with high potential human health value and, at the same time, to reduce environmental pollution caused by them (dos Santos et al., 2021; Li et al., 2021; Martinez-Burgos et al., 2021; Norouzi et al., 2022). The hydrolysates and potential benefits of these animal proteins are immense and far-reaching for humanity and sustainability (Wadhwa and Bakshi, 2016; Peydayesh et al., 2022). Therefore, it is crucial to explore and implement ways in which animal waste proteins can be utilized to the fullest extent to support sustainable development and improve our overall quality of life (Cheung et al., 2015).
Several methods were used to generate the desired proteins and peptides, including direct extraction, chemical methods, enzymatic hydrolysis, and microbial fermentation (Pagán et al., 2021; Wen et al., 2023b). However, the choice of the method for the hydrolysis of proteins usually depends on their sources. The enzymatic hydrolysis and microbial fermentation methods were demonstrated to improve the solubility, viscosity, emulsification, and gelation propriety of peptides generated. These methods improved the peptide’s nutritional quality, which may hold significant advantages for human health by reducing any associated factors that affect their applications (Marciniak et al., 2018; Zhu et al., 2022). The peptides unlocked from parent proteins can boost the immune system, improve digestion and adsorption of food, reduce inflammation, and promote the regeneration of cells and tissues (like skin and hair), remarkably improving our quality of life (Ullah et al., 2018; Wang B. et al., 2021). Furthermore, these peptides can be used as food additives, like natural preservatives and nutrition enhancers (Chi et al., 2015a; Nielsen et al., 2017).
To date, due to the strengthening of environmental protection policies, resource scarcity, and food security, livestock and aquaculture industries are meeting the harmless treatment and resource application problems of animal waste proteins, which attract the most interest of scientists to find better ways to solve them. Nowadays, much research has improved the enzymatic hydrolysis or microbial fermentation methods to produce various protein or peptide-based products, including food for both humans and animals, medicine, fertilizers, and antibiotics (Korhonen and Pihlanto, 2006; Dai et al., 2016; Minj and Anand, 2020). To show a whole picture of the application potential of animal waste proteins and the bioactive peptides derived from them, this study focuses on the production method for bioactive peptides derived from protein-rich animal wastes and their applications in agriculture, food industry, and medicine (Figure 1).
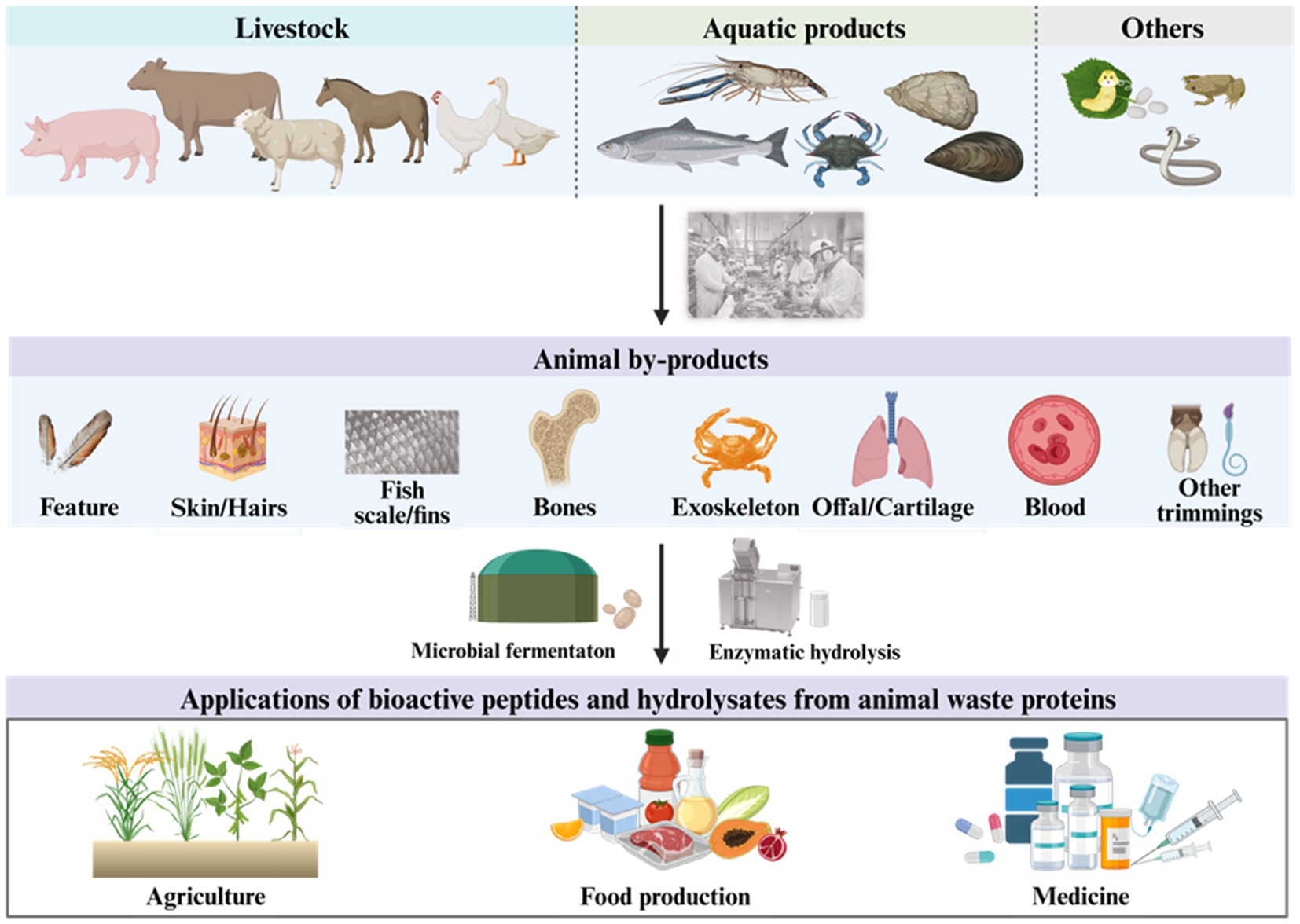
Figure 1. Schematic of the valorization of animal waste proteins. Created with BioRender.com.
2 Methods
2.1 Literature search
For the purpose of the review, Web of Science and Google Scholar were searched for all published studies. During searching, the following topics were used for each section: “livestock,” “meat by-product,” “aquatic by-product,” “fish waste,” “food processing waste,” “animal waste protein” or “waste animal protein” for sources of animal waste proteins; “animal waste protein” or “waste animal protein,” “burning” or “combustion,” “burying” or “burial,” “rendering,” and “compost” or “composting” for the traditional treatment of animal waste proteins; “microbial fermentation” or “fermentation” and “enzymatic hydrolysis” or “hydrolysis” for biotechnological methods for releasing peptides from animal waste proteins; “agricultural application,” “plant growth promotion,” “abiotic stress tolerance” or “heat stress” or “salinity stress” or “drought stress,” “biotic stress tolerance” or “resistance to microorganism” or “resistance to fungi/bacteria/virus,” and “animal waste proteins” for agricultural application; “food addictive,” “functional food,” “enzyme in gastrointestinal system,” and “animal waste proteins” for food application; “medicinal application” or “pharmaceuticals,” “bioactivity” or “antihypertensive” or “antioxidant” or “antimicrobial” or “antidiabetic” or “antithrombotic,” “peptides,” and “animal waste protein” for medicinal application. The data range was restricted in the past decade (January 1, 2013 to December 12, 2023). Furthermore, a backward citation search was performed for the searched articles.
2.2 Study selection
The searched articles were all imported into Endnote 20 (Clarivate Analytics, United States). After removing the duplicate records, two investigators independently screened the titles and the abstracts of all the retained articles, according to the inclusion and exclusion criteria. After excluding irrelevant articles in the initial screening, the same two investigators carefully read the relevant sections of the retained articles and extracted the useful information. Any disagreements were resolved by consulting other authors.
Studies were included if they: (1) involved the source, treatment, or application of animal waste proteins; (2) involved the direct use of peptides or other components from animal waste proteins in field studies, in vitro studies, and in vivo studies, but had no description of the treatment of animal waste proteins; (3) were the statistical analysis based on database or computer simulation using a software; (4) were the latest reviews that can provide a part of data to this review or the relevant reports from influential governments and international organizations. Studies were excluded if they: (1) were related to this review, but the argument of the relevant section is not tenable. (2) investigated the application of the hydrolysates and peptides from animal waste proteins other than in agriculture, food, and medicinal industries; (3) investigated the health-promoting effects of the hydrolysates and peptides from animal waste proteins other than antihypertension, antioxidant, antimicrobial, hypoglycemic, and antithrombosis. The statistical data of the number of articles used for this study is shown in Figure 2.
3 Sources of animal waste proteins
Animal waste proteins are mainly from the by-products of meat and aquatic products processing industries. According to the National Bureau of Statistics of China (National Bureau of Statistics of China, 2023), in 2020, the raw meat yield (including pork, beef, mutton, and poultry meat) was 7.639 million tons. The aquatic product yield in 2020 was 6.549 million tons (Ministry of Agriculture and Rural Affairs of the People’s Republic of China, 2020). The production of raw meat and aquatic food was accompanied by large amounts of animal wastes and fish wastes, which caused burdensome disposal problems and environmental concerns. For instance, the meat yield percentage for pork is around 72–80%, while for beef it is 50–60%. Since disposal costs and efficiency are previously prioritized, these wastes are directly burned or buried. Later, people realized that the lipids in the animal and fish products processing wastes could be recovered for animal feeds, cosmetics, etc., and thus used as a raw material for the rendering system. Besides, agriculturists found that the animal and fish products processing wastes could be converted to small-molecular organic compounds, like humus, through microbial metabolism under favorable conditions. Therefore, agriculturists compost the animal and fish products processing wastes.
3.1 Meat source
A huge amount of waste is generated during meat product processing due to the rapid growth of meat consumption throughout the world. These wastes can be classified into two groups: liquid blood and solid bones and trimmings. The blood consists of plasma and blood cells and is rich in proteins. After separation and hydrolysis or extraction, the protein hydrolysate, bioactive peptides, and especially thrombin, fibrinogen, heme iron peptide, and globin, can be obtained (Figure 3). These extracted proteins or peptides are beneficial for human health as dietary supplements or pharmaceuticals.
The trimmings and bones are rich in fat and proteins. Mature beef cattle or pigs have skeletal muscles that contain roughly 70% protein on a dry-matter basis (Sun et al., 2016; Bravo et al., 2023). The trimmings include skin, hair/bristle, feathers, horns, hooves, tails, viscera/cartilage, and deboning residues (Wadhwa and Bakshi, 2016; Marciniak et al., 2018). Through the rendering technique, the lard/tallow, fat, chemicals, pharmaceuticals, and animal feeds can be produced using trimmings. The lard/tallow and fat can be further processed into biodiesel through an esterification reaction, which helps to alleviate not only the environmental concerns but also the energy crisis. Due to being rich in proteins and other nutrients [like minerals (Tran et al., 2020)], the trimmings are one of the best raw materials to extract collagens and the bones can be ground into powder to produce animal feeds.
3.2 Aquatic source
The fish processing industry has a meat yield percentage of about 60%. The fish products processing waste includes skin, scale/fin, head, viscera (like liver), roes, bones, exoskeletons, shells, and carcasses (Ahn et al., 2014; Sila et al., 2014; Silva et al., 2014; Chi et al., 2015c). These wastes often contain protein-rich materials, which are typically processed into the animals’ dietary supplements and feeds, fish meal, and fertilizers (Subhan et al., 2021). However, these products do not make full use of the value of the fish products processing wastes because some proteins and peptides derived from these wastes through enzymatic hydrolysis can play a huge role in treating chronic diseases (Lee and Hur, 2017; Phadke et al., 2021; Ucak et al., 2021). For example, the protein hydrolysate obtained by hydrolyzing the stomach and intestine of smooth hound sharks by Purafect, Esperase, and Neutrase, exhibited a good therapeutic effect on hypertension, cancer, and infections (Abdelhedi et al., 2016). Compared to the proteins and peptides derived from meat processing sources, those derived from aquatic sources characterized by short chain length, the presence of lysine or arginine at the C-terminal, and possessing more hydrophobic amino acids, exhibit a high capability of regulating blood pressure and immune system and killing microorganisms (Ngo et al., 2016). For instance, the gelatin obtained by using Alcalase to hydrolyze giant squid (Dosidicus gigas) exhibited an extremely high angiotensin-converting enzyme inhibitory (ACE-I) ability (IC50 = 0.34 mg/mL) and those obtained by using Esperase exhibited an extremely high cytotoxic effect on cancer cells (IC50 = 0.13 mg/mL for human breast carcinoma and IC50 = 0.10 mg/mL for glioma cell lines). These two gelations were mostly composed of peptides with molecular weights of 500–1,400 Da.
4 Traditional treatment methods for animal waste proteins
4.1 Burning process
Burning or incineration reduces the volume of animal products processing wastes and animal carcasses volume by converting them to ash, which is very beneficial concerning limited waste disposal space. The volume of solid wastes can be reduced by over 90% by burning (Yamamoto et al., 2018; Velusamy et al., 2020). Besides, burning has two other great advantages. The one is that high temperatures destroy pathogens (e.g., Escherichia coli and Salmonella sp.), mitigating disease transmission risks (Franke-Whittle and Insam, 2013; Mozhiarasi and Natarajan, 2022). It is because the temperature during burning is usually maintained at 850–1,200°C to thermally decompose the animal by-products and carcasses completely. The other is that burning generates heat energy, which can be used to supply heat directly or generate electricity by steam turbines to satisfy the heating and electricity demands of livestock cultivation, aquaculture and even surrounding. However, burning has a disadvantage of air pollutants release. The pollutants produced during burning animal products processing wastes include particulate matter, noxious gases (sulfur dioxide and nitrogen oxides), odors, and potentially toxic substances, depending on the composition of the wastes. Therefore, incinerators should guarantee the purification of exhaust gases to reduce secondary environmental pollution and comply with local environmental regulations and emission control policies. Considering burning animal by-products and carcasses can raise local public concerns about air pollution, community engagement, transparency, and emission control technologies are essential for its public acceptance. Besides, animal by-products and carcasses contain many valuable substances with high commercial application potential, like protein and fat. Thus, Burning is not a particularly good method of disposal.
4.2 Burying process
Burying animal by-products and carcasses in the soil allows them to decompose naturally. This method emits less greenhouse gas and particulate matter compared to burning. The process involves digging a hole, placing the waste by-products or carcasses inside, and covering it with soil. The time it takes for buried objects to decompose varies depending on several factors, including the type of buried objects, burial depth, and burial site conditions. Burying offers several advantages, including quick disposal, reduced costs and logistical challenges, and enhanced soil structure and organic matter content (Yuan et al., 2013). However, the natural decomposition is slow and thus the resultant low release of nutrients will limit the agronomic benefits of buried animal by-products and carcasses as a nutrient source for crops or soil improvement. It also has potential disadvantages of land occupation, groundwater contamination, odor issues, disease transmission, and regulatory compliance requirements (Kim and Kim, 2017). Burying animal by-products and carcasses is banned in the European Union and some states of the United States. Although burying is permitted in some countries, strict regulations are implemented, like Scotland (Agriculture and Rural Economy Directorate of Scotland, 2023). Proper techniques are crucial for burying animal by-products and carcasses to avoid environmental contamination and disease spread. The proper techniques involve selecting appropriate burial sites, ensuring proper ventilation, monitoring the site regularly for potential environmental contamination, and handling and disposing of protective gear properly during the burial process to prevent disease spread.
4.3 Rendering process
Burning and burying are two simple and fast methods for disposing of animal by-products and carcasses, but not ideal methods because these wastes and mortalities contain various valuable components, for instance, proteins and fat (McGauran et al., 2021; Pagán et al., 2021). In contrast, the rendering process is favorable for recovering these valuable components for value-added applications. The basic process of rendering is shown in Figure 4. This process can be classified into wet rendering and dry rendering, which both include heating (or cooking), pressing, separation, and drying stages (Adewale et al., 2015). The difference between the two kinds is that hot water or hot steam is used to pressurize feedstocks to separate fats in wet rendering, while direct heating is used in dry rendering (Shi and Ge, 2020). Dry rendering is the most commonly used method to convert animal by-products and mortalities to useful industrial, agricultural, and pharmaceutical materials. After the heating and pressing stages, the proteins and lipids are mostly separated. The obtained lipids (including fats and oils) include tallow, grease, poultry fast, and lard, and are good raw materials for the oleochemical industry to produce animal feed, soap and cosmetics, pet food, and more recently to produce biofuels (Mekonnen et al., 2016). The use of them to produce biodiesel can be realized through microemulsions, pyrolysis (Ben Hassen-Trabelsi et al., 2014), and transesterification reactions (Emiroglu et al., 2018; Keskin, 2018). The obtained proteins can be used to produce bio-based plastics, wood adhesives, surfactants, firefighting foams, and flocculants (Thakur et al., 2023). Therefore, the rendering process can be considered as a sustainable strategy of resource utilization, not only mitigating the environmental contamination caused by animal by-products and mortalities but also fully utilizing these discards. Due to the high temperature (up to 100°C), animal-borne pathogenic microbes [like Listeria monocytogenes and Salmonella species (Karyotis et al., 2017)] can be killed during rendering, but prion proteins that can cause transmissible spongiform encephalopathies cannot be destroyed. Consequently, certain cattle tissues, like the brain and spinal cord, denoted as the specified risk materials (SRM) due to the highest possibility of carrying prion proteins, are banned from rendering industries to produce protein-and fat-rich materials (Mekonnen et al., 2016).
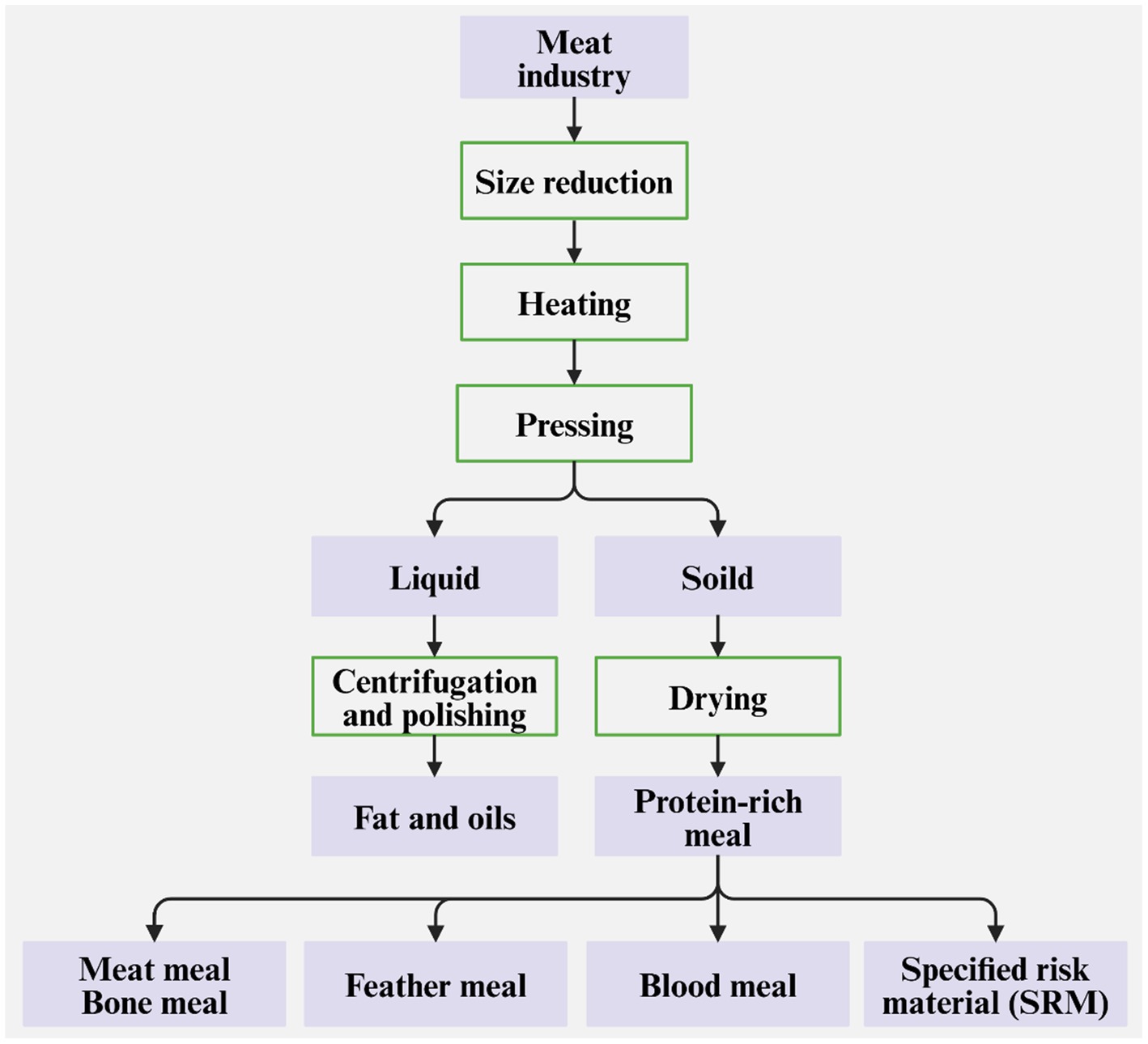
Figure 4. Basic process flow of rendering to recover fats, oils, and protein-rich meal products (Mekonnen et al., 2016).
4.4 Composting process
Composting is a sustainable and natural processing method to convert organic materials, including animal by-products and carcasses, food scraps, yard waste, and biogas residues, into soil amendment or fertilizers by passive or active methods (Gooding and Meeker, 2016; Lim et al., 2017). The passive methods include static piles and turned windrows, while the active ones include aerated static pile systems and in-vessel systems. The regular turning of windrows is one of the most important management techniques for composting, aimed at supplying enough oxygen for aerobic microorganisms (Hong et al., 2014). Regular turning can also expedite water evaporation and maintain proper temperature ranges for compost piles, promoting the maturity of compost piles and making the humus crumblier. Since the anaerobic fermentation inside piles is avoided by turning, the odors and potential nuisances for nearby communities are minimized. However, the regular turning of windrows is laborious and time-consuming (Wan et al., 2022). The time required ranges from months to a year, depending on materials, pile size, and desired decomposition. Thus, it requires sufficient space for piles, which may be a challenge for facilities with limited land availability.
During composting, animal by-products and carcasses are decomposed into simple or dissolved inorganic materials under aerobic microorganisms [mainly bacteria like Actinomycetes and Bacteroidetes (Huhe et al., 2017)] and finally converted into stabilized organic matter (compost or humus), which is a dark, crumbly substance rich in organic matter and essential nutrients like nitrogen, phosphorus, and potassium (Thomson et al., 2022). Therefore, it is a great soil amendment to ameliorate the fertility, structure, and moisture-holding capacity of soil and promote crop growth. To meet the needs of microorganisms’ metabolism and promote the maturity of compost piles, other organic materials, like sawdust (Michalopoulos et al., 2019), are usually mixed with animal by-products and mortalities for the carbon-nitrogen (C/N) ratio adjustment. Microorganism agents are also inoculated into compost piles (Gaind, 2014). With the use of humus, chemical fertilizers can be minimized, contributing to maintaining favorable physicochemical properties [like pH (Fleming et al., 2013)], nutrition, and structure for soil and sustainable agriculture practices. Moreover, by diverting from landfills or open-air storage, composting helps reduce the emissions of odors and volatile compounds during the breaking of organic matter, avoiding waterbody eutrophication and mitigating air pollution. As with the rendering, a properly managed composting process can effectively inactivate most pathogens, parasites, and weed seeds present in the wastes to be processed, for example, Escherichia coli, Ascaris eggs, cockspur grass seeds (Khadra et al., 2021; Rai et al., 2021), due to elevated temperature and pH (Lepesteur, 2022).
5 Biotechnological methods for extracting peptides from animal waste proteins
Although fat, proteins, minerals, and other organic matter are recovered or used based on rendering or composting processes, the values of these substances have not been fully reflected in the obtained products, especially the proteins. Numerous studies indicated that the protein hydrolysates of animal by-products contain various bioactive peptides, like antihypertensive peptides and antioxidant peptides, which are most beneficial for disease treatment (Vázquez et al., 2020; Ramakrishnan et al., 2023). Thus, using animal waste proteins as a source of bioactive peptides is a way to further expand their application range, transforming them into more valuable and profitable products than meat meals. Currently, two biotechniques, enzymatic hydrolysis, and microbial fermentation, have been considered to be the most valuable for decoding bioactive peptides from precursor proteins (Cruz-Casas et al., 2021). Their applications in valorizing animal waste proteins are detailed below (see Table 1).
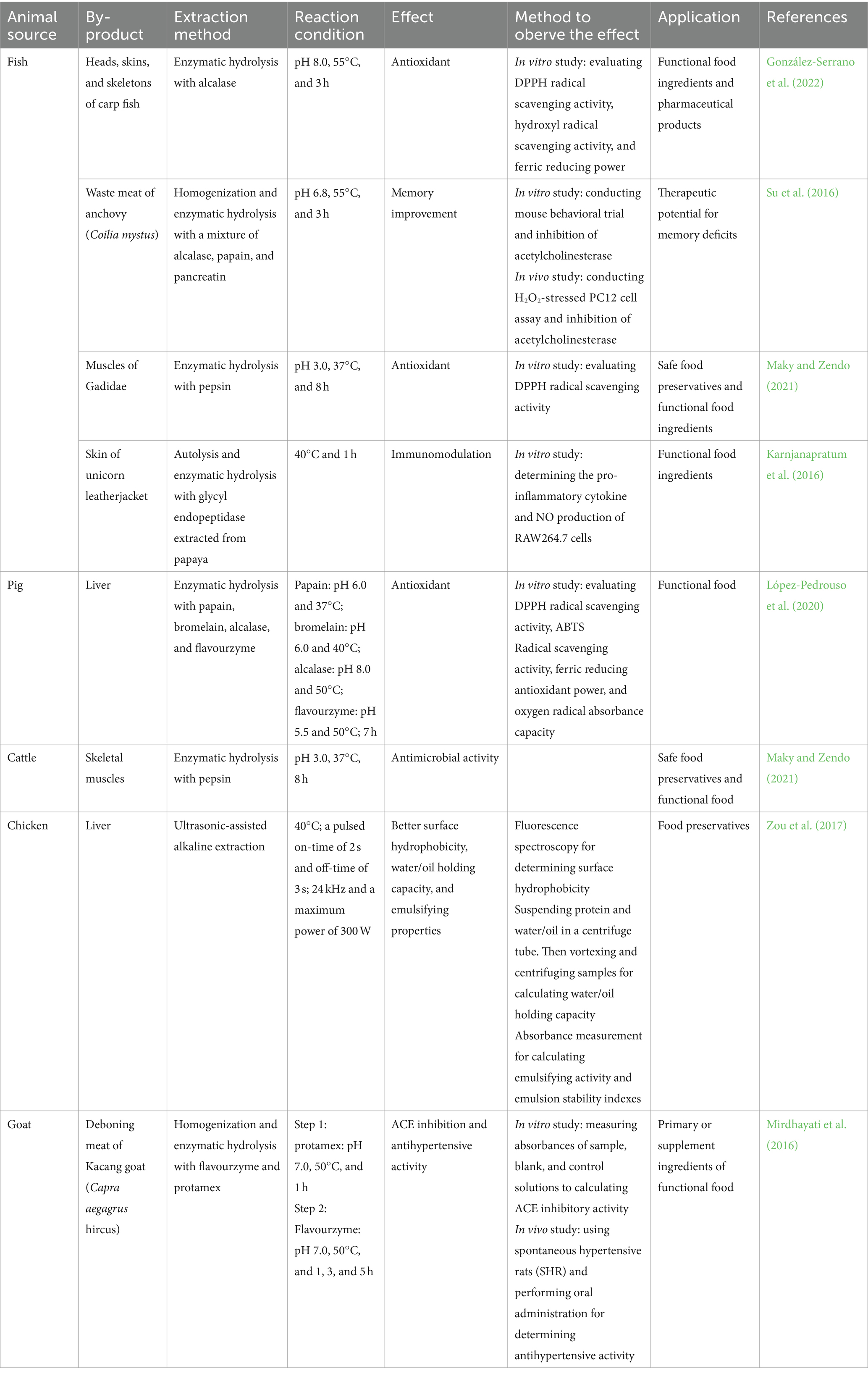
Table 1. Some application examples in the food industry of bioactive peptides and hydrolysates from animal waste proteins.
5.1 Microbial fermentation
Microbial fermentation is the other typical biotechnological method to release bioactive peptides from animal waste proteins. It utilizes the proteolytic enzymes synthesized by indigenous or inoculating microorganisms during their metabolism to break down proteins into small molecules and release the peptides and amino acids (Nasri et al., 2022; Wen et al., 2023b). Therefore, the use and control of microorganisms is one of the most important factors for realizing complete hydrolysis of proteins and obtaining peptides with high bioactivity.
The microorganisms involved in fermentation are bacteria and fungi. Among the bacteria, the lactic acid bacteria are the most beneficial because of their safety, high proteolytic ability, and high adaptability (Cruz-Casas et al., 2021). Lactic acid bacteria are found not only in nature but also in people’s digestive systems, for example, Lactobacillus acidophilus and Lactobacillus Casei (Krasaekoopt and Watcharapoka, 2014). It has been recognized as “generally recognized as safe” (GRAS) by the U.S. Food and Drug Administration (FDA) and has been used in food industries since the 1940s. The commonly used lactic acid bacteria genera include Carnobacterium, Enterococcus, Lactobacillus, Lactococcus, Leuconostoc, Oenococcus, Pediococcus, Streptococcus, Tetragenococcus, and Weissella in food fermentations. The protein hydrolysis by lactic acid bacteria is generally divided into three steps: first, casein is broken into oligopeptides by cell envelope protease. Second, oligopeptides are transported into lactic acid bacteria cells by transporters, including permeases, ABC transporters, and antiports (Lorca et al., 2015). Finally, oligopeptides are hydrolyzed into small peptides or free amino acids by intracellular endopeptidases (e.g., calpins and cathespins) and exopeptidases (e.g., aminopeptidases) (Zou et al., 2023). Some lactic acid bacteria are widely employed in research due to their efficient hydrolysis performance, and they are Lactococcus lactis, Lactobacillus helveticus, and Lactobacillus delbrueckii ssp. Bulgaricus. For instance, L. lactis RQ1066 had a degree of hydrolysis of mung bean milk of 16.62 ± 0.75% after 24 h fermentation and 18.45 ± 0.29% after 48 h fermentation at room temperature (Liang et al., 2022). Lactic acid bacteria can adapt to various environments and change their metabolism accordingly. It was reported that a typical lactic acid bacteria is aerotolerant, acid-tolerant, organotrophic, and a strictly fermentative rod or coccus (König and Fröhlich, 2017). Compared to bacteria, fungi also have been used to ferment animal by-products but not so common due to the limited source and proteolytic activity (Sadh et al., 2018; Cruz-Casas et al., 2021).
The fermentation conditions and time should be carefully controlled to realize high protein hydrolysis of animal waste proteins and obtain peptides with high bioactivities. This is because fermentation conditions significantly affect the metabolism of microorganisms, and microorganisms will continue to break bioactive peptides for growth if fermentation time exceeds the optimal time length. The condition generally involves temperature, pH, moisture, nutrients, etc. (Melini et al., 2019). For instance, the prevailing anaerobic condition, low initial pH, and higher salt and sugar facilitate the growth of lactic acid bacteria. However, in some unsmoked meat products that should have a sour taste, the amount of the added lactic acid bacteria, sugar level, and water activity are carefully controlled (Kumar et al., 2017).
Compared to enzymatic hydrolysis, microbial fermentation is a more inexpensive biotechnique to extract bioactive peptides from animal waste proteins. This is because the microorganisms used and their culture processes are not costly (Akbarian et al., 2022). Besides, the microorganisms secrete an entire set of proteases, instead of one or several, which makes proteins in substrates adequate and shortens the production cycle of peptide-based products (Song et al., 2023). Additionally, if lactic acid bacteria are employed, the proteases secreted by them will expressed in the cell membrane, simplifying the subsequent purification of peptides (Agyei and Danquah, 2011). However, microbial fermentation has disadvantages of the generation of undesirable substances [like live bacteria, bacteria debris, exopolysaccharides, and organic acids (Mora-Villalobos et al., 2020)] and the implementation of optimal fermentation conditions. In a study, to recover proteins from monkfish by-products (heads and viscera), the effect of temperature, pH, and protease concentration was first investigated using a mixture of monkfish and water [ratio = 1:1 (w/v)], based on which the optimal fermentation conditions were obtained and were 57.4°C, pH 8.31, alcalase with a concentration of 0.05% (v/w), and 3 h for hydrolysis.
5.2 Enzymatic hydrolysis
Enzymatic hydrolysis is a way of using enzymes to cleave peptide bonds to liberate the encrypted peptides and has been widely used to extract bioactive peptides from animal waste proteins on an industrial scale (Figure 5). The often-used enzymes include pepsin, bromelain, trypsin, neutrase, chymotrypsin, alcalase, papain, flavourzyme, and protamex. The source of the often-used enzyme is usually well-known in the bioengineering field. For example, the papain is from papayas, and the trypsin is from the pancreas of pigs, cows, or sheep. Each enzyme has two crucial functions in catalyzing protein hydrolysis: binding affinity and catalytic performance. The protease contains one or several active sites with catalysis. The amino acid residue on the catalytic site can recognize and bind to a special peptide bond in the substrate, based on which protease can easily combine with the substrate and then for the enzyme-substrate complex. Besides, the amino acid residue on the catalytic site can supply the acidic or alkalic environment or functional groups required by catalytic reaction, based on which proteases promote the break of peptide bonds. After protease finishes hydrolyzing substrates, it will release products, return to the active state, and come into the next protein hydrolysis (Jovanović et al., 2016).
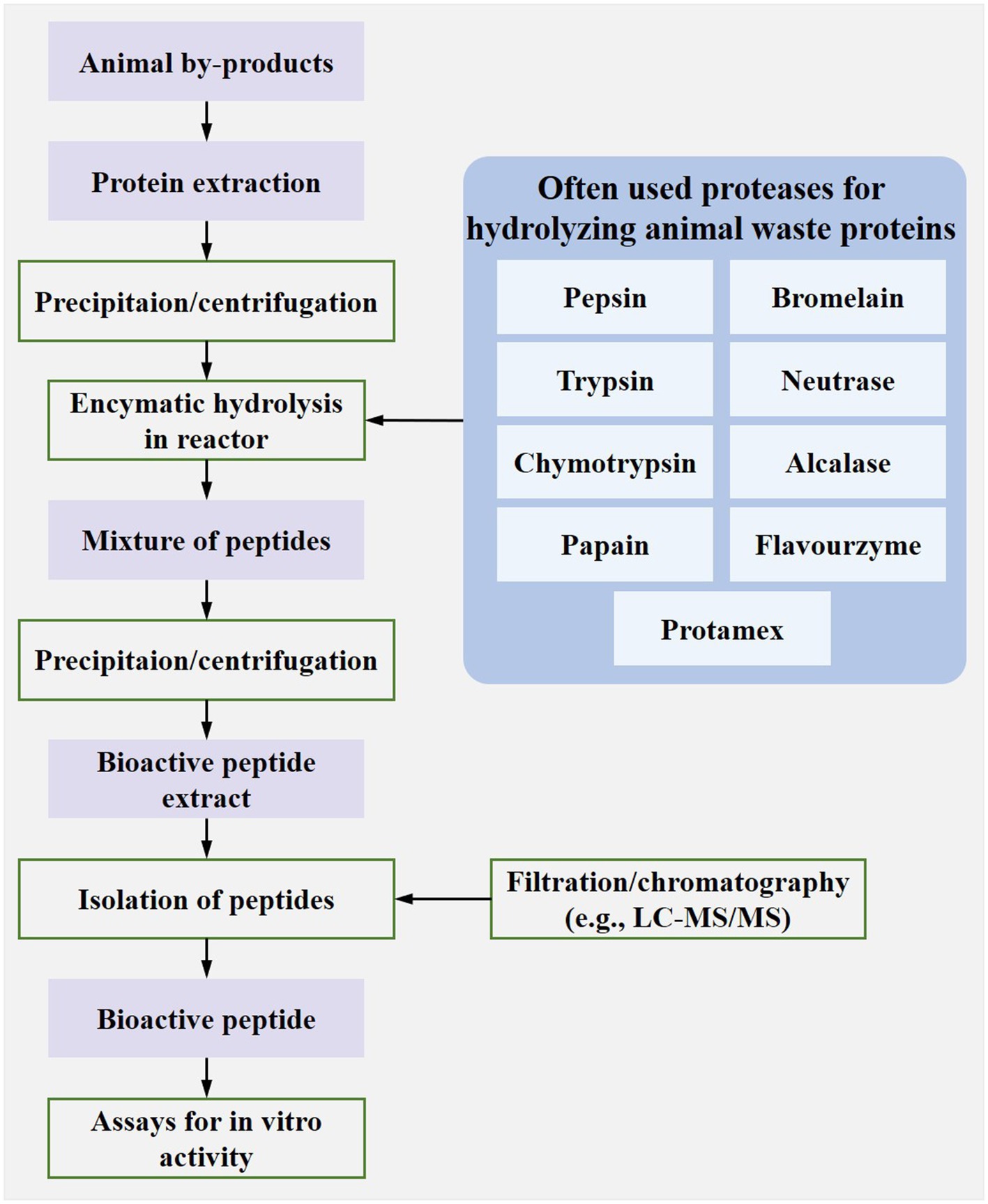
Figure 5. Schematic of the typical industrial production of bioactive peptides from animal waste proteins [modified from Mora et al. (2014)].
The protease used for hydrolyzing animal waste proteins had a wide source, including animal, plant, and microorganisms. The most used proteases are pepsin, trypsin, chymotrypsin, papain, bromelain, neutrase, alcalase (e.g., As1398 and protease K), flavourzyme, and protamex (Dey and Dora, 2014; Gajanan et al., 2016; Teshnizi et al., 2020; Tacias-Pascacio et al., 2021). For instance, the pepsin was usually obtained from porcine gastric mucosa and was used to hydrolyze the by-products of marine fishes, like the skin and bone of Spanish mackerel (Li et al., 2013) and the spines and skulls of skipjack tuna (Yu et al., 2014), to obtain collagen and peptides. Like microbial fermentation, appropriate conditions, including time, pH, temperature, enzyme specificity, and substrate/enzyme ratio, should be guaranteed to maximize the catalytic activity of proteases and the efficiency of enzymatic hydrolysis. A study found that the alcalase had the highest degree of hydrolysis (DH) for shrimp waste proteins (mainly consisting of head and shell of Penaeus monodon) and DH increased with temperature (50–60°C) during alcalase hydrolysis. The response surface graphs revealed that the optimal hydrolysis conditions were 59.37°C, pH 8.25, 1.84%, and 84.42 min (Dey and Dora, 2014). Another study observed that the porcine gastric mucin could not be hydrolyzed by pepsin at neutral pH because of the inactivity of stomach-derived pepsin at pH 7 (Schömig et al., 2016).
The enzymatic hydrolysis for animal waste proteins is characterized by mild reaction conditions and selectivity. The rational temperature range for most proteases is 50–60°C, and the higher one for some proteases does not exceed 70°C. The pH used for hydrolysis using microorganism-derived protease, which is more often used in hydrolyzing animal waste proteins on the industrial scale, is located at 5.5–8.0 (Dey and Dora, 2014; Razzaq et al., 2019). Therefore, this biotechnology has no high requirement of energy, facilities, and control, saving cost and simplifying management. In addition, due to the substrate specificity of protease, the enzymatic hydrolysis has remarkable regioselectivity, for instance, the preferential cleaving hydrophobic amino acid residues, especially the aromatic residues, of pepsin (Tavano, 2013). This substrate specificity offers an excellent suggestion to determine the protease for the given substrate, resulting in a high DH and hydrolysates with desirable compositions and properties. Moreover, no secondary products are generally generated during the enzymatic hydrolysis. The production of desirable amino acid sequences with secondary products renders the enzymatic hydrolysis ecologically sound.
However, enzymatic hydrolysis is plagued with low yield and high cost at an industrial scale. To improve DH, pretreatment is carried out, like thermal and acid treatment, but it has the risk of destroying peptides (Fauzi et al., 2016; Feng et al., 2017). The higher cost compared with microbial fermentation is attributed to the high price of protease (Aspevik et al., 2016). To solve the two problems, some technologies are incorporated into enzymatic hydrolysis, including microwave heating, ultrasound, high voltage electrical treatments (including pulsed electric field and electrical arc), and high hydrostatic pressure (Mikhaylin et al., 2017; Thoresen et al., 2020; López-Pedrouso et al., 2023b).
6 Applications of bioactive peptides and hydrolysates from animal waste proteins
6.1 Agricultural application of bioactive peptides and hydrolysates from animal waste proteins
Peptides derived from animal waste proteins have potential applications in agriculture. They can be used as natural fertilizers, biostimulants, and biopesticides, improving soil health, promoting plant growth and yield, enhancing plant stress tolerance, and providing protection against pests and diseases (Da Silva, 2018).
6.1.1 Plant growth promotion
The ability to promote plant growth of animal waste proteins can be attributed to two aspects: ameliorating soil quality and directly enhancing the physiological processes. For one thing, the free amino acids, soluble proteins, and peptides that occur in hydrolyzed animal waste proteins can directly adjust the C/N ratio (Bhari et al., 2021). These nitrogen-containing nutrients also can increase the total count of heterotrophic bacteria in soil (e.g., nitrogen fixers and phosphate solubilizers), which is an important indicator for soil fertility, and thus change the rhizosphere microorganisms indirectly promote plant growth (Paul et al., 2013; Bhange et al., 2016). For another, amino acids, peptides, and proteins are the essential nutrients of plants and are required by a series of metabolic activities, including synthesizing nucleic acids, proteins, chlorophyll, vitamins, alkaloids, terpenoids, and forming vegetable tissues and organs. Thus, these materials from animal waste proteins can effectively promote plant growth in each stage, from seed germination to early root and shoot growth, and finally to blossom and fruition (Figures 6A,B) (Nurdiawati et al., 2019; Jagadeesan et al., 2023).
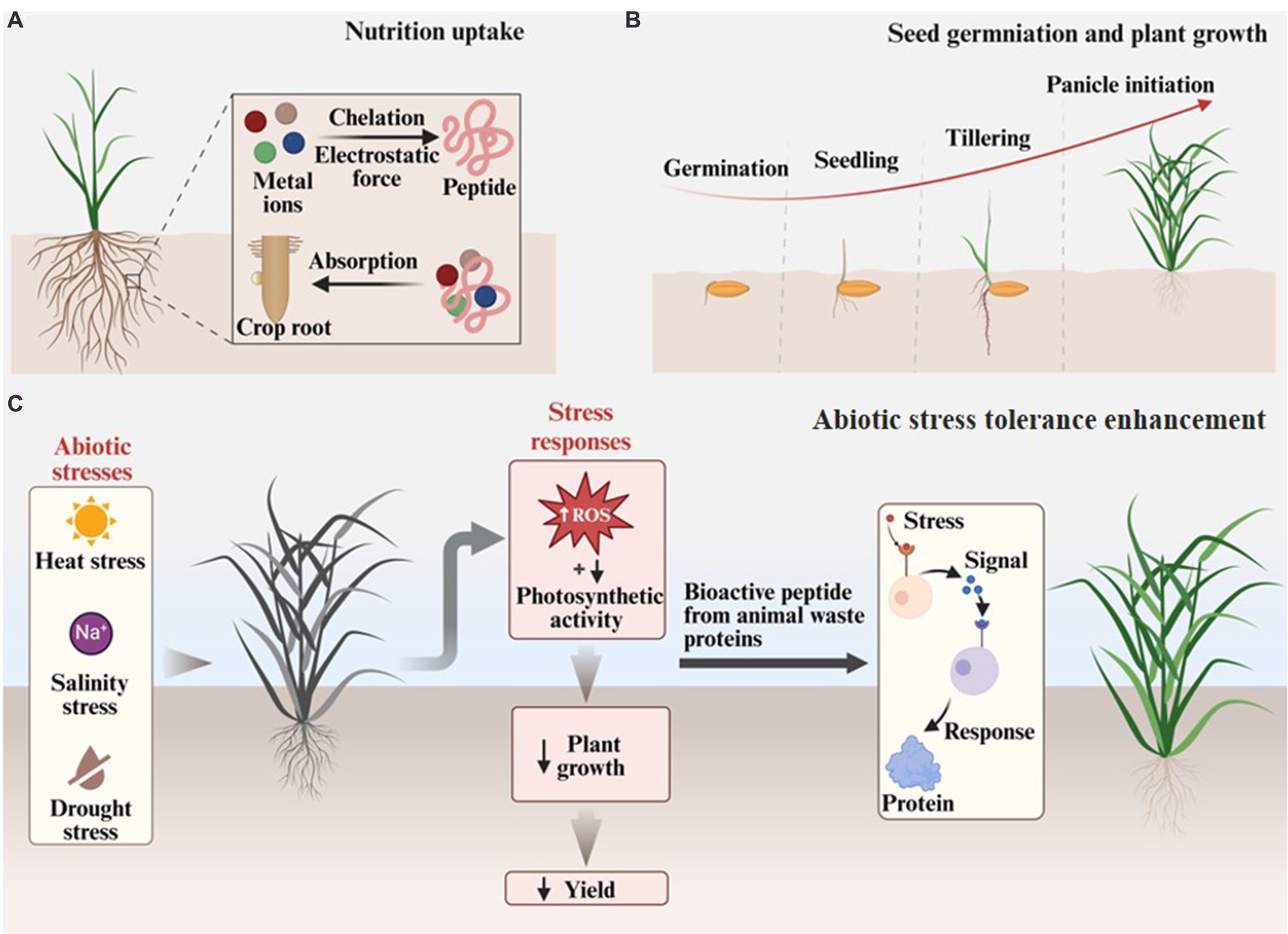
Figure 6. Agricultural application of animal waste proteins. (A) Nutrition uptake. (B) Seed germination and plant growth. (C) Abiotic stress tolerance enhancement.
6.1.2 Abiotic stress tolerance enhancement of plants
Research demonstrates that the peptides and amino acids in animal waste protein hydrolysates can induce plant defense responses to some unfavorable conditions, including heat/cold, salinity, drought, and acidity, and thus enhance their tolerance to these abiotic stresses (Figure 6C) (Colla et al., 2015). A study used commercial animal-originated protein hydrolysates, neutralized with calcium salts, to treat Diospyros kaki L. cv. “Rojo Brillante” grafted on Diospyros lotus L. to explore their effects on the tolerance to soil affinity of Diospyros lotus L. Diospyros lotus L. is highly sensitive to salinity, especially chloride. The tree treated with protein hydrolysates had a lower leaf chloride uptake, stem water potential, and leaf necrosis than the untreated trees, indicating the used animal-originated protein hydrolysates enabled an improved tolerance to salinity for persimmon trees. The improvement of tolerance to salinity was due to two complementary mechanisms of salts: first, Ca2+ enhanced the tree’s ability to exclude chloride; second, the Ca2+, proline, glycine, glutamate, and glycine betaine in the protein hydrolysates stimulated the tree’s mechanism to increase compatible solutes proline and glycine betaine, which was indirectly demonstrated by the lower stem water potential (Visconti et al., 2015). The beneficial effect of proline on enhancing plant tolerances to abiotic stresses has also been observed in another study (Lucini et al., 2015), where a remarkable increase of proline occurred in lettuce under saline conditions. The enhanced tolerance to heat stress of the plant treated by animal-originated protein hydrolysates was observed in research. In a study, the lettuce (Lactuca sativa L. var. capitata) treated by Terra-Sorb Foliar, an animal-derived protein hydrolysate obtained by enzymatic hydrolysis, had a higher total fresh weight (root and aerial part) and stomatal conductance in three controlled cold environments, i.e., diurnal cold (4°C/20°C), nocturnal cold (22°C/2°C), and radicular cold (6°C at root zone and 4°C/20°C in air), than controlled environment (22°C/20°C). Besides, the heat stress tolerance of perennial ryegrass (Lolium perenne L.) under three temperatures (20°C, 28°C, and 36°C) was evaluated. The results showed that the ryegrass treated with Terra-Sorb falior had a higher photosynthetic efficiency and higher levels of photosynthetic pigments (chlorophylls and carotenoids). The comparison between Terra-Sorb falior treatment and the other three treatments (Terra-Sorb falior + nutrient solution, nutrient solution, and nutrient solution matching to Terra-Sorb falior) revealed that it was the biostimulant effect exerted by the amino acids in Terra-Sorb falior, instead of its nitrogen fertility effect, that enhanced the plant’s tolerance to heat stress.
6.1.3 Biotic stress tolerance enhancement of livestock and fish
The huge demand for meat and fish brings about the continual development of an intensive culture of livestock and fish. In intensive livestock and fish farms, the animals have a high risk of infectious diseases caused by pathogens. The use of antibiotics is a quick and powerful method to control these diseases. However, it suffers from several adverse effects, including the development of drug resistance in animals and antibiotic residues in both animals and the environment, which pose a threat to food quality, environmental protection, and human health. The search for alternative strategies is important. In this sense, research found that some protein hydrolysates of animals could improve the disease resistance of farmed livestock and fish, and thus higher yield and healthier food was obtained.
The mechanisms that animal-originated protein hydrolysates improve animals’ disease resistance involve immune stimulation, pathogen destruction, oxidative radical clearance, or stress and satiety adjustment. A study found that juvenile red seabream (Pagrus major) fed with the diet prepared by using about 5% protein hydrolysates (krill hydrolysates, shrimp hydrolysates, or tilapia hydrolysate) to replace 10% fish meal had increased antiprotease and superoxide dismutase activities and enhanced total immunoglobulin level than the fish fed with basal fish meal. Moreover, the juvenile red seabream fed with the prepared diet exhibited a higher resistance against Edwardsiella tarda (Bui et al., 2014). Another study also showed that the abalone (Haliotis midae) fed with a commercial protein hydrolysate at a low inclusion level (ACTIPAL HP 1, 6%) showed increased cellular immunity because the phagocytic activities of their hemocytes were improved by 18% compared to the control diet (Goosen et al., 2014). Some protein hydrolysates from animal by-products have exhibited antimicrobial effects against pathogenic species, like bovine hemoglobin hydrolysates (the main component of bovine cruor by-products), seafood skin hydrolysates, beef sarcoplasmic protein hydrolysates, and fish collagen (Anal et al., 2013; Zamorano-Apodaca et al., 2020; Beaubier et al., 2021). Compared with the antibiotics on the market, the peptides from animal waste proteins have a broader spectrum and faster action, despite they are not as powerful as antibiotics. Specifically, a study showed a peptide faction from collagen hydrolysates obtained by mixed by-products (skins, heads, and skeletons) of various fish species (pompano dolphinfish, seabass, squid, ray, snapper, weakfish, guitarfish, mullet, and different sharks) exhibited antimicrobial and antioxidant activities, which could be as a potential ingredient in both agricultural and pharmaceutical industries (Zamorano-Apodaca et al., 2020). In addition, the opioid-like peptides have been extracted from fish and bovine hemoglobin and can affect the nervous system, adjusting pain, sleep, and behavior, showing interesting applications as anti-stress agents (Lafarga and Hayes, 2014; Mora et al., 2014). They can also regulate the digestion and ingestion of food and can be used as satiety agents for animal obesity control (Iwaniak et al., 2018; Tyagi et al., 2020).
6.1.4 Post-harvest preservation
Postharvest storage is an equally important stage as seedling and growth for agriculture because the harvested products are perishable, especially the fresh vegetables and fruits. For instance, strawberries are susceptible to postharvest decay mainly due to gray mold and rhizopus rot caused by Botrytis cinerea (Pers.) and Rhizopus stolonifer (Ehrenb.) (Romanazzi et al., 2013). Traditionally, chemosynthetic fungicides are applied to retain the freshness of vegetables and fruits during the periods of storage and transportation. However, they are not permitted in the context of sustainable and organic agriculture because of environmental and health issues. In this regard, alternatives are required. Among these, resistant inducers can increase plant disease defenses and also can exert their antimicrobial activities, with the potential for large-scale application. Among the natural materials, protein hydrolysates produced with animal or plant extracts have gained scientific interest. A study found that six hydrolysates of casein, soybean, pea, lupin, malt, and yeast, with a concentration of 1.6 mg/mL, could significantly reduce the disease incidence and severity of wounded citrus fruit caused by Penicillium digitatum, the main postharvest pathogen of citrus fruit. Among the six hydrolysates, casein, lupin, and soybean exhibited the most powerful introduction of resistance. This indicated that these protein hydrolysates could be used as resistant inducers to extend the storage duration (Lachhab et al., 2015). Similarly, another study showed that when used in the field during grape growth, casein hydrolysates enable a gray mold incidence reduction of 94%. When used in vivo trials, the protein hydrolysates of casein could reduce gray mold by 54% at a concentration of 0.8 g/L. When simultaneously used before and after harvest, they enabled a storage rot reduction of 40% (Lachhab et al., 2016). These studies indicate protein hydrolysates enable an extension of the postharvest storage period, with a low risk of pesticide-resistant strains and a better satisfaction of increasingly high requirements of food safety (Albert, 2013). In addition, considering that the microbial infection of fruits may happen at the flowering phase (Romanazzi et al., 2016), a combination of preharvest and postharvest can further extend the storage periods of vegetables and fruits because the latent infection and pathogen inoculum in the field are decreased more compared the only use of postharvest (Lachhab et al., 2016).
6.2 Food application of bioactive peptides and hydrolysates from animal waste proteins
Protein hydrolysates are the best use form of protein concerning nutritional value with variety and balance of amino acids and high solubility. Through enzymatic hydrolysis (Figure 5), animal waste proteins can be decomposed into free amino acids and peptides, which are high-value substances used to develop new healthy foods as additives and functional ingredients and produce food-grade enzymes (Figure 7) (Sila and Bougatef, 2016; Zou et al., 2019).
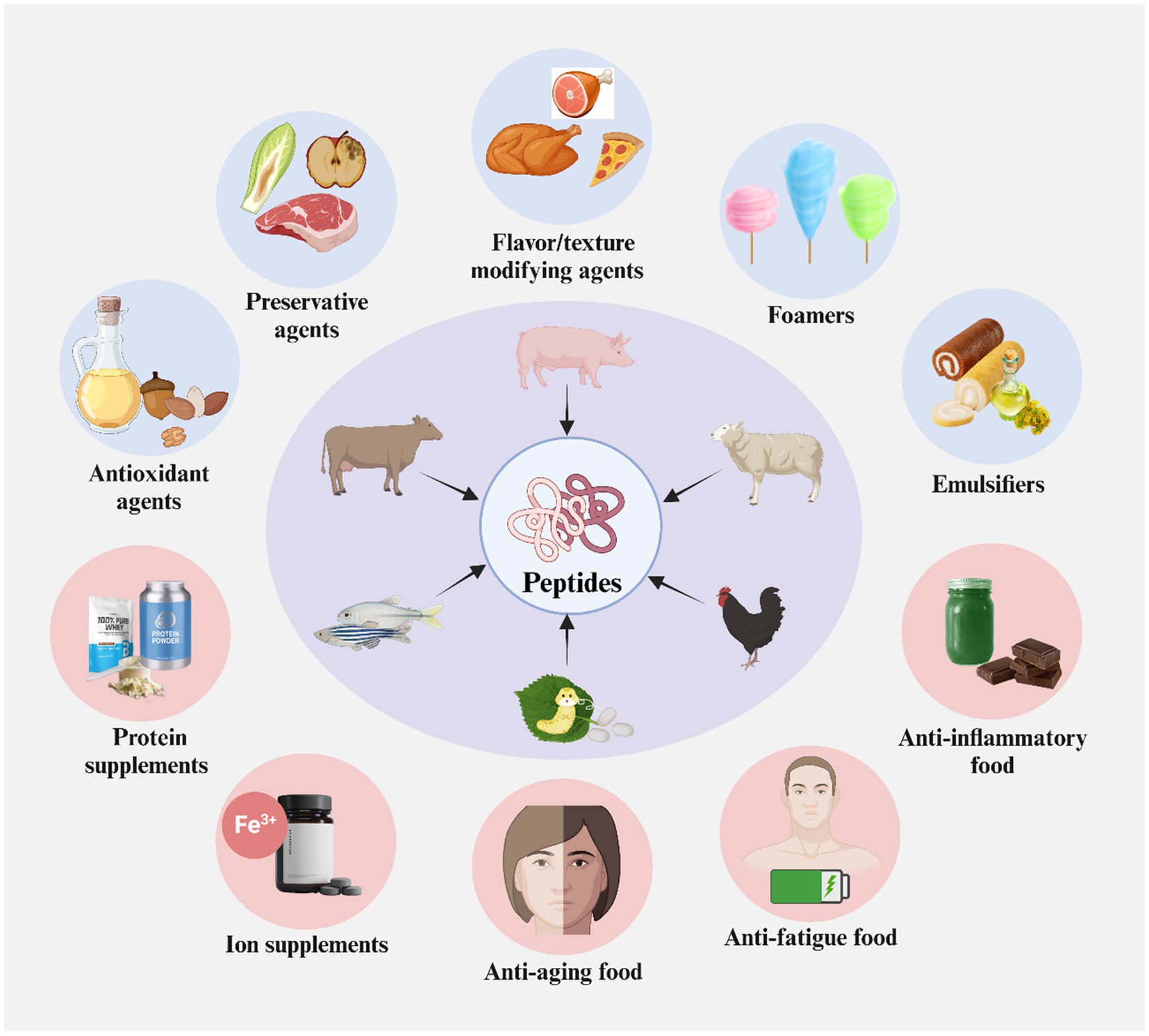
Figure 7. Food application of proteins and peptides derived from animal products processing wastes. Created with BioRender.com.
6.2.1 Food additives
Plentiful studies have reported that the protein and its hydrolysates derived from animal by-products possess various favorable characteristics for food processing, such as antioxidant and antimicrobial activities and good abilities of foaming, emulsion, and fat adsorption, and have the potential use as additives (Lafarga et al., 2015; Lorenzo et al., 2018; Fang et al., 2020; Zhang et al., 2023b). The antioxidant peptides, used as food additives, can delay the irreversible decay of the food matrix from a few hours to several months and even years when proper strategies are implemented. For instance, a study extracted the α137–141 fragment (Thr-Ser-Lys-Tyr-Arg), a small (653 Da) and hydrophilic peptide, from bovine cruor, which contained mainly hemoglobin. The study found that the α137–141 (0.5%, w/w) reduced the lipid oxidation of ground beef by 60%, delaying its rancidity. Moreover, the α137–141 inhibited the growths of microbes, including coliform, mold, yeast, and lactic acid bacteria. These results indicated that the α137–141 possessed antioxidant and antimicrobial activities and could be used as functional ingredients for food preservatives (Przybylski et al., 2016). Interestingly, another study showed that four peptide fractions of collagen hydrolysates of common carp by-products (skeletons, skins, and heads) exhibited antioxidant activity and emulsifying and foaming properties. Among these peptide fractions, the fraction (<3 kDa) exhibited the strongest hydroxyl radical (95.4%, 10 mg/mL) and 2,2-diphenyl-1-picrylhydrazyl (DPPH) (87%, 1 mg/mL) scavenging activities and reducing power (0.34, 10 mg/mL), while the faction (>30 kDa) exhibited the greatest emulsifying activity index, foaming activity, and foaming stability, but the lowest emulsion stability (González-Serrano et al., 2022). The good foaming and emulsifying properties of proteins hydrolysates derived from fish by-products (skeletons, heads, and skins) (Zamorano-Apodaca et al., 2020) and porcine livers (Verma et al., 2019) have also been demonstrated.
6.2.2 Functional foods
Meat and fish contain not only the complete set of essential amino acids but also based on these amino acids, contain plentiful bioactive peptides. Thus, the peptides or hydrolysates of animal waste proteins show potential use as functional ingredients for foods besides as basic nutritive sources. These functions involve antioxidants, iron supplements, fatigue resistance, and anti-inflammation (Zou et al., 2021). In a study, the porcine liver was hydrolyzed with different enzymes, time lengths, and membrane pore sizes. The hydrolysates obtained using alcalase at 8 h exhibited the strongest antioxidant activity: the fraction (>30 kDa) obtained using alcalase exhibited the best DPPH (562 μg/Trolox/g), ferric reducing antioxidant power (FRAP) (82.9 μmol Fe2+/100 g), and oxygen radical absorbance capacity (ORAC) (53.2 mg Trolox/g) activities. The fraction (>30 kDa) obtained using bromelain at 4 h exhibited the strongest antimicrobial activity with a Brochothrix inhibition of 91.7% (Borrajo et al., 2020). More surprisingly, a study first extracted a peptide, AJHbα, with strong antimicrobial activities from the hemoglobin alpha chain in the liver of a Japanese eel (Anguilla japonica), finding that the AJHbα, with a molecular weight of 2,388.05 Da, could kill 8.64% ± 3.91% of E. tarda (Zhang et al., 2013). Similarly, a study observed the peptides and amino acids with antioxidant and anti-fatigue effects in monkfish hydrolysates using both in vitro and in vivo assays. The in vivo assay showed mice administrated with monkfish liver hydrolysates had a longer climbing period than the control group, and in their hepatic and kidney homogenate, a higher level of superoxide dismutase was detected (Xu et al., 2017). Another study extracted a tripeptide (Pro-Ala-Tyr) from salmon pectoral fin hydrolysates and found it could significantly inhibit the NO (63.80%), prostaglandin E2 (45.33%), and three pro-inflammatory cytokines syntheses in RAW264.7 cells because of its inhibitory effect on inducible NO synthesis protein and cyclooxygenase-2 (Ahn et al., 2015).
However, studies on peptides or protein hydrolysates of animal by-products used as ingredients of functional foods are limited, especially in clinical studies. Therefore, further studies are warranted to develop their uses in the food industry and bioavailability. Besides, some animal wastes, like the brain and spinal cord of cattle denoted as the specified risk materials (SRM), cannot be used to produce food ingredients due to the highest possibility of carrying prion proteins (Mekonnen et al., 2016). Moreover, different guidelines and safety assessments have been established by regulatory agencies, such as FDA [U.S. Food and Drug Administration (FDA), 2022] and the European Food Safety Authority (EFSA) [Madende and Hayes, 2020; European Food Safety Authority (EFSA), 2023], for the food use of extracts from animal by-products. Thus, thorough testing and evaluation are required before approval, though it has certain economic, environmental, and social benefits.
6.2.3 Food-grade enzymes
Multiple enzymes have been extracted from animal by-products and become one of the important ingredients in food processing. These enzymes generally are gastrointestinal proteases and include pepsin, trypsin, and chymotrypsin (Udenigwe and Howard, 2013). The pepsin can be extracted from the stomachs of pigs, cattle, and sheep. An excellent review found that pepsin was more often used to hydrolyze eggs to release ACE inhibitory peptides compared to the enzymes extracted from microorganisms (Lee and Hur, 2017). The trypsin and chymotrypsin are found in the small intestine and secreted by the duodenum (Sauer and Merchant, 2018). The three food-grade enzymes have been excessively used in hydrolyzing animal waste proteins, for instance, using pepsin to explore novel bioactive peptides in fish and beef skeletal muscles (Maky and Zendo, 2021) and to release antihypertensive peptides from bovine lactoferrin (Fernández-Musoles et al., 2014) and using trypsin to hydrolyze rice bran to obtain antioxidant and ACE inhibitory peptides (Wang X. et al., 2017). These food-grade enzymes can also be used together to enhance the release of encrypted peptides from parental proteins. For instance, the deer skin hydrolysates prepared by the combination of pepsin and trypsin to exhibited a much more potent DPP-IV inhibitory activity than that prepared by pepsin (Jin et al., 2015). Similarly, pepsin and pancreatin, an agent containing trypsin, were used together to treat trout frame proteins (Ketnawa et al., 2018). The discovery of these food-grade enzymes in animal waste proteins and the commercial versions based on them have played a huge role in the extraction of bioactive peptides from natural sources (Lee and Hur, 2017).
6.3 Medicinal application of bioactive peptides and hydrolysates from animal waste proteins
The peptides extracted from animal waste proteins exhibit various bioactivities. Besides, due to the cost-efficiency and the smaller possibilities of drug resistance and side effects, these peptides show a promising application prospect in the medicinal industry, on which much research has been done (Mahgoub et al., 2021; Wen et al., 2023b). Among these investigations, the antihypertensive, antioxidant, antimicrobial, antidiabetic, and antithrombotic activities of peptides extracted from animal waste protein have occupied much attention, and are summarized in the study.
6.3.1 Antihypertensive drugs
These years have witnessed a growth of hypertension due to the changes in diet and work styles, leading to an increasing demand for cost-effective and safe hypertensive therapy (Zaky et al., 2022). Numerous studies have found that naturally occurring peptides showed antihypertensive activity through different action mechanisms and could be an effective ingredient for hypotension (Khiari et al., 2014; Meinert et al., 2016; Mahdi and Ojagh, 2017; Pujiastuti et al., 2019; Bravo et al., 2023).
These action mechanisms can be classified into two types: renin-angiotensin system and kinin-arginine-nitric oxide system (Figure 8). In the renin-angiotensin system, some peptides can inhibit the release renin, an enzyme catalyzing the conversion of angiotensinogen to angiotensin I (Harnedy and FitzGerald, 2013), while some peptides can inactivate ACE, an enzyme that can catalyze the conversion of angiotensin I to angiotensin II and also the degradation of bradykinin, an enzyme that can relax blood vessels, to inactive peptide fragments in kinin-arginine-nitric oxide system (Siltari et al., 2016; Wang X. et al., 2017). Besides, some peptides act as angiotensin II receptor blockers, inhibiting angiotensin II-mediated vasoconstriction and releases of antidiuretic hormone and aldosterone, all of which can induce blood pressure (Fernández-Musoles et al., 2014). In the kinin-arginine-nitric oxide system, apart from the effect of inactivation ACE, the peptides rich in arginine contribute to synthesizing more nitric oxide, a substance that enables vasodilation, lowering blood pressure (Mas-Capdevila et al., 2019). A study showed found many peptides isolated from the fibrinogen hydrolysates of bovine slaughterhouse blood had ACE and renin inhibition. Among these peptides, a tripeptide SLR had ACE and renin inhibitory IC50 values of 0.17 and 7.29 mM and a peptide RR was resistant to gastrointestinal digestion (Lafarga et al., 2015). Similarly, the peptide fraction (<1 kDa) of the skin gelatin hydrolysate and bone gelatin hydrolysate of pangasius catfish (Pangasius sutchi) had ACE inhibitory IC50 values of 3.2 and 1.3 μg/mL respectively, higher than untreated gelatins and the other two fraction (>10 kDa and 3–10 kDa), but all three fractions showed resistance to gastrointestinal digestion. Besides, the fraction (<1 kDa) was rich in hydrophobic amino acids, like glycine and proline (Mahmoodani et al., 2014). A further study of Lafarga et al. (2015) identified three peptides (His-Phe, Tyr-Arg, and His-Arg) with both ACE and renin-inhibitory activities and one peptide (His-Leu-Pro) with ACE inhibitory activity in the bovine hemoglobin hydrolyzed by papain. His-Arg had ACE and renin-inhibitory IC50 values of 0.19 and 7.09 mM, respectively (Lafarga et al., 2016). These studies demonstrated that the peptide fractions could have a role in improving human health as a functional ingredient of drugs or nutraceuticals, but also reflect that there is a relationship between the antihypertensive activity and amino acid sequence and molecular weight and the bioavailability of peptide after administration should be considered, which necessitate the more studies.
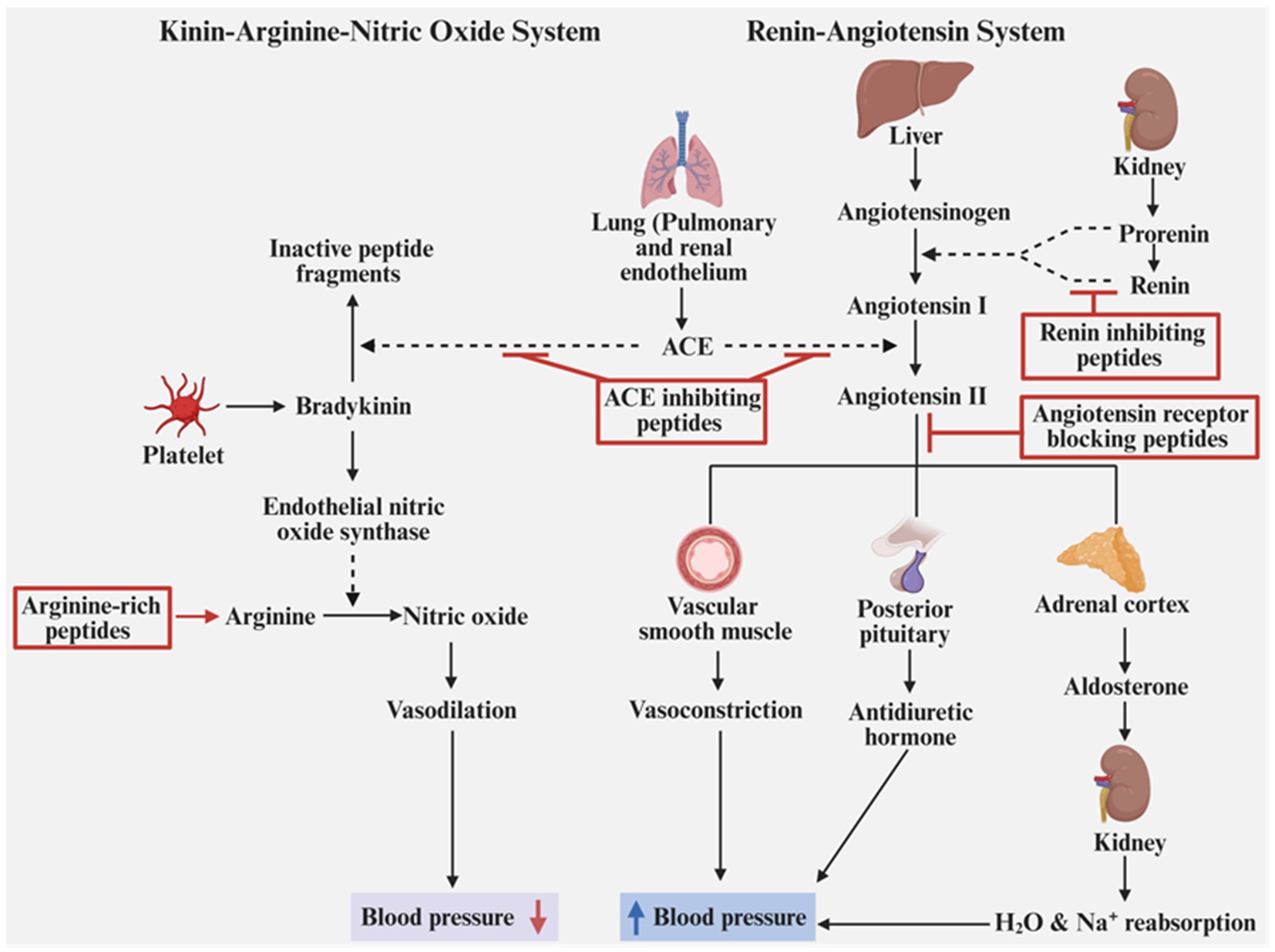
Figure 8. Mechanisms of the action of antihypotensive peptides. Created with BioRender.com.
6.3.2 Antioxidant drugs
When many more free radicals [like reactive oxygen/nitrogen species (ROS and NOS)] are produced in human bodies, causing the amount to exceed the scavenging capability of antioxidant enzymes and other antioxidants (like glutathione and vitamins), the free radicals that are not scavenged will oxidate nucleic acids, proteins, and lipids, leading to serious damage of cells and tissues. This phenomenon is called oxidative stress. If oxidative stress is not controlled, various illnesses might happen, such as tumors, aging, Parkinson’s disease, and Alzheimer’s disease (Forman and Zhang, 2021; Zhang et al., 2023a). In this sense, the study of antioxidants with high efficiency, safety, and low cost is significant for human well-being.
Many antioxidant peptides have been identified in protein hydrolysates of animal by-products, most of which consist of 4–16 amino acids and have a small molecular weight [e.g., a range of 0.4–2 kDa claimed by two studies (Khiari et al., 2014; Zaky et al., 2022)] (López-Pedrouso et al., 2020; Akbarian et al., 2022; González-Serrano et al., 2022; López-Pedrouso et al., 2023a). Although the mechanisms of antioxidant peptides to mitigate oxidative stress have not been clear yet, studies have found that the antioxidant effects of peptides are based on donating proton or electron to free radicals, chelating metals to prevent the production of free radicals, and trapping lipid peroxyl radicals and are related to the size, hydrophobicity, and amino acid composition (Zaky et al., 2022). A study extracted three novel peptides with strong antioxidant activities from protein hydrolysate of bluefin leatherjacket skin. They are Gly-Ser-Gly-Gly-Leu, Phe-Ile-Gly-Pro, and Gly-Pro-Gly-Gly-Phe-Ile, with molecular weights of 389.41, 432.52, and 546.63 Da, respectively. Their antioxidant activities were evaluated by scavenging capabilities of free radicals, including DPPH•, HO•, and •. These strong antioxidant activities were supposed to be due to the small size and the presence of hydrophobic and aromatic amino acid residues (Chi et al., 2015b). Smaller peptides are thought to have easier access to free radicals compared to larger ones and a higher possibility of crossing the intestinal barrier and exert antioxidant functions. Hydrophobic amino acids containing non-polar aliphatic groups, including leucine, isoleucine, proline, alanine, tryptophan, tyrosine, and valine, have a high reactivity to polyunsaturated fatty acids. Among aromatic amino acids, His, can donate protons to inactive free radicals, while tyrosine, tryptophan, and phenylalanine can donate electrons to free radicals to convert them into stable substances. Apart from free radical scavenging capabilities, some studies also demonstrated that the antioxidant activity of extracted peptides from animal waste proteins based on high FRAP values, for instance, a porcine liver hydrolysate (0.09%, w/w) with a FRAP value of 21.50 ± 0.78 (Verma et al., 2019) and the peptide LGEHNIDVLEGNEQFINAAK extracted from porcine liver hydrolysates with a positive correlation with FRAP (0.592) (López-Pedrouso et al., 2020). The ferric ion is a pro-oxidant metal, playing an important role in lipid peroxidation. Therefore, the conversion of ferric form to ferrous form enables a mitigation of lipid peroxidation. Some amino acids with reducibility, like Tyr and Trp, can chelate with ferric ions at their functional groups with lone pairs of electrons, like-NH2, contributing to the reduction of ferric ions.
6.3.3 Antimicrobial drugs
The antimicrobial effect exhibited by peptides of hydrolysates derived from animal waste proteins intrigues scientists, doctors, and health workers because it provides a safe alternative to antibiotics, which are deeply plagued with its induction of microorganism resistance in human bodies, farming animals, and even nature (Wang et al., 2016; Maky and Zendo, 2021).
Over 75% of antimicrobial peptides (AMPs) originated from animals, according to a statistic (as of September 2017) (Kumar et al., 2018). Based on the structure, antimicrobials can be classified into three categories: α-helical peptides, β-sheet peptides, and extended/flexible peptides. The AMPs originated from common animal (like bovines and pigs) waste proteins have all three structures, for instance, bovine myeloid AMP (BMAP)-27 and porcine myeloid AMP (PMAP)-36 both have α-helical structures (Lv et al., 2014; Yang et al., 2019). In terms of subcategory, most of these AMPs belong to cathelicidins, one of the most diverse vertebrate AMPs with 12–80 amino acids (Valdez-Miramontes et al., 2021). For instance, PMAP-36 has a sequence of GRFRRLRKKTRKRLKKIGKVLKWIPPIVGSIPLGCG-NH2. Despite that AMPs from different sources have different sequences and structures, they share several common points, including a net positive charge with a range of +2 to +13 (even to +14), hydrophobicity, and amphipathicity (Lv et al., 2014; Kumar et al., 2018). Many AMPs contain positively charged amino acids, including leucine, arginine, or histidine, for instance, the AMP GLSRLFTALK derived from Anchovy (Engraulis japonicus) cooking wastewater (Tang et al., 2015). In AMPs, hydrophobic amino acids typically occupy 50% of the total. Hydrophobicity is a very important property for AMPs because it determines the extent to which AMPs partition into the membrane lipid bilayer when AMPs interact with microorganisms. Amphipathicity can be considered as a result of the balance between the cationic and hydrophobic residues at both the primary sequence level and the two-dimensional or three-dimensional structure of AMPs. The antimicrobial activity of peptides is closely related to their hydrophobicity, net charge, and hydrophobicity (Hollmann et al., 2016; Wang C.-K. et al., 2017).
These AMPs originated from animal waste proteins and are characterized by a wider spectrum of activity than those derived from microorganisms (Bhat et al., 2015). They can be used as the primary functional ingredient of antiviral, antibacterial, antifungal, and antiparasitic agents and exert their antimicrobial effect by acting on microorganisms or hosts (Figure 9) (Schmidt and Wong, 2013; Mahdi and Ojagh, 2017; Bechaux et al., 2019; Maestri et al., 2019; Yang et al., 2019). They can kill the microorganism by inhibiting the synthesis of nucleic acid (DNA and RNA) and proteins, proteins from functioning, cell wall formation, intercalating DNA, disrupting cell membranes, and activating autolysis. When acting on the host of pathogens, they can protect the host by binding or neutralizing microbial products, promoting the translation, stability, and processing of inflammatory cytokines, inhibiting Nuclear factor κB (NF-κB) movement, blocking the signal pathway of protein kinase, and activating immunocytes. For example, the leakage of unbroken cytoplasm and DNA fiber and the loss of cell integrity were observed in the treated E. coli by an AMP faction derived from camel whey hydrolysate, indicating the peptide exerted an antimicrobial effect through the inhibition of cell wall formation and disruption cell membrane (Abdel-Hamid et al., 2016).
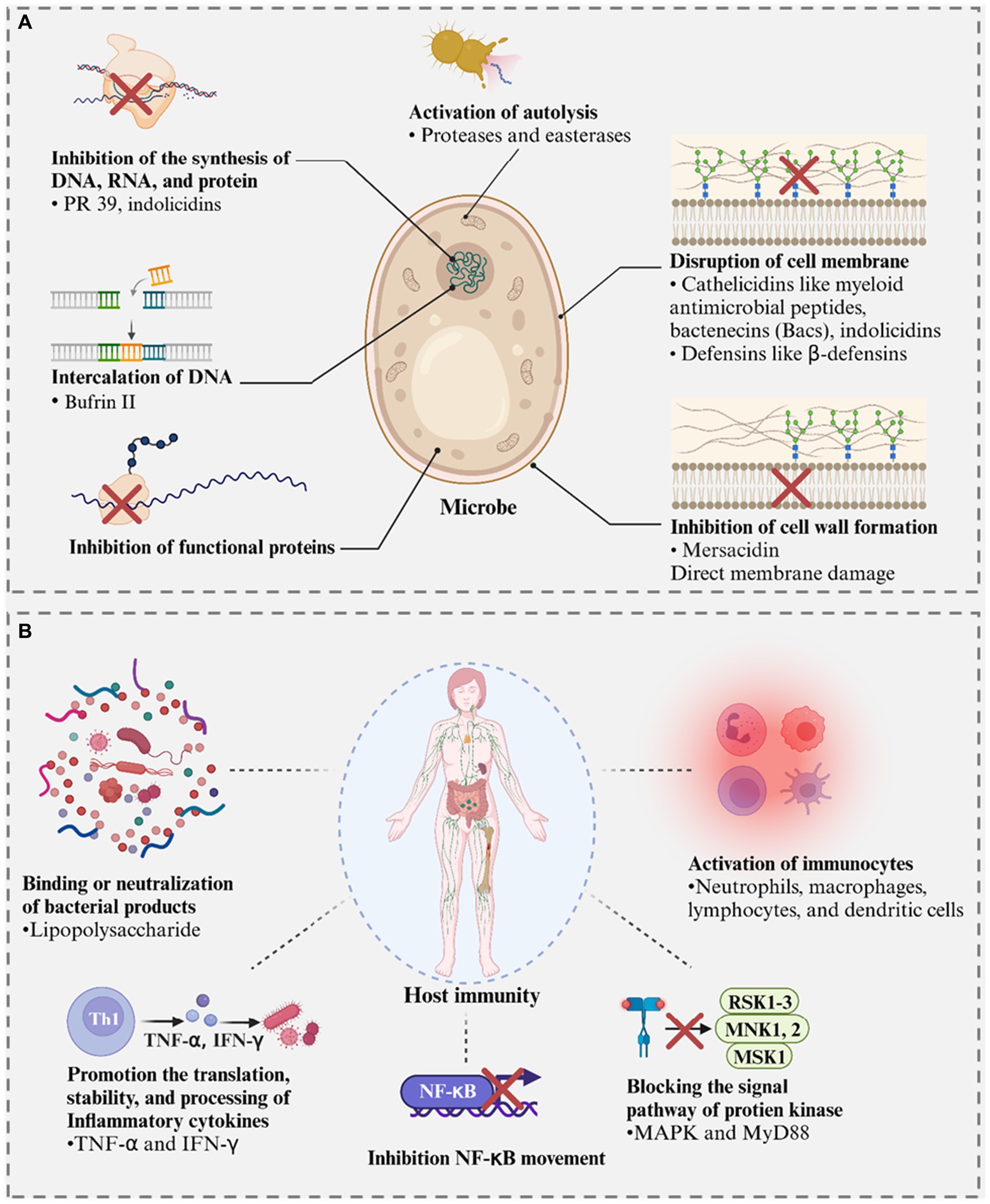
Figure 9. Mechanisms of the action of antimicrobial peptides (AMPs). (A) Inhibiting the growth of microbes and killing microbes. (B) Activating immune response and reducing the impact of microbial products. Created with BioRender.com.
6.3.4 Hypoglycemic drugs
Diabetes is a serious chronic disease and mainly includes Type 1 diabetes and Type 2 diabetes. Compared to Type 1 diabetes, Type 2 diabetes is much more common, occupying 90–95% of the total diabetes cases (Patil et al., 2015). It occurs when the body cannot generate or use insulin and thus blood glucose rises to a higher level. The generation of insulin is primarily modulated by two important peptide incretin hormones, glucagon-like peptide 1 (GLP-1) and glucose-dependent insulinotropic polypeptide (GIP). However, these two incretin hormones can be rapidly cleaved by a metabolic enzyme, dipeptidyl peptidase-IV (DPP-IV) (Figure 10A) (Kęska et al., 2019). Therefore, the peptide with DPP-IV inhibitory activity has the potential for Type 2 diabetes treatment.
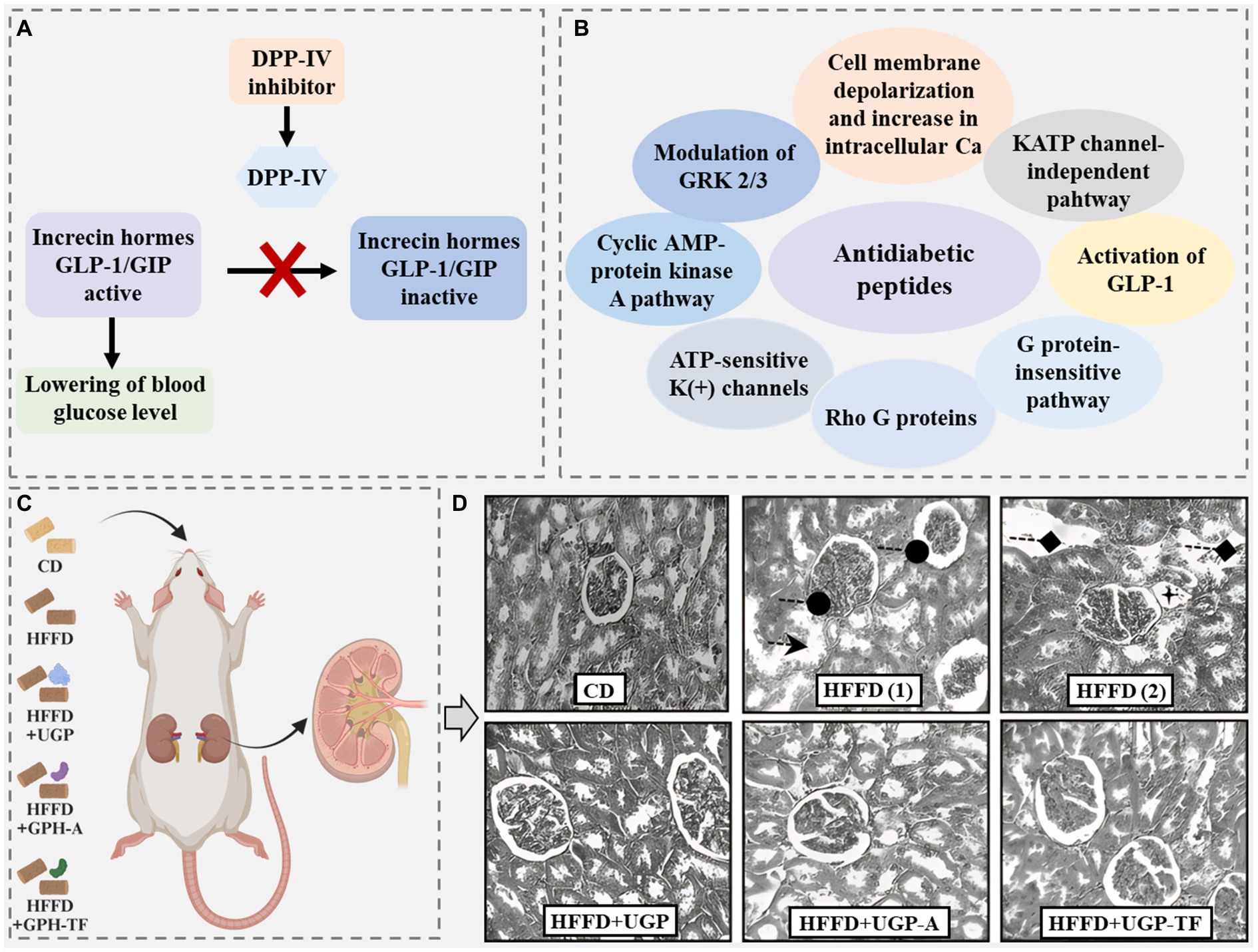
Figure 10. (A) Proposed mechanisms of action for antidiabetic peptides (Soltaninejad et al., 2021). (B) Scheme of the activity of the dipeptidyl peptidase IV (DPP-IV) inhibitor. GLP-1, glucagon-like peptide-1; GIP, glucose-dependent insulinotropic peptide (Kęska et al., 2019). (C) Wistar rat-feeding assay by different diets: CD, control diet; HFFD, high-fat-high-fructose diet; HFFD + UGP, HFFD + undigested goby fish muscle proteins; HFFD + GPH-A, HFFD + goby fish muscle protein hydrolysate obtained with the Bacillus mojavensis A21 protease fraction; HFFD + GPH-TF, HFFD + goby fish muscle protein hydrolysate obtained with the triggerfish protease fraction. (D) Histopathology of kidney tissues from CD group, high-fat-high-fructose diet (HFFD) group [(1) and (2)], HFFD + undigested goby fish muscle proteins (HFFD + UGP) group, HFFD + (HFFD+GPH-A) group, and HFFD + (HFFD + GPH-TF) group. Photomicrographs were taken by optic microscopy: ×200. : tubular dilatation;
: glomerular space;
: vacualization;
: epithelial cells necrosis of the proximal tubules. Modified from Nasri et al. (2015).
Many antidiabetic peptides have been identified in animal waste proteins, such as the peptides Gly-Pro-Phe-Pro-Leu-Pro-Asp and Gly-Ala-Thr-Phe-Gly-Phe-Phe-Tyr-Leu identified in porcine skin gelatin hydrolysate (Huang et al., 2014). Most of these peptides consist of no more than eight amino acids and have molecular weights of 200–2,000 Da. They generally have a sequence of X-Pro or X-Ala at the N-terminal, where X is a hydrophobic amino acid and probably has a small size. Considering that DPP-IV has specificity for cleaving X-Pro or X-Ala fragments from the N-terminal of peptides and proteins, antidiabetic peptides with a sequence of X-Pro or X-Ala at the N-terminal may act as substrate-type inhibitors (FitzGerald et al., 2014; Nongonierma et al., 2014; Jin et al., 2015). Differently, a study acquired 45 peptides with antidiabetic activity from the amphibian innated immune system through bioinformatic analysis, summarizing their proposed action mechanisms and recognizing their consensus amino acids, including alanine, glycine, lysine, and leucine (Figure 10B) (Soltaninejad et al., 2021). These proposed action mechanisms and main amino acids are not the same as those of the antidiabetic peptides mentioned above.
The antidiabetic effect of peptides has been thought to pertain to their sequence length, charge, and hydrophobicity (Kuo-Chiang et al., 2013), but there is no consensus in terms of the antidiabetic peptides derived from animal waste proteins. For instance, the tripeptide Gly-Pro-Hyp and tetrapeptide Gly-Pro-Ala-Gly, derived from a porcine skin hydrolysate fraction, exhibited DPP-IV inhibitory activity (IC50 = 49.6 and 41.9 μM, respectively) (Kuo-Chiang et al., 2013) similar to the pentapeptide Ile-Pro-Ala-Val-Phe derived from porcine skin hydrolysate (IC50 = 44.7 μM) (Silveira et al., 2013). Besides, the peptides Gly-Pro-Val-Gly-Hyp-Ala-Gly-Pro-Pro-Gly-Lys and Gly-Pro-Val-Gly-Pro-Ser-Gly-Pro-Hyp-Gly-Lys, derived from deer skin hydrolysate, exhibited similar DPP-IV inhibitory activity (IC50 = 83.3 and 93.7 μM, respectively) (Jin et al., 2015). Likely, the peptides Arg-Ala-Ser-Asp-Pro-Leu-Leu-Ser-val, Arg-Asn-Asp-Asp-Leu-Asn-Tyr-Ile-Gln, and Leu-Ala-Pro-Ser-Leu-Pro-Gly-Lys-Pro-Lys-Pro-Asp, derived from an egg-yolk protein by-product exhibited a similar DPP-IV inhibitory activity (IC50 ranging from 361.50 to 426.25 μM) (Zambrowicz et al., 2015).
These comparisons seem to mean that the antidiabetic peptides with similar sequence lengths have similar activity. However, the peptide Gly-Pro-Val-Gly-Pro-Ser-Gly-Pro-Hyp-Gly-Lys, also derived from deer skin hydrolysate, exhibited an antidiabetic activity (IC50 = 318.1 μM) much lower than the other two peptides consisting of 11 amino acids mentioned above (Jin et al., 2015). Additionally, based on the statistical analysis of 45 antidiabetic peptides from the amphibian innate immune system, a study supposed that the antidiabetic peptides with a higher net positive charge and weaker hydrophobicity exhibited a stronger insulinotropic effect (Soltaninejad et al., 2021). However, this relationship has not been observed in animal-derived peptides (Rivero-Pino et al., 2020; He et al., 2023; Li et al., 2023). Specifically, the study (Nasri et al., 2015) showed that two protein hydrolysates derived from goby fish using Bacillus mojavensis A21 protease fraction (HFFD + GPH-A) and triggerfish protease fraction (HFFD + GPH-TF), respectively, could reduce blood glucose level and hepatic glycogen and protect the kidney of high-fat-high-fructose diet (HFFD)-fed rats by reversing the HFFD-induced uric acid reduction and creatinine level increase in serum and preventing some HFFD-induced changes in the kidney (including tubular dilatation, glomerular space, vacuolization, and epithelial cells necrosis of the proximal tubule) (Figure 10C). However, the amino acid composition analysis showed higher percentages of hydrophobic amino acids (41.33 and 38.42%) in both HFFD + GPH-A and HFFD + GPH-TF.
6.3.5 Antithrombotic drugs
Apart from hypertension, thrombosis (i.e., the blood clotting inside the vessels) is another major cause of cardiovascular disease and can lead to several serious results, including paralysis, myocardial infarction, and vascular diseases. Due to the safety and comparable antithrombotic effect to synthetic drugs [like aspirin and heparin (Indumathi and Mehta, 2016; Cheng et al., 2018)], antithrombotic peptides derived from animal waste proteins are considered to be a good alternative to them and have continuously gained attention in the past 10 years, though not as much as the aforementioned four kinds of bioactive peptides (Madhu et al., 2022; Wen et al., 2023a). For instance, a study found that the mackerel skin gelatine hydrolysate exhibited high antithrombotic activity, probably owing to the presence of the peptide tripeptide Phe-Gly-Asn with a molecular weight of 337 Da (Khiari et al., 2014).
Anticoagulants are one of the main therapeutic drugs for antithrombic diseases. Fortunately, research has identified anticoagulant peptides in animal meat and by-products (Kong et al., 2014; Cheng et al., 2018; Qiao et al., 2018; Bezerra et al., 2019; Ucak et al., 2021). The anticoagulant effect of these peptides is often evaluated by the extension of activated partial thromboplastin time, thrombin time, and prothrombin time. The study (Bezerra et al., 2019) proved that the peptides extracted from the hydrolysate of a mixture of chicken combs and wattles were anticoagulant and very ACE-inhibitory. The anticoagulant effect was achieved by activating partial thromboplastin time.
7 Challenges
Animal waste proteins will continue to grow worldwide, along with consumer demands. Their adding-value applications through advanced biotechnological methods in agricultural, food processing, and medical fields not only mitigate the environmental pressure brought by their discard but also bring new opportunities for the progress of agriculture, food processing, and medicine. However, these adding-value applications also face some challenges, including bioavailability or stability during gastrointestinal digestion and the relationship between the bioactivity and properties of peptides.
7.1 Stability and bioavailability of bioactive peptides from animal waste proteins
The stability and bioavailability of bioactive peptides are extremely important to exert their activities in functional food and pharmaceuticals. After oral administration, peptides may be decomposed into smaller molecules during gastrointestinal digestion, resulting in the reduction and even loss of their activities (Ketnawa et al., 2018; Wang K. et al., 2021; Cai et al., 2022; Zhang et al., 2023b). For example, a study identified many peptides with ACE, renin, and DPP-IV inhibitory activities in bovine fibrinogen fraction. However, the computer simulation of gastrointestinal digestion predicted some peptides were cleaved by pepsin, trypsin, and chymotrypsin into amino acids (Lafarga et al., 2015). Therefore, the resistance of peptides to gastrointestinal digestion should be considered when assessing their effects on human bodies and animals. Recently, the computer simulation of proteolysis has been used for predicting the decomposition of proteins in the gastrointestinal system, like the ExPASy PeptideCutter used in Lafarga et al. (2015) and the BIOPEP-UWM database used in Kęska et al. (2019). However, in the gastrointestinal system of humans and animals, the digestion and adsorption of proteins is a more complicated process than the simulated cleavage of proteins in silico analysis, due to the effect of multiple factors, including intestinal motility and body temperature fluctuation (Li et al., 2020; Sensoy, 2021). Therefore, in vivo study and the subsequent clinic trial are essential to explore the absolute bioavailability and effect of bioactive peptides after being oral administration. The combination of in silico analysis and in vivo study may open new avenues in the rapid development of functional food and pharmaceuticals with bioactive peptides as primary ingredients (Ketnawa et al., 2018).
7.2 Action mechanisms of bioactive peptides from animal waste proteins
The comprehension of the action mechanisms of bioactive peptides is fundamental for them to be used as functional ingredients in industrial products. The action mechanisms of bioactive peptides are associated with their amino acid composition, structure, hydrophobicity, and charge. The comprehension of these associations will be beneficial to the identification of novel bioactive peptides and the synthesis of bioactive peptides. However, the association of some bioactivities of peptides with their feathers has not been clear yet. As mentioned above, the peptides with X-Pro or X-Ala (X represents a hydrophobic amino acid) at the N-terminal derived from livestock or aquatic product protein hydrolysates are competitive with GLP-1 GIP as the substrate of DPP-IV, based on which these peptides enable the reduction of blood glucose level. However, a database analysis of amphibian-originated antidiabetic peptides concluded that these peptides exert the antidiabetic through eight different mechanisms and have four consensus amino acids (alanine, glycine, lysine, and leucine), an average sequence length of 22.24, and an average net charge of 3.50 (Soltaninejad et al., 2021), quite different from features of those derived from livestock and aquatic sources, including DPP-IV inhibition, no more than eight amino acids, and richness in proline.
8 Conclusion
This paper reviewed the advancement of the value-added application of animal waste proteins in the past decade. Microbial fermentation and enzymatic hydrolysis are the most favorable biotechnological methods to treat animal waste proteins to decode bioactive peptides from parental proteins, especially enzymatic hydrolysis, which is more efficient at producing the peptide with a specific activity and more used in finding novel bioactive peptides in animal waste proteins, than microbial fermentation. The bioactive peptides produced enable the promotion of whole-life growth of plants, enhancement of both abiotic and biotic stress tolerance, and prolongation of post-harvest preservation of agricultural products. In the food industry, these peptides have been used as additives and primary functional ingredients. Besides, animal waste proteins are also good sources of food-grade enzymes. In addition, these bioactive peptides show a prospective application in medicine as a functional ingredient, including antihypertension, antioxidant, hypotensive, and antithrombosis. These value-added applications of animal waste proteins may be a step towards sustainable animal by-products management and circular bioeconomy and, simultaneously, open new avenues in the rapid development of nutraceuticals and pharmaceuticals.
Author contributions
SB: Writing – review & editing, Writing – original draft. QW: Writing – review & editing, Writing – original draft. LZ: Writing – review & editing, Project administration, Conceptualization. YC: Writing – review & editing. JY: Writing – review & editing. PC: Writing – review & editing. YS: Writing – review & editing, Supervision. TZ: Writing – review & editing, Supervision, Project administration, Funding acquisition, Conceptualization.
Funding
The author(s) declare that financial support was received for the research, authorship, and/or publication of this article. Our study received funding from the National Natural Science Foundation of China (Grant Number: 22278395) and the China Postdoctoral Science Foundation (Grant Number: 2023M743507).
Acknowledgments
The authors would like to thank other team members from the Guangzhou Institute of Energy Conversion, Chinese Academy of Sciences, including Ying Guo, Yingxin, Deng, and Shaopeng Chen.
Conflict of interest
YC was employed by Jiangsu Weiguang Biotechnology Co., Ltd.
The remaining authors declare that the research was conducted in the absence of any commercial or financial relationships that could be construed as a potential conflict of interest.
The author(s) declared that they were an editorial board member of Frontiers, at the time of submission. This had no impact on the peer review process and the final decision.
Publisher’s note
All claims expressed in this article are solely those of the authors and do not necessarily represent those of their affiliated organizations, or those of the publisher, the editors and the reviewers. Any product that may be evaluated in this article, or claim that may be made by its manufacturer, is not guaranteed or endorsed by the publisher.
References
Abdel-Hamid, M., Goda, H. A., De Gobba, C., Jenssen, H., and Osman, A. (2016). Antibacterial activity of papain hydrolysed camel whey and its fractions. Int. Dairy J. 61, 91–98. doi: 10.1016/j.idairyj.2016.04.004
Abdelhedi, O., Jridi, M., Jemil, I., Mora, L., Toldrá, F., Aristoy, M.-C., et al. (2016). Combined biocatalytic conversion of smooth hound viscera: protein hydrolysates elaboration and assessment of their antioxidant, anti-ACE and antibacterial activities. Food Res. Int. 86, 9–23. doi: 10.1016/j.foodres.2016.05.013
Adewale, P., Dumont, M.-J., and Ngadi, M. (2015). Recent trends of biodiesel production from animal fat wastes and associated production techniques. Renew. Sust. Energ. Rev. 45, 574–588. doi: 10.1016/j.rser.2015.02.039
Agriculture and Rural Economy Directorate of Scotland . (2023). Animal by-products: disposal guidance. Available at: https://www.gov.scot/publications/animal-by-products-disposal-guidance/pages/fallen-stock-and-other-animal-carcases/
Agyei, D., and Danquah, M. K. (2011). Industrial-scale manufacturing of pharmaceutical-grade bioactive peptides. Biotechnol. Adv. 29, 272–277. doi: 10.1016/j.biotechadv.2011.01.001
Ahn, C.-B., Cho, Y.-S., and Je, J.-Y. (2015). Purification and anti-inflammatory action of tripeptide from salmon pectoral fin byproduct protein hydrolysate. Food Chem. 168, 151–156. doi: 10.1016/j.foodchem.2014.05.112
Ahn, C.-B., Kim, J.-G., and Je, J.-Y. (2014). Purification and antioxidant properties of octapeptide from salmon byproduct protein hydrolysate by gastrointestinal digestion. Food Chem. 147, 78–83. doi: 10.1016/j.foodchem.2013.09.136
Akbarian, M., Khani, A., Eghbalpour, S., and Uversky, V. N. (2022). Bioactive peptides: synthesis, sources, applications, and proposed mechanisms of action. Int. J. Mol. Sci. 23:1445. doi: 10.3390/ijms23031445
Albert, M. (2013). Peptides as triggers of plant defence. J. Exp. Bot. 64, 5269–5279. doi: 10.1093/jxb/ert275
Anal, A. K., Noomhorm, A., and Vongsawasdi, P. (2013). “Protein hydrolysates and bioactive peptides from seafood and crustacean waste: their extraction, bioactive properties and industrial perspectives” in Marine proteins and peptides: biological activities and applications. New York, America: John Wiley & Sons, Ltd. 709–735.
Aspevik, T., Egede-Nissen, H., and Oterhals, Ĺ. (2016). A systematic approach to the comparison of cost efficiency of endopeptidases for the hydrolysis of Atlantic salmon (Salmo salar) by-products. Food Technol. Biotechnol. 54, 421–431. doi: 10.17113/ftb.54.04.16.4553
Beaubier, S., Przybylski, R., Bodin, A., Nedjar, N., Dhulster, P., and Kapel, R. (2021). Ultrafiltration fractionation of bovine hemoglobin hydrolysates: prediction of separation performances for optimal enrichment in antimicrobial peptide. Membranes 11:73. doi: 10.3390/membranes11020073
Bechaux, J., Gatellier, P., Le Page, J.-F., Drillet, Y., and Sante-Lhoutellier, V. (2019). A comprehensive review of bioactive peptides obtained from animal byproducts and their applications. Food Funct. 10, 6244–6266. doi: 10.1039/C9FO01546A
Ben Hassen-Trabelsi, A., Kraiem, T., Naoui, S., and Belayouni, H. (2014). Pyrolysis of waste animal fats in a fixed-bed reactor: production and characterization of bio-oil and bio-char. Waste Manag. 34, 210–218. doi: 10.1016/j.wasman.2013.09.019
Bezerra, T. K. A., de Lacerda, J., Salu, B. R., Oliva, M. L. V., Juliano, M. A., Pacheco, M. T. B., et al. (2019). Identification of angiotensin I-converting enzyme-inhibitory and anticoagulant peptides from enzymatic hydrolysates of chicken combs and wattles. J. Med. Food 22, 1294–1300. doi: 10.1089/jmf.2019.0066
Bhange, K., Chaturvedi, V., and Bhatt, R. (2016). Ameliorating effects of chicken feathers in plant growth promotion activity by a keratinolytic strain of Bacillus subtilis PF1. Bioresour. Bioprocess. 3:13. doi: 10.1186/s40643-016-0091-y
Bhari, R., Kaur, M., and Sarup Singh, R. (2021). Chicken feather waste hydrolysate as a superior biofertilizer in agroindustry. Curr. Microbiol. 78, 2212–2230. doi: 10.1007/s00284-021-02491-z
Bhat, Z. F., Kumar, S., and Bhat, H. F. (2015). Bioactive peptides of animal origin: a review. J. Food Sci. Technol. 52, 5377–5392. doi: 10.1007/S13197-015-1731-5
Borrajo, P., Pateiro, M., Gagaoua, M., Franco, D., Zhang, W., and Lorenzo, J. M. (2020). Evaluation of the antioxidant and antimicrobial activities of porcine liver protein hydrolysates obtained using alcalase, bromelain, and papain. Appl. Sci. 10:2290. doi: 10.3390/app10072290
Bravo, F. I., Calvo, E., López-Villalba, R. A., Torres-Fuentes, C., Muguerza, B., García-Ruiz, A., et al. (2023). Valorization of chicken slaughterhouse byproducts to obtain antihypertensive peptides. Nutrients 15:15. doi: 10.3390/NU15020457
Bui, H. T. D., Khosravi, S., Fournier, V., Herault, M., and Lee, K.-J. (2014). Growth performance, feed utilization, innate immunity, digestibility and disease resistance of juvenile red seabream (Pagrus major) fed diets supplemented with protein hydrolysates. Aquaculture 418-419, 11–16. doi: 10.1016/j.aquaculture.2013.09.046
Cai, W.-W., Hu, X.-M., Wang, Y.-M., Chi, C.-F., and Wang, B. (2022). Bioactive peptides from skipjack tuna cardiac arterial bulbs: preparation, identification, antioxidant activity, and stability against thermal, pH, and simulated gastrointestinal digestion treatments. Mar. Drugs 20:626. doi: 10.3390/md20100626
Chakrabarti, S., Guha, S., and Majumder, K. (2018). Food-derived bioactive peptides in human health: challenges and opportunities. Nutrients 10:1738. doi: 10.3390/NU10111738
Chavez, M., and Uchanski, M. (2021). Insect left-over substrate as plant fertiliser. J. Insects Food Feed 7, 683–694. doi: 10.3920/JIFF2020.0063
Cheng, S., Tu, M., Chen, H., Xu, Z., Wang, Z., Liu, H., et al. (2018). Identification and inhibitory activity against α-thrombin of a novel anticoagulant peptide derived from oyster (Crassostrea gigas) protein. Food Funct. 9, 6391–6400. doi: 10.1039/C8FO01635F
Cheung, R. C. F., Ng, T. B., and Wong, J. H. (2015). Marine peptides: bioactivities and applications. Mar. Drugs 13, 4006–4043. doi: 10.3390/MD13074006
Chi, C.-F., Hu, F.-Y., Wang, B., Ren, X.-J., Deng, S.-G., and Wu, C.-W. (2015a). Purification and characterization of three antioxidant peptides from protein hydrolyzate of croceine croaker (Pseudosciaena crocea) muscle. Food Chem. 168, 662–667. doi: 10.1016/j.foodchem.2014.07.117
Chi, C.-F., Wang, B., Hu, F.-Y., Wang, Y.-M., Zhang, B., Deng, S.-G., et al. (2015b). Purification and identification of three novel antioxidant peptides from protein hydrolysate of bluefin leatherjacket (Navodon septentrionalis) skin. Food Res. Int. 73, 124–129. doi: 10.1016/j.foodres.2014.08.038
Chi, C.-F., Wang, B., Wang, Y.-M., Zhang, B., and Deng, S.-G. (2015c). Isolation and characterization of three antioxidant peptides from protein hydrolysate of bluefin leatherjacket (Navodon septentrionalis) heads. J. Funct. Foods 12, 1–10. doi: 10.1016/j.jff.2014.10.027
Colla, G., Nardi, S., Cardarelli, M., Ertani, A., Lucini, L., Canaguier, R., et al. (2015). Protein hydrolysates as biostimulants in horticulture. Sci. Hortic. 196, 28–38. doi: 10.1016/j.scienta.2015.08.037
Cruz-Casas, D. E., Aguilar, C. N., Ascacio-Valdés, J. A., Rodríguez-Herrera, R., Chávez-González, M. L., and Flores-Gallegos, A. C. (2021). Enzymatic hydrolysis and microbial fermentation: the most favorable biotechnological methods for the release of bioactive peptides. Food Chem. 3:100047. doi: 10.1016/J.FOCHMS.2021.100047
Da Silva, R. R. (2018). Enzymatic synthesis of protein hydrolysates from animal proteins: exploring microbial peptidases. Front. Microbiol. 9:735. doi: 10.3389/fmicb.2018.00735
Dai, Y. Q., Formo, E., Li, H. X., Xue, J. J., and Xia, Y. N. (2016). Surface-functionalized electrospun titania nanofibers for the scavenging and recycling of precious metal ions. ChemSusChem 9, 2912–2916. doi: 10.1002/cssc.201600787
Dey, S. S., and Dora, K. C. (2014). Optimization of the production of shrimp waste protein hydrolysate using microbial proteases adopting response surface methodology. J. Food Sci. Technol. 51, 16–24. doi: 10.1007/s13197-011-0455-4
dos Santos, C. M., Godoy, A. C., Oxford, J. H., Rodrigues, R., dos Santos Cardoso, M., Bittencourt, F., et al. (2021). Apparent digestibility of protein hydrolysates from chicken and swine slaughter residues for Nile tilapia. Aquaculture 530:735720. doi: 10.1016/J.AQUACULTURE.2020.735720
Emiroglu, A. O., Keskin, A., and Sen, M. (2018). Experimental investigation of the effects of Turkey rendering fat biodiesel on combustion, performance and exhaust emissions of a diesel engine. Fuel 216, 266–273. doi: 10.1016/j.fuel.2017.12.026
Etemadian, Y., Ghaemi, V., Shaviklo, A. R., Pourashouri, P., Sadeghi Mahoonak, A. R., and Rafipour, F. (2021). Development of animal/plant-based protein hydrolysate and its application in food, feed and nutraceutical industries: state of the art. J. Clean. Prod. 278:123219. doi: 10.1016/J.JCLEPRO.2020.123219
European Food Safety Authority (EFSA) . (2023). Animal by-products. Available at: https://food.ec.europa.eu/safety/animal-products_en
Fang, Y., Liu, J., Li, J., Chen, W., Huang, G., and Ding, Y. (2020). Rapid preparation of protein powder from high-moisture tuna liver: new insight into subcritical dimethyl ether. LWT 124:109179. doi: 10.1016/j.lwt.2020.109179
Fauzi, M. B., Lokanathan, Y., Aminuddin, B. S., BHI, R., and Chowdhury, S. R. (2016). Ovine tendon collagen: extraction, characterisation and fabrication of thin films for tissue engineering applications. Mater. Sci. Eng. C 68, 163–171. doi: 10.1016/j.msec.2016.05.109
Feng, D., Xue, Y., Li, Z., Wang, Y., and Xue, C. (2017). Effects of microwave radiation and water Bath heating on the physicochemical properties of Actomyosin from silver carp (Hypophthalmichthys molitrix) during setting. J. Food Process. Preserv. 41:e13031. doi: 10.1111/jfpp.13031
Fernández-Musoles, R., Castelló-Ruiz, M., Arce, C., Manzanares, P., Ivorra, M. D., and Salom, J. B. (2014). Antihypertensive mechanism of lactoferrin-derived peptides: angiotensin receptor blocking effect. J. Agric. Food Chem. 62, 173–181. doi: 10.1021/jf404616f
FitzGerald, R. J., Jakeman, P., Nongonierma, A. B., and Power, O. (2014). Food protein hydrolysates as a source of dipeptidyl peptidase IV inhibitory peptides for the management of type 2 diabetes. Proc. Nutr. Soc. 73, 34–46. doi: 10.1017/S0029665113003601
Fleming, M., Tai, Y., Zhuang, P., and MB, M. B. (2013). Extractability and bioavailability of Pb and as in historically contaminated orchard soil: effects of compost amendments. Environ. Pollut. 177, 90–97. doi: 10.1016/j.envpol.2013.02.013
Forman, H. J., and Zhang, H. (2021). Targeting oxidative stress in disease: promise and limitations of antioxidant therapy. Nat. Rev. Drug Discov. 20, 689–709. doi: 10.1038/s41573-021-00233-1
Franke-Whittle, I. H., and Insam, H. (2013). Treatment alternatives of slaughterhouse wastes, and their effect on the inactivation of different pathogens: a review. Crit. Rev. Microbiol. 39, 139–151. doi: 10.3109/1040841X.2012.694410
Gaind, S. (2014). Effect of fungal consortium and animal manure amendments on phosphorus fractions of paddy-straw compost. Int. Biodeterior. Biodegrad. 94, 90–97. doi: 10.1016/j.ibiod.2014.06.023
Gajanan, P. G., Elavarasan, K., and Shamasundar, B. A. (2016). Bioactive and functional properties of protein hydrolysates from fish frame processing waste using plant proteases. Environ. Sci. Pollut. Res. 23, 24901–24911. doi: 10.1007/s11356-016-7618-9
Giordano, D., Costantini, M., Coppola, D., Lauritano, C., Núñez Pons, L., Ruocco, N., et al. (2018). Biotechnological applications of bioactive peptides from marine sources. Adv. Microb. Physiol. 73, 171–220. doi: 10.1016/bs.ampbs.2018.05.002
González-Serrano, D. J., Hadidi, M., Varcheh, M., Jelyani, A. Z., Moreno, A., and Lorenzo, J. M. (2022). Bioactive peptide fractions from collagen hydrolysate of common carp fish byproduct: antioxidant and functional properties. Antioxidants 11:509. doi: 10.3390/ANTIOX11030509
Gooding, C. H., and Meeker, D. L. (2016). Review: comparison of 3 alternatives for large-scale processing of animal carcasses and meat by-products. Prof. Anim. Sci. 32, 259–270. doi: 10.15232/pas.2015-01487
Goosen, N. J., de Wet, L. F., and Görgens, J. F. (2014). The effects of protein hydrolysates on the immunity and growth of the abalone Haliotis midae. Aquaculture 428–429, 243–248. doi: 10.1016/j.aquaculture.2014.03.018
Harnedy, P. A., and FitzGerald, R. J. (2013). In vitro assessment of the cardioprotective, anti-diabetic and antioxidant potential of Palmaria palmata protein hydrolysates. J. Appl. Phycol. 25, 1793–1803. doi: 10.1007/s10811-013-0017-4
He, L., Wang, X., Wang, Y., Luo, J., Zhao, Y., Han, G., et al. (2023). Production and identification of dipeptidyl peptidase IV (DPP-IV) inhibitory peptides from discarded cowhide collagen. Food Chem. 405:134793. doi: 10.1016/j.foodchem.2022.134793
Hollmann, A., Martínez, M., Noguera, M. E., Augusto, M. T., Disalvo, A., Santos, N. C., et al. (2016). Role of amphipathicity and hydrophobicity in the balance between hemolysis and peptide-membrane interactions of three related antimicrobial peptides. Colloids Surf. B 141, 528–536. doi: 10.1016/j.colsurfb.2016.02.003
Hong, N., Chen, J., Gao, D., Chen, T.-B., Zhang, X.-H., and Cai, L. (2014). Enhanced water reduction by turning during sewage sludge composting. J. Chem. Technol. Biotechnol. 89, 756–762. doi: 10.1002/jctb.4183
Huang, S. L., Hung, C. C., Jao, C. L., Tung, Y. S., and Hsu, K. C. (2014). Porcine skin gelatin hydrolysate as a dipeptidyl peptidase IV inhibitor improves glycemic control in streptozotocin-induced diabetic rats. J. Funct. Foods 11, 235–242. doi: 10.1016/j.jff.2014.09.010
Huhe, J. C., Wu, Y., and Cheng, Y. (2017). Bacterial and fungal communities and contribution of physicochemical factors during cattle farm waste composting. Microbiology 6:e00518. doi: 10.1002/mbo3.518
Indumathi, P., and Mehta, A. (2016). A novel anticoagulant peptide from the nori hydrolysate. J. Funct. Foods 20, 606–617. doi: 10.1016/j.jff.2015.11.016
Iwaniak, A., Darewicz, M., and Minkiewicz, P. (2018). Peptides derived from foods as supportive diet components in the prevention of metabolic syndrome. Compr. Rev. Food Sci. Food Saf. 17, 63–81. doi: 10.1111/1541-4337.12321
Jagadeesan, Y., Meenakshisundaram, S., Raja, K., and Balaiah, A. (2023). Sustainable and efficient-recycling approach of chicken feather waste into liquid protein hydrolysate with biostimulant efficacy on plant, soil fertility and soil microbial consortium: a perspective to promote the circular economy. Process Saf. Environ. Prot. 170, 573–583. doi: 10.1016/j.psep.2022.12.029
Jin, Y., Yan, J., Yu, Y., and Qi, Y. (2015). Screening and identification of DPP-IV inhibitory peptides from deer skin hydrolysates by an integrated approach of LC-MS/MS and in silico analysis. J. Funct. Foods 18, 344–357. doi: 10.1016/j.jff.2015.07.015
Jovanović, J. R., Stefanović, A. B., Šekuljica, N. Ž., Tanasković, S. M. J., Dojčinović, M. B., Bugarski, B. M., et al. (2016). Ultrasound pretreatment as an useful tool to enhance egg white protein hydrolysis: kinetics, reaction model, and thermodinamics. J. Food Sci. 81, C2664–C2675. doi: 10.1111/1750-3841.13503
Karami, Z., and Akbari-Adergani, B. (2019). Bioactive food derived peptides: a review on correlation between structure of bioactive peptides and their functional properties. J. Food Sci. Technol. 56, 535–547. doi: 10.1007/S13197-018-3549-4
Karnjanapratum, S., O'Callaghan, Y. C., Benjakul, S., and O'Brien, N. (2016). Antioxidant, immunomodulatory and antiproliferative effects of gelatin hydrolysate from unicorn leatherjacket skin. J. Sci. Food Agric. 96, 3220–3226. doi: 10.1002/jsfa.7504
Karyotis, D., Skandamis, P. N., and Juneja, V. K. (2017). Thermal inactivation of listeria monocytogenes and Salmonella spp. in sous-vide processed marinated chicken breast. Food Res. Int. 100, 894–898. doi: 10.1016/j.foodres.2017.07.078
Kęska, P., Stadnik, J., Bąk, O., and Borowski, P. (2019). Meat proteins as dipeptidyl peptidase IV inhibitors and glucose uptake stimulating peptides for the management of a type 2 diabetes mellitus in silico study. Nutrients 11:2537. doi: 10.3390/nu11102537
Keskin, A. (2018). Two-step methyl ester production and characterization from the broiler rendering fat: the optimization of the first step. Renew. Energy 122, 216–224. doi: 10.1016/j.renene.2018.01.123
Ketnawa, S., Wickramathilaka, M., and Liceaga, A. M. (2018). Changes on antioxidant activity of microwave-treated protein hydrolysates after simulated gastrointestinal digestion: purification and identification. Food Chem. 254, 36–46. doi: 10.1016/j.foodchem.2018.01.133
Khadra, A., Ezzariai, A., Kouisni, L., and Hafidi, M. (2021). Helminth eggs inactivation efficiency by sludge co-composting under arid climates. Int. J. Environ. Health Res. 31, 530–537. doi: 10.1080/09603123.2019.1671960
Khiari, Z., Rico, D., Martin-Diana, A. B., and Barry-Ryan, C. (2014). Structure elucidation of ACE-inhibitory and antithrombotic peptides isolated from mackerel skin gelatine hydrolysates. J. Sci. Food Agric. 94, 1663–1671. doi: 10.1002/jsfa.6476
Kim, M. H., and Kim, G. (2017). Analysis of environmental impacts of burial sites. J. Mater. Cycles Waste Manag. 19, 432–442. doi: 10.1007/s10163-015-0439-y
Kong, Y., Li, S., Shao, Y., He, Z.-l., Chen, M.-m., Ming, X., et al. (2014). Antithrombotic peptides from Scolopendra subspinipes mutilans hydrolysates. Int. J. Pept. Res. Ther. 20, 245–252. doi: 10.1007/s10989-013-9387-3
König, H., and Fröhlich, J. (2017). “Lactic acid bacteria” in Biology of microorganisms on grapes, in must and in wine. eds. H. König, G. Unden, and J. Fröhlich (Cham: Springer International Publishing), 3–41.
Korhonen, H., and Pihlanto, A. (2006). Bioactive peptides: production and functionality. Int. Dairy J. 16, 945–960. doi: 10.1016/J.IDAIRYJ.2005.10.012
Krasaekoopt, W., and Watcharapoka, S. (2014). Effect of addition of inulin and galactooligosaccharide on the survival of microencapsulated probiotics in alginate beads coated with chitosan in simulated digestive system, yogurt and fruit juice. LWT 57, 761–766. doi: 10.1016/j.lwt.2014.01.037
Kumar, P., Chatli, M. K., Verma, A. K., Mehta, N., Malav, O. P., Kumar, D., et al. (2017). Quality, functionality, and shelf life of fermented meat and meat products: a review. Crit. Rev. Food Sci. Nutr. 57, 2844–2856. doi: 10.1080/10408398.2015.1074533
Kumar, P., Kizhakkedathu, J. N., and Straus, S. K. (2018). Antimicrobial peptides: diversity, mechanism of action and strategies to improve the activity and biocompatibility in vivo. Biomol. Ther. 8:8. doi: 10.3390/biom8010004
Kuo-Chiang, H., Yu-Shan, T., Shih-Li, H., and Chia-Ling, J. (2013). “Dipeptidyl peptidase-IV inhibitory activity of peptides in porcine skin gelatin hydrolysates” in Bioactive food peptides in health and disease. eds. H.-L. Blanca and H. Chia-Chien (Rijeka: IntechOpen), 8.
Lachhab, N., Sanzani, S. M., Bahouaoui, M. A., Boselli, M., and Ippolito, A. (2016). Effect of some protein hydrolysates against gray mould of table and wine grapes. Eur. J. Plant Pathol. 144, 821–830. doi: 10.1007/s10658-015-0749-x
Lachhab, N., Sanzani, S. M., Fallanaj, F., Youssef, K., Nigro, F., Boselli, M., et al. (2015). Protein hydrolysates as resistance inducers for controlling green mould of citrus fruit. Acta Hortic. 1065, 1593–1598. doi: 10.17660/ActaHortic.2015.1065.203
Lafarga, T., and Hayes, M. (2014). Bioactive peptides from meat muscle and by-products: generation, functionality and application as functional ingredients. Meat Sci. 98, 227–239. doi: 10.1016/j.meatsci.2014.05.036
Lafarga, T., Rai, D. K., O’Connor, P., and Hayes, M. (2015). A bovine fibrinogen-enriched fraction as a source of peptides with in vitro renin and angiotensin-I-converting enzyme inhibitory activities. J. Agric. Food Chem. 63, 8676–8684. doi: 10.1021/acs.jafc.5b03167
Lafarga, T., Rai, D. K., O'connor, P., and Hayes, M. (2016). Generation of bioactive hydrolysates and peptides from bovine hemoglobin with in vitro renin, angiotensin-I-converting enzyme and dipeptidyl peptidase-IV inhibitory activities. J. Food Biochem. 40, 673–685. doi: 10.1111/jfbc.12259
Lee, S. Y., and Hur, S. J. (2017). Antihypertensive peptides from animal products, marine organisms, and plants. Food Chem. 228, 506–517. doi: 10.1016/J.FOODCHEM.2017.02.039
Lee, S., Jo, K., Yong, H. I., Choi, Y. S., and Jung, S. (2021). Comparison of the in vitro protein digestibility of Protaetia brevitarsis larvae and beef loin before and after defatting. Food Chem. 338:128073. doi: 10.1016/j.foodchem.2020.128073
Lee, S. Y., Lee, D. Y., and Hur, S. J. (2021). Changes in the stability and antioxidant activities of different molecular weight bioactive peptide extracts obtained from beef during in vitro human digestion by gut microbiota. Food Res. Int. 141:110116. doi: 10.1016/J.FOODRES.2021.110116
Lepesteur, M. (2022). Human and livestock pathogens and their control during composting. Crit. Rev. Environ. Sci. Technol. 52, 1639–1683. doi: 10.1080/10643389.2020.1862550
Li, Y. Y., Fan, Y. Z., Liu, J. L., Meng, Z. S., Huang, A. X., Xu, F. R., et al. (2023). Identification, characterization and in vitro activity of hypoglycemic peptides in whey hydrolysates from rubing cheese by-product. Food Res. Int. 164:112382. doi: 10.1016/j.foodres.2022.112382
Li, Z.-R., Wang, B., Chi, C.-f., Zhang, Q.-H., Gong, Y.-d., Tang, J.-J., et al. (2013). Isolation and characterization of acid soluble collagens and pepsin soluble collagens from the skin and bone of Spanish mackerel (Scomberomorous niphonius). Food Hydrocoll. 31, 103–113. doi: 10.1016/j.foodhyd.2012.10.001
Li, Y., Yao, L., Zhang, L., Zhang, Y., Zheng, T., Liu, L., et al. (2021). Enhanced physicochemical stabilities of cyanidin-3-O-glucoside via combination with silk fibroin. Food Chem. 355:129479. doi: 10.1016/j.foodchem.2021.129479
Li, C., Yu, W., Wu, P., and Chen, X. D. (2020). Current in vitro digestion systems for understanding food digestion in human upper gastrointestinal tract. Trends Food Sci. Technol. 96, 114–126. doi: 10.1016/j.tifs.2019.12.015
Liang, Z., Sun, J., Yang, S., Wen, R., Liu, L., Du, P., et al. (2022). Fermentation of mung bean milk by Lactococcus lactis: focus on the physicochemical properties, antioxidant capacities and sensory evaluation. Food Biosci. 48:101798. doi: 10.1016/j.fbio.2022.101798
Lim, L. Y., Bong, C. P. C., Lee, C. T., Klemes, J., Sarmidi, M. R., and Lim, J. S. (2017). Review on the current composting practices and the potential of improvement using two-stage composting. Chem. Eng. Trans. 61, 1051–1056. doi: 10.3303/CET1761173
López-Pedrouso, M., Borrajo, P., Pateiro, M., Lorenzo, J. M., and Franco, D. (2020). Antioxidant activity and peptidomic analysis of porcine liver hydrolysates using alcalase, bromelain, flavourzyme and papain enzymes. Food Res. Int. 137:109389. doi: 10.1016/J.FOODRES.2020.109389
López-Pedrouso, M., Lorenzo, J. M., Bou, R., Vazquez, J. A., Valcarcel, J., Toldrà, M., et al. (2023a). Valorisation of pork by-products to obtain antioxidant and antihypertensive peptides. Food Chem. 423:136351. doi: 10.1016/J.FOODCHEM.2023.136351
López-Pedrouso, M., Zaky, A. A., Lorenzo, J. M., Camiña, M., and Franco, D. (2023b). A review on bioactive peptides derived from meat and by-products: extraction methods, biological activities, applications and limitations. Meat Sci. 204:109278. doi: 10.1016/j.meatsci.2023.109278
Lorca, G. L., Twiddy, T. A., and Saier, M. H. Jr. (2015). “Lactic acid bacteria: comparative genomic analyses of transport systems” in Biotechnology of lactic acid bacteria: novel applications. New York, America: John Wiley & Sons, Ltd. 55–79.
Lorenzo, J. M., Munekata, P. E. S., Gómez, B., Barba, F. J., Mora, L., Pérez-Santaescolástica, C., et al. (2018). Bioactive peptides as natural antioxidants in food products—a review. Trends Food Sci. Technol. 79, 136–147. doi: 10.1016/j.tifs.2018.07.003
Lucini, L., Rouphael, Y., Cardarelli, M., Canaguier, R., Kumar, P., and Colla, G. (2015). The effect of a plant-derived biostimulant on metabolic profiling and crop performance of lettuce grown under saline conditions. Sci. Hortic. 182, 124–133. doi: 10.1016/j.scienta.2014.11.022
Lv, Y., Wang, J., Gao, H., Wang, Z., Dong, N., Ma, Q., et al. (2014). Antimicrobial properties and membrane-active mechanism of a potential α-helical antimicrobial derived from cathelicidin PMAP-36. PLoS One 9:e86364. doi: 10.1371/journal.pone.0086364
Madende, M., and Hayes, M. (2020). Fish by-product use as biostimulants: An overview of the current state of the art, including relevant legislation and regulations within the EU and USA. Molecules 25:1122. doi: 10.3390/molecules25051122
Madhu, M., Kumar, D., Sirohi, R., Tarafdar, A., Dhewa, T., Aluko, R. E., et al. (2022). Bioactive peptides from meat: current status on production, biological activity, safety, and regulatory framework. Chemosphere 307:135650. doi: 10.1016/j.chemosphere.2022.135650
Maestri, E., Pavlicevic, M., Montorsi, M., and Marmiroli, N. (2019). Meta-analysis for correlating structure of bioactive peptides in foods of animal origin with regard to effect and stability. Compr. Rev. Food Sci. Food Saf. 18, 3–30. doi: 10.1111/1541-4337.12402
Mahdi, A. M., and Ojagh, S. (2017). Health benefits and food applications of bioactive compounds from fish byproducts: a review. J. Funct. Foods 35, 673–681. doi: 10.1016/j.jff.2017.06.034
Mahgoub, S., Alagawany, M., Nader, M., Omar, S. M., Abd El-Hack, M. E., Swelum, A., et al. (2021). Recent development in bioactive peptides from plant and animal products and their impact on the human health. Food Rev. Int. 39, 511–536. doi: 10.1080/87559129.2021.1923027
Mahmoodani, F., Ghassem, M., Babji, A. S., Yusop, S. M., and Khosrokhavar, R. (2014). ACE inhibitory activity of Pangasius catfish (Pangasius sutchi) skin and bone gelatin hydrolysate. J. Food Sci. Technol. 51, 1847–1856. doi: 10.1007/s13197-012-0742-8
Maky, M. A., and Zendo, T. (2021). Generation and characterization of novel bioactive peptides from fish and beef hydrolysates. Appl. Sci. 11:10452. doi: 10.3390/app112110452
Marciniak, A., Suwal, S., Naderi, N., Pouliot, Y., and Doyen, A. (2018). Enhancing enzymatic hydrolysis of food proteins and production of bioactive peptides using high hydrostatic pressure technology. Trends Food Sci. Technol. 80, 187–198. doi: 10.1016/j.tifs.2018.08.013
Martinez-Burgos, W. J., Bittencourt Sydney, E., Bianchi Pedroni Medeiros, A., Magalhães, A. I., de Carvalho, J. C., Karp, S. G., et al. (2021). Agro-industrial wastewater in a circular economy: characteristics, impacts and applications for bioenergy and biochemicals. Bioresour. Technol. 341:125795. doi: 10.1016/j.biortech.2021.125795
Mas-Capdevila, A., Iglesias-Carres, L., Arola-Arnal, A., Aragonès, G., Aleixandre, A., Bravo, F. I., et al. (2019). Evidence that nitric oxide is involved in the blood pressure lowering effect of the peptide AVFQHNCQE in spontaneously hypertensive rats. Nutrients 11:225. doi: 10.3390/nu11020225
McGauran, T., Dunne, N., Smyth, B. M., and Cunningham, E. (2021). Feasibility of the use of poultry waste as polymer additives and implications for energy, cost and carbon. J. Clean. Prod. 291:125948. doi: 10.1016/J.JCLEPRO.2021.125948
Meinert, L., Broge, E. H. L., Bejerholm, C., and Jensen, K. (2016). Application of hydrolyzed proteins of animal origin in processed meat. Food Sci. Nutr. 4, 290–297. doi: 10.1002/FSN3.289
Mekonnen, T., Mussone, P., and Bressler, D. (2016). Valorization of rendering industry wastes and co-products for industrial chemicals, materials and energy: review. Crit. Rev. Biotechnol. 36, 120–131. doi: 10.3109/07388551.2014.928812
Melini, F., Melini, V., Luziatelli, F., Ficca, A. G., and Ruzzi, M. (2019). Health-promoting components in fermented foods: an up-to-date systematic review. Nutrients 11:1189. doi: 10.3390/nu11051189
Michalopoulos, I., Mathioudakis, D., Premetis, I., Michalakidi, S., Papadopoulou, K., and Lyberatos, G. (2019). Anaerobic co-digestion in a pilot-scale periodic anaerobic baffled reactor (PABR) and composting of animal by-products and whey. Waste Biomass Valorization 10, 1469–1479. doi: 10.1007/s12649-017-0155-z
Mikhaylin, S., Boussetta, N., Vorobiev, E., and Bazinet, L. (2017). High voltage electrical treatments to improve the protein susceptibility to enzymatic hydrolysis. ACS Sustain. Chem. Eng. 5, 11706–11714. doi: 10.1021/acssuschemeng.7b03192
Ministry of Agriculture and Rural Affairs of the People’s Republic of China . (2020). National Fishery Economic Statistics Bulletin. Available at: https://www.gov.cn/xinwen/2021-07/30/content_5628346.htm. (Accessed November 16, 2023)
Minj, S., and Anand, S. (2020). Whey proteins and its derivatives: bioactivity, functionality, and current applications. Dairy 1, 233–258. doi: 10.3390/DAIRY1030016
Mirdhayati, I., Hermanianto, J., Wijaya, C. H., Sajuthi, D., and Arihara, K. (2016). Angiotensin converting enzyme (ACE) inhibitory and antihypertensive activities of protein hydrolysate from meat of Kacang goat (Capra aegagrus hircus). J. Sci. Food Agric. 96, 3536–3542. doi: 10.1002/JSFA.7538
Mora, L., Reig, M., and Toldrá, F. (2014). Bioactive peptides generated from meat industry by-products. Food Res. Int. 65, 344–349. doi: 10.1016/j.foodres.2014.09.014
Mora-Villalobos, J. A., Montero-Zamora, J., Barboza, N., Rojas-Garbanzo, C., Usaga, J., Redondo-Solano, M., et al. (2020). Multi-product lactic acid bacteria fermentations: a review. Fermentation 6:23. doi: 10.3390/fermentation6010023
Mozhiarasi, V., and Natarajan, T. S. (2022). Slaughterhouse and poultry wastes: management practices, feedstocks for renewable energy production, and recovery of value added products. Biomass Convers. Biorefin., 1–24. doi: 10.1007/s13399-022-02352-0
Nasri, R., Abdelhedi, O., Jemil, I., Daoued, I., Hamden, K., Kallel, C., et al. (2015). Ameliorating effects of goby fish protein hydrolysates on high-fat-high-fructose diet-induced hyperglycemia, oxidative stress and deterioration of kidney function in rats. Chem. Biol. Interact. 242, 71–80. doi: 10.1016/j.cbi.2015.08.003
Nasri, R., Abdelhedi, O., Nasri, M., and Jridi, M. (2022). Fermented protein hydrolysates: biological activities and applications. Curr. Opin. Food Sci. 43, 120–127. doi: 10.1016/j.cofs.2021.11.006
National Bureau of Statistics of China . Grain production has another bumper harvest and pig production has recovered quickly. (2023). Available at: http://m.ce.cn/bwzg/202101/19/t20210119_36237026.shtml. (Accessed November 16, 2023).
Ngo, D.-H., Vo, T.-S., Ryu, B., and Kim, S.-K. (2016). Angiotensin-I-converting enzyme (ACE) inhibitory peptides from Pacific cod skin gelatin using ultrafiltration membranes. Process Biochem. 51, 1622–1628. doi: 10.1016/j.procbio.2016.07.006
Nielsen, S. D., Beverly, R. L., Qu, Y., and Dallas, D. C. (2017). Milk bioactive peptide database: a comprehensive database of milk protein-derived bioactive peptides and novel visualization. Food Chem. 232, 673–682. doi: 10.1016/j.foodchem.2017.04.056
Nongonierma, A. B., Mooney, C., Shields, D. C., and RJ, F. G. (2014). In silico approaches to predict the potential of milk protein-derived peptides as dipeptidyl peptidase IV (DPP-IV) inhibitors. Peptides 57, 43–51. doi: 10.1016/j.peptides.2014.04.018
Norouzi, P., Mirmohammadi, M., and Houshdar Tehrani, M. H. (2022). Anticancer peptides mechanisms, simple and complex. Chem. Biol. Interact. 368:110194. doi: 10.1016/j.cbi.2022.110194
Nurdiawati, A., Suherman, C., Maxiselly, Y., Akbar, M. A., Purwoko, B. A., Prawisudha, P., et al. (2019). Liquid feather protein hydrolysate as a potential fertilizer to increase growth and yield of patchouli (Pogostemon cablin Benth) and mung bean (Vigna radiata). Int. J. Recycl. Org. Waste Agric. 8, 221–232. doi: 10.1007/s40093-019-0245-y
Owji, H., Nezafat, N., Negahdaripour, M., Hajiebrahimi, A., and Ghasemi, Y. (2018). A comprehensive review of signal peptides: structure, roles, and applications. Eur. J. Cell Biol. 97, 422–441. doi: 10.1016/J.EJCB.2018.06.003
Pagán, J., Benítez, R., and Ibarz, A. (2021). Effect of enzymatic hydrolyzed protein from pig bones on some biological and functional properties. J. Food Sci. Technol. 58, 4626–4635. doi: 10.1007/s13197-020-04950-0
Patil, P., Mandal, S., Tomar, S. K., and Anand, S. (2015). Food protein-derived bioactive peptides in management of type 2 diabetes. Eur. J. Nutr. 54, 863–880. doi: 10.1007/s00394-015-0974-2
Paul, T., Halder, S. K., Das, A., Bera, S., Maity, C., Mandal, A., et al. (2013). Exploitation of chicken feather waste as a plant growth promoting agent using keratinase producing novel isolate Paenibacillus woosongensis TKB2. Biocatal. Agric. Biotechnol. 2, 50–57. doi: 10.1016/j.bcab.2012.10.001
Peydayesh, M., Bagnani, M., Soon, W. L., and Mezzenga, R. (2022). Turning food protein waste into sustainable technologies. Chem. Rev. 123, 2112–2154. doi: 10.1021/acs.chemrev.2c00236
Phadke, G. G., Rathod, N. B., Ozogul, F., Elavarasan, K., Karthikeyan, M., Shin, K. H., et al. (2021). Exploiting of secondary raw materials from fish processing industry as a source of bioactive peptide-rich protein hydrolysates. Mar. Drugs 19:480. doi: 10.3390/MD19090480
Przybylski, R., Firdaous, L., Châtaigné, G., Dhulster, P., and Nedjar, N. (2016). Production of an antimicrobial peptide derived from slaughterhouse by-product and its potential application on meat as preservative. Food Chem. 211, 306–313. doi: 10.1016/j.foodchem.2016.05.074
Pujiastuti, D. Y., Ghoyatul Amin, M. N., Alamsjah, M. A., and Hsu, J. L. (2019). Marine organisms as potential sources of bioactive peptides that inhibit the activity of angiotensin I-converting enzyme: a review. Molecules 24:24. doi: 10.3390/molecules24142541
Qiao, M., Tu, M., Wang, Z., Mao, F., Chen, H., Qin, L., et al. (2018). Identification and antithrombotic activity of peptides from blue mussel (Mytilus edulis) protein. Int. J. Mol. Sci. 19:138. doi: 10.3390/ijms19010138
Rai, R., Singh, R. K., and Suthar, S. (2021). Production of compost with biopesticide property from toxic weed Lantana: quantification of alkaloids in compost and bacterial pathogen suppression. J. Hazard. Mater. 401:123332. doi: 10.1016/j.jhazmat.2020.123332
Ramakrishnan, S. R., Jeong, C. R., Park, J. W., Cho, S. S., and Kim, S. J. (2023). A review on the processing of functional proteins or peptides derived from fish by-products and their industrial applications. Heliyon 9:e14188. doi: 10.1016/J.HELIYON.2023.E14188
Razzaq, A., Shamsi, S., Ali, A., Ali, Q., Sajjad, M., Malik, A., et al. (2019). Microbial proteases applications. Front. Bioeng. Biotechnol. 7:110. doi: 10.3389/fbioe.2019.00110
Rivero-Pino, F., Espejo-Carpio, F. J., and Guadix, E. M. (2020). Production and identification of dipeptidyl peptidase IV (DPP-IV) inhibitory peptides from discarded sardine pilchardus protein. Food Chem. 328:127096. doi: 10.1016/j.foodchem.2020.127096
Romanazzi, G., Feliziani, E., Santini, M., and Landi, L. (2013). Effectiveness of postharvest treatment with chitosan and other resistance inducers in the control of storage decay of strawberry. Postharvest Biol. Technol. 75, 24–27. doi: 10.1016/j.postharvbio.2012.07.007
Romanazzi, G., Smilanick, J. L., Feliziani, E., and Droby, S. (2016). Integrated management of postharvest gray mold on fruit crops. Postharvest Biol. Technol. 113, 69–76. doi: 10.1016/j.postharvbio.2015.11.003
Sadh, P. K., Kumar, S., Chawla, P., and Duhan, J. S. (2018). Fermentation: a boon for production of bioactive compounds by processing of food industries wastes (by-products). Molecules 23:2560. doi: 10.3390/molecules23102560
Sauer, J., and Merchant, H. (2018). “Physiology of the gastrointestinal system” in Comprehensive toxicology (Oxford: Elsevier), 16–44.
Schmidt, N. W., and Wong, G. C. L. (2013). Antimicrobial peptides and induced membrane curvature: geometry, coordination chemistry, and molecular engineering. Curr. Opinion Solid State Mater. Sci. 17, 151–163. doi: 10.1016/j.cossms.2013.09.004
Schömig, V. J., Käsdorf, B. T., Scholz, C., Bidmon, K., Lieleg, O., and Berensmeier, S. (2016). An optimized purification process for porcine gastric mucin with preservation of its native functional properties. RSC Adv. 6, 44932–44943. doi: 10.1039/c6ra07424c
Sensoy, I. (2021). A review on the food digestion in the digestive tract and the used in vitro models. Curr. Res. Food Sci. 4, 308–319. doi: 10.1016/j.crfs.2021.04.004
Shi, Y., and Ge, L. (2020). Effects of production conditions on physicochemical and flavor quality of lard. Meat Res. 34, 40–45. doi: 10.7506/rlyj1001-8123-20191231-315
Sila, A., and Bougatef, A. (2016). Antioxidant peptides from marine by-products: isolation, identification and application in food systems: a review. J. Funct. Foods 21, 10–26. doi: 10.1016/j.jff.2015.11.007
Sila, A., Sayari, N., Balti, R., Martinez-Alvarez, O., Nedjar-Arroume, N., Moncef, N., et al. (2014). Biochemical and antioxidant properties of peptidic fraction of carotenoproteins generated from shrimp by-products by enzymatic hydrolysis. Food Chem. 148, 445–452. doi: 10.1016/j.foodchem.2013.05.146
Siltari, A., Korpela, R., and Vapaatalo, H. (2016). Bradykinin—induced vasodilatation: role of age, ACE1-inhibitory peptide, mas-and bradykinin receptors. Peptides 85, 46–55. doi: 10.1016/j.peptides.2016.09.001
Silva, J. F. X., Ribeiro, K., Silva, J. F., Cahú, T. B., and Bezerra, R. S. (2014). Utilization of tilapia processing waste for the production of fish protein hydrolysate. Anim. Feed Sci. Technol. 196, 96–106. doi: 10.1016/j.anifeedsci.2014.06.010
Silveira, S. T., Martínez-Maqueda, D., Recio, I., and Hernández-Ledesma, B. (2013). Dipeptidyl peptidase-IV inhibitory peptides generated by tryptic hydrolysis of a whey protein concentrate rich in β-lactoglobulin. Food Chem. 141, 1072–1077. doi: 10.1016/j.foodchem.2013.03.056
Soltaninejad, H., Zare-Zardini, H., Ordooei, M., Ghelmani, Y., Ghadiri-Anari, A., Mojahedi, S., et al. (2021). Antimicrobial peptides from amphibian innate immune system as potent antidiabetic agents: a literature review and bioinformatics analysis. J. Diabetes Res. 2021, 1–10. doi: 10.1155/2021/2894722
Song, P., Zhang, X., Wang, S. H., Xu, W., Wang, F., Fu, R. Z., et al. (2023). Microbial proteases and their applications. Front. Microbiol. 14:1236368. doi: 10.3389/fmicb.2023.1236368
Su, G., Zhao, T., Zhao, Y., Sun-Waterhouse, D., Qiu, C., Huang, P., et al. (2016). Effect of anchovy (Coilia mystus) protein hydrolysate and its Maillard reaction product on combating memory-impairment in mice. Food Res. Int. 82, 112–120. doi: 10.1016/J.FOODRES.2016.01.022
Subhan, F., Hussain, Z., Tauseef, I., Shehzad, A., and Wahid, F. (2021). A review on recent advances and applications of fish collagen. Crit. Rev. Food Sci. Nutr. 61, 1027–1037. doi: 10.1080/10408398.2020.1751585
Sun, N., Wu, H., Du, M., Tang, Y., Liu, H., Fu, Y., et al. (2016). Food protein-derived calcium chelating peptides: a review. Trends Food Sci. Technol. 58, 140–148. doi: 10.1016/J.TIFS.2016.10.004
Tacias-Pascacio, V. G., Castaneda-Valbuena, D., Morellon-Sterling, R., Tavano, O., Berenguer-Murcia, Á., Vela-Gutiérrez, G., et al. (2021). Bioactive peptides from fisheries residues: a review of use of papain in proteolysis reactions. Int. J. Biol. Macromol. 184, 415–428. doi: 10.1016/j.ijbiomac.2021.06.076
Tang, W., Zhang, H., Wang, L., Qian, H., and Qi, X. (2015). Targeted separation of antibacterial peptide from protein hydrolysate of anchovy cooking wastewater by equilibrium dialysis. Food Chem. 168, 115–123. doi: 10.1016/j.foodchem.2014.07.027
Tavano, O. L. (2013). Protein hydrolysis using proteases: an important tool for food biotechnology. J. Mol. Catal. B 90, 1–11. doi: 10.1016/j.molcatb.2013.01.011
Teshnizi, Z. M., Robatjazi, S. M., and Mosaabadi, J. M. (2020). Optimization of the enzymatic hydrolysis of poultry slaughterhouse wastes using alcalase enzyme for the preparation of protein hydrolysates. Appl. Food Biotechnol. 7, 153–160. doi: 10.22037/afb.v7i3.28417
Thakur, R., Santhosh, R., Kumar, Y., Suryavanshi, V. R., Singhi, H., Madhubabu, D., et al. (2023). Characteristics and application of animal byproduct-based films and coatings in the packaging of food products. Trends Food Sci. Technol. 140:104143. doi: 10.1016/J.TIFS.2023.104143
Thomson, A., Price, G. W., Arnold, P., Dixon, M., and Graham, T. (2022). Review of the potential for recycling CO2 from organic waste composting into plant production under controlled environment agriculture. J. Clean. Prod. 333:130051. doi: 10.1016/j.jclepro.2021.130051
Thoresen, P. P., Álvarez, R. G., Vaka, M. R., Rustad, T., Sone, I., and Fernández, E. N. (2020). Potential of innovative pre-treatment technologies for the revalorisation of residual materials from the chicken industry through enzymatic hydrolysis. Innov. Food Sci. Emerg. Technol. 64:102377. doi: 10.1016/j.ifset.2020.102377
Timorshina, S., Popova, E., and Osmolovskiy, A. (2022). Sustainable applications of animal waste proteins. Polymers 14:1601. doi: 10.3390/polym14081601
Tran, N. V. N., Yu, Q. J., Nguyen, T. P., and Wang, S. L. (2020). Coagulation of chitin production wastewater from shrimp scraps with by-product chitosan and chemical coagulants. Polymers 12:12. doi: 10.3390/polym12030607
Tyagi, A., Daliri, E. B.-M., Kwami Ofosu, F., Yeon, S.-J., and Oh, D.-H. (2020). Food-derived opioid peptides in human health: a review. Int. J. Mol. Sci. 21:8825. doi: 10.3390/ijms21228825
U.S. Food and Drug Administration (FDA) . (2022). Food code. Available at: https://www.fda.gov/food/fda-food-code/food-code-2022
Ucak, I., Afreen, M., Montesano, D., Carrillo, C., Tomasevic, I., Simal-Gandara, J., et al. (2021). Functional and bioactive properties of peptides derived from marine side streams. Mar. Drugs 19:19. doi: 10.3390/MD19020071
Udenigwe, C. C., and Howard, A. (2013). Meat proteome as source of functional biopeptides. Food Res. Int. 54, 1021–1032. doi: 10.1016/j.foodres.2012.10.002
Ullah, S., Zainol, I., Chowdhury, S. R., and Fauzi, M. B. (2018). Development of various composition multicomponent chitosan/fish collagen/glycerin 3D porous scaffolds: effect on morphology, mechanical strength, biostability and cytocompatibility. Int. J. Biol. Macromol. 111, 158–168. doi: 10.1016/j.ijbiomac.2017.12.136
Valdez-Miramontes, C. E., De Haro-Acosta, J., Aréchiga-Flores, C. F., Verdiguel-Fernández, L., and Rivas-Santiago, B. (2021). Antimicrobial peptides in domestic animals and their applications in veterinary medicine. Peptides 142:170576. doi: 10.1016/j.peptides.2021.170576
Vázquez, J. A., Menduíña, A., Nogueira, M., Durán, A. I., Sanz, N., and Valcarcel, J. (2020). Optimal production of protein hydrolysates from monkfish by-products: chemical features and associated biological activities. Molecules 25:4068. doi: 10.3390/molecules25184068
Velusamy, M., Chakali, B., Ganesan, S., Tinwala, F., and Shanmugham Venkatachalam, S. (2020). Investigation on pyrolysis and incineration of chrome-tanned solid waste from tanneries for effective treatment and disposal: an experimental study. Environ. Sci. Pollut. Res. 27, 29778–29790. doi: 10.1007/s11356-019-07025-6
Verma, A. K., Chatli, M. K., Kumar, P., and Mehta, N. (2019). Antioxidant and antimicrobial activity of porcine liver hydrolysate in meat emulsion and their influence on physico-chemical and color deterioration during refrigeration storage. J. Food Sci. 84, 1844–1853. doi: 10.1111/1750-3841.14683
Visconti, F., de Paz, J. M., Bonet, L., Jordà, M., Quiñones, A., and Intrigliolo, D. S. (2015). Effects of a commercial calcium protein hydrolysate on the salt tolerance of Diospyros kaki L. cv. “Rojo Brillante” grafted on Diospyros lotus L. Sci. Hortic. 185, 129–138. doi: 10.1016/j.scienta.2015.01.028
Wadhwa, M., and Bakshi, M. P. S. (2016). “Application of waste-derived proteins in the animal feed industry” in Protein byproducts: transformation from environmental burden into value-added products. Cambridge, Massachusetts: Elsevier Inc. Academic Press, 161–192.
Wan, X., Li, J., Xie, L., Wei, Z., Wu, J., Wah Tong, Y., et al. (2022). Machine learning framework for intelligent prediction of compost maturity towards automation of food waste composting system. Bioresour. Technol. 365:128107. doi: 10.1016/j.biortech.2022.128107
Wang, X., Chen, H., Fu, X., Li, S., and Wei, J. (2017). A novel antioxidant and ACE inhibitory peptide from rice bran protein: biochemical characterization and molecular docking study. LWT 75, 93–99. doi: 10.1016/j.lwt.2016.08.047
Wang, K., Luo, Q., Hong, H., Liu, H., and Luo, Y. (2021). Novel antioxidant and ACE inhibitory peptide identified from Arthrospira platensis protein and stability against thermal/pH treatments and simulated gastrointestinal digestion. Food Res. Int. 139:109908. doi: 10.1016/j.foodres.2020.109908
Wang, C.-K., Shih, L.-Y., and Chang, K. Y. (2017). Large-scale analysis of antimicrobial activities in relation to amphipathicity and charge reveals novel characterization of antimicrobial peptides. Molecules 22:2037. doi: 10.3390/molecules22112037
Wang, B., Yu, Z., Yokoyama, W., Chiou, B. S., Chen, M., Liu, F., et al. (2021). Collagen peptides with DPP-IV inhibitory activity from sheep skin and their stability to in vitro gastrointestinal digestion. Food. Bioscience 42:101161. doi: 10.1016/j.fbio.2021.101161
Wang, S., Zeng, X., Yang, Q., and Qiao, S. (2016). Antimicrobial peptides as potential alternatives to antibiotics in food animal industry. Int. J. Mol. Sci. 17:603. doi: 10.3390/IJMS17050603
Wen, Q., Zhang, L., Chen, Y., Su, Y., Yu, J., Chen, P., et al. (2023a). Novel applications of silk proteins based on their interactions with metal ions. Sustainability 15:16053. doi: 10.3390/su152216053
Wen, Q., Zhang, L., Zhao, F., Chen, Y., Su, Y., Zhang, X., et al. (2023b). Production technology and functionality of bioactive peptides. Curr. Pharm. Des. 29, 652–674. doi: 10.2174/1381612829666230201121353
Xu, J., Li, Y., Regenstein, J., and Su, X. (2017). In vitro and in vivo anti-oxidation and anti-fatigue effect of monkfish liver hydrolysate. Food Biosci. 18, 9–14. doi: 10.1016/j.fbio.2017.03.002
Yamamoto, T., Noma, Y., and Sakai, S.-I. (2018). Thermal destruction of wastes containing polychlorinated naphthalenes in an industrial waste incinerator. Environ. Sci. Pollut. Res. 25, 31819–31827. doi: 10.1007/s11356-016-7100-8
Yang, S., Lee, C. W., Kim, H. J., Jung, H.-H., Kim, J. I., Shin, S. Y., et al. (2019). Structural analysis and mode of action of BMAP-27, a cathelicidin-derived antimicrobial peptide. Peptides 118:170106. doi: 10.1016/j.peptides.2019.170106
Yang, C., Yao, L., and Zhang, L. (2023). Silk sericin-based biomaterials shine in food and pharmaceutical industries. Smart Mater. Med. 4, 447–459. doi: 10.1016/j.smaim.2023.01.003
Yao, L., Hao, M., Zhao, F., Wang, Y., Zhou, Y., Liu, Z., et al. (2022). Fabrication of silk sericin-anthocyanin nanocoating for chelating and saturation-visualization detection of metal ions. Nanoscale 14, 17277–17289. doi: 10.1039/d2nr04047f
Yu, D., Chi, C.-F., Wang, B., Ding, G.-F., and Li, Z.-R. (2014). Characterization of acid-and pepsin-soluble collagens from spines and skulls of skipjack tuna (Katsuwonus pelamis). Chin. J. Nat. Med. 12, 712–720. doi: 10.1016/S1875-5364(14)60110-2
Yuan, Q., Snow, D. D., and Bartelt-Hunt, S. L. (2013). Potential water quality impacts originating from land burial of cattle carcasses. Sci. Total Environ. 456–457, 246–253. doi: 10.1016/j.scitotenv.2013.03.083
Zaky, A. A., Simal-Gandara, J., Eun, J. B., Shim, J. H., and Abd El-Aty, A. M. (2022). Bioactivities, applications, safety, and health benefits of bioactive peptides from food and by-products: a review. Front. Nutr. 8:815460. doi: 10.3389/FNUT.2021.815640/FULL
Zambrowicz, A., Eckert, E., Pokora, M., Bobak, Ł., Dąbrowska, A., Szołtysik, M., et al. (2015). Antioxidant and antidiabetic activities of peptides isolated from a hydrolysate of an egg-yolk protein by-product prepared with a proteinase from Asian pumpkin (Cucurbita ficifolia). RSC Adv. 5, 10460–10467. doi: 10.1039/C4RA12943A
Zamorano-Apodaca, J. C., García-Sifuentes, C. O., Carvajal-Millán, E., Vallejo-Galland, B., Scheuren-Acevedo, S. M., and Lugo-Sánchez, M. E. (2020). Biological and functional properties of peptide fractions obtained from collagen hydrolysate derived from mixed by-products of different fish species. Food Chem. 331:127350. doi: 10.1016/j.foodchem.2020.127350
Zhang, D. L., Guan, R. Z., Huang, W. S., and Xiong, J. (2013). Isolation and characterization of a novel antibacterial peptide derived from hemoglobin alpha in the liver of Japanese eel, Anguilla japonica. Fish Shellfish Immunol. 35, 625–631. doi: 10.1016/j.fsi.2012.08.022
Zhang, L., Hao, M., Yao, L., Xing, C., Wen, Q., Zhang, Z., et al. (2023a). Sericin “hairpin structure”-based multifunctional anthocyanin nanoencapsulation for remodeling ROS-dependent cutaneous wound healing. Chem. Eng. J. 475:145863. doi: 10.1016/j.cej.2023.145863
Zhang, L., Yao, L., Zhao, F., Yu, A., Zhou, Y., Wen, Q., et al. (2023b). Protein and peptide-based nanotechnology for enhancing stability, bioactivity, and delivery of anthocyanins. Adv. Healthcare Mater. 12:e2300473. doi: 10.1002/adhm.202300473
Zhao, X., Zhang, X., and Liu, D. (2021). Collagen peptides and the related synthetic peptides: a review on improving skin health. J. Funct. Foods 86:104680. doi: 10.1016/j.jff.2021.104680
Zhu, Y., Lao, F., Pan, X., and Wu, J. (2022). Food protein-derived antioxidant peptides: molecular mechanism, stability and bioavailability. Biomol. Ther. 12:1622. doi: 10.3390/BIOM12111622
Zou, Y., Li, P. P., Zhang, K., Wang, L., Zhang, M. H., Sun, Z. L., et al. (2017). Effects of ultrasound-assisted alkaline extraction on the physiochemical and functional characteristics of chicken liver protein isolate. Poult. Sci. 96, 2975–2985. doi: 10.3382/ps/pex049
Zou, Y., Shahidi, F., Shi, H., Wang, J., Huang, Y., Xu, W., et al. (2021). Values-added utilization of protein and hydrolysates from animal processing by-product livers: a review. Trends Food Sci. Technol. 110, 432–442. doi: 10.1016/j.tifs.2021.02.033
Zou, Y., Shi, H., Chen, X., Xu, P., Jiang, D., Xu, W., et al. (2019). Modifying the structure, emulsifying and rheological properties of water-soluble protein from chicken liver by low-frequency ultrasound treatment. Int. J. Biol. Macromol. 139, 810–817. doi: 10.1016/j.ijbiomac.2019.08.062
Zou, H., Wang, H., Zhang, Z., Lin, H., and Li, Z. (2023). Immune regulation by fermented milk products: the role of the proteolytic system of lactic acid bacteria in the release of immunomodulatory peptides. Crit. Rev. Food Sci. Nutr., 1–19. doi: 10.1080/10408398.2023.2225200
Glossary
Keywords: animal waste protein, valorization, bioactive peptide, functional ingredient, agriculture, food, pharmaceutical
Citation: Boboua SYB, Wen Q, Zhang L, Chen Y, Yu J, Chen P, Sun Y and Zheng T (2024) Valorization of animal waste proteins for agricultural, food production, and medicinal applications. Front. Sustain. Food Syst. 8:1366333. doi: 10.3389/fsufs.2024.1366333
Edited by:
Debabandya Mohapatra, Indian Council of Agricultural Research (ICAR), IndiaReviewed by:
Muthukumar Serva Peddha, Central Food Technological Research Institute (CSIR), IndiaPoonam Singh, LaserLeap Technologies (Portugal), Portugal
Guadalupe Virginia Nevárez-Moorillón, Autonomous University of Chihuahua, Mexico
Copyright © 2024 Boboua, Wen, Zhang, Chen, Yu, Chen, Sun and Zheng. This is an open-access article distributed under the terms of the Creative Commons Attribution License (CC BY). The use, distribution or reproduction in other forums is permitted, provided the original author(s) and the copyright owner(s) are credited and that the original publication in this journal is cited, in accordance with accepted academic practice. No use, distribution or reproduction is permitted which does not comply with these terms.
*Correspondence: Lei Zhang, bDc4emhhbmdAdXdhdGVybG9vLmNh; Yong Sun, c3VueW9uZ0BuZWF1LmVkdS5jbg==; Tao Zheng, emhlbmd0YW9AbXMuZ2llYy5hYy5jbg==
†These authors have contributed equally to this work