- 1Institute for Global Food Security, School of Biological Sciences, Queen’s University Belfast, Belfast, United Kingdom
- 2Agri-Food and Biosciences Institute, Belfast, United Kingdom
- 3Northern Ireland Environment Agency, Lisburn, United Kingdom
- 4School of Biological Sciences, University of Aberdeen, Aberdeen, United Kingdom
Per- and polyfluoroalkyl substances (PFAS) are a group of persistent organic pollutants which pose significant risks to human health and the environment. This article comprehensively examines the implications of new legislation concerning PFAS for food sustainability. The current legislative frameworks governing PFAS in food production and distribution are explored, highlighting the need for robust mitigation strategies to safeguard food safety and environmental integrity. It delves into the challenges posed by the legislation, raising questions about the balance between environmental protection and the sustainability of the food system. It provides a review of the state-of-the-art analytical methods for PFAS detection and quantification in water and food matrices. Their advantages and limitations are discussed, offering valuable insights for researchers in the field. In addition, a range of mitigation strategies to combat PFAS contamination in the food supply chain are explored. By collating current knowledge on PFAS contamination in sustainable food systems, this article aims to provide a comprehensive resource for researchers, policymakers, and practitioners striving to ensure the safety and sustainability of our global food supply. The integration of legislative insights, advanced analytical techniques, and practical mitigation approaches offers a holistic perspective on managing PFAS-related challenges in the context of sustainable food systems.
1 Introduction
So-called “forever chemicals” are pollutants of rising concern due to the thriving industry of plastic-associated substances. Highly persistent in the environment and human body due to the strength and stability of their unique chemistry, their extreme difficulty to degrade in the environment has led them to be a major outlet of contamination to date (HBM4EU, 2022; United Nations Environment Programme, 2023). Forever chemicals have been widely used since the 1940s in various industrial and consumer products including firefighting foams, cleaning agents, non-stick cookware, water-repellent clothing, additives in paints, and food packaging, due to their resistance to heat, oil, and water (Buck et al., 2011; ECHA, 2022).
Per- and polyfluoroalkyl substances (PFAS) constitute part of these man-made chemicals of increasing global concern. They are technically defined as “any substance that contains at least one fully fluorinated methyl or methylene carbon atom (without any H/Cl/Br/I attached to it)” (OECD, 2021, p. 7). This means that any chemical with a minimum of one perfluorinated methyl (–CF3) or methylene (–CF2–) group is classified as a PFAS. This group of chemicals can be synthesized by two processes: electrochemical fluorination (ECF) or fluorotelomerisation (Evich et al., 2022), resulting in a large and complex class of thousands of compounds with highly diverse functional groups. Table 1 illustrates this collection with a comprehensive but not exhaustive summary of PFAS groups, their chemical structural characteristics, acronyms and examples. It is important to note that more of these groups are being produced each time one gets banned (Hammel et al., 2022).
PFAS have been classified as ubiquitous, persistent, bioaccumulative and toxic, posing a massive concern to regulators worldwide (Environment Agency, 2021). These highly persistent organic compounds can accumulate in the environment, particularly in water bodies, and subsequently in the tissues of aquatic organisms that enter the food chain (Houde et al., 2011). This bioaccumulation can pose significant health risks to both animals and humans who consume contaminated drinking water or food products, including potential links to cancer, hormone disruption, and immune system effects (Steenland et al., 2010). It is estimated that all biota on the planet, including humans, already has a PFAS body burden (Ingelido et al., 2018). Biomonitoring studies are currently underway globally to generate representative evidence of PFAS chemical exposure in the human population (EFSA CONTAM Panel et al., 2020; ATSDR, 2021).
An investigation conducted by the International Pollutants Elimination Network carried out in 17 countries across Asia, Africa, Europe, Latin America and the Caribbean found PFAS contents in 54% of sampled single-use food packaging and tableware (Straková et al., 2023). This highlights the global exposure of human population to PFAS from packaging and contaminated food. Similar findings have also been observed across a range of more site-specific studies. For example, PFAS pollution was reported to threaten water quality in North and Southeast Asia (Liu et al., 2021; Tang et al., 2023). A study on pregnant women in Shanghai, China, found all participants to contain PFAS in their blood at different concentrations (Tian et al., 2018). Further, PFAS were also found to be ubiquitous in infants in West Africa (Sørensen et al., 2023). The global threat posed by these pervasive chemicals is exacerbated by their association with multiple exposure pathways.
Two of the oldest and most commonly employed PFAS, perfluorooctane sulfonic acid (PFOS) and perfluorooctanoic acid (PFOA), have been listed in the annexes of the Stockholm Convention on Persistent Organic Pollutants (POPs) since 2009 and 2019, respectively (European Union Reference Laboratory for Halogenated POPs in Feed and Food, 2022). PFOS is listed under Annex B aiming for the restriction of production and use of chemicals, whereas PFOA is listed under Annex A which aims to eliminate production and uses completely (Stockholm Convention, 2019). Additionally, PFOS is on the List of Chemicals for Priority Action associated with the Convention for the Protection of the Marine Environment of the North-East Atlantic (OSPAR). Several of PFOS precursors are also included in the OSPAR List of Substances of Possible Concern (OSPAR Commission, 2013). The recommendation by OSPAR is to take proactive steps in studying various PFAS in the marine environment and staying alert for new findings in their designated area, while focusing on evaluating eco-friendly alternatives to replace these substances.
In October 2020 the European Commission published a Chemicals Strategy for Sustainability Toward a Toxic-Free Environment aiming to implement tangible actions to increase the level of protection of public human health and the environment from hazardous chemicals (European Commission, 2020). The strategy sets the goal of transforming the EU industry via the production and consequent use of safe and sustainable chemicals. In doing so, the criteria for essential uses of certain substances will be redefined “to ensure that the most harmful chemicals are only allowed if their use is necessary for health, safety or is critical for the functioning of society and if there are no alternatives that are acceptable from the standpoint of environment and health” (European Commission, 2020, p. 10).
2 PFAS REACH restriction proposal
International efforts are being made to restrict the use of PFAS in order to reduce their release into the environment and subsequent transfer into the food chain. A restriction proposal under the Regulation for Registration, Evaluation, Authorization and Restriction of Chemicals (REACH) (European Parliament and Council of the European Union, 2006) was prepared and made available to the European Chemicals Agency (ECHA) at the beginning of 2023 by five EU authorities (Denmark, Germany, the Netherlands, Norway and Sweden). The restriction proposal, which is under consideration, would apply to any product containing PFAS, including food and food contact materials. The basis for this proposal is the concern in relation to the properties of PFAS chemicals, in particular their very high persistence and mobility within the environment, which could progressively lead to harmful exposure for biota and humans via soil, water, air and the pertinent food chains. Other properties of concern are their long-range transport potential, bioaccumulation, and endocrine activity and toxicity. Figure 1 shows the lifecycle of the emissions from PFAS from their origin at production sites to subsequent uses and product end-life disposal and waste recycling, including the exposure pathways to environmental and human health. PFAS also have been found in recycled bedding materials used in poultry farms, whereby these chemicals have subsequently made their way into the eggs and tissues of chickens entering the food market (Fernandes et al., 2023). Consequently, the presence of PFAS in products recovered from former waste could deeply affect safe and sustainable feed and food production in a circular economy approach.
3 European policy on PFAS in food, feed, and drinking water
According to the risk assessment carried out by the European Food Safety Authority (EFSA) on the human health risks associated with the presence of perfluoroalkyl contaminants in foodstuffs, the tolerable weekly intake for the sum of PFAS, represented by PFOS, PFOA, perfluorononanoic acid (PFNA) and perfluorohexane sulfonic acid (PFHxS), is 4.4 ng/kg body weight (EFSA CONTAM Panel et al., 2020). It is of concern that the exposure to these chemicals in certain parts of Europe exceeds this level (EFSA CONTAM Panel et al., 2020). Regulation (EU) 2023/915 sets separate maximum levels for PFOS, PFOA, PFNA, and PFHxS, and the sum of the four PFAS in animal and fish meat (European Commission and Directorate-General for Health and Food Safety, 2023). It has also been recommended to monitor other PFAS with different alkyl chains in a broad range of foodstuffs including fruits, vegetables, cereals, food for infants and young children, wine, beer and non-alcoholic drinks, among others (European Commission and Directorate-General for Health and Food Safety, 2022b). Table 2 showcases a list of over 20 PFAS compounds structurally similar to the identified significant PFOS, PFOA, PFNA, and PFHxS recommended for monitoring by the EU member states. In addition, arising PFAS substitutes need to be taken into account such as DONA, GenX, F-53B, Capstone A, and Capstone B (European Union Reference Laboratory for Halogenated POPs in Feed and Food, 2022).
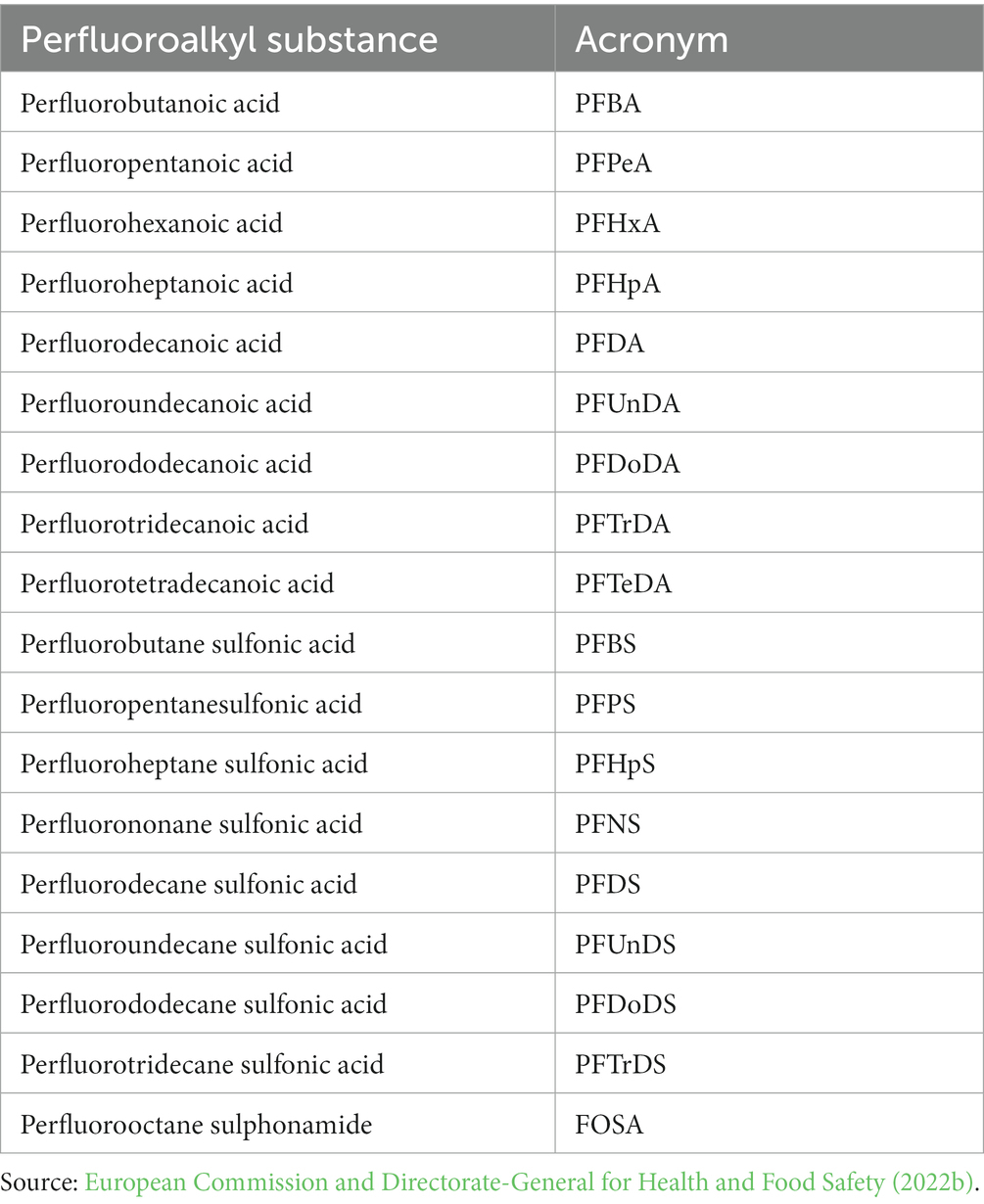
Table 2. PFAS compounds similar to PFOS, PFOA, PFNA, and PFHxS with high occurrence in food, drinking water and human serum.
The sampling and analytical methods for the official control of perfluoroalkyl substances in food are laid down in Regulation (EU) 2022/1428 (European Commission and Directorate-General for Health and Food Safety, 2022a). Target limits of quantification (LOQs) are displayed in Table 3 (European Commission and Directorate-General for Health and Food Safety, 2022b).
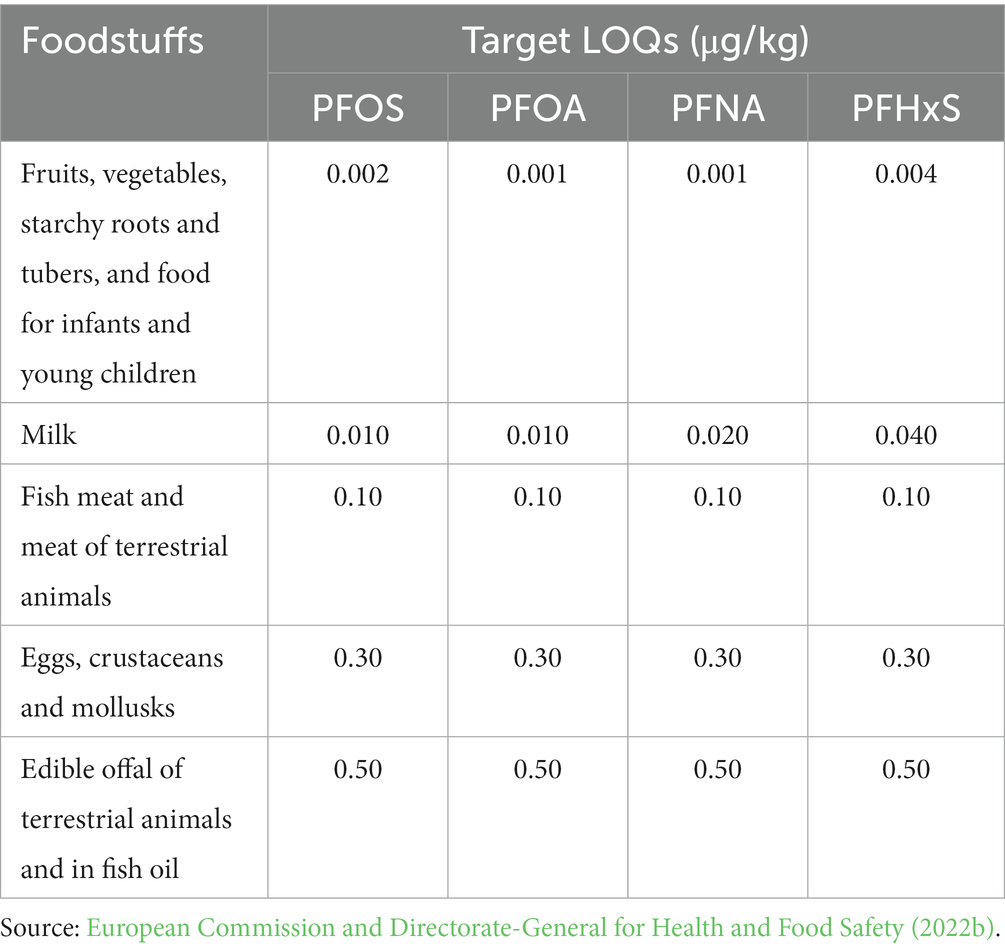
Table 3. Targeted limits of quantification for the monitoring of the four main PFAS compounds in food.
The maximum regulatory levels proposed by the European Commission are of concern as the contamination of food products of animal origin may be a result of bioaccumulation of existing PFAS levels in feed consumed by those animals (European Commission and Directorate-General for Health and Food Safety, 2022c). In January 2023, Danish organic eggs from large commercial farms around Denmark were found to be contaminated with PFAS as a result of contamination in fishmeal contained in chicken feed (DTU National Food Institute, 2023). Subsequent exposure to children is of high concern, as the consumption of only 2.5 organic eggs per week would exceed the tolerable weekly intake proposed by EFSA. Another concern is associated with the sensitivity of current methods of analysis of PFAS levels in feed. Therefore, it is difficult to establish the maximum PFAS levels in feed which would not lead to the exceedance of maximum levels in food. Although it has been acknowledged that present analytical methods require further development for setting these values, there are bigger challenges associated with the management of risks posed by feed. There exists a series of variables that have not been studied in detail which are linked to the uptake of PFAS in feed materials or products. Firstly, there may be some variation in PFAS levels in specific feed materials due to species, origin, season or production method. Secondly, variable amounts may be transferred from feed to food producing animals and their products. Thirdly, variable sensitivity of animals to PFAS and the duration of the contamination in their body. Finally, there is a lack of specific knowledge of many of the PFAS substances. Until more scientific evidence is available on these issues, the solution is to reduce or eliminate the sources of contamination, so feed does not represent a danger to human, animal health or the environment.
PFAS contamination is ubiquitous and as such, it also affects drinking water to a large extent. Not only that, but water is also used and consumed in food production as well as manufacturing and home cooking. Directive (EU) 2020/2184 on the quality of water intended for human consumption set maximum levels for PFAS in drinking water, although these parametric values would not be enforced until the year 2026 (European Parliament and Council of the European Union, 2020). The maximum level for the sum of a subset of 20 PFAS of concern (see Table 2 excluding PFTeDA and FOSA) is 0.1 μg/L, whereas the limit for the totality of PFAS present is 0.5 μg/L. By 2024, the European Commission shall establish technical guidelines with respect to sampling frequency, detection limits and analytical methods. As mentioned above, there is an absence of regulatory levels in force in both feed and drinking water to date. Nonetheless, monitoring of perfluoroalkyl substances using reliable methods of analysis should be the focus of food business operators to ensure food safety in the years to come.
In low-income countries, the regulation and monitoring of contaminants in food may be limited when compared to more affluent states. Factors such as insufficient infrastructure, lack of resources or enforcement of standards, and competing priorities may contribute to challenges in adequately addressing PFAS contamination. In general, global standards for drinking water lack universal acceptance, and only in the USA and EU are water specifications considered mandatory (Whitehead, 2020). In the EU, there is a collective standard, but individual member states can add restrictions without weakening it. In the USA, local authorities can enhance the national standard. Elsewhere, guidelines are non-enforceable with varying targets, depending on local conditions. Water quality specifications differ widely between regions and countries, reflecting unique concerns and issues. In the case of PFAS, the USA proposed enforceable Maximum Contaminant Levels for six PFAS (US EPA, 2023), the EU updated safety standards under the revised Drinking Water Directive, and the UK follows a precautionary approach with no statutory standards but tiered guideline values (DWI, 2022). Contrary to this, certain parts of Asia are still in the early stages of PFAS awareness and regulation (Baluyot et al., 2021). International organizations, non-governmental organizations, and research institutions may play a role in supporting low-income countries in assessing and mitigating the impact of PFAS on food safety, taking into consideration that many Western guidelines may not be totally applicable across countries in the Middle East and Asia due to differences in diets, as well as the ways that food products and drinking water are sourced (Baluyot et al., 2021).
4 PFAS analytical methods
As has been made evident above, it is crucial to monitor and address PFAS contamination at all points in the PFAS emissions lifecycle, due to the widespread nature of these chemicals. The standard analytical methods, such as ISO 21675 (2019), US EPA 533 (2019), US EPA 537.1 (2020), US EPA Draft Method 1,633 (2021), ASTM D7979-19 or DIN 38407–42 (2011) (Winchell et al., 2021; Nahar et al., 2023; Ogunbiyi et al., 2023), used for PFAS testing are based on chromatographic techniques coupled with mass spectrometry (Getzinger and Ferguson, 2021; Ganesan et al., 2022). (Ultra) High performance liquid chromatography [(U)HPLC] coupled to low resolution, high resolution or tandem mass spectrometry (MS/MS) is the recommended method by the European Union Reference Laboratory for halogenated POPs in feed and food for PFAS analysis (European Union Reference Laboratory for Halogenated POPs in Feed and Food, 2022). The suggested instrumentation consists of a C18 LC column packed with solid phase particles, and a mass spectrometer capable of electrospray ionization in the negative ion mode. In regard to sample preparation, the sample material needs to be homogenized carefully to not introduce secondary contamination, followed by PFAS extraction, and subsequent (U)HPLC–MS analysis. No AOAC International official method for PFAS in foodstuff has been published yet, leading to disparities in result consistency across commercial testing laboratories covering PFOS, PFOA, PFNA, and PFHxS analysis (Delatour et al., 2023). However, at the time of writing, an AOAC integrated project was underway to define Standard Method Performance Requirements for PFAS in foods (AOAC International, 2022). Additionally, US EPA Method 1,633 is under draft for the determination of PFAS in aqueous, solid and tissue samples. The results of these projects would contribute to the development of analytical metrics for both screening and confirmatory methods.
The main challenges in mass spectrometry analysis arise due to the low target LOQs and the high sensitivity required to achieve those. Another challenging factor is the presence of several compounds with different properties and different sources of environmental contamination. Additional critical key issues linked with PFAS analysis are background contamination from other laboratory equipment or consumables (e.g., solvents, detergents, glassware, aluminum foil, lubricants, Decon 90, Teflon tubing used in (U) HPLC and sample extraction instrumentation, etc.), as well as quantification of the sum of linear and branched isomers as a result of the ECF synthesis of PFOS-related substances (European Union Reference Laboratory for Halogenated POPs in Feed and Food, 2022).
LC–MS and LC–MS/MS are targeted techniques which require analytical standards. As the list of chemical compounds classified as PFAS is in the thousands, the limited availability of reference standards, currently only available for a small subset, reduces the effectiveness of a targeted approach. Targeted techniques also measure only those substances for which the MS instrumentation has been calibrated and so fail to identify all PFAS substances present in the sample, therefore underestimating the full extent of PFAS contamination. Hence, it could be beneficial to make use of non-targeted methods (Strynar et al., 2023) such as non-target liquid chromatography-high resolution mass spectrometry, Adsorbable Organic Fluorine, Extractable Organic Fluorine, Total Oxidizable Precursor Assay (Göckener et al., 2023) or Total Organic Fluorine Assay. These methods quantify the presence of fluorine atoms within a sample. Nonetheless, although these chromatographic non-targeted methods can be used for water samples, food and feed samples represent a more challenging matrix. Another method that can be used for total PFAS detection are bioassays similar to the ones currently accepted for dioxins (Eichbaum et al., 2014; European Commission and Directorate-General for Health and Food Safety, 2017). Bioassays measure the biological responses of certain strains of bacteria or cell cultures via signal transduction pathways when exposed to chemicals (Tian et al., 2012). In this case, the bioassay does not determine concentrations of PFAS, but the effect of any bioactive PFAS which would, in turn, give a positive response. At present, bioassays are not a realistic possibility as regulations for food do not consider relative potency factors. Moreover, these methods are costly, limited in their transferability between laboratories and can often give false positives due to other contaminants being present in the sample.
While MS methods are highly sensitive and accurate, they are also time-consuming, expensive, and require specialized equipment and trained personnel. In contrast, biosensors such as lateral flow assays offer a promising alternative for PFAS detection as they are typically faster, cheaper, and easier to use than traditional methods, and they can be designed for on-site, real-time detection, which is crucial for effective environmental management and immediate response to contamination events (Huang et al., 2019). A non-exhaustive list of currently available sensor technologies for PFAS detection is displayed in Table 4. Recent efforts in biosensing applications are also focused on the use of binding proteins or protein bioreceptors for the detection of PFAS (Moro et al., 2020; Daems et al., 2021; Mann et al., 2022; Kowalska et al., 2023). The development of biosensors for PFAS detection, particularly in complex food matrices remains a challenge, as current efforts for end-product sensor development in the field focus heavily on water samples as their target analyte (Rodriguez et al., 2020; Garg et al., 2022).
5 Mitigation
PFAS remediation processes for contaminated drinking water are difficult and costly to implement (Brunn et al., 2023). Strategies should focus on regulating the use of PFAS in consumer products to minimize the appearance of these chemical substances at the contamination sources (Voulgaropoulos, 2022). Initial legislative steps toward this goal are already being taken via the implementation of maximum levels in drinking water and foodstuffs. Nonetheless, water purification treatments should be a priority until the effects of recent policies and regulations begin to materialize. PFAS removal technologies for water systems consist of diverse approaches, such as granular activated carbon, reverse osmosis, nanofiltration or ion exchange resins (Voulgaropoulos, 2022; Brunn et al., 2023). All of these processes have limitations and are largely ineffective in removing PFAS from water. Granular activated carbon is ineffective for short-chain PFAS. Ion exchange resins, although more efficient, are more expensive as loading volumes are small and regeneration is restricted (US EPA, 2018). Reverse osmosis is energy-intensive and often not as effective. An alternative to purification is PFAS degradation. Advances in this field have increased as the extent of PFAS pollution becomes more apparent. Degradation of PFAS can occur as a result of defluorination or biotransformation caused by a number of microbial species and their enzymes. Examples of bacteria capable of microbial degradation are Pseudomonads, Acidimicrobium sp., and Gordonia sp. (Huang and Jaffé, 2019; Berhanu et al., 2023). An active sludge community in anaerobic conditions can also be used for PFAS biodegradation (Yu et al., 2022). Microbial degradation, although promising, still requires more work on identifying robust microorganisms and enzymes that can degrade PFAS in a targeted way (Berhanu et al., 2023). Thermal methods are another type of treatment processes used for the removal of PFAS (Winchell et al., 2022). These include microwave hydrothermal treatment, subcritical or supercritical treatment, electrical discharge plasma technology, or incineration, among others (Verma et al., 2023). These processes show good results at a laboratory scale but their applications in the real world still need to be investigated. Additional limitations are by-product formation, poor performance and high energy consumption (Verma et al., 2023). Oxidative and reductive processes can also be used as a mechanism for PFAS mitigation (Gar Alalm and Boffito, 2022). Electrochemical oxidation (Wang et al., 2022; Barisci and Suri, 2023; Mirabediny et al., 2023) as well as UV photolysis (Chen et al., 2022) are some of those methods which are gaining popular attention. Their efficiency depends on the chemical structure of the PFAS to be destructed. On top of that, nanomaterials are being explored for the elimination of PFAS (Saleh et al., 2019; Modiri-Gharehveran et al., 2023). Although all of these processes would serve a purpose of PFAS remediation, they could also have a detrimental effect on the environment, failing to restore the polluted sites to their original state (Buttle et al., 2023). Combinations of different techniques and further research will point us in the right direction for targeted PFAS degradation in complex matrices, being mindful of careful optimization and the formation of co-contaminants in the process.
One of the critical global environmental and public health challenges is the concentration of PFAS in wastewater biosolids, which is exacerbated by wastewater treatment plants aggregating these substances (Thompson et al., 2022). Crucially, treatment processes such as anaerobic digestion can transform PFAS precursors into more harmful terminal end compounds subject to regulation, which persist in biosolids applied to land (Lenka et al., 2021; O’Connor et al., 2022). This cycle poses a risk of PFAS accumulation in soil and crops, underscoring the need for advanced treatment methods and regulatory control to mitigate food chain contamination (Link et al., 2024). There are many understudied variables linked to PFAS uptake in feed materials and products such as the transfer of variable PFAS amounts from feed to animals and products; variation in PFAS feed levels due to species, origin, season, and production method; animal sensitivity and contamination duration; and limited knowledge of PFAS substances. To ensure food safety and sustainability, it is crucial to reduce or eliminate PFAS sources in feed to protect human and animal health, as well as the broader environment, until more scientific evidence is available. Furthermore, in certain foodstuffs such as seafood, studies have reported that cooking methods such as boiling, frying and baking do not reduce the concentration of PFAS (Taylor et al., 2019; Chen et al., 2023). Surprisingly, in some cases, the cooking actually increased the concentration of the sum of PFAS in seafood, increasing the risks of dietary exposure of these chemicals.
Due to the large extent of PFAS occurrence in foodstuffs, combined with the low legislative maximum levels of detection, there is a possibility that PFAS contamination will be present in all tested foods as official standard analytical methods become more readily available. In this scenario, researchers and policymakers are faced with an uneasy question: Would the recommended course of action be to destroy all the contaminated food above regulatory PFAS limits? This solution would not be sustainable in a world where the population in need of food is estimated to grow exponentially. There needs to be a way to ensure sustainability in the current food system while dealing with and mitigating the PFAS problem. The use of PFAS-free feed sources would be a crucial step in this process. Further scientific evidence in relation to PFAS and feed would help to better understand PFAS behavior in the food chain and develop mitigation strategies accordingly. Additional measures would involve the implementation of strict control and monitoring of PFAS-containing products entering the food chain, regulatory oversight to enforce legislation on PFAS maximum levels, safe husbandry practices, providing education and awareness among stakeholders, and fostering collaboration between agriculture, food industry and regulatory bodies to adapt and implement the best mitigation strategies possible. Moreover, the challenge of PFAS contamination takes on a different dimension in low-income countries, as these regions often lack the infrastructure and resources to effectively monitor and manage such contaminants. The limited availability of advanced analytical methods for detecting PFAS compounds exacerbates the issue, potentially leading to higher exposure levels in local food supplies. Furthermore, the economic constraints and dependence on agricultural outputs make it difficult for these countries to discard contaminated foodstuffs, as doing so could aggravate food insecurity and economic hardships. In addition, the trend of high-income countries importing high-quality food from low-income countries presents a complex dilemma in the context of PFAS contamination. This practice could lead to a situation where low-income countries are incentivized to export their best produce, potentially leaving lower quality, and possibly more contaminated, foodstuffs for local consumption. This dynamic not only raises concerns about food safety and equity but also about the sustainability of food systems, as developing nations might face increased pressure to meet export demands while grappling with contamination issues. In these settings, sustainable approaches to the PFAS problem are not only crucial but also need to be tailored to local capacities and realities. This includes developing cost-effective methods for PFAS detection and remediation, strengthening regulatory frameworks, and enhancing local expertise and awareness about PFAS risks. International cooperation and support are vital to establish sustainable strategies for managing PFAS contamination, ensuring that measures taken do not compromise food security and livelihoods while safeguarding public health and the environment.
6 Conclusion
PFAS assessment and management requires holistic and cross-cutting approaches combined with novel analytical tools in a more dynamic legislative framework. There is still a need for the development of standardized reliable sensitive and cost-effective methods for the detection of sub-nanogram levels of PFAS in complex food matrixes (Garg et al., 2022). Both targeted and non-targeted screening procedures should be further evaluated in order to broaden the control and monitoring of the vast number of per- and polyfluoroalkyl substances in the environment. In addition, a greater understanding of the occurrence of these compounds is required and without highly sensitive inexpensive analytical tools this can only be performed in limited laboratories specializing in this area of work. The ultimate goal is to both minimize PFAS exposure and ensure a sustainable food supply. This requires a multi-faceted approach involving regulatory, industry, and consumer efforts.
Author contributions
DS-H: Writing – original draft, Writing – review & editing. HM: Writing – review & editing. MS: Writing – review & editing. RT: Writing – review & editing. SH: Writing – review & editing. LM: Writing – review & editing. KC: Writing – review & editing.
Funding
The author(s) declare financial support was received for the research, authorship, and/or publication of this article. This work was supported by the Natural Environment Research Council (grant no. NE/S007377/1).
Conflict of interest
The authors declare that the research was conducted in the absence of any commercial or financial relationships that could be construed as a potential conflict of interest.
The author(s) declared that they were an editorial board member of Frontiers, at the time of submission. This had no impact on the peer review process and the final decision.
Publisher’s note
All claims expressed in this article are solely those of the authors and do not necessarily represent those of their affiliated organizations, or those of the publisher, the editors and the reviewers. Any product that may be evaluated in this article, or claim that may be made by its manufacturer, is not guaranteed or endorsed by the publisher.
References
AOAC International. (2022). New initiative: integrated standards and official methods development project for per- and Polyfluoroalkyl substances (PFAS) in foods. Available at: https://www.aoac.org/news/new-initiative-integrated-standards-and-official-methods-development-project-for-per-and-polyfluoroalkyl-substances-pfas-in-foods/#:~:text=New%20Initiative%3A%20Integrated%20Standards%20and%20Official%20Methods%20Development,processed%20and%20packaged%20foods%20and%20raw%20agricultural%20commodities (Accessed August 9, 2023).
ATSDR (2021). Toxicological profile for Perfluoroalkyls. Atlanta: Department of Health and Human Services, Public Health Service.
Baluyot, J. C., Reyes, E. M., and Velarde, M. C. (2021). Per- and polyfluoroalkyl substances (PFAS) as contaminants of emerging concern in Asia’s freshwater resources. Environ. Res. 197:111122. doi: 10.1016/j.envres.2021.111122
Barisci, S., and Suri, R. (2023). Degradation of emerging per- and polyfluoroalkyl substances (PFAS) using an electrochemical plug flow reactor. J. Hazard. Mater. 460:132419. doi: 10.1016/j.jhazmat.2023.132419
Berhanu, A., Mutanda, I., Taolin, J., Qaria, M. A., Yang, B., and Zhu, D. (2023). A review of microbial degradation of per- and polyfluoroalkyl substances (PFAS): biotransformation routes and enzymes. Sci. Total Environ. 859:160010. doi: 10.1016/j.scitotenv.2022.160010
Breshears, L. E., Mata-Robles, S., Tang, Y., Baker, J. C., Reynolds, K. A., and Yoon, J.-Y. (2023). Rapid, sensitive detection of PFOA with smartphone-based flow rate analysis utilizing competitive molecular interactions during capillary action. J. Hazard. Mater. 446:130699. doi: 10.1016/j.jhazmat.2022.130699
Brunn, H., Arnold, G., Körner, W., Rippen, G., Steinhäuser, K. G., and Valentin, I. (2023). PFAS: forever chemicals—persistent, bioaccumulative and mobile. Reviewing the status and the need for their phase out and remediation of contaminated sites. Environ. Sci. Eur. 35:20. doi: 10.1186/s12302-023-00721-8
Buck, R. C., Franklin, J., Berger, U., Conder, J. M., Cousins, I. T., De Voogt, P., et al. (2011). Perfluoroalkyl and polyfluoroalkyl substances in the environment: terminology, classification, and origins. Integr. Environ. Assess. Manag. 7, 513–541. doi: 10.1002/ieam.258
Buttle, E., Sharp, E. L., and Fisher, K. (2023). Managing ubiquitous ‘forever chemicals’: more-than-human possibilities for the problem of Pfas. N. Z. Geogr. 79, 97–106. doi: 10.1111/nzg.12365
Cennamo, N., D’agostino, G., Porto, G., Biasiolo, A., Perri, C., Arcadio, F., et al. (2018a). A molecularly imprinted polymer on a Plasmonic plastic optical Fiber to detect Perfluorinated compounds in water. Sensors 18:1836. doi: 10.3390/s18061836
Cennamo, N., Zeni, L., Tortora, P., Regonesi, M. E., Giusti, A., Staiano, M., et al. (2018b). A high sensitivity biosensor to detect the presence of perfluorinated compounds in environment. Talanta 178, 955–961. doi: 10.1016/j.talanta.2017.10.034
Chen, Q., Cheng, Z., Du, L., Zhu, P., and Tan, K. (2018). A sensitive three-signal assay for the determination of PFOS based on the interaction with Nile blue A. Anal. Methods 10, 3052–3058. doi: 10.1039/C8AY00938D
Chen, L. D., Lai, C.-Z., Granda, L. P., Fierke, M. A., Mandal, D., Stein, A., et al. (2013). Fluorous membrane ion-selective electrodes for Perfluorinated surfactants: trace-level detection and in situ monitoring of adsorption. Anal. Chem. 85, 7471–7477. doi: 10.1021/ac401424j
Chen, S., Li, A., Zhang, L., and Gong, J. (2015). Molecularly imprinted ultrathin graphitic carbon nitride nanosheets–based electrochemiluminescence sensing probe for sensitive detection of perfluorooctanoic acid. Anal. Chim. Acta 896, 68–77. doi: 10.1016/j.aca.2015.09.022
Chen, G., Liu, S., Shi, Q., Gan, J., Jin, B., Men, Y., et al. (2022). Hydrogen-polarized vacuum ultraviolet photolysis system for enhanced destruction of perfluoroalkyl substances. J. Hazard. Mater. Lett. 3:100072. doi: 10.1016/j.hazl.2022.100072
Chen, Z., Zhan, X., Zhang, J., Diao, J., Su, C., Sun, Q., et al. (2023). Bioaccumulation and risk mitigation of legacy and novel perfluoroalkyl substances in seafood: insights from trophic transfer and cooking method. Environ. Int. 177:108023. doi: 10.1016/j.envint.2023.108023
Chen, Q., Zhu, P., Xiong, J., Gao, L., and Tan, K. (2019). A sensitive and selective triple-channel optical assay based on red-emissive carbon dots for the determination of PFOS. Microchem. J. 145, 388–396. doi: 10.1016/j.microc.2018.11.003
Chen, Q., Zhu, P., Xiong, J., Gao, L., and Tan, K. (2020). A new dual-recognition strategy for hybrid ratiometric and ratiometric sensing perfluorooctane sulfonic acid based on high fluorescent carbon dots with ethidium bromide. Spectrochim. Acta A Mol. Biomol. Spectrosc. 224:117362. doi: 10.1016/j.saa.2019.117362
Cheng, Y. H., Barpaga, D., Soltis, J. A., Shutthanandan, V., Kargupta, R., Han, K. S., et al. (2020). Metal–organic framework-based microfluidic impedance sensor platform for ultrasensitive detection of Perfluorooctanesulfonate. ACS Appl. Mater. Interfaces 12, 10503–10514. doi: 10.1021/acsami.9b22445
Cheng, Z., Zhang, F., Chen, X., Du, L., Gao, C., and Tan, K. (2016). Highly sensitive and selective detection of perfluorooctane sulfonate based on the Janus green B resonance light scattering method. Anal. Methods 8, 8042–8048. doi: 10.1039/C6AY02739C
Daems, E., Moro, G., Berghmans, H., Moretto, L. M., Dewilde, S., Angelini, A., et al. (2021). Native mass spectrometry for the design and selection of protein bioreceptors for perfluorinated compounds. Analyst 146, 2065–2073. doi: 10.1039/D0AN02005B
Delatour, T., Theurillat, X., Mujahid, C., Eriksen, B. R., and Mottier, P. (2023). Determination of poly- and Perfluoroalkylated substances in food: how consistent are results across laboratories? J. Agric. Food Chem. 71, 4767–4768. doi: 10.1021/acs.jafc.3c01306
DTU National Food Institute (2023). Indhold af PFAS i fiskemel og via indhold i økologisk foder i økologiske æg. DTU Fødevareinstituttet. Available at: https://www.bing.com/ck/a?!&&p=295fb98868be87afJmltdHM9MTcwNTk2ODAwMCZpZ3VpZD0zZTcyNDg5Zi1lNWQyLTY2YTUtMmU0NC01YWYzZTRkNDY3ODAmaW5zaWQ9NTE4OA&ptn=3&ver=2&hsh=3&fclid=3e72489f-e5d2-66a5-2e44-5af3e4d46780&psq=PFAS-i-oekologiske-aeg-og-foder-Jan-2023%2520(2).pdf&u=a1aHR0cHM6Ly93d3cuZm9vZC5kdHUuZGsvLS9tZWRpYS9pbnN0aXR1dHRlci9mb2VkZXZhcmVpbnN0aXR1dHRldC9wdWJsaWthdGlvbmVyL3B1Yi0yMDIzL3BmYXMtaS1vZWtvbG9naXNrZS1hZWctb2ctZm9kZXItamFuLTIwMjMucGRmP2xhPWRhJmhhc2g9MzA4RjE0NDZGOUFFQzhFNjc0NkY4NDQ4MkRCREY1Rjg0QjJGRDc2Ng&ntb=1
DWI (2022). PFAS and forever chemicals. Available at: https://www.dwi.gov.uk/pfas-and-forever-chemicals/#top (Accessed December 29, 2023).
ECHA (2022). Proposal to ban ‘forever chemicals’ in firefighting foams throughout the Eu. Available at: https://echa.europa.eu/-/proposal-to-ban-forever-chemicals-in-firefighting-foams-throughout-the-eu (Accessed December 28, 2022).
EFSA CONTAM Panel Schrenk, D., Bignami, M., Bodin, L., Chipman, J. K., Del Mazo, J., et al. (2020). Risk to human health related to the presence of perfluoroalkyl substances in food. EFSA J. 18:e06223. doi: 10.2903/j.efsa.2020.6223
Eichbaum, K., Brinkmann, M., Buchinger, S., Reifferscheid, G., Hecker, M., Giesy, J. P., et al. (2014). In vitro bioassays for detecting dioxin-like activity—application potentials and limits of detection, a review. Sci. Total Environ. 487, 37–48. doi: 10.1016/j.scitotenv.2014.03.057
Environment Agency (2021). Poly- and perfluoroalkyl substances (PFAS): sources, pathways and environmental data. Bristol: Environment Agency (2021).
European Commission (2020). Chemicals strategy for sustainability towards a toxic-free environment. Brussels. Publications Office of the European Union. Available at: https://eur-lex.europa.eu/LexUriServ/LexUriServ.do?uri=SWD:2020:0250:FIN:EN:PDF
European Commission and Directorate-General for Health and Food Safety (2017). Commission regulation (Eu) 2017/644 of 5 April 2017 laying down methods of sampling and analysis for the control of levels of dioxins, dioxin-like Pcbs and non-dioxin-like Pcbs in certain foodstuffs and repealing regulation (Eu) no 589/2014. Brussels: Official Journal of the European Union.
European Commission and Directorate-General for Health and Food Safety (2022a). Commission implementing regulation (Eu) 2022/1428 of 24 august 2022 laying down methods of sampling and analysis for the control of perfluoroalkyl substances in certain foodstuffs. Brussels: Official Journal of the European Union.
European Commission and Directorate-General for Health and Food Safety (2022b). Commission recommendation (Eu) 2022/1431 of 24 august 2022 on the monitoring of perfluoroalkyl substances in food. Brussels: Official Journal of the European Union.
European Commission and Directorate-General for Health and Food Safety (2022c). Commission regulation (Eu) 2022/2388 of 7 December 2022 amending regulation (Ec) no 1881/2006 as regards maximum levels of perfluoroalkyl substances in certain foodstuffs. Brussels: Official Journal of the European Union.
European Commission and Directorate-General for Health and Food Safety (2023). Commission regulation (Eu) 2023/915 of 25 April 2023 on maximum levels for certain contaminants in food and repealing regulation (Ec) no 1881/2006. Brussels: Official Journal of the European Union.
European Parliament and Council of the European Union (2006). Regulation (Ec) no 1907/2006 of the European Parliament and of the council of 18 December 2006 concerning the registration, evaluation, authorisation and restriction of chemicals (Reach), establishing a European chemicals Agency, amending directive 1999/45/Ec and repealing council regulation (Eec) no 793/93 and commission regulation (Ec) no 1488/94 as well as council directive 76/769/Eec and commission directives 91/155/Eec, 93/67/Eec, 93/105/Ec and 2000/21/Ec. Brussels: Official journal of the European Union.
European Parliament and Council of the European Union (2020). Directive (Eu) 2020/2184 of the European Parliament and of the council of 16 December 2020 on the quality of water intended for human consumption. Brussels: Official Journal of the European Union.
European Union Reference Laboratory for Halogenated POPs in Feed and Food (2022). Guidance document on analytical parameters for the determination of per- and Polyfluoroalkyl substances (Pfas) in food and feed, version 1.2 of 11 May 2022. EURL for halogenated POPs in Feed and Food c/o State Institute for Chemical and Veterinary Analysis Freiburg. Available at: https://eurl-pops.eu/user/pages/05.news/18.Guidance-Document-PFAS/Guidance%20Document%20on%20Analytical%20Parameters%20for%20the%20Determination%20of%20Per-%20and%20Polyfluoroalkyl%20Substances%20(PFAS)%20in%20Food%20and%20Feed%20V1.2%20(incl.%20Annex%20V1.0).pdf?g-64cde584
Evich, M. G., Davis, M. J. B., Mccord, J. P., Acrey, B., Awkerman, J. A., Knappe, D. R. U., et al. (2022). Per- and polyfluoroalkyl substances in the environment. Science 375:eabg9065. doi: 10.1126/science.abg9065
Fang, C., Chen, Z., Megharaj, M., and Naidu, R. (2016). Potentiometric detection of Afffs based on Mip. Environ. Technol. Innov. 5, 52–59. doi: 10.1016/j.eti.2015.12.003
Fang, C., Zhang, X., Dong, Z., Wang, L., Megharaj, M., and Naidu, R. (2018). Smartphone app-based/portable sensor for the detection of fluoro-surfactant PFOA. Chemosphere 191, 381–388. doi: 10.1016/j.chemosphere.2017.10.057
Feng, H., Wang, N., Tran, T. T., Yuan, L., Li, J., and Cai, Q. (2014). Surface molecular imprinting on dye–(Nh2)–SiO2 Nps for specific recognition and direct fluorescent quantification of perfluorooctane sulfonate. Sensors Actuators B Chem. 195, 266–273. doi: 10.1016/j.snb.2014.01.036
Fernandes, A. R., Lake, I. R., Dowding, A., Rose, M., Jones, N. R., Smith, F., et al. (2023). The transfer of environmental contaminants (brominated and chlorinated dioxins and biphenyls, PBDEs, HBCDDs, PCNs and PFAS) from recycled materials used for bedding to the eggs and tissues of chickens. Sci. Total Environ. 892:164441. doi: 10.1016/j.scitotenv.2023.164441
Ganesan, S., Chawengkijwanich, C., Gopalakrishnan, M., and Janjaroen, D. (2022). Detection methods for sub-nanogram level of emerging pollutants – per and polyfluoroalkyl substances. Food Chem. Toxicol. 168:113377. doi: 10.1016/j.fct.2022.113377
Gar Alalm, M., and Boffito, D. C. (2022). Mechanisms and pathways of Pfas degradation by advanced oxidation and reduction processes: a critical review. Chem. Eng. J. 450:138352. doi: 10.1016/j.cej.2022.138352
Garg, S., Kumar, P., Greene, G., Mishra, V., Avisar, D., Sharma, R., et al. (2022). Nano-enabled sensing of per−/poly-fluoroalkyl substances (Pfas) from aqueous systems – a review. J. Environ. Manag. 308:114655. doi: 10.1016/j.jenvman.2022.114655
Getzinger, G. J., and Ferguson, P. L. (2021). High-throughput trace-level suspect screening for per- and Polyfluoroalkyl substances in environmental waters by peak-focusing online solid phase extraction and high-resolution mass spectrometry. ACS ES T Water 1, 1240–1251. doi: 10.1021/acsestwater.0c00309
Göckener, B., Fliedner, A., Weinfurtner, K., Rüdel, H., Badry, A., and Koschorreck, J. (2023). Tracking down unknown Pfas pollution – the direct top assay in spatial monitoring of surface waters in Germany. Sci. Total Environ. 898:165425. doi: 10.1016/j.scitotenv.2023.165425
Hammel, E., Webster, T. F., Gurney, R., and Heiger-Bernays, W. (2022). Implications of Pfas definitions using fluorinated pharmaceuticals. iScience 25:104020. doi: 10.1016/j.isci.2022.104020
HBM4EU (2022). Substance report: per- and poly-fluoroalkyl substances (PFAS). HBM4EU. Available at: https://www.hbm4eu.eu/wpcontent/uploads/2022/07/PFAS_Substance-report.pdf
Houde, M., De Silva, A. O., Muir, D. C. G., and Letcher, R. J. (2011). Monitoring of perfluorinated compounds in aquatic biota: an updated review. Environ. Sci. Technol. 45, 7962–7973. doi: 10.1021/es104326w
Huang, S., and Jaffé, P. R. (2019). Defluorination of Perfluorooctanoic acid (PFOA) and Perfluorooctane sulfonate (PFOS) by Acidimicrobium sp. strain A6. Environ. Sci. Technol. 53, 11410–11419. doi: 10.1021/acs.est.9b04047
Huang, Y., Xu, T., Wang, W., Wen, Y., Li, K., Qian, L., et al. (2019). Lateral flow biosensors based on the use of micro- and nanomaterials: a review on recent developments. Microchim. Acta 187:70. doi: 10.1007/s00604-019-3822-x
Ingelido, A. M., Abballe, A., Gemma, S., Dellatte, E., Iacovella, N., De Angelis, G., et al. (2018). Biomonitoring of perfluorinated compounds in adults exposed to contaminated drinking water in the Veneto region, Italy. Environ. Int. 110, 149–159. doi: 10.1016/j.envint.2017.10.026
Karimian, N., Stortini, A. M., Moretto, L. M., Costantino, C., Bogialli, S., and Ugo, P. (2018). Electrochemosensor for trace analysis of Perfluorooctanesulfonate in water based on a molecularly imprinted poly (o-phenylenediamine) polymer. ACS Sens. 3, 1291–1298. doi: 10.1021/acssensors.8b00154
Kowalska, D., Sosnowska, A., Bulawska, N., Stępnik, M., Besselink, H., Behnisch, P., et al. (2023). How the structure of per- and Polyfluoroalkyl substances (Pfas) influences their binding potency to the peroxisome proliferator-activated and thyroid hormone receptors–an in silico screening study. Molecules 28:479. doi: 10.3390/molecules28020479
Lenka, S. P., Kah, M., and Padhye, L. P. (2021). A review of the occurrence, transformation, and removal of poly- and perfluoroalkyl substances (Pfas) in wastewater treatment plants. Water Res. 199:117187. doi: 10.1016/j.watres.2021.117187
Li, J., Zhang, C., Yin, M., Zhang, Z., Chen, Y., Deng, Q., et al. (2019). Surfactant-sensitized covalent organic frameworks-functionalized lanthanide-doped nanocrystals: an ultrasensitive sensing platform for Perfluorooctane sulfonate. ACS Omega 4, 15947–15955. doi: 10.1021/acsomega.9b01996
Link, G. W., Reeves, D. M., Cassidy, D. P., and Coffin, E. S. (2024). Per- and polyfluoroalkyl substances (PFAS) in final treated solids (biosolids) from 190 Michigan wastewater treatment plants. J. Hazard. Mater. 463:132734. doi: 10.1016/j.jhazmat.2023.132734
Liu, L., Qu, Y., Huang, J., and Weber, R. (2021). Per- and polyfluoroalkyl substances (PFASS) in Chinese drinking water: risk assessment and geographical distribution. Environ. Sci. Eur. 33:6. doi: 10.1186/s12302-020-00425-3
Mann, M. M., Tang, J. D., and Berger, B. W. (2022). Engineering human liver fatty acid binding protein for detection of poly- and perfluoroalkyl substances. Biotechnol. Bioeng. 119, 513–522. doi: 10.1002/bit.27981
Mirabediny, M., Sun, J., Yu, T. T., Åkermark, B., Das, B., and Kumar, N. (2023). Effective PFAS degradation by electrochemical oxidation methods-recent progress and requirement. Chemosphere 321:138109. doi: 10.1016/j.chemosphere.2023.138109
Modiri-Gharehveran, M., Choi, Y., Zenobio, J. E., and Lee, L. S. (2023). Perfluoroalkyl acid transformation and mitigation by nNiFe-activated carbon nanocomposites in steady-state flow column studies. J. Environ. Sci. 127, 678–687. doi: 10.1016/j.jes.2022.06.040
Moro, G., Bottari, F., Liberi, S., Covaceuszach, S., Cassetta, A., Angelini, A., et al. (2020). Covalent immobilization of delipidated human serum albumin on poly (pyrrole-2-carboxylic) acid film for the impedimetric detection of perfluorooctanoic acid. Bioelectrochemistry 134:107540. doi: 10.1016/j.bioelechem.2020.107540
Nahar, K., Zulkarnain, N. A., and Niven, R. K. (2023). A review of analytical methods and Technologies for Monitoring per- and Polyfluoroalkyl Substances (PFAS) in water. Water 15:3577. doi: 10.3390/w15203577
Niu, H., Wang, S., Zhou, Z., Ma, Y., Ma, X., and Cai, Y. (2014). Sensitive colorimetric visualization of Perfluorinated compounds using poly (ethylene glycol) and Perfluorinated thiols modified gold nanoparticles. Anal. Chem. 86, 4170–4177. doi: 10.1021/ac403406d
O’connor, J., Bolan, N. S., Kumar, M., Nitai, A. S., Ahmed, M. B., Bolan, S. S., et al. (2022). Distribution, transformation and remediation of poly- and per-fluoroalkyl substances (PFAS) in wastewater sources. Process Saf. Environ. Prot. 164, 91–108. doi: 10.1016/j.psep.2022.06.002
OECD (2021). Reconciling terminology of the universe of per- and Polyfluoroalkyl substances: Recommendations and practical guidance. OECD Environment, Health and Safety Publications, Paris.
Ogunbiyi, O. D., Ajiboye, T. O., Omotola, E. O., Oladoye, P. O., Olanrewaju, C. A., and Quinete, N. (2023). Analytical approaches for screening of per- and poly fluoroalkyl substances in food items: a review of recent advances and improvements. Environ. Pollut. 329:121705. doi: 10.1016/j.envpol.2023.121705
OSPAR Commission (2013). Ospar list of chemicals for priority action (revised 2013). OSPAR Convention for the Protection of the Marine Environment of the North-East Atlantic. Available at: https://view.officeapps.live.com/op/view.aspx?src=https%3A%2F%2Fospar-archive.s3.eu-west-1.amazonaws.com%2FDECRECS%2FAGREEMENTS%2F04-12e_agreement_list_of_chemicals_for_priority_action.doc%3FX-Amz-Content-Sha256%3DUNSIGNED-PAYLOAD%26X-Amz-Algorithm%3DAWS4-HMAC-SHA256%26X-Amz-Credential%3DAKIAJIACMW2T5USCSU5A%252F20240123%252Feu-west-1%252Fs3%252Faws4_request%26X-Amz-Date%3D20240123T155414Z%26X-Amz-SignedHeaders%3Dhost%26X-Amz-Expires%3D900%26X-Amz-Signature%3D95226355b85cfaad1ed08a8e79c9d1c34bc72f0cfe5f19ca2f1ae820af96c69f&wdOrigin=BROWSELINK
Park, J., Yang, K., Choi, Y., and Choe, J. (2022). Novel ssdna aptamer-based fluorescence sensor for perfluorooctanoic acid detection in water. Environ. Int. 158:107000. doi: 10.1016/j.envint.2021.107000
Rodriguez, K., Hwang, J., Esfahani, A., Sadmani, A., and Lee, W. (2020). Recent developments of Pfas-detecting sensors and future direction: a review. Micromachines 11:667. doi: 10.3390/mi11070667
Saleh, N. B., Khalid, A., Tian, Y., Ayres, C., Sabaraya, I. V., Pietari, J., et al. (2019). Removal of poly- and per-fluoroalkyl substances from aqueous systems by nano-enabled water treatment strategies. Environ. Sci. 5, 198–208. doi: 10.1039/C8EW00621K
Sørensen, M. M., Fisker, A. B., Dalgård, C., Jensen, K. J., Nielsen, F., Benn, C. S., et al. (2023). Predictors of serum- per- and polyfluoroalkyl substance (PFAS) concentrations among infants in Guinea-Bissau, West Africa. Environ. Res. 228:115784. doi: 10.1016/j.envres.2023.115784
Steenland, K., Fletcher, T., and Savitz, D. A. (2010). Epidemiologic evidence on the health effects of Perfluorooctanoic acid (PFOA). Environ. Health Perspect. 118, 1100–1108. doi: 10.1289/ehp.0901827
Stockholm Convention. (2019). All pops listed in the stockholm convention. Switzerland: Secretariat of the Stockholm Convention. Available at: https://chm.pops.int/TheConvention/Thepops/Listingofpops/tabid/2509/Default.aspx (Accessed January 5, 2023).
Straková, J., Brosché, S., and Grechko, V. (2023). Forever chemicals in single-use food packaging and tableware from 17 countries IPEN. Available at: https://ipen.org/sites/default/files/documents/ipen-packaging-report-fin2.pdf
Strynar, M., Mccord, J., Newton, S., Washington, J., Barzen-Hanson, K., Trier, X., et al. (2023). Practical application guide for the discovery of novel Pfas in environmental samples using high resolution mass spectrometry. J. Expo. Sci. Environ. Epidemiol. 33, 575–588. doi: 10.1038/s41370-023-00578-2
Takayose, M., Akamatsu, K., Nawafune, H., Murashima, T., and Matsui, J. (2012). Colorimetric detection of Perfluorooctanoic acid (PFOA) utilizing polystyrene-modified gold nanoparticles. Anal. Lett. 45, 2856–2864. doi: 10.1080/00032719.2012.696225
Tang, Z. W., Shahul Hamid, F., Yusoff, I., and Chan, V. (2023). A review of PFAS research in Asia and occurrence of PFOA and PFOS in groundwater, surface water and coastal water in Asia. Groundw. Sustain. Dev. 22:100947. doi: 10.1016/j.gsd.2023.100947
Taylor, M. D., Nilsson, S., Bräunig, J., Bowles, K. C., Cole, V., Moltschaniwskyj, N. A., et al. (2019). Do conventional cooking methods alter concentrations of per- and polyfluoroalkyl substances (PFASs) in seafood? Food Chem. Toxicol. 127, 280–287. doi: 10.1016/j.fct.2019.03.032
Thompson, K. A., Mortazavian, S., Gonzalez, D. J., Bott, C., Hooper, J., Schaefer, C. E., et al. (2022). Poly- and Perfluoroalkyl substances in municipal wastewater treatment plants in the United States: seasonal patterns and Meta-analysis of long-term trends and average concentrations. ACS ES T Water 2, 690–700. doi: 10.1021/acsestwater.1c00377
Tian, W., Xie, H. Q., Fu, H., Pei, X., and Zhao, B. (2012). Immunoanalysis methods for the detection of dioxins and related chemicals. Sensors 12, 16710–16731. doi: 10.3390/s121216710
Tian, Y., Zhou, Y., Miao, M., Wang, Z., Yuan, W., Liu, X., et al. (2018). Determinants of plasma concentrations of perfluoroalkyl and polyfluoroalkyl substances in pregnant women from a birth cohort in Shanghai, China. Environ. Int. 119, 165–173. doi: 10.1016/j.envint.2018.06.015
Tran, T. T., Li, J., Feng, H., Cai, J., Yuan, L., Wang, N., et al. (2014). Molecularly imprinted polymer modified TiO2 nanotube arrays for photoelectrochemical determination of perfluorooctane sulfonate (PFOS). Sensors Actuators B Chem. 190, 745–751. doi: 10.1016/j.snb.2013.09.048
United Nations Environment Programme (2023). Chemicals in plastics: a technical report. Geneva. UNEP. Available at: https://www.unep.org/resources/report/chemicals-plastics-technical-report
US EPA. (2018). Reducing PFAS in drinking water with treatment technologies. Available at: https://www.epa.gov/sciencematters/reducing-pfas-drinking-water-treatment-technologies (Accessed December 29, 2023).
US EPA. (2023). Proposed PFAS national primary drinking water regulation. Available at: https://www.epa.gov/sdwa/and-polyfluoroalkyl-substances-pfas#:~:text=On%20May%204%2C%202023%2C%20epa,Da%20%28commonly (Accessed December 29, 2023).
Verma, S., Lee, T., Sahle-Demessie, E., Ateia, M., and Nadagouda, M. N. (2023). Recent advances on PFAS degradation via thermal and nonthermal methods. Chem. Eng. J. Adv. 13:100421, –100411. doi: 10.1016/j.ceja.2022.100421
Voulgaropoulos, A. (2022). Mitigation of Pfas in U.S. public water systems: future steps for ensuring safer drinking water. Environ. Prog. Sustain. Energy 41:e13800. doi: 10.1002/ep.13800
Wang, Y., Li, L., and Huang, Q. (2022). Electrooxidation of per- and polyfluoroalkyl substances in chloride-containing water on surface-fluorinated Ti4O7 anodes: mitigation and elimination of chlorate and perchlorate formation. Chemosphere 307:135877. doi: 10.1016/j.chemosphere.2022.135877
Whitehead, P. (2020). What you are allowed to drink depends on where you live! Available at: https://www.elgalabwater.com/blog/what-you-are-allowed-drink-depends-where-you-live (Accessed January 8, 2024).
Winchell, L. J., Ross, J. J., Brose, D. A., Pluth, T. B., Fonoll, X., Norton, J. W. Jr., et al. (2022). High-temperature technology survey and comparison among incineration, pyrolysis, and gasification systems for water resource recovery facilities. Water Environ. Res. 94:e10715. doi: 10.1002/wer.10715
Winchell, L. J., Wells, M. J. M., Ross, J. J., Fonoll, X., Norton, J. W., Kuplicki, S., et al. (2021). Analyses of per- and polyfluoroalkyl substances (PFAS) through the urban water cycle: toward achieving an integrated analytical workflow across aqueous, solid, and gaseous matrices in water and wastewater treatment. Sci. Total Environ. 774:145257. doi: 10.1016/j.scitotenv.2021.145257
Xia, W., Wan, Y.-J., Wang, X., Li, Y.-Y., Yang, W.-J., Wang, C.-X., et al. (2011). Sensitive bioassay for detection of Pparα potentially hazardous ligands with gold nanoparticle probe. J. Hazard. Mater. 192, 1148–1154. doi: 10.1016/j.jhazmat.2011.06.023
Yu, Y., Che, S., Ren, C., Jin, B., Tian, Z., Liu, J., et al. (2022). Microbial Defluorination of unsaturated per- and Polyfluorinated carboxylic acids under anaerobic and aerobic conditions: a structure specificity study. Environ. Sci. Technol. 56, 4894–4904. doi: 10.1021/acs.est.1c05509
Keywords: per- and polyfluoroalkyl substances, methods of detection, food safety, food policy and governance, sustainability
Citation: Senovilla-Herrero D, Moore H, Service M, Thomas R, Helyar S, Mbadugha L and Campbell K (2024) In light of the new legislation for per- and polyfluoroalkyl substances, can continued food sustainability be achieved? Front. Sustain. Food Syst. 8:1339868. doi: 10.3389/fsufs.2024.1339868
Edited by:
Lin Luo, South China Agricultural University, ChinaReviewed by:
Zi-Jian Chen, Zhaoqing University, ChinaSurat Hongsibsong, Chiang Mai University, Thailand
Katherine Bell, Brown and Caldwell, United States
Copyright © 2024 Senovilla-Herrero, Moore, Service, Thomas, Helyar, Mbadugha and Campbell. This is an open-access article distributed under the terms of the Creative Commons Attribution License (CC BY). The use, distribution or reproduction in other forums is permitted, provided the original author(s) and the copyright owner(s) are credited and that the original publication in this journal is cited, in accordance with accepted academic practice. No use, distribution or reproduction is permitted which does not comply with these terms.
*Correspondence: Diana Senovilla-Herrero, dsenovillaherrero01@qub.ac.uk; Katrina Campbell, katrina.campbell@qub.ac.uk