- 1College of Animal Science, Guizhou University, Guiyang, China
- 2School of Agriculture and the Environment, Massey University, Palmerston North, New Zealand
The availability of soil phosphorus (P), a crucial nutrient influencing plant productivity and ecosystem function, is impacted by continuously increasing nitrogen (N) enrichment, which changes the soil P cycle. The effect of varying forms of N input on soil P dynamics in P-limited karst grassland ecosystems remains unclear. To address this knowledge gap, we conducted a greenhouse experiment to explore the effects of various forms of N addition [Ca(NO3)2, NH4Cl, NH4NO3, Urea] on soil P fractions in these ecosystems, applying two levels (N1: 50 mg N kg−1soil, N2: 100 mg N kg−1soil) of N input in two soils (yellow soil, limestone soil). Results indicated that P fractions in both soil types were significantly affected by N additions, with yellow soil demonstrating a higher sensitivity to these additions, and this effect was strongly modulated by the form and level of N added. High N addition, rather than low N, significantly affect the P fractions in both soil types. Specially, except for Ca(NO 3 ) 2 , high N addition significantly increased the available P in both soils, following the order: Urea and NH 4 NO 3 > NH 4 Cl > Ca(NO 3 ) 2 , and decreased NaHCO 3 -Pi in both soils. High N addition also significantly reduced NaOH-Po and C.HCl-Po fractions in yellow soil. Additionally, the response of root biomass and alkaline phosphatase activity in both soils to N input paralleled the trends observed in the available P fractions. Notably, changes in soil available P were strongly correlated with plant root biomass and soil alkaline phosphatase activity. Our study highlights that the N addition form significantly influences soil P availability, which is closely tied to plant root biomass and alkaline phosphatase activity. This finding underscores the importance of considering N input form to boost soil fertility and promote sustainable agriculture.
1 Introduction
Phosphorus (P) is a crucial nutrient required for plant growth and the integrity of terrestrial ecosystem function, playing a pivotal role in biodiversity conservation, global sustainability, and biogeochemical cycling (Sattari et al., 2016; Hou et al., 2021; Bai and Cotrufo, 2022). A significant amount of P in soil is bound by insoluble compounds, leaving only a limited amount of inorganic P accessible to plants and microbes, leading to widespread P limitation across terrestrial ecosystems (Ashley et al., 2011; Ahmad et al., 2018). Concurrently, nitrogen (N) enrichment from atmospheric deposition, biological fixation, and over-fertilization has more than doubled the input of reactive N in various forms, such as ammonium, nitrate, and organic N, including urea and amino acids (Penuelas et al., 2020). This increase in N input not only alters P cycling in the soil but also exacerbates P scarcity in ecosystems (Hou et al., 2021). Understanding the effects of N input on soil P cycling is therefore crucial for predicting the future functioning of terrestrial ecosystems.
P in soil exists in multiple complex chemical forms, and its availability, behavior, and mobility change with varying soil conditions (Wang et al., 2022). Previous studies have developed a framework categorizing inorganic (Pi) and organic P (Po) into different labile and non-labile pools via a sequential fractionation procedure (Hedley et al., 1982; Tiessen and Moir, 1993). N input can affect these P fractions by altering factors such as soil pH, microbial activity, content of alkaline cations, cation exchange capacity, and plant biomass (Mahmood et al., 2021; Cui et al., 2022). Despite extensive research, findings on the effects of N on soil P fractions are inconsistent. For instance, some studies have found that N input increases NaHCO3-Pi and decreases or does not affect moderate-labile P fractions (Liu et al., 2022; Guan et al., 2023). Conversely, other studies indicate that N addition reduces labile P (Resin-Pi, NaHCO3-Pi and NaHCO3-Po) while increasing moderately labile P (NaOH-Pi, NaOH-Po) (Kai et al., 2014; Chen et al., 2018; Zhang and Shenglei, 2020). At the same time, compared to labile and moderately labile P, non-labile P fractions are less affected by N input (Chen et al., 2018; Liu et al., 2022; Guan et al., 2023). Additionally, while some studies suggest that N input generally increases soil inorganic P and reduces soil organic P fractions (Jing et al., 2021), there are also reports to the contrary (Zhang et al., 2022). Such variation could be attributed to the different N forms used in these studies (Hu et al., 2022). As terrestrial ecosystems receive a variety of N inputs, it’s important to understand how these different N forms affect soil P fractions.
Phosphatases, particularly acid phosphatase, and alkaline phosphatase, secreted by plant roots and soil microorganisms, are crucial in the transformation and cycling of soil P (Fan et al., 2019; Wang et al., 2023). N input is known to alter the activity of these enzymes, which catalyze the conversion of organically bound P to inorganic P (Yokoyama et al., 2017; Chen et al., 2020). While acid phosphatase is primarily produced by plants, bacteria, and fungi, alkaline phosphatase is predominantly secreted by soil microorganisms, especially bacteria (Nannipieri et al., 2011; Cao et al., 2022). The phoC gene and phoD gene, encoding soil acid and alkaline phosphatase respectively, play a significant role in the soil P cycle (Tan et al., 2013; Wang et al., 2021). However, the specific effects of different N forms, such as ammonium, nitrate, and organic N, on phosphatase activity and the abundance of these genes are not well understood.
Karst ecosystems are widely distributed around the world, and the subtropical karst area located in southwest China is one of the largest carbonate bedrock development areas in the world (Li et al., 2018; Wu et al., 2020). In the past 20 years, most degraded land in the karst region has been gradually transformed into woodland and grassland (Wen et al., 2016). During this succession process, the soil N content has increased, primarily due to atmospheric N deposition, fertilizer application, and biological N fixation. However, due to the shallow, uneven and fragile characteristics, the soil in this area is susceptible to leaching, resulting in the loss of N and P (Wen et al., 2016; Fenton et al., 2017; Xiao et al., 2020). Moreover, in karst areas, the high calcium ion content in the soil leads to a serious lack of P, which makes P a key nutrient limiting secondary succession and ecological restoration in the southwest karst area (Liu et al., 2018). Despite the substantial N input from land use activities, few studies have focused on the impact of different N forms on soil P fractions in karst areas. This study aims to explore these effects through greenhouse experiments, examining changes in soil P fractions, phosphatase activity, and the abundance of phoC and phoD genes under various N additions. We hypothesize that: (1) different N forms have distinct impacts on soil P fractions, considering that different forms of N have different effects on factors that control soil P cycling; (2) alterations in soil P fractions due to N input are predominantly influenced by biotic factors, considering that abiotic factors typically exhibit less variability in the short term.
2 Materials and methods
2.1 Experimental design and treatments
We conducted a greenhouse experiment to explore the effect of N input on soil P fractions at Guizhou University (106° 39′ 29″ E, 26° 26′ 59″ N), Guiyang, Guizhou. The experiment comprised three N addition levels (N0: 0 mg N kg−1 soil,N1: 50 mg N kg−1 soil, N2: 100 mg N kg−1 soil) combined with four forms of N [Ca(NO3)2, NH4Cl, NH4NO3 and Urea] and two soil types (yellow soil and limestone soil). This resulted in a total of 72 experimental pots, with each treatment replicated four times. Topsoil samples (0–15 cm) of two types of soils were collected from Guizhou, located in the southwestern karst area’s core area, which are the primary soil types in the karst region (Piao et al., 2000; Zhang et al., 2021). The limestone soil was collected from Dafang County grasslands and yellow soil from Huaxi County grasslands (Table 1). Based on the FAO-UNESCO soil classification framework, the limestone soil utilized in our research is classified as Calcic Cambisol, and the yellow soil is designated as Ferralsol. The soils were air-dried at room temperature, homogenized and placed into pots (16 cm in diameter, 12 cm in depth), each containing 1,500 g of soil with an average particle size of <2 mm. The greenhouse was maintained under a 20°C/15°C day (16 h)/night (8 h) temperature cycle.
White clover (Trifolium repens L.) and perennial ryegrass (Lolium perenne L.) were selected as their prevalence in the area’s mixed plantings. Each pot was planted with eight seedlings (four perennial ryegrass and four white clovers). The experiment was carried out in a greenhouse during the autumn and winter (August to December), with plants watered 1–2 times weekly to maintain soil water holding capacity at about 60%. Seedling management and pest control were also conducted (Van Lenteren, 1988). Specifically, clear weeds in time during seedling stage, spray 50% carbendazim and 50% mancozeb 500 times solution to control pests and diseases after thin out seedling (Zhang et al., 2004).
2.2 Sampling and chemical analysis
After 4 months, the plants were harvested, divided into above and below-ground parts, and the roots were dried at 65°C for 48 h to measure dry weight. Soil from each pot was sieved (2 mm mesh) and divided into three subsamples for different analyses. The first subsample was stored at −80°C for phoC and phoD gene abundance analysis. The second subsample was kept at 4°C for phosphatase activity determination, and the third subsample was air-dried for assessing soil physical and chemical properties and P fractions.
2.3 Soil chemical and biological properties
Soil samples were dried at 105°C for soil moisture content (SM) determination (Geng et al., 2022). Soil pH was measured in triplicate at a 1: 5 soil to deionized water (w/v) using digital PHS-3E/pH meter (Leici Instrument Branch of Shanghai Yidian Scientific Instrument Co., Ltd., Shanghai, China); NH4+-N and NO3−-N concentrations were assessed with a continuous flow analyzer (Vario EL III, Elementar, Germany) after 2 mol L−1 KCl solution leaching (Ding et al., 2023). Soil phosphatase activities (S-ACP and S-ALP) were measured using commercially available kits (Beijing Solarbio Technology Co., Ltd.), with the activity determined by the phenol produced at 660 nm after hydrolyzing benzene disodium phosphate (Ding et al., 2023; Yang et al., 2023).
2.4 Soil P fractions
The soil P fractions were determined using the Hedley continuous extraction method (Hedley et al., 1982). The procedure is detailed below: A 0.5 g sample of air-dried soil, sieved through a 2 mm mesh, was placed in a 50 mL centrifuge tube. Sequential extractions were conducted using 30 mL of distilled water, 0.5 mol L−1 NaHCO3, 0.1 mol L−1 NaOH solution, 0.5 mol L−1 HCl solutions, and 1 mol L−1 HCl solutions. Each extraction involved shaking the sample for 4 h using an overhead shaker. After shaking, the soil suspension from each step was centrifuged for 10 min to separate the supernatant. The final extract was then digested using concentrated H2SO4 and H2O2. The P content in 0.5 mol L−1 NaHCO3, 0.1 mol L−1 NaOH, and concentrated HCl extracts was determined using the molybdate-ascorbic acid method, post-digestion with H2SO4 and potassium persulfate. Total P in the extracts was quantified, and the concentration of organic P (Po) was calculated by subtracting inorganic P (Pi) from the total P in each extract (Tiessen and Moir, 1993).
2.5 DNA extraction and real-time PCR
Soil microbial genomic DNA was extracted from 0.5 g of fresh soil using the Omega soil DNA isolation kit (Omega Biotek Inc., Georgia, USA), following the manufacturer’s instructions. The concentration of extracted DNA was evaluated through agarose gel electrophoresis and quantification with a NanoDrop ND-2000 spectrophotometer (Thermo Scientific, Wilmington, DE, USA). Quantitative PCR (qPCR) was performed using the ABI 7500 Real-Time PCR System (Applied Biosystems, Germany) to amplify the phoC and phoD genes (Walker, 2001). The primer pairs for amplifying the phoC and phoD genes were phoC-A-F1 and phoC-A-R1, and phoD-ALPS-F730 and phoD-ALPS-1101 (Sakurai et al., 2007).
The PCR mixture consisted of 10 μL of Power SYBR® Green PCR Master Mix (Applied Biosystems™, Thermo Fisher Scientific Inc., MA, USA), 0.4 μL of each primer, and 1 μL of the extracted DNA template. The PCR conditions were as follows: an initial denaturation at 95°C for 5 min, followed by 40 cycles of denaturation at 95°C for 15 s, and annealing at 60°C for 30 s. The specificity of the amplification was verified by analyzing the melting curve. To quantify the gene copies, a standard curve was constructed by serial tenfold dilution of a plasmid containing the target gene. The copy numbers of the phoC and phoD genes were calculated based on this standard curve and expressed in units per gram of dry soil. These copy numbers represent the abundance of the phoC and phoD genes.
2.6 Statistical analyses
Statistical analyses were performed using SPSS 20.0 (SPSS, Inc., Chicago, IL, USA). A three-way ANOVA was conducted to assess the effects of N addition level (NL), N addition form (NF), and soil type (SF) on soil physical and chemical properties, plant root biomass, soil phosphatase activity, and the abundance of phoC and phoD genes, as well as soil P fractions. Additionally, an independent samples T-test was utilized to compare differences between the various N treatments and the control group. Pearson correlation analysis was used to explore the relationships between soil P fractions and other variables, including soil physiochemical properties, plant root biomass, phosphatase activity, and the abundance of phoC and phoD genes. All statistical tests were performed with a significance level set at 0.05.
3 Results
3.1 Effects of N addition on soil properties and root biomass
Soil properties responded variably to N addition, dependent on the level and form applied (Table 2 and Figure 1). Low-N treatment generally did not significantly affect soil properties (p > 0.05; Figure 1), whereas high-N treatment significantly altered these characteristics (p < 0.05; Figure 1). Specifically, under high N levels, all four forms of N input [NH4Cl, Ca(NO3)2, NH4NO3, and Urea] significantly reduced the NH4+ and AN content in yellow soil. However, in limestone soil, this significant reduction in NH4+ and AN content was only observed with the addition of NH4NO3 and Urea. There were significant differences in NH4+ and AN contents across different N forms treatments in both soil types, following a consistent pattern: NH4Cl > Ca(NO3)2 > NH4NO3 > Urea. Regarding NO3 content, high NH4Cl and NH4NO3 had a negative effect in both soils. Additionally, soil pH significantly decreased under high NH4Cl, more so than with other N forms. Although N treatments generally had less effect on soil moisture (SM), high Urea addition significantly reduced SM in both soils.
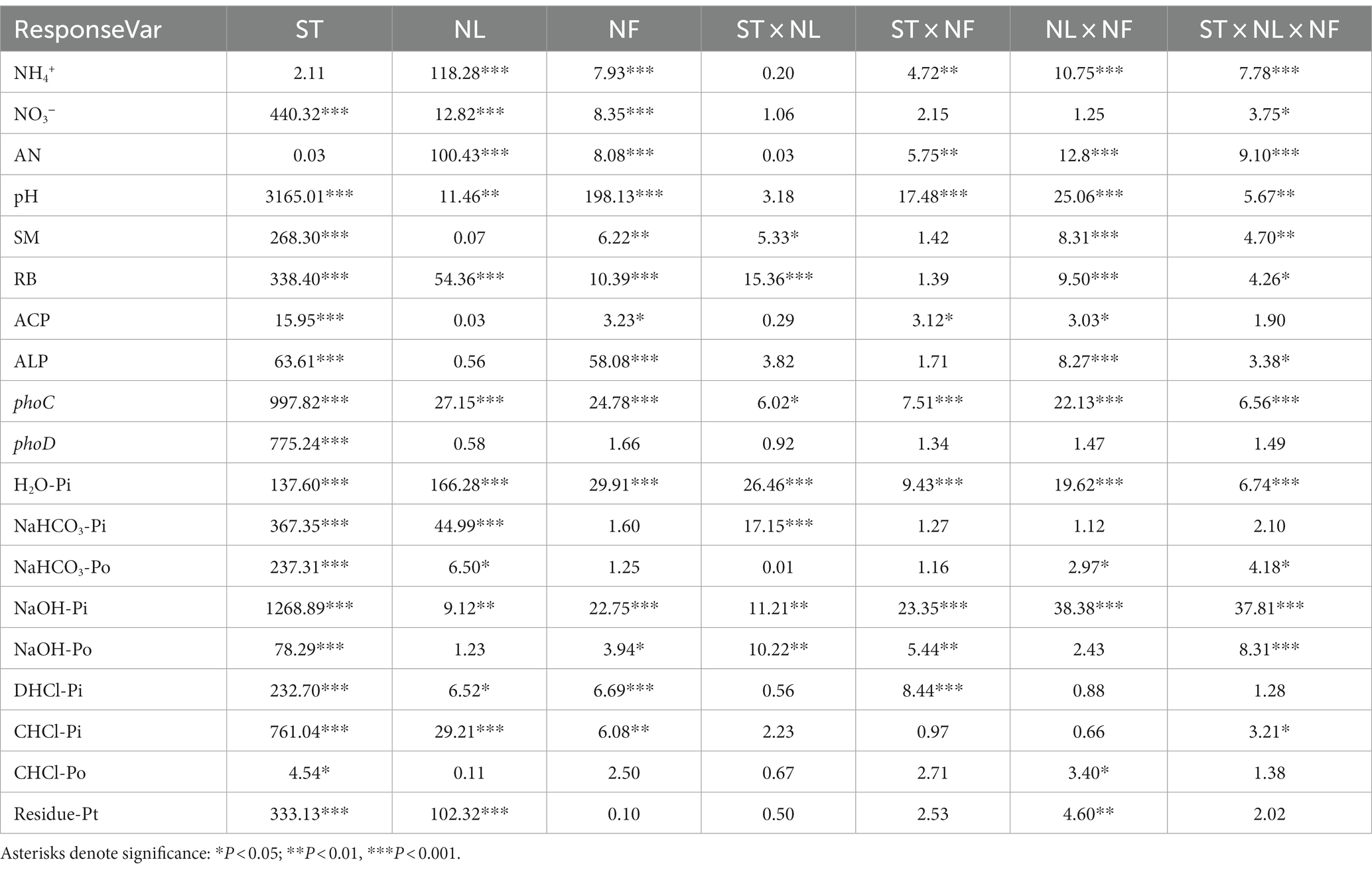
Table 2. Effects of soil type (ST), N addition levels (NL), N addition forms (NF) and their interaction on soil properties (NH4+, NO3−, AN, pH, and SM), phosphatase activity (ACP, ALP), phoC and phoD gene abundance, root biomass (RB) and soil P fractions.
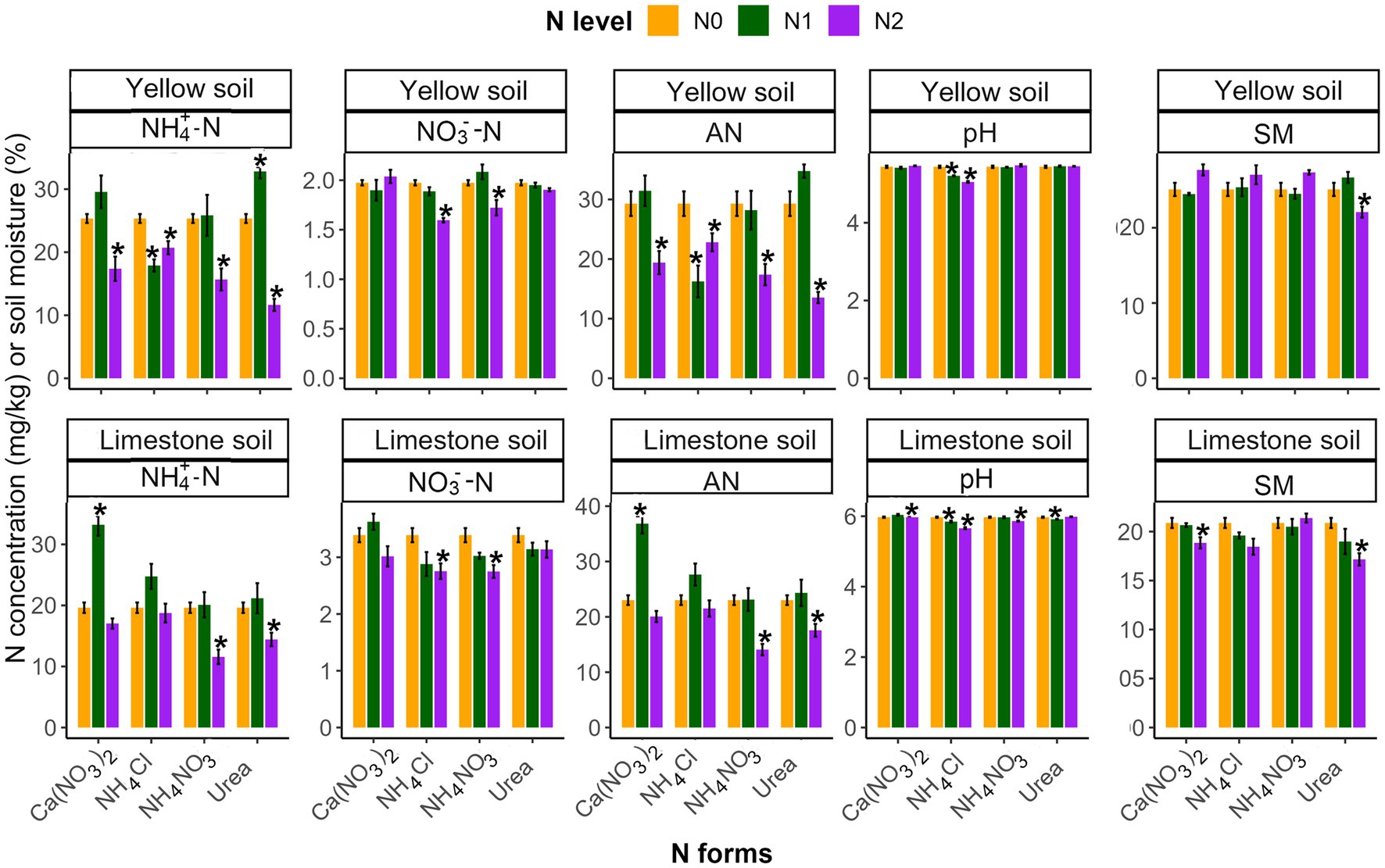
Figure 1. The effects of different N addition levels (N0, 0 mg N kg−1 soil; N1, 50 mg N kg−1 soil; N2, 100 mg N kg−1 soil) and N addition forms [Ca(NO3)2, NH4Cl, NH4NO3 and Urea] on the properties of yellow soil and limestone soil. The error bars represent the standard error (n = 4). “*” Indicates significant differences between the N addition treatment and the control group (p < 0.05).
All N treatments significantly increased plant root biomass (RB) in both soil types (p < 0.05; Figure 2). In our study, Urea resulted in the greatest increase in RB, whereas Ca(NO3)2 contributed to the least increase in this parameter (p < 0.05; Supplementary Table S2). In addition, limestone soil exhibited a higher RB compared to yellow soil, with increased N levels contributing to more significant enhancements in RB (Figure 2 and Supplementary Table S2).
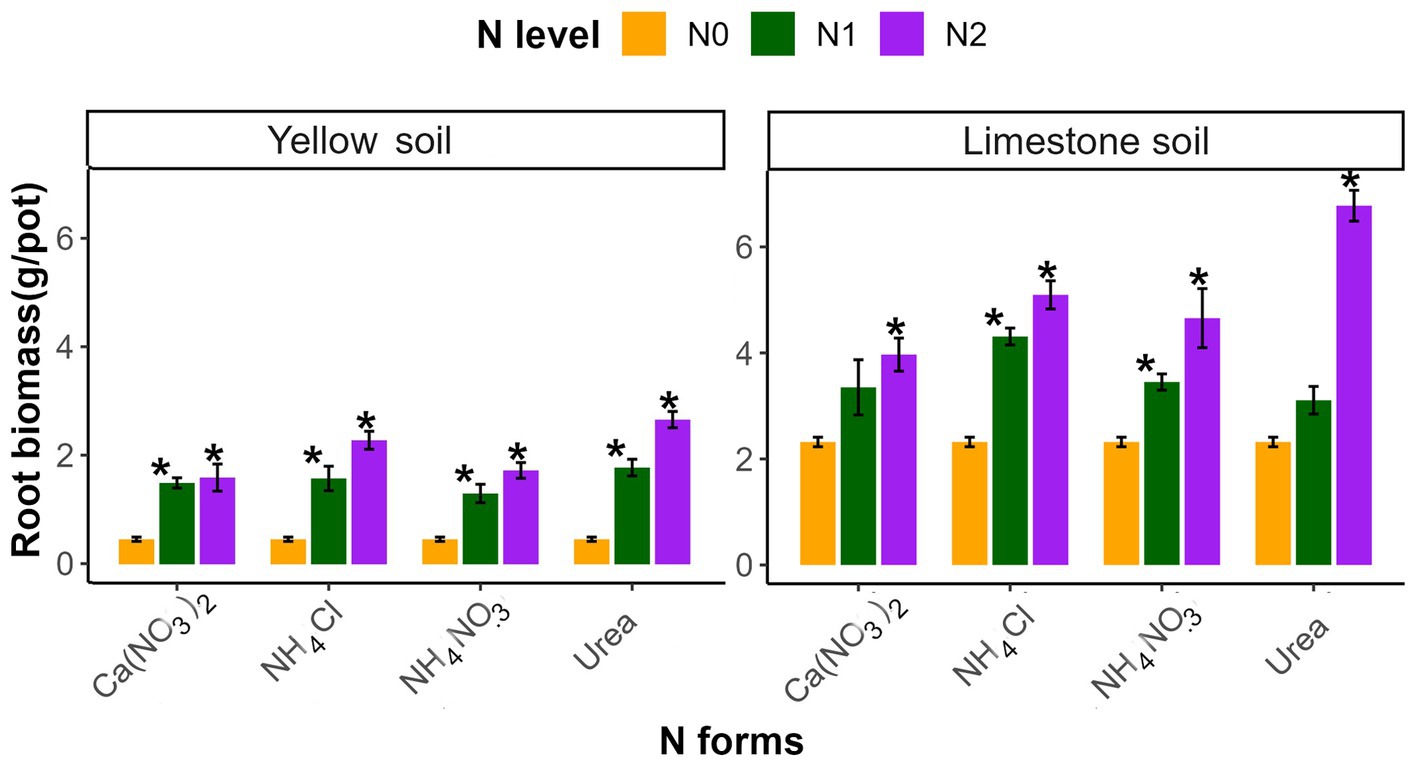
Figure 2. The effects of different N addition levels (N0, 0 mg N kg−1 soil; N1, 50 mg N kg−1 soil; N2, 100 mg N kg−1 soil) and N addition forms (Ca(NO3)2, NH4Cl, NH4NO3 and Urea) on the root biomass of yellow soil and limestone soil. The error bars represent the standard error (n = 4). “*” Indicates significant differences between the N addition treatment and the control group (p < 0.05).
3.2 Effect of N addition on soil P fractions
In both yellow and limestone soils, inorganic P was the predominant form, accounting for 66.55 and 56.78% of total P, respectively (Supplementary Table S1). Additionally, yellow soil exhibited higher contents and proportions of moderately labile P fractions (NaOH-Pi, NaOH-Po) and non-labile P fractions (C.HCl-Pi, C.HCl-Po, and Residual-Pt) compared to limestone soil. However, the content and proportion of NaHCO3-Po and H2O-Pi were lower in yellow soil than in limestone soil (Table S1).
The influence of N addition on soil P fractions varied based on soil type, N level, and form (Table 2 and Figures 3, 4). Generally, high-N additions significantly affected the P fraction in both soils, unlike low-N additions (Figures 3, 4). With the exception of Ca(NO3)2, high N addition significantly increased the H2O-Pi fraction and decreased the NaHCO3-Pi fraction, without affecting the NaHCO3-Po fraction (p < 0.05; Figures 3, 4). Significant differences in the soil H2O-Pi fraction were found among various high-N treatments, with NH4NO3 and Urea showing higher levels than Ca(NO3)2 (Supplementary Table S3). Other P fractions in the two soils, however, exhibited divergent responses to N inputs (Figures 3, 4). In yellow soil, high N negatively affected the C.HCl-Pi, C.HCl-Po, and NaOH-Po fractions, except for C.HCl-Pi fractions with Urea and NaOH-Po fractions with Ca(NO3)2 (Figure 3). Conversely, these P fractions in limestone soil were not significantly impacted. Moreover, at high N levels, there were significant differences between various N forms in C.HCl-Pi and NaOH-Po fractions (p < 0.05; Figure 3). Specifically, the NaOH-Po fraction in Ca(NO3)2 was significantly higher than in other N forms, and the C.HCl-Pi fraction was significantly higher with NH4NO3 and Urea compared to other N forms (Supplementary Table S3).
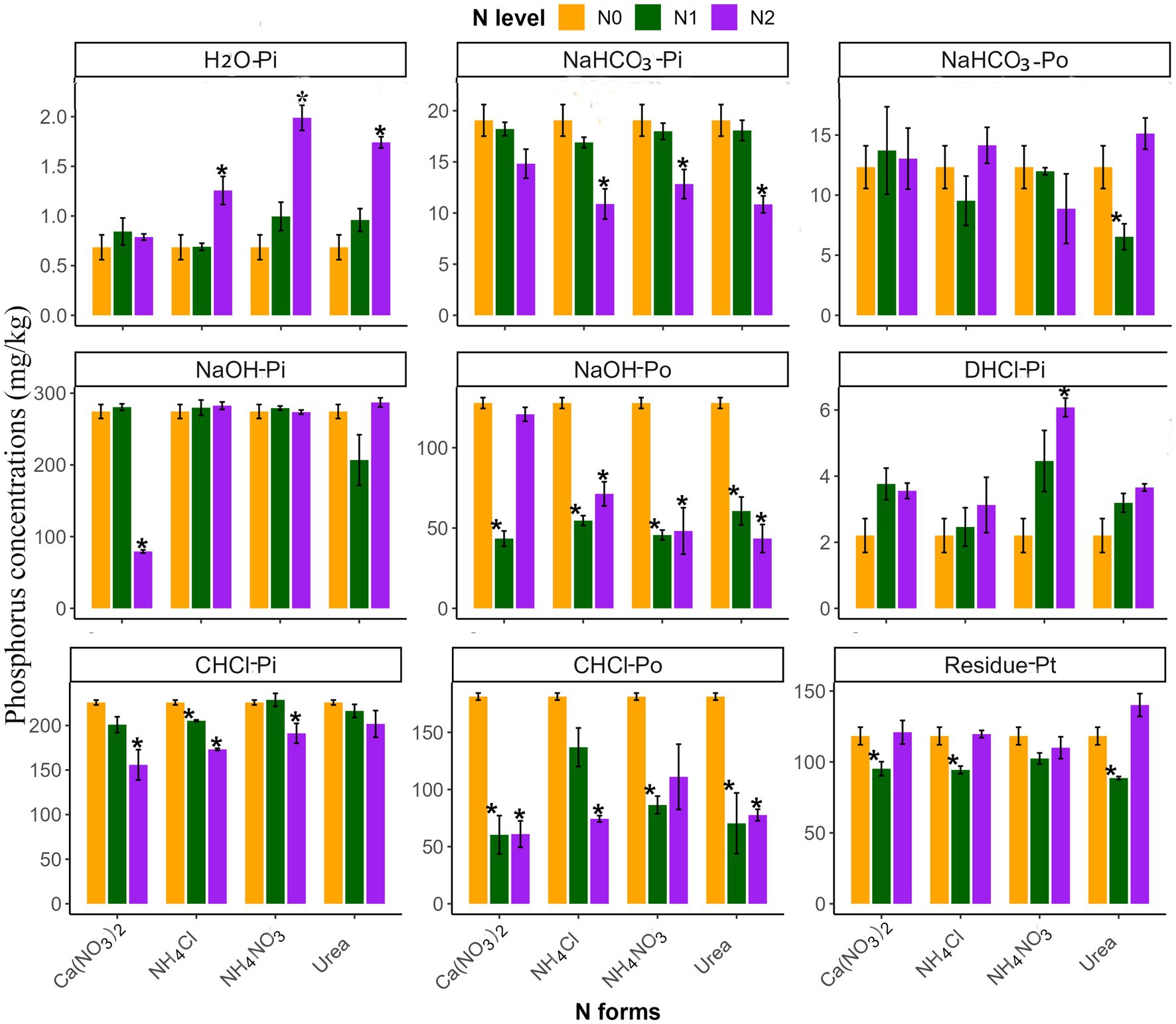
Figure 3. The effects of different N addition levels (N0, 0 mg N kg−1 soil; N1, 50 mg N kg−1 soil; N2, 100 mg N kg−1 soil) and N addition forms (Ca(NO3)2, NH4Cl, NH4NO3 and Urea) on the P fractions concentrations (mg kg−1) in yellow soil. The error bars represent the standard error (n = 4). “*” Indicates significant differences between the N addition treatment and the control group (p < 0.05).
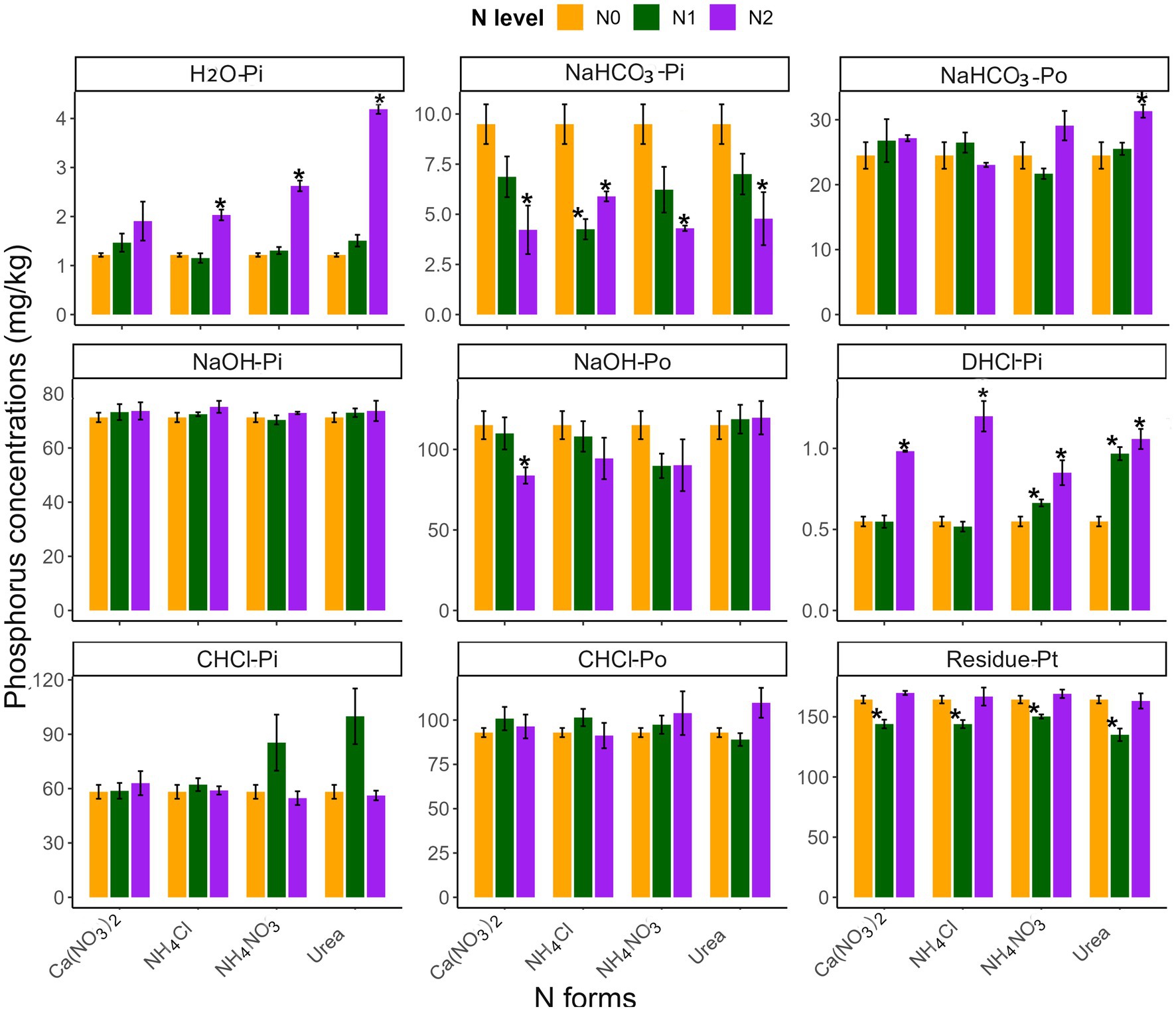
Figure 4. The effects of different N addition levels (N0, 0 mg N kg−1 soil; N1, 50 mg N kg−1 soil; N2, 100 mg N kg−1 soil) and N addition forms [Ca(NO3)2, NH4Cl, NH4NO3 and Urea] on the P fractions concentrations (mg kg−1) in limestone soil. The error bars represent the standard error (n = 4). “*” Indicates significant differences between the N addition treatment and the control group (p < 0.05).
3.3 Effect of N addition on soil phosphatase activity and phoC and phoD gene abundance
In both yellow and limestone soils, the activity of acid phosphatase (ACP) exceeded that of alkaline phosphatase (ALP). While ACP activity generally remained unaffected by N addition, ALP activity was significantly influenced by various N treatments (p < 0.05; Figure 5). The impact of N treatments varied based on soil type, N level, and form (Table 2). The Ca(NO3)2 treatment significantly reduced ALP activity in both soils, regardless of the addition level. Conversely, high levels of the other three N forms significantly increased ALP activity in yellow soil but decreased it in limestone soil, with the exception of NH4Cl (p < 0.05; Figure 5). Notably, the ALP activity with Ca(NO3)2 treatment was significantly lower than that with the other three N forms in both soils (p < 0.05, Figure 5). Moreover, low-level additions of Urea and NH4NO3 significantly increased ALP activity in yellow soil (p < 0.05), but did not significantly affect limestone soil (p > 0.05; Figure 5).
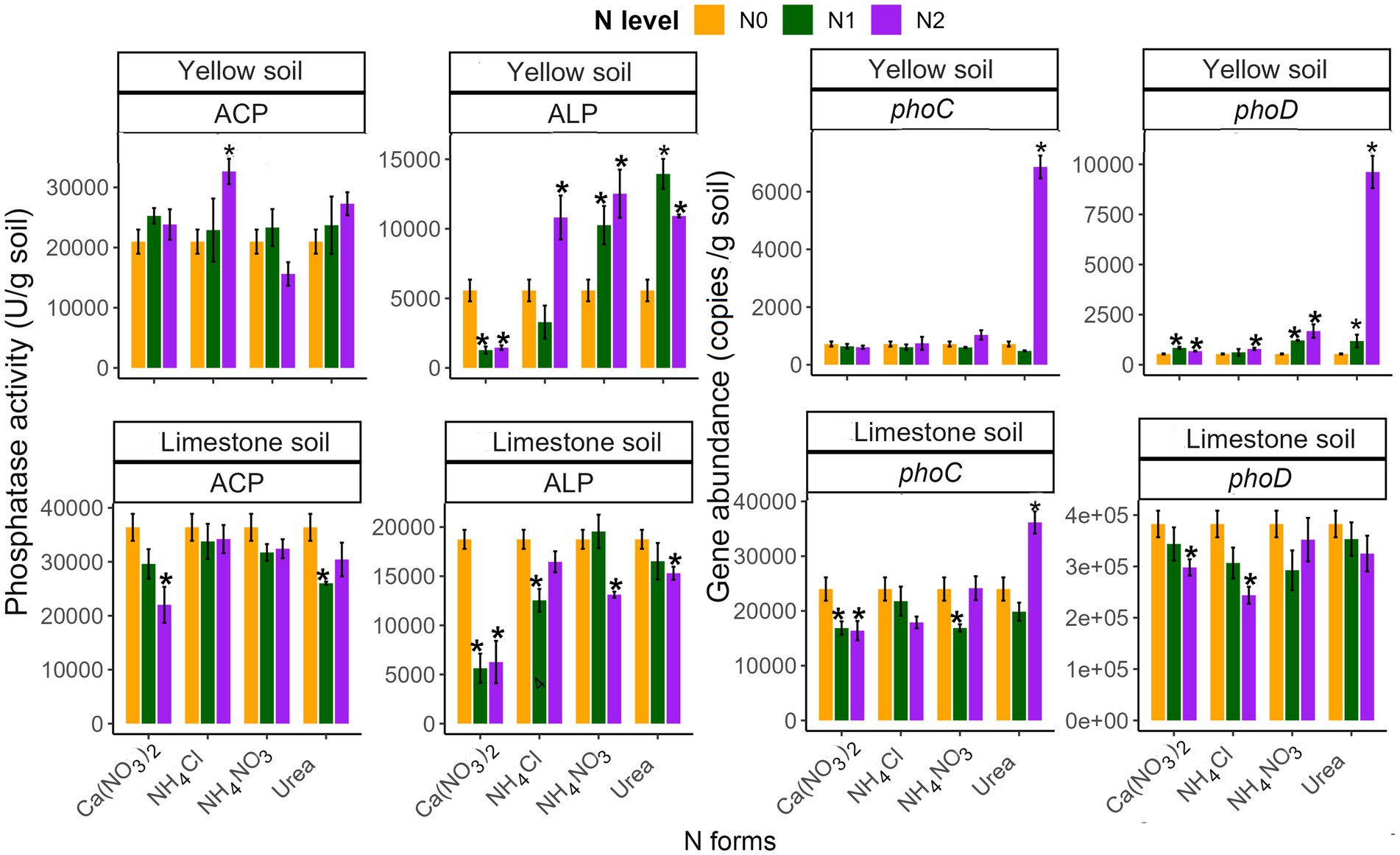
Figure 5. The effects of different N addition levels (N0, 0 mg N kg−1 soil; N1, 50 mg N kg−1 soil; N2, 100 mg N kg−1 soil) and N addition forms [Ca (NO3)2, NH4Cl, NH4NO3 and Urea] on the activities of acid phosphatase (ACP) and alkaline phosphatase (ALP), the abundance of phoC and phoD genes in yellow soil and limestone soil. The error bars represent the standard error (n = 4). “*” Indicates significant differences between the N addition treatment and the control group (p < 0.05).
The abundance of phoC and phoD, in response to N input, varies based on N level, and N form (Figure 5 and Table 2). Notably, high Urea addition significantly increased the phoC abundance in both soils (p < 0.05; Figure 5). On the other hand, Ca(NO3)2 addition only decreased phoC abundance in limestone soil, while other N forms did not notably affect phoC abundance in either soil type (p < 0.05; Figure 5). Furthermore, in both soil types, the phoC abundance resulting from high Urea addition was significantly higher compared to other N additions (Supplementary Table S2). As for the phoD gene abundance in yellow soil, all forms of N addition generally had a positive effect, showing the same order as above (p < 0.05; Figure 5). In contrast, in limestone soil, high NH4Cl and Ca(NO3)2 addition significantly reduced phoD gene abundance, while other N treatments had no significant effect. Additionally, compared to yellow soil, limestone soil exhibited higher phoC and phoD gene abundance (Figure 5 and Table 2).
3.4 Correlation between soil properties and P fractions
Correlation analysis revealed distinct relationships between soil properties and P fractions in yellow and limestone soils. In yellow soil, H2O-Pi exhibited a significant negative correlation with NH4+-N, NO3−-N and AN (p < 0.05; Figure 6A), while it showed a positive correlation with ALP activity, phoC gene abundance, phoD gene abundance, and RB (p < 0.05; Figure 6A). Conversely, NaHCO3-Pi displayed an opposite trend in its correlations with these factors. Additionally, both NaOH-Po and C.HCl-Po were significantly negatively correlated with RB in yellow soil (p < 0.05; Figure 6A). In limestone soil, H2O-Pi was significantly negatively correlated with NH4+-N and AN, and positively correlated with phoC gene abundance and RB (p < 0.05; Figure 6B). Furthermore, NaOH-Pi, D.HCl-Pi, and C.HCl-Po in limestone soil showed significant positive correlations with RB (p < 0.05; Figure 6B).
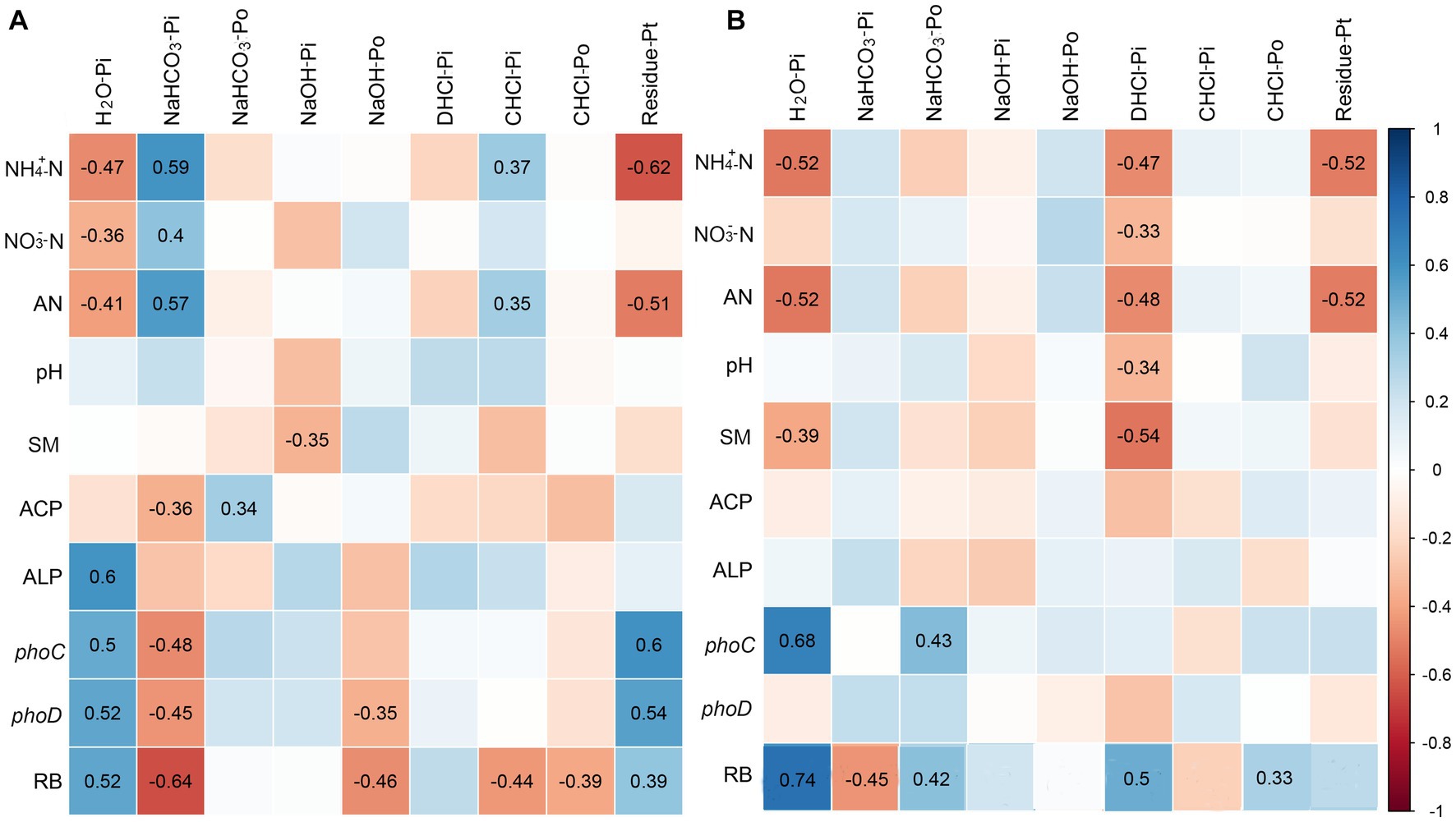
Figure 6. Relationship (Pearson’s) between P fractions of yellow soil (A) and limestone soil (B) and biotic and abiotic factors. Only significant correlation coefficients are marked in the figure (p < 0.05). AN, available nitrogen; ACP, acid phosphatase; ALP, alkaline phosphatase; RB, root biomass; SM, soil water contents.
4 Discussion
4.1 The impact of N input on soil P availability depends on the input level and form of N
N input modifies P fractions by influencing biotic and abiotic factors in the soil P cycle (Sales et al., 2017; Hou et al., 2018; Fan et al., 2019). Based on the availability of plants and organisms, soil P can be categorized into available P (H2O-Pi), labile P fraction (NaHCO3-Pi and NaHCO3-Po), moderately labile P fraction (NaOH-Pi, NaOH-Po and D.HCl-Pi), non-labile P fraction (C.HCl-Pi, C.HCl-Po and residual-Pt), respectively (Hedley et al., 1982; Redel et al., 2019; Mahmood et al., 2021). Notably, available P and labile P are more influenced by human activities than moderately and non-labile P fractions (Chen et al., 2018; Guan et al., 2023). In this study, two distinct soil types were examined: limestone soil and yellow soil. In yellow soil, the fractions of H2O-Pi, NaHCO3-Pi, NaOH-Po, C.HCl-Pi, and C.HCl-Po were significantly affected by N input, while in limestone soil, only H2O-Pi and NaHCO3-Pi fractions were significantly influenced, suggesting a higher sensitivity of yellow soil to N inputs (Figures 3, 4). The variation in soil available P can be attributed to the differences in initial available P concentrations and soil pH between these soil types. A recent meta-analysis reported that pH plays an important role in change of soil P contents (Li et al., 2023). In comparison to limestone soil (available P: 1.22 mg kg−1, pH: 6.01), yellow soil has lower available P and soil pH (available P: 0.68 mg kg−1; pH: 5.46). Prior research has highlighted that soil P dynamics are highly responsive to N inputs in P-limited environments, particularly in acidic soils (pH < 5.5) (Deng et al., 2017). Addition of N significantly affects soil properties, primarily by lowering soil pH and leading to soil acidification (Fan et al., 2019). This change can substantially alter soil phosphorus availability by influencing the chemical and biological reactions that control P migration (Li et al., 2019).
Furthermore, our findings support the first hypothesis that N input significantly alters soil P fractions, with the effect depending on both the N level and form. This aligns with Liu et al. (2021), who found that low-level N inputs in the short term do not significantly affect soil P fractions, while high-level inputs do. This difference might be attributed to the lesser influence of low-level N on biotic and abiotic factors controlling soil P fractions in the short term (Figures 1, 5). Fertilization and land use mainly influence H2O-Pi and NaHCO3-Pi fractions (Tian et al., 2020). Our findings indicated that high N inputs, except for Ca(NO3)2, significantly increased available P and decreased the NaHCO3-Pi fraction in both soils (Figures 3, 4). This result is similar to previous findings in subtropical forests and temperate larch plantations (Fan et al., 2019; Huang et al., 2021). Additionally, in yellow soil, but not limestone soil, N input also reduced the NaOH-Po and C.HCl-Po fractions. The dynamic behavior of soil P, which involves processes like dissolution, adsorption, desorption, precipitation, and transformation (Rawat et al., 2020), implies that N additions could increase the availability of soil P. This potential increase may result from the enhanced desorption of inorganic P and the accelerated mineralization of organic P. This interpretation was supported by the significant correlation between soil available P and ALP activity, and plant root biomass (Figure 6A).
In our study, different forms of nitrogen exhibited varied effects on the available phosphorus, consistently following the order in both yellow soil and limestone soil: NH4NO3 and Urea >NH4Cl > Ca(NO3)2 (Figures 3, 4). This pattern was consistent with Amin’s (2023) research on the efficiency of different N fertilizers in increasing P availability. The differential effects of N forms on soil available P can be attributed to several factors. Firstly, Ca2+, as an alkaline cation, can form low-soluble calcium phosphate with phosphate anions in soil, potentially reducing available P, especially when adding Ca(NO3)2 (Jalali and Jalali, 2016; Deng et al., 2017; Xu et al., 2020). Furthermore, the higher soil pH following Ca(NO3)2 addition could contribute to this phenomenon (Penn and Camberato, 2019). Conversely, NH4Cl application leads to more severe soil acidification due to nitrification, promoting inorganic P desorption (Raza et al., 2019; Mahmood et al., 2021). Differently, in contrast to Ca(NO3)2 and NH4Cl, the addition of Urea, particularly in limestone soil, results in a greater increase in root biomass and phosphatase activity, thereby enhancing the availability of soil P. Similarly, high NH4NO3 treatment significantly increased soil available phosphorus compared to the addition of Ca(NO3)2 and NH4Cl, yet this trend was not mirrored in the root biomass (Figure 2). Considering that this study included only white clover and perennial ryegrass, we speculate that the enhanced soil available phosphorus due to NH4NO3 treatment may be associated with a higher proportion of white clover in the community (unpublished data). Previous research shows that white clover is more efficient than perennial ryegrass at releasing organic ions, which aids in the desorption, chelation, and complexation of iron and aluminum oxides, ultimately improving phosphorus release for plant uptake (Touhami et al., 2020). Considering urea’s affordability and accessibility (Apthorp et al., 1987), using it as the primary N source can improve soil P availability, reduce fertilizer costs in the studied grasslands. Additionally, the role of different N forms should be considered in future research on N deposition, given the global changes in N deposition components, to accurately evaluate their impact on grassland soil P cycling.
4.2 Relationship between changes in soil available P and plant root biomass and phosphatase activity
Our research revealed a clear connection between alterations in soil available P, plant root biomass, and phosphatase activity, aligning with our second hypothesis. These findings illuminate the dynamic interplay among these factors in soil P cycling, particularly under N input. In both yellow and limestone soils, we observed a close link between available P and plant root biomass. This finding is consistent with previous studies in various ecosystems, which highlight the pivotal role of plant roots in soil P dynamics (Fan et al., 2019).
Plant roots are critical in mediating soil P availability, especially following N input. Increased N leads to a greater P demand, consequently stimulating root growth and enhancing carbon allocation towards P acquisition (Zhang et al., 2016; Fan et al., 2018). The resulting increase in root biomass facilitates the release of root exudates, including organic acids and phosphatases, which are instrumental in mobilizing mineral P and promoting phosphate dissolution (Kai et al., 2014; Yang et al., 2019). Our study observed a significant rise in soil available P, particularly H2O-Pi, under N input, which is likely a direct consequence of enhanced P desorption and bioavailability. This observation parallels findings by Yang et al. (2019), who noted similar effects in short-term N addition studies.
Soil phosphatase, pivotal in mineralizing soil organic P, is also influenced by N input (George et al., 2018; Cao et al., 2022; Ma et al., 2023). We found that N input significantly increased ALP activity and the abundance of the phoD gene in yellow soil, while the response was less pronounced for acid phosphatase (ACP) and phoC abundance (Figure 5). This variation might be due to the distinct origins of these enzymes. Microorganisms that harbor the phoD gene tend to increase alkaline phosphatase production under soil P scarcity, a response that is likely exacerbated by N input (Marklein and Houlton, 2012; Nasto et al., 2015; Wang et al., 2021). Conversely, the response of these enzymes to N input in limestone soil differed (Figure 5). This may be related to the fact that the pH in limestone soil is more sensitive to N input, N-induced decreases in soil pH are positively correlated with N-induced changes in soil phosphatase activity (Chen et al., 2020). The significant increase in alkaline phosphatase activity under N input, particularly with Urea and NH4NO3 treatments, underscores the role of these enzymes in mitigating P limitation. This is further supported by the observation that N-induced changes in soil phosphatase activity are positively correlated with alterations in soil P fractions (Figure 6A). This may be due to the Urea input providing microbially available carbon, enhancing microbial activity (Zhang et al., 2020). However, Ca(NO3)2 appeared to inhibit phosphatase activity, likely due to Ca2+ inhibition (Halstead, 1964), suggesting it may not be the optimal N source for enhancing P availability in these soils. The discrepancy in phoD gene abundance and alkaline phosphatase activity trends under different N inputs (Figure 5) could stem from the fact that alkaline phosphatase is encoded not only by phoD but also by phoA and phoX genes (Hu et al., 2018).
Our study highlights the importance of considering the form of N input when assessing its impact on soil P dynamics. While all forms of N input altered alkaline phosphatase activity to some degree, the distinct patterns observed suggest varying effects on the microbial processes involved in P mineralization, necessitating further exploration to fully grasp the implications of different N forms on soil P cycling and plant-microbe interactions.
5 Conclusion
This study investigated the effects of N addition on two typical soil P fractions in the karst grasslands of southwestern China and found that this effect was significantly and strongly modulated by both the form and amount of N added. In particular, compared with Ca(NO3)2 addition, other high-level N sources (especially Urea and NH4NO3) significantly increased available P in both soils, with the order of effects being Urea and NH4NO3 > NH4Cl > Ca(NO3)2. This highlights the considerable role of N form in determining soil P availability. Further analysis revealed a significant correlation between changes in soil available P and factors such as plant root biomass and alkaline phosphatase activity, underscoring the importance of biological factors in the dynamics of soil P in the context of N input. In terms of management implications, we recommend that high levels of Urea and NH4NO3 be prioritized over Ca(NO3)2 in management practices to increase soil P availability and plant utilization. This has important implications for improving soil fertility in the region and supporting sustainable agricultural practices.
Data availability statement
The original contributions presented in the study are included in the article/Supplementary material, further inquiries can be directed to the corresponding author.
Author contributions
JZ: Data curation, Writing – original draft. FY: Data curation, Writing – review & editing. XZ: Writing – review & editing. XG: Writing – review & editing. CC: Writing – review & editing. JC: Funding acquisition, Writing – review & editing.
Funding
The author(s) declare financial support was received for the research, authorship, and/or publication of this article. This work was financially supported by the Guizhou Provincial Science and Technology Projects ([Qian Ke He [2022] Yi Ban040]; QKHPTRC-CXTD [2022] 011), the Guizhou Education Cooperation (Qianjiaoji [2022] 120) and the Guizhou University Projects (Gui Da Ren Ji He [2020] 70).
Conflict of interest
The authors declare that the research was conducted in the absence of any commercial or financial relationships that could be construed as a potential conflict of interest.
Publisher’s note
All claims expressed in this article are solely those of the authors and do not necessarily represent those of their affiliated organizations, or those of the publisher, the editors and the reviewers. Any product that may be evaluated in this article, or claim that may be made by its manufacturer, is not guaranteed or endorsed by the publisher.
Supplementary material
The Supplementary material for this article can be found online at: https://www.frontiersin.org/articles/10.3389/fsufs.2024.1343283/full#supplementary-material
References
Ahmad, M., El-Naggar, A. H., Usman, A. R. A., Abduljabbar, A., Vithanage, M., Elfaki, J., et al. (2018). Aging effects of organic and inorganic fertilizers on phosphorus fractionation in a calcareous Sandy loam soil. Pedosphere 28, 873–883. doi: 10.1016/S1002-0160(17)60363-1
Amin, A. E.-E. A. Z. (2023). Effect of co-applying different nitrogen fertilizers with bone char on enhancing phosphorus release in calcium carbonate-rich soil: an incubation study. J. Soil Sci. Plant Nutr. 23, 1565–1575. doi: 10.1007/s42729-023-01217-3
Apthorp, J., Hedley, M., and Tillman, R. (1987). The effects of nitrogen fertilizer form on the plant availability of phosphate from soil, phosphate rock and mono-calcium phosphate. Fertilizer Res. 12, 269–283. doi: 10.1007/BF01315111
Ashley, K., Cordell, D., and Mavinic, D. (2011). A brief history of phosphorus: from the philosopher's stone to nutrient recovery and reuse. Chemosphere 84, 737–746. doi: 10.1016/J.CHEMOSPHERE.2011.03.001
Bai, Y., and Cotrufo, M. F. (2022). Grassland soil carbon sequestration: current understanding, challenges, and solutions. Science 377, 603–608. doi: 10.1126/science.abo2380
Cao, N., Zhi, M., Zhao, W., Pang, J., Hu, W., Zhou, Z., et al. (2022). Straw retention combined with phosphorus fertilizer promotes soil phosphorus availability by enhancing soil P-related enzymes and the abundance of phoC and phoD genes. Soil Tillage Res. 220:105390. doi: 10.1016/j.still.2022.105390
Chen, H., Chen, M., Li, D., Mao, Q., Zhang, W., and Mo, J. (2018). Responses of soil phosphorus availability to nitrogen addition in a legume and a non-legume plantation. Geoderma 322, 12–18. doi: 10.1016/j.geoderma.2018.02.017
Chen, J., van Groenigen, K. J., Hungate, B. A., Terrer, C., van Groenigen, J. W., Maestre, F. T., et al. (2020). Long-term nitrogen loading alleviates phosphorus limitation in terrestrial ecosystems. Glob. Chang. Biol. 26, 5077–5086. doi: 10.1111/gcb.15218
Cui, H., Fan, M., Wang, Y., Zhang, X., Xu, W., Li, Y., et al. (2022). Impacts of mowing and N addition on soil organic phosphorus mineralization rates in a semi-natural grassland in Northeast China. Plant Soil 482, 7–23. doi: 10.1007/s11104-022-05670-4
Deng, Q., Hui, D., Dennis, S., Reddy, K. C., and Xu, X. (2017). Responses of terrestrial ecosystem phosphorus cycling to nitrogen addition: a meta-analysis. Glob. Ecol. Biogeogr. 26, 713–728. doi: 10.1111/geb.12576
Ding, Y., Geng, Y., Zhou, W., and Li, D. (2023). Habitat-specific environmental factors regulate the spatial variability of biological soil crust microbial communities on the Qinghai-Tibet Plateau. Sci. Total Environ. 901:165937. doi: 10.1016/j.scitotenv.2023.165937
Fan, Y., Lin, F., Yang, L., Zhong, X., and Yang, Y. (2018). Decreased soil organic P fraction associated with ectomycorrhizal fungal activity to meet increased P demand under N application in a subtropical forest ecosystem. Biol. Fertil. Soils 54, 149–161. doi: 10.1007/s00374-017-1251-8
Fan, Y., Zhong, X., Lin, F., Liu, C., Yang, L., Wang, M., et al. (2019). Responses of soil phosphorus fractions after nitrogen addition in a subtropical forest ecosystem: insights from decreased Fe and Al oxides and increased plant roots. Geoderma 337, 246–255. doi: 10.1016/j.geoderma.2018.09.028
Fenton, O., Mellander, P.-E., Daly, K., Wall, D., Jahangir, M., Jordan, P., et al. (2017). Integrated assessment of agricultural nutrient pressures and legacies in karst landscapes. Agric. Ecosyst. Environ. 239, 246–256. doi: 10.1016/j.agee.2017.01.014
Geng, Y., Peng, C., Wang, Z., Huang, S., Zhou, P., and Li, D. (2022). Insights into the spatiotemporal differences in tailings seepage pollution by assessing the diversity and metabolic functions of the soil microbial community. Environ. Pollut. 306:119408. doi: 10.1016/j.envpol.2022.119408
George, T. S., Giles, C. D., Menezes-Blackburn, D., Condron, L. M., and Haygarth, P. M. (2018). Organic phosphorus in the terrestrial environment: a perspective on the state of the art and future priorities. Plant Soil 427, 191–208. doi: 10.1007/s11104-017-3488-2
Guan, Z.-H., Cao, Z., Li, X. G., Kühn, P., Hu, G., Scholten, T., et al. (2023). Effects of winter grazing and N addition on soil phosphorus fractions in an alpine grassland on the Qinghai-Tibet Plateau. Agric. Ecosyst. Environ. 357:108700. doi: 10.1016/j.agee.2023.108700
Halstead, R. L. (1964). Phosphatase activity of soils as influenced by lime and other treatments. Can. J. Soil Sci. 44, 137–144. doi: 10.4141/cjss64-017
Hedley, M. J., Stewart, J. W. B., and Chauhan, B. S. (1982). Changes in inorganic and organic soil phosphorus fractions induced by cultivation practices and by laboratory incubations. Soil Sci. Soc. Am. J. 46, 970–976. doi: 10.2136/sssaj1982.03615995004600050017x
Hou, E., Wen, D., Jiang, L., Luo, X., Kuang, Y., Lu, X., et al. (2021). Latitudinal patterns of terrestrial phosphorus limitation over the globe. Ecol. Lett. 24, 1420–1431. doi: 10.1111/ele.13761
Hou, E., Wen, D., Kuang, Y., Cong, J., Chen, C., He, X., et al. (2018). Soil pH predominantly controls the forms of organic phosphorus in topsoils under natural broadleaved forests along a 2500 km latitudinal gradient. Geoderma 315, 65–74. doi: 10.1016/j.geoderma.2017.11.041
Hu, W., Tan, J., Shi, X., Lock, T. R., Kallenbach, R. L., and Yuan, Z. (2022). Nutrient addition and warming alter the soil phosphorus cycle in grasslands: a global meta-analysis. J. Soils Sediments 22, 2608–2619. doi: 10.1007/s11368-022-03276-y
Hu, Y., Xia, Y., Sun, Q., Liu, K., Chen, X., Ge, T., et al. (2018). Effects of long-term fertilization on phoD-harboring bacterial community in karst soils. Sci. Total Environ. 628-629, 53–63. doi: 10.1016/j.scitotenv.2018.01.314
Huang, J., Zhou, L. H., Liu, S. J., Han, T. F., Hayatu, N. G., Li, D. C., et al. (2021). Vertical distribution of phosphorus fractions and the environmental critical phosphorus level in acidic red soil under long-term fertilizer and lime application in southern China. J. Plant Nutr. Soil Sci. 184, 585–595. doi: 10.1002/jpln.202100098
Jalali, M., and Jalali, M. (2016). Relation between various soil phosphorus extraction methods and sorption parameters in calcareous soils with different texture. Sci. Total Environ. 566-567, 1080–1093. doi: 10.1016/j.scitotenv.2016.05.133
Jing, D., Yan, Y., Ren, T., Lu, J., Wang, X., Chen, J., et al. (2021). Effects of nitrogen application rate on phosphorus transformation in an Alfisol: results from phosphate-oxygen isotope ratios. Appl. Geochem. 134:105094. doi: 10.1016/j.apgeochem.2021.105094
Kai, Y., Jiaojun, Z., Jiacun, G., Lizhong, Y., and Wang, Z. (2014). Changes in soil phosphorus fractions after 9 years of continuous nitrogen addition in a Larix gmelinii plantation. Ann. For. Sci. 72, 435–442. doi: 10.1007/s13595-014-0444-7
Li, Y., Cui, S., Chang, S. X., and Zhang, Q. (2019). Liming effects on soil pH and crop yield depend on lime material type, application method and rate, and crop species: a global meta-analysis. J. Soils Sediments 19, 1393–1406. doi: 10.1007/s11368-018-2120-2
Li, Y., Li, Y., Zhang, Q., Xu, G., Liang, G., Kim, D., et al. (2023). Enhancing soil carbon and nitrogen through grassland conversion from degraded croplands in China: assessing magnitudes and identifying key drivers of phosphorus reduction. Soil Tillage Res. 236:105943. doi: 10.1016/j.still.2023.105943
Li, D., Liu, J., Chen, H., Zheng, L., and Wang, K. (2018). Soil gross nitrogen transformations in responses to land use conversion in a subtropical karst region. J. Environ. Manag. 212, 1–7. doi: 10.1016/j.jenvman.2018.01.084
Liu, Y., Bing, H., Wu, Y., Zhu, H., Tian, X., Wang, Z., et al. (2022). Nitrogen addition promotes soil phosphorus availability in the subalpine forest of eastern Tibetan plateau. J. Soils Sediments 22, 1–11. doi: 10.1007/s11368-021-03064-0
Liu, C., Li, W., Xu, J., Wei, W., and Yan, H. (2021). Response of soil nutrients and stoichiometry to grazing management in alpine grassland on the Qinghai-Tibet plateau. Soil Tillage Res. 206:104822. doi: 10.1016/j.still.2020.104822
Liu, C., Liu, Y., Guo, K., Qiao, X., Zhao, H., Wang, S., et al. (2018). Effects of nitrogen, phosphorus and potassium addition on the productivity of a karst grassland: plant functional group and community perspectives. Ecol. Eng. 117, 84–95. doi: 10.1016/j.ecoleng.2018.04.008
Ma, X., Zhou, Z., Chen, J., Xu, H., Ma, S., Dippold, M. A., et al. (2023). Long-term nitrogen and phosphorus fertilization reveals that phosphorus limitation shapes the microbial community composition and functions in tropical montane forest soil. Sci. Total Environ. 854:158709. doi: 10.1016/j.scitotenv.2022.158709
Mahmood, M., Tian, Y., Ma, Q., Hui, X., Elrys, A. S., Ahmed, W., et al. (2021). Changes in phosphorus fractions in response to long-term nitrogen fertilization in loess plateau of China. Field Crop Res. 270:108207. doi: 10.1016/j.fcr.2021.108207
Marklein, A. R., and Houlton, B. Z. (2012). Nitrogen inputs accelerate phosphorus cycling rates across a wide variety of terrestrial ecosystems. New Phytol. 193, 696–704. doi: 10.1111/j.1469-8137.2011.03967.x
Nannipieri, P., Giagnoni, L., Landi, L., and Renella, G. (2011). “Role of phosphatase enzymes in soil” in Phosphorus in action. eds. E. Bunemann, A. Oberson, and E. Frossard (Berlin, Heidelberg: Springer), 215–243.
Nasto, M. K., Alvarez-Clare, S., Lekberg, Y., Sullivan, B. W., Townsend, A. R., Cleveland, C. C., et al. (2015). Interactions among nitrogen fixation and soil phosphorus acquisition strategies in lowland tropical rain forests. Ecol. Lett. 17, 1282–1289. doi: 10.1111/ele.12335
Penn, C. J., and Camberato, J. J. (2019). A critical review on soil chemical processes that control how soil pH affects phosphorus availability to plants. Agriculture 9:120. doi: 10.3390/agriculture9060120
Penuelas, J., Janssens, I. A., Ciais, P., Obersteiner, M., and Sardans, J. (2020). Anthropogenic global shifts in biospheric N and P concentrations and ratios and their impacts on biodiversity, ecosystem productivity, food security, and human health. Glob. Chang. Biol. 26, 1962–1985. doi: 10.1111/gcb.14981
Piao, H., Wu, Y., Hong, Y., and Yuan, Z. (2000). Soil-released carbon dioxide from microbial biomass carbon in the cultivated soils of karst areas of Southwest China. Biol. Fertil. Soils 31, 422–426. doi: 10.1007/s003749900189
Rawat, P., Das, S., Shankhdhar, D., and Shankhdhar, S. C. (2020). Phosphate-solubilizing microorganisms: mechanism and their role in phosphate Solubilization and uptake. J. Soil Sci. Plant Nutr. 21, 49–68. doi: 10.1007/s42729-020-00342-7
Raza, S., Chen, Z., Ahmed, M., Afzal, M. R., Aziz, T., and Zhou, J. (2019). Dicyandiamide application improved nitrogen use efficiency and decreased nitrogen losses in wheat-maize crop rotation in loess plateau. Arch. Agron. Soil Sci. 65, 450–464. doi: 10.1080/03650340.2018.1506584
Redel, Y., Staunton, S., Durán, P., Gianfreda, L., Rumpel, C., and de La Luz Mora, M. (2019). Fertilizer P uptake determined by soil P fractionation and phosphatase activity. J. Soil Sci. Plant Nutr. 19, 166–174. doi: 10.1007/s42729-019-00024-z
Sakurai, M., Wasaki, J., Tomizawa, Y., Shinano, T., and Osaki, M. (2007). Analysis of bacterial communities on alkaline phosphatase genes in soil supplied with organic matter. Soil Sci. Plant Nutr. 54, 62–71. doi: 10.1111/j.1747-0765.2007.00210.x
Sales, M. V., Aleixo, S., Gama-Rodrigues, A. C., and Gama-Rodrigues, E. F. (2017). Structural equation modeling for the estimation of interconnections between the P cycle and soil properties. Nutr. Cycl. Agroecosyst. 109, 193–207. doi: 10.1007/s10705-017-9879-1
Sattari, S., Bouwman, A., Martinez Rodríguez, R., Beusen, A., and Van Ittersum, M. (2016). Negative global phosphorus budgets challenge sustainable intensification of grasslands. Nat. Commun. 7:10696. doi: 10.1038/ncomms10696
Tan, H., Barret, M., Mooij, M. J., Rice, O., Morrissey, J. P., Dobson, A., et al. (2013). Long-term phosphorus fertilisation increased the diversity of the total bacterial community and the phoD phosphorus mineraliser group in pasture soils. Biol. Fertil. Soils 49, 661–672. doi: 10.1007/s00374-012-0755-5
Tian, H., Xu, R., Pan, S., Yao, Y., Bian, Z., Cai, W. J., et al. (2020). Long-term trajectory of nitrogen loading and delivery from Mississippi River basin to the Gulf of Mexico. Glob. Biogeochem. Cycles 34:e2019GB006475. doi: 10.1029/2019GB006475
Tiessen, H., and Moir, J. O. (1993). Characterization of available P by sequential extraction. Soil sampling and methods of analysis. 7, 225–229.
Touhami, D., McDowell, R. W., and Condron, L. M. (2020). Role of organic anions and phosphatase enzymes in phosphorus acquisition in the rhizosphres of legumes and grasses grown in a low phosphorus pasture soil. Plan. Theory 9:1185. doi: 10.3390/plants9091185
Van Lenteren, J. E., and Woets, J. V. (1988). Biological and integrated pest control in greenhouses. Annu. Rev. Entomol. 33, 239–269. doi: 10.1007/0-306-47585-5_22
Walker, N. J. (2001). Real-time and quantitative PCR: applications to mechanism-based toxicology. J. Biochem. Mol. Toxicol. 15, 121–127. doi: 10.1002/jbt.8
Wang, Z., Tao, T., Wang, H., Chen, J., Small, G. E., Johnson, D., et al. (2023). Forms of nitrogen inputs regulate the intensity of soil acidification. Glob. Chang. Biol. 29, 4044–4055. doi: 10.1111/gcb.16746
Wang, C., Xue, L., and Jiao, R. (2021). Soil phosphorus fractions, phosphatase activity, and the abundance of phoC and phoD genes vary with planting density in subtropical Chinese fir plantations. Soil Tillage Res. 209:104946. doi: 10.1016/j.still.2021.104946
Wang, R., Yang, J., Liu, H., Sardans, J., Zhang, Y., Wang, X., et al. (2022). Nitrogen enrichment buffers phosphorus limitation by mobilizing mineral-bound soil phosphorus in grasslands. Ecology 103:e3616. doi: 10.1002/ecy.3616
Wen, L., Li, D., Yang, L., Luo, P., Chen, H., Xiao, K., et al. (2016). Rapid recuperation of soil nitrogen following agricultural abandonment in a karst area, Southwest China. Biogeochemistry 129, 341–354. doi: 10.1007/s10533-016-0235-3
Wu, L., Wang, S., Bai, X., Tian, Y., Luo, G., Wang, J., et al. (2020). Climate change weakens the positive effect of human activities on karst vegetation productivity restoration in southern China. Ecol. Indic. 115:106392. doi: 10.1016/j.ecolind.2020.106392
Xiao, D., Xiao, L., Che, R., Tan, Y., Liu, X., Yang, R., et al. (2020). Phosphorus but not nitrogen addition significantly changes diazotroph diversity and community composition in typical karst grassland soil. Agric. Ecosyst. Environ. 301:106987. doi: 10.1016/j.agee.2020.106987
Xu, X., Mao, X., Zwieten, L. V., Niazi, N. K., and Wang, H. (2020). Wetting-drying cycles during a rice-wheat crop rotation rapidly (im) mobilize recalcitrant soil phosphorus. J. Soils Sediments 20, 3921–3930. doi: 10.1007/s11368-020-02712-1
Yang, C., Xia, L., Zeng, Y., Chen, Y., and Zhang, S. (2023). Hexaploid Salix rehderiana is more suitable for remediating lead contamination than diploids, especially male plants. Chemosphere 333:138902. doi: 10.1016/j.chemosphere.2023.138902
Yang, L., Zhijie, P., Yuanzhen, L., Yanyu, X., Decheng, L., and Yusheng, Y. Y. (2019). Evaluating P availability influenced by warming and N deposition in a subtropical forest soil: a bioassay mesocosm experiment. Plant Soil 444, 87–99. doi: 10.1007/s11104-019-04246-z
Yokoyama, D., Imai, N., and Kitayama, K. (2017). Effects of nitrogen and phosphorus fertilization on the activities of four different classes of fine-root and soil phosphatases in Bornean tropical rain forests. Plant Soil 416, 463–476. doi: 10.1007/s11104-017-3225-x
Zhang, T., Qin, M., Wei, C., Li, D., Lu, X., and Zhang, L. (2020). Suspended particles phoD alkaline phosphatase gene diversity in large shallow eutrophic Lake Taihu. Sci. Total Environ. 728:138615. doi: 10.1016/j.scitotenv.2020.138615
Zhang, L. F., and Shenglei,. (2020). Effects of nitrogen deposition and increased precipitation on soil phosphorus dynamics in a temperate forest. Geoderma 380:114650. doi: 10.1016/j.geoderma.2020.114650
Zhang, H., Shi, L., Wen, D., and Yu, K. (2016). Soil potential labile but not occluded phosphorus forms increase with forest succession. Biol. Fertil. Soils 52, 41–51. doi: 10.1007/s00374-015-1053-9
Zhang, Y., Wang, H., Zhao, Y., Wang, J., and Chen, S. (2004). Causes and control countermeasures of main pests and diseases in artificial grassland in Moqi, Inner Mongolia. Grassland Res. 21:3. doi: 10.3969/j.issn.1001-0629.2004.07.015
Zhang, W., Zhang, L., and Deng, J. (2021). Comparison of retention and output of sulfur in limestone soil and yellow soil and their responses to acid deposition in a small karst catchment of Guizhou Province, Southwest China. Environ. Sci. Pollut. Res. 28, 60993–61007. doi: 10.1007/s11356-021-15039-2
Keywords: phosphorus fractions, nitrogen input, nitrogen form, phosphatase activity, phoC, phoD
Citation: Zhou J, Yang F, Zhao X, Gu X, Chen C and Chen J (2024) Influences of nitrogen input forms and levels on phosphorus availability in karst grassland soils. Front. Sustain. Food Syst. 8:1343283. doi: 10.3389/fsufs.2024.1343283
Edited by:
Yuan Li, Lanzhou University, ChinaReviewed by:
Hong Xiao, Gansu Agricultural University, ChinaPhesheya Dlamini, University of Limpopo, South Africa
Copyright © 2024 Zhou, Yang, Zhao, Gu, Chen and Chen. This is an open-access article distributed under the terms of the Creative Commons Attribution License (CC BY). The use, distribution or reproduction in other forums is permitted, provided the original author(s) and the copyright owner(s) are credited and that the original publication in this journal is cited, in accordance with accepted academic practice. No use, distribution or reproduction is permitted which does not comply with these terms.
*Correspondence: Jihui Chen, amhjaGVuM0BnenUuZWR1LmNu