- 1Trace and Save, Jeffreys Bay, Eastern Cape, South Africa
- 2Department of Agronomy, Stellenbosch University, Stellenbosch, Western Cape, South Africa
- 3Climate Neutral Group South Africa, Cape Town, Western Cape, South Africa
Environmental impact evaluation of intensive dairy farming systems has been of growing interest recently as agriculture has several negative influences on the surrounding environment, including eutrophication, declines in biodiversity, and pollution of nearby waterbodies. Dairy production in particular is characterized by the emission of greenhouse gasses (GHG) contributing toward climate change. In this study, the carbon footprint of South African pasture-based dairy farming systems was assessed using a farm-gate life-cycle assessment (LCA) approach. A total of 82 pasture-based dairy farms across South Africa were assessed (2012–2022). The average carbon footprint across all dairy farming systems was 1.36 ± 0.21 kg CO2eq kg−1 fat- and protein-corrected milk produced (FPCM), which is higher than similar studies performed outside South Africa. Enteric fermentation had the largest influence on the carbon footprint, indicating the key role of methane as an emission source in ruminant dominated livestock systems. A difference in milk production efficiency was found between farming systems with the lowest and highest carbon footprints. Pasture-based dairy farming systems must be managed with adaptive management such as regenerative agriculture. Future research agendas should explore modeling approaches to assess the economic and environmental impact of dairy production, formulating a holistic understanding of the system dynamics while also quantifying net carbon emissions or sinks.
Introduction
The profitability of South African pasture-based dairy farming systems is constantly under pressure due to the ever-increasing costs of inputs such as fertilizer, electricity, feed, labor, and agrochemicals (Galloway et al., 2018a; Viljoen et al., 2020). To sustainably increase net farm income dairy farmers must ensure resources are utilized optimally (Capper et al., 2009) while also improving the environmental impact of the farming system. The link between economic viability and environmental awareness for intensively managed pasture-based dairy farming systems is therefore critical to consider as both play a pivotal role in securing continuous dairy production to address global and local needs. Environmental impact evaluation of dairy production has been a growing interest recently (Food and Agriculture Organization and Global Dairy Platform, 2018; Mazzetto et al., 2022). Dairy farming generally has several negative influences on the surrounding environment, including water body pollution caused by nutrients (eutrophication) and pesticide runoff, soil erosion, and declines in biodiversity (Food and Agriculture Organization, 2010). Dairy production is characterized by the emission of greenhouse gasses (GHG) such as methane (CH4), nitrous oxide (N2O), and carbon dioxide (CO2), and is carefully quantified globally by conducting farm-gate life cycle assessment (LCA) studies (Bartl et al., 2011; Pirlo et al., 2014; Guest et al., 2017; Hietala et al., 2021). However, inconsistent results indicate the sensitivity of the LCA method to different modeling approaches and GHG calculations (Flysjö et al., 2011). Moreover, dairy farming systems are subjected to a wide variety of climate variables, soil conditions and agronomic management practices, resulting in different modeling inputs and variables affecting the calculations (Flysjö et al., 2011; Mazzetto et al., 2022). This is evident from LCA studies conducted in Europe, Australasia and Northern America, where GHG emissions ranged between 0.80 and 1.72 kg CO2eq kg−1 fat- and protein-corrected milk produced (FPCM) for pasture-based dairy systems (O'Brien et al., 2014; Chobtang et al., 2017; Christie, 2019; Rotz et al., 2021). Despite using similar metrics to quantify the GHG emissions of dairy farming systems, comparing GHG emissions between dairy systems located in different countries will result in inaccurate conclusions due to varying methodological aspects, such as emission factors, which restricts the direct comparison.
In a recent systematic review, Mazzetto et al. (2022) found that in pasture-based dairy systems, the main sources of GHG emissions were from enteric fermentation, manure management, fertilizer use, and livestock feed production, depending on the GHG profile of the specific country. Comparing the carbon footprints of dairy farming systems between countries is therefore a complex task and may lead to erroneous conclusions. Mitigation strategies will consequently differ between countries and multifaceted dairy farming systems (O'Brien et al., 2010; Zehetmeier et al., 2014) such as improved grazing and effluent management, effective nitrogen fertilizer strategies, and enhanced irrigation efficiency (Galloway et al., 2018a; Viljoen et al., 2020; Phohlo et al., 2022). Galloway et al. (2018b) indicated that carbon footprint quantification helps explore the underlying factors influencing the profitability of dairy production due to the correlation between lower GHG emissions and higher gross margins.
Despite the growing interest in dairy-based carbon footprint studies globally, assessments for South African pasture-based dairy farming systems are currently absent (Smit et al., 2021; Mazzetto et al., 2022) although livestock accounts for ~50% of annual CH4 emissions in South Africa (Department of Forestry, Fisheries and the Environment, 2021). In addition, dairy cattle account for ~12% of the annually emitted enteric CH4 from all livestock in South Africa (Tongwane and Moeletsi, 2020). This indicates a critical gap and highlights the need to evaluate the carbon footprint of South African pasture-based dairy farming systems in order to establish the effects of current on-farm agronomic and livestock management practices on the environment (Du Toit et al., 2013). The aim of this study was to assess the carbon footprint of South African pasture-based dairy farming systems using a farm-gate LCA approach. Our analysis explored the amount and range of GHG emissions and the specific factors influencing the GHG emissions. Sustainable opportunities to lower the carbon footprint of pasture-based dairy farming systems and future research options are presented.
Materials and methods
Data sources and overview
A total of 82 pasture-based dairy farming systems across three provinces in South Africa, namely the Western Cape, Eastern Cape, and KwaZulu-Natal provinces, were included in the assessment, representing data ranging from 2012 to 2022. Data were collected from farmers that form part of the Trace & Save farmer program and the Woodlands Dairy Sustainability Project (Trace and Save, 2023). Many of these farms use a comprehensive data management and reporting tool called Fourth Quadrant (2023) which were used to obtain data from farmers. Data points included dairy cattle numbers, milk production, animal movement practices, total nitrogen fertilizer application rate, fodder production and herbage growth rates. Raising heifers within each farming system was not general practice across the various farming systems. Therefore, in order to create a dataset that is representative of the entire pasture-based dairy farming system, and to allow for suitable comparisons between farms, observations were removed from the dataset based on the following criteria: (i) where the heifer proportion of the entire herd was <17% (too few heifers for replacement); (ii) where the average heifer weights were <150 kg and the proportion <30% (for the case where the farming system is raising a lot of young heifers and the older heifers are not within the system of the collected data); and (iii) where the dry cow proportion was <4.5% thereby assuming the dry cows were managed on separate land outside of the farming system of the collected data. As farmers complete the datasets themselves, for example in Fourth Q, data screening to ensure data quality was done manually by Trace & Save, which forms part of the operational and professional services provided to farmers, and if there were any discrepancies, the farmers were contacted for clarification.
Farm-gate life-cycle assessment of GHG emissions
Greenhouse gas emissions were assessed and categorized as specified by the FAO's Global Livestock Environmental Assessment Model (GLEAM) “cradle-to-gate” emissions (Food and Agriculture Organization, 2021), as well as the IPCC's 2019 refinements to the 2006 IPCC Guidelines for National Greenhouse Gas Inventories (International Panel on Climate Change, 2019a). Direct emissions included enteric fermentation and manure management, pasture, and crop production parameters (i.e., N fertilizer usage; International Panel on Climate Change, 2006a,b, 2019a,b; Du Toit et al., 2013), and fossil fuel usage (International Panel on Climate Change, 2006c; Department of Environmental Affairs, 2017; Figure 1). Indirect emissions included electricity usage, purchased feed production (Food and Agriculture Organization, 2018; Blignaut et al., 2019; Food Agriculture Organization, 2020a,b), fertilizer and pesticide production (Audsley et al., 2009; Kool et al., 2012), transport (Department of Environment, Food and Rural Affairs and Department of Energy and Climate Change, 2022), and embedded energy (Food and Agriculture Organization, 2018; Figure 2). The physical allocation method is used to allocate emissions between milk and meat production as recommended by the International Dairy Federation (2015). Since the assessment was carried out at the farm gate, the functional unit to assess GHG emissions was a kilogram of fat- and protein-corrected milk (International Dairy Federation, 2015). Therefore, to establish the carbon footprint and GHG emissions of the dairy products from the farming systems, the amount of carbon dioxide equivalents per kilogram of fat- and protein-corrected milk produced (kg CO2eq kg−1 FPCM) were determined. According to the IPCC Sixth Assessment Report (International Panel on Climate Change, 2021), 1 kg of CH4 is calculated as 27 kg of CO2eq and 1 kg of N2O is calculated as 273 kg of CO2eq.
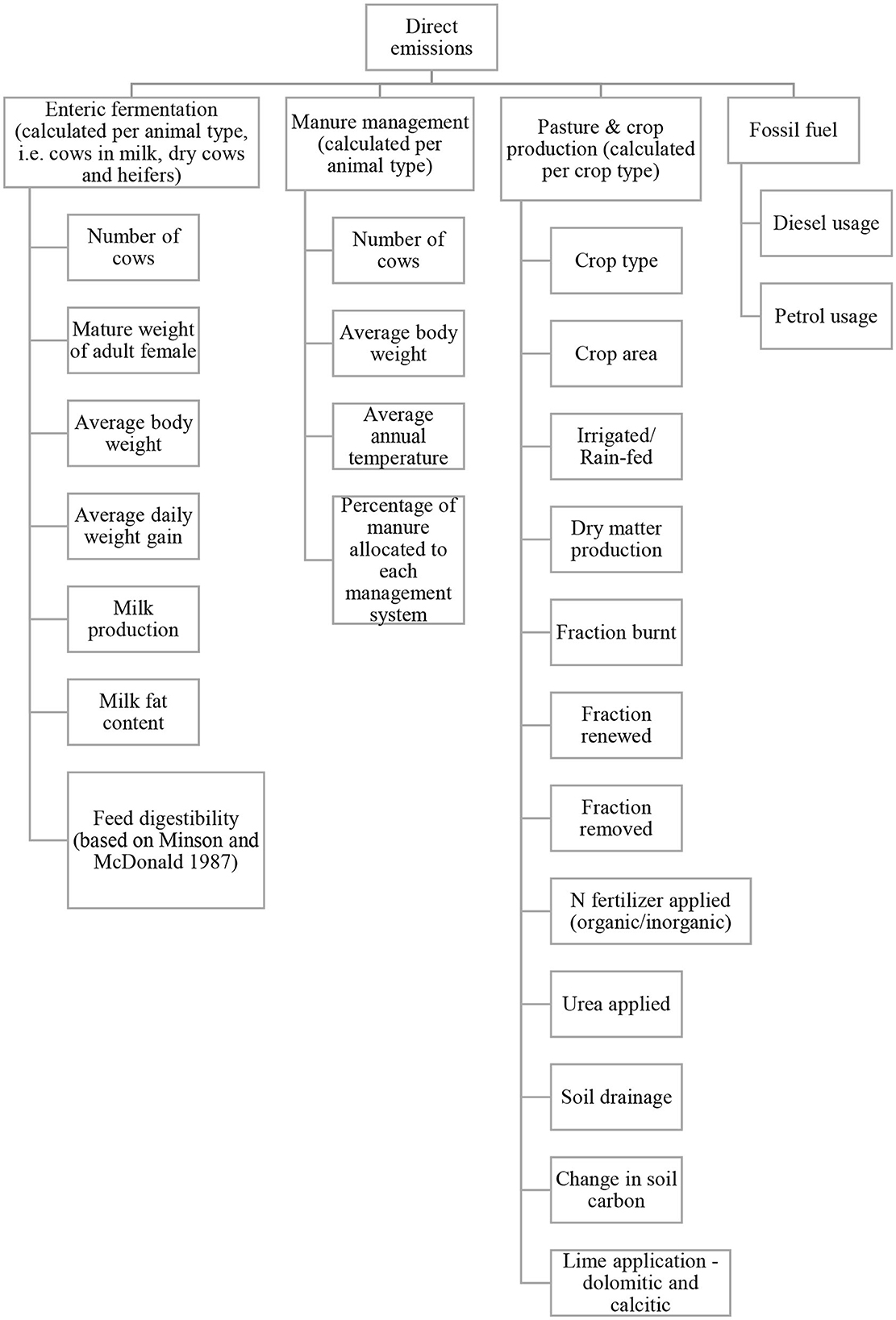
Figure 1. Life-cycle inventory flow diagram indicating the direct GHG emissions datapoints collected from each dairy farming system to determine the emissions per source.
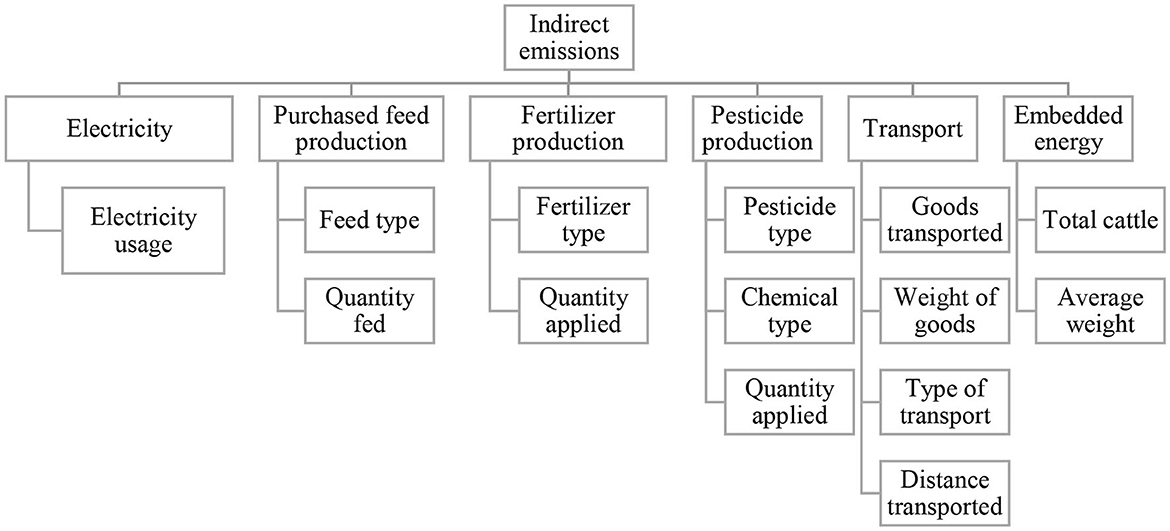
Figure 2. Life-cycle inventory flow diagram indicating the indirect GHG emissions datapoints collected from each dairy farming system to determine the emissions per source.
While we acknowledge the importance of assessing the impact of dairy farming per unit area of land that is used for production, this is however outside the scope of this study and should be investigated in future.
Data processing and statistical analyses
To explore the range of GHG emissions and carbon footprints resulting from pasture-based dairy farming practices in South Africa, the minimum, maximum and the average emissions per tercile was calculated per emission source. The terciles were created by grouping the observations into thirds, i.e., a lowest third, middle third and top third of emissions per source. The average amount of GHG emissions was then calculated per tercile per source.
Partial productivity measures were used to explore the underlying effects of the crop and livestock management practices on the carbon footprint within the dairy farming systems. Spearman's correlations (the data were not normally distributed, Shapiro-Wilk = 0.826; P < 0.001) were carried out between GHG emissions and the partial productivity measures. The partial productivity measures were further used to investigate the similarities between farming systems characterized by vastly different carbon footprints (high vs. low). The average level of all the partial productivity measures was calculated for farming systems with the lowest carbon footprint (i.e., the observations with the lowest 15% of kg CO2eq kg−1 FPCM), and the farms with the highest carbon footprints (i.e., the observations with the highest 15% of kg CO2eq kg−1 FPCM). A Student's t-test was used to test for differences between the average partial productivity measures for the lowest and highest carbon footprint groups (α = 0.05).
Results and discussion
Carbon footprint of pasture-based dairy farming systems in SA
The average carbon footprint across all farming systems was 1.36 ± 0.21 kg CO2eq kg−1 FPCM, which was in alignment with (Meissner and Ohlhoff, 2022) who reported GHG emissions from milk production in South Africa range between 1.2 and 1.4 kg CO2eq kg−1 milk. Smit et al. (2021) measured GHG emissions directly from soil in response to N fertilizer treatments in the Western Cape province of South Africa and reported a carbon footprint of 1.3 kg CO2eq kg−1 energy corrected milk, for treatments receiving <200 kg N ha−1 year−1, and a maximum carbon footprint of 2.6 kg CO2eq kg−1 ECM when 800 kg N ha−1 year−1 was applied. Compared to similar cradle-to-farmgate life-cycle assessment of GHG emissions studies performed outside South Africa, the average carbon footprint found in our assessment was greater. For example, the average GHG emissions from pasture-based dairy farms in Ireland was 1.11 kg CO2eq kg−1 FPCM, with a range of 0.87–1.72 kg CO2eq kg−1 FPCM (O'Brien et al., 2014). In New Zealand (Chobtang et al., 2017), reported average GHG emissions of 0.8 kg CO2eq kg−1 FPCM based on 53 pasture-based dairy farming systems, while (Christie, 2019) reported a value of 1.04 kg CO2eq kg−1 FPCM based on 41 farming systems in Australia. Similarly, the average GHG emissions from dairy farms representing various sizes and management practices in six regions of the United States of America was 1.01 ± 0.09 kg CO2eq kg−1 FPCM (Rotz et al., 2021). When compared to the global carbon footprint for milk production in 2015, the carbon footprint of pasture-based dairy farming systems in South Africa was 55.5% lower and fairly similar to dairy farming systems located in the Oceania region (Food and Agriculture Organization and Global Dairy Platform, 2018).
GHG emission sources
The contribution of each emission source to the carbon footprint are shown in Table 1. Exploring these factors provides insights to understanding the contributing sources of GHG emissions and designing management to appropriately manage or mitigate them on farm-level (Mazzetto et al., 2022). Enteric fermentation had the largest influence on the carbon footprint, coinciding with the findings of Pirlo et al. (2014) in Italy. In addition, Du Toit et al. (2013) found that the average CH4 emitted per head of cattle was 71.8 kg CH4 yr−1 in South African pasture-based farming systems. Methane production is unavoidable in farming systems with ruminants, as the release of CH4 is a by-product during enteric fermentation and allows dairy cattle to absorb protein and fatty acids from roughages. Several strategies to reduce enteric CH4 emissions have been proposed thus far (Galloway et al., 2018b), mainly focusing on breeding, feeding, and dietary supplements (de Boer et al., 2011). Increasing the ratio of concentrates over roughages may reduce enteric CH4 emissions, however, the additional N2O and CO2 emissions associated with the transport and production of higher volumes of concentrates may limit the total net GHG reduction. Importantly, including forages like white clover (Trifolium repens) or chicory (Cichorium intybus) in the diets of dairy cows may reduce enteric CH4 emissions. Although white clover produce very low levels of tannins in leaves (Kagan, 2021), some tannins are found in the flowers and seeds coats. Previous breeding attempts to increase tannin levels in the foliage of white clovers has been unsuccessful, although progress has been made (Roldan et al., 2022). Tannins have been found to affect methanogenesis (Haque, 2018) by inhibiting the growth and activity of the methanogen population, however, reducing enteric CH4 production using tannins has been inconsistent (Bodas et al., 2012; Cieslak et al., 2013; Ku-Vera et al., 2020).
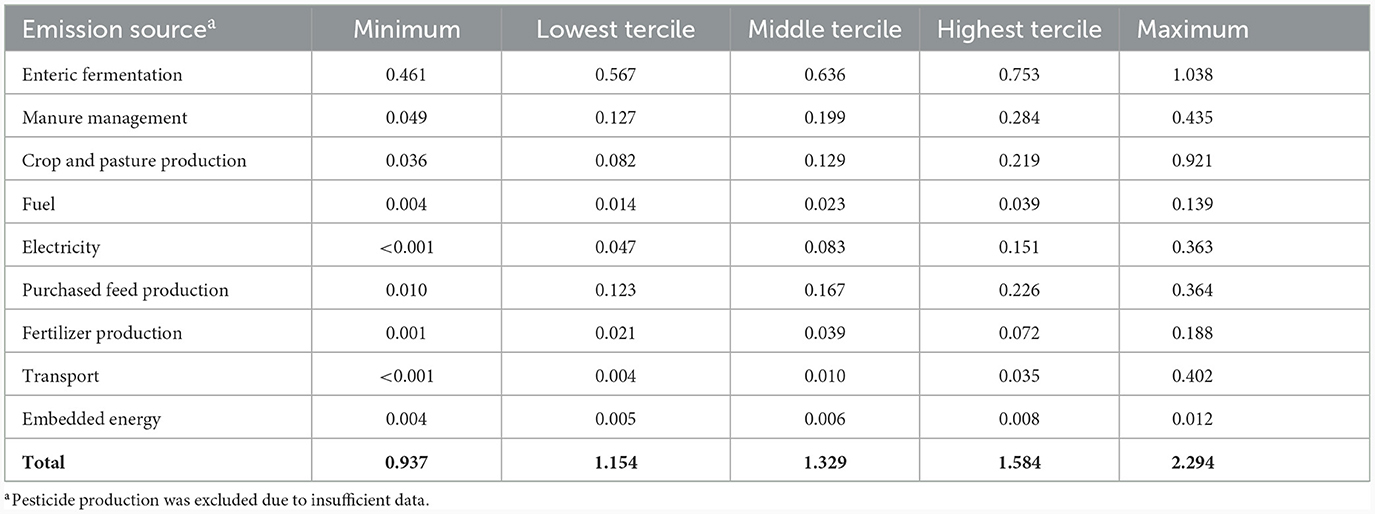
Table 1. Minimum, maximum and average GHG emissions (kg CO2eq kg−1 fat- and protein corrected milk) per tercile (n = 119) per emissions source from 357 observations on 82 pasture-based dairy farming systems in South Africa.
White and red (T. pratense) clovers and chicory often form part of the species-composition for dairy pastures in the Western and Eastern Cape provinces (Viljoen et al., 2020), as they are adapted to the cooler weather experienced along coastal production areas. Nitrogen fixation by clover enhances soil fertility, a crucial benefit for productive dairy pastures under intensive grazing conditions with dairy cows (Botha et al., 2008a). Nonetheless, two limitations associated with clover inclusion are often encountered by farmers: suboptimal establishment and reduced herbage production, particularly when contrasted with grass species. Notably, these limitations are not commonly observed with chicory, underscoring the significance of meticulous forage species selection to optimize pasture productivity and sustainability (Botha et al., 2008b; Van der Colf, 2011) within the context of low carbon footprint dairy farming.
Apart from enteric fermentation, significant contributors to the carbon footprint also included manure management, crop and pasture production, fertilizer and water management, electricity and purchased feed production. Cattle manure is a source for CH4, N2O, ammonia (NH3) gas and urea, and limited management strategies are available to reduce manure GHG (Pirlo et al., 2014). Improving the N use efficiency of milk not only reduces the amount of N emitted from manure (and N losses to the environment), but also reduces unnecessary costs such as feeds and N fertilizers. The application of N fertilizers to promote pasture productivity produces significant quantities of nitrogenous gasses like N2O and NH3, highlighting the importance of implementing site-specific management practices to enhance N use efficiency therefore mitigating these gas emissions.
The relationship between crops, pasture, and livestock management can also mediate various other GHG mitigation pathways, such as soil organic carbon sequestration (Soussana et al., 2010; Paustian et al., 2016; Brewer et al., 2023). Increasing soil organic carbon content provides the opportunity to negate the need for high volumes of inorganic N fertilizers, a characteristic of these intensively managed pastures. Greater soil organic carbon levels lead to improved nutrient cycling in the soil and consequently improved plant-available nutrients such as N, phosphorus, and potassium (Fageria, 2012; Lal, 2015). Indeed, the soil C:N ratio can be taken as proxy of relative soil N availability (Terrer et al., 2019) where a ratio of 15:1 may be used as a threshold between relatively abundant or limited N in soils (Bai et al., 2023). A higher ratio may result in poor soil microbial activity due to the limited N levels present (Lal, 2015). Management practices that improve soil organic carbon content are generally also less intensive on the environment, such as long-term perennial pastures (permanent soil cover and actively growing roots), rotational high-density grazing, optimal fertilizer application rates and minimum- to no-tillage (Badgery et al., 2014; Rutledge et al., 2015; Swanepoel et al., 2015). A recent study by Frasier et al. (2022) highlighted the importance of continuously growing plant roots when restoring and increasing soil organic carbon levels in pasture-dominated systems alongside minimum soil disturbance. Cropping systems with large root inputs such as mixed legume-grass crops (Porwollik et al., 2022) under minimal soil tillage can lead to higher soil carbon content.
Efficient milk production
Table 2 shows the average partial productivity measures for the observations with the lowest and highest GHG emissions. Table 3 provides an overview of the relationship between partial productivity measures and the various sources of GHG emissions. Dairy farming systems with the lowest carbon footprints had an average milk production efficiency of 1,323 L 100 kg−1 live weight, 107 kg solids 100 kg−1 live weight, 20.4 L cow day−1 and 17,650 L ha−1, as opposed to the farming systems with the highest carbon footprints showing an average milk production efficiency of 1,052 L 100 kg−1 live weight, 79 kg solids 100 kg−1 live weight, 17.0 L cow day−1 and 11,909 L ha−1 (Table 2). The opposite effect is implied for higher GHG emissions, where the opposite effect is at play. For example, for influencing factors where a lower value is associated with lower emissions, a higher value for this influencing factor will result in higher emissions. A response in milk efficiency production indicates that the less productive farming systems can reduce their carbon footprint by increasing milk production efficiency.
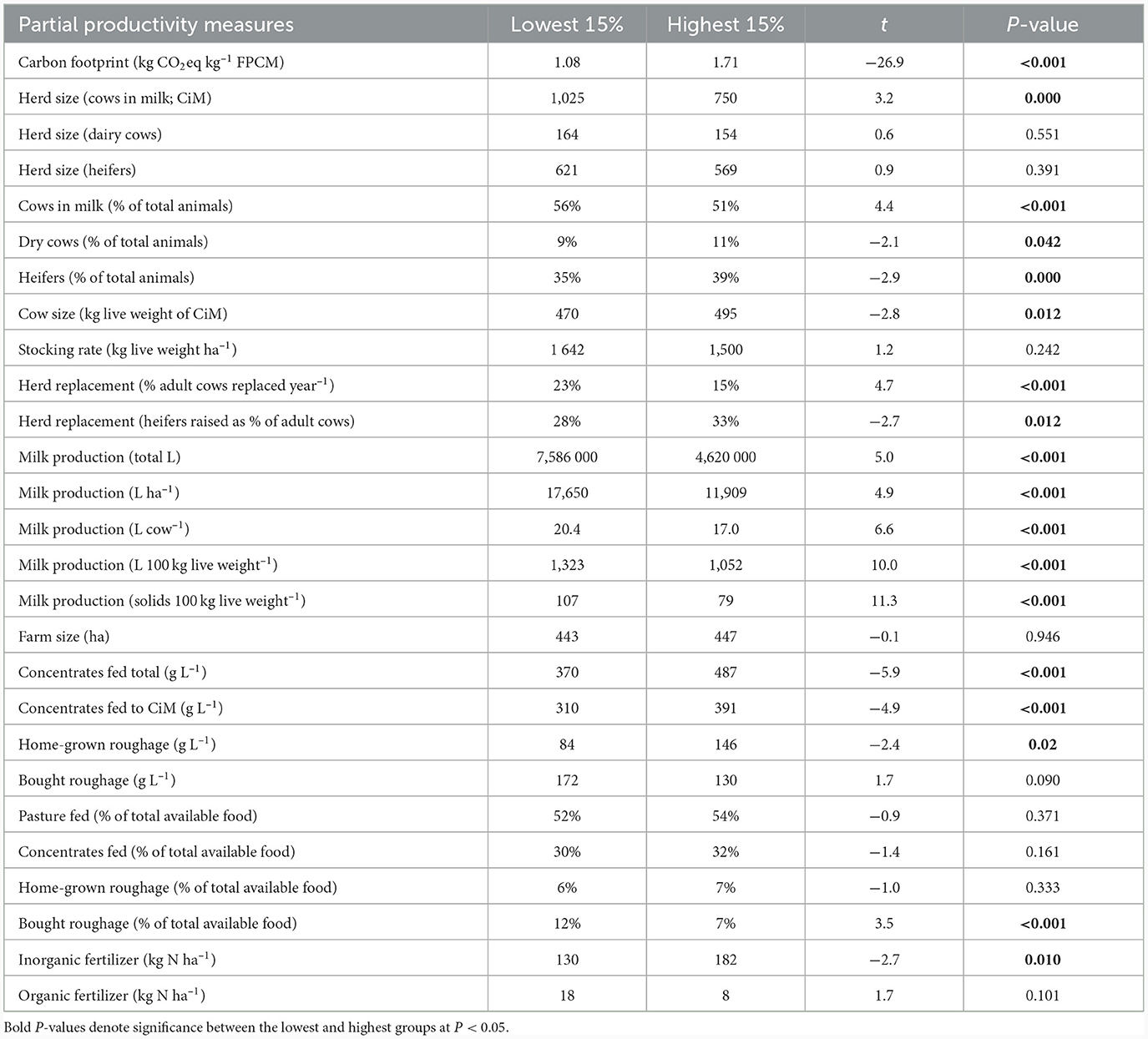
Table 2. The average partial productivity measures for the 357 observations on 82 pasture-based dairy farming systems in South Africa representing the highest (n = 53) and lowest (n = 53) 15% of GHG emissions (kg CO2eq) per kilogram of fat- and protein-corrected milk (FPCM), and the student t-test results indicating significant differences between the average measures of the two groups.
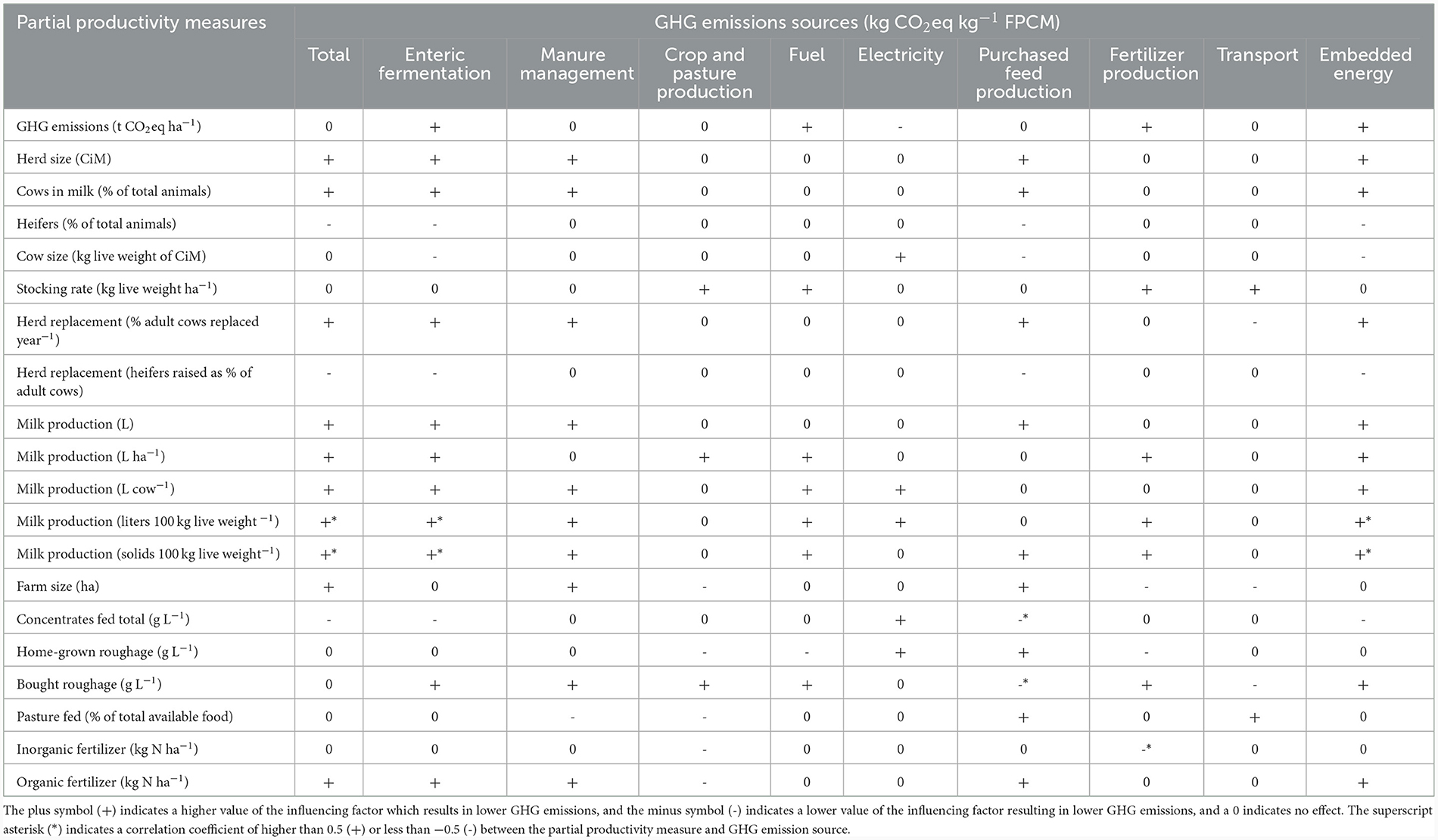
Table 3. The relationship between partial productivity indicators and GHG emissions (kg CO2eq kg−1 FPCM) based on Spearman correlations for 357 observations on 82 pasture-based dairy farming systems in South Africa.
In our assessment, the highest correlation coefficients were the negative correlation between total GHG emissions and milk production efficiency (milk solids per 100 kg−1 live weight: r = −0.58, P < 0.001; liters of milk produced 100 kg−1 live weight: r = −0.53, P < 0.001; Table 4). Efficient milk production is a critical factor to reduce GHG emissions from pasture-based dairy farming systems (Wall et al., 2009). Also negatively correlated, but with lower coefficients, are GHG emissions and milk production per hectare and per cow (Table 4). This is aligned with previous studies, which have found the same relationship between higher milk production efficiency and lower carbon footprints (O'Brien et al., 2014; Morais et al., 2018; Lorenz et al., 2019).
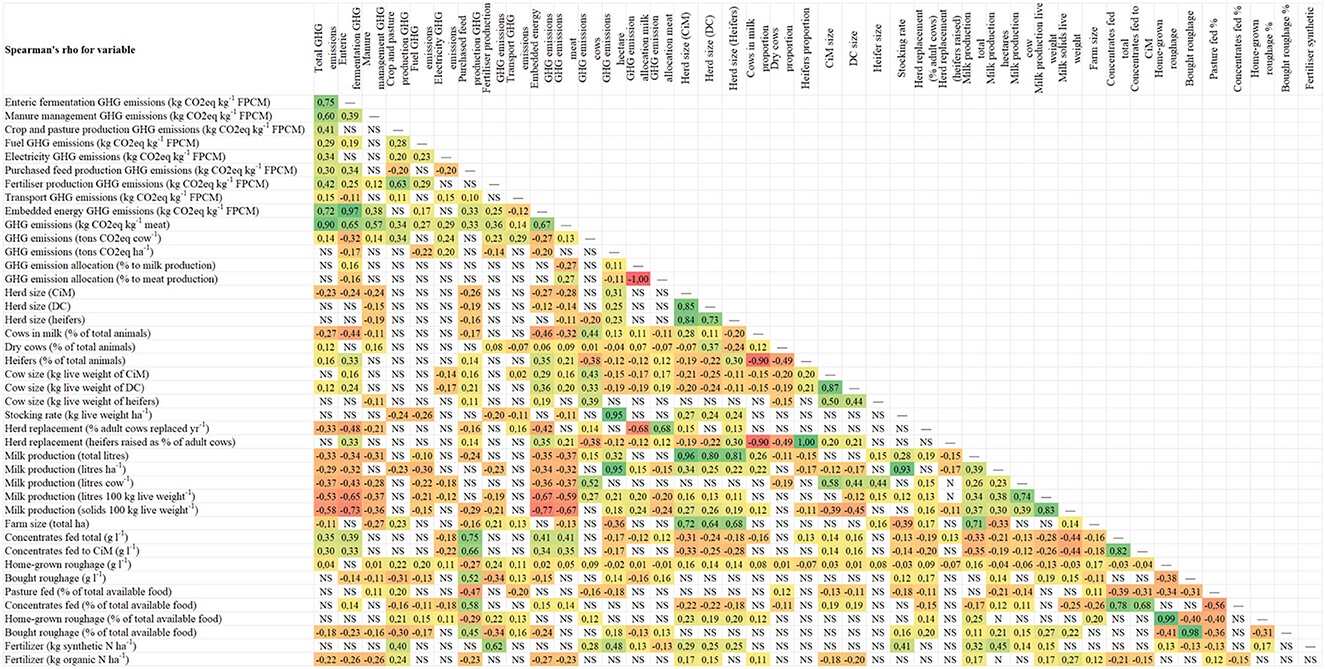
Table 4. Correlation results between all the variables investigated in the life cycle assessment of 82 pasture-based dairy farming systems in South Africa.
Breeding efficiency has a large impact on milk production efficiency (Clark et al., 2007; Capper et al., 2009). To avoid inefficient milk production and hence greater carbon footprints, breeding management systems should be established to ensure effective heat-spotting and artificial insemination to guarantee efficient breeding strategies. Additional management factors influencing milk production efficiency include optimal health care, the selection of ideal cow genetics for the farm system and climate, and correct ration formulation (Clark et al., 2007; Capper et al., 2009).
Despite the importance of milk production efficiency to reduce GHG emissions per milk production (Yan et al., 2010), dairy farmers should caution to maximize milk production thoughtlessly. Additional environmental and profitability factors should also be accounted for when considering the overall sustainability of pasture-based dairy production. For example, pasture herbage utilization per hectare is a critical indicator of profitability on pasture-based dairy farms (Hanrahan et al., 2018). In this study higher pasture percentage of the total diet was negatively correlated with cow size (r = −13, P = 0.01) and milk production per cow (r = −14, P = 0.01) with no correlation to milk production per 100 kg liveweight (liters and solids). A higher percentage of pasture in the diet for smaller cows is probably due to the selection of smaller cows for grazing systems, where cows are expected to walk longer distances and spend more time grazing, which is better suited to smaller animals. The negative correlation between pasture percentage in the diet and milk production per cow could be an indirect association to the cow size factor, where smaller cows would produce a smaller amount of milk per day, than larger cows which are fed a higher concentrate and roughage diet to supplement pasture intake. The lack of correlation between percentage of pasture intake and milk production per 100 kg liveweight indicates that the other associations discussed in this paragraph are not indicative of milk production efficiency. There are other management factors which contribute to the efficiency of milk production (O'Brien et al., 2014) when assessed per liveweight, rather than per cow. There is a negative correlation between milk production per 100 kg and concentrates fed (g l−1), both in total and to cows in milk. The association between milk production efficiency and feed conversion efficiency (Beever and Doyle, 2007) is further indicative that the difference between farms is related to management practices rather than specific farm systems, as it relates to cow size and percentage of pasture in the diet.
Herd dynamics
A negative correlation was found between the proportion of cows in milk on the farm, and total GHG emissions (Table 4). Also, a positive correlation was found between the proportion of heifers on the farm, and the herd replacement rate (heifers being raised on the farm as a percentage of adult cows) and total GHG emissions. This was expected, as the carbon footprint assessment calculations are mostly influenced by the emission sources like enteric fermentation and manure management from animals, which is divided by total milk production. Therefore, the more milk producing animals there are proportionately on the farm, the lower the emissions will be, explaining why dairy farmers aim to raise a greater number of heifers than they require for replacement in the future (O'Brien et al., 2010; Zehetmeier et al., 2014).
The herd replacement rate (percentage of adult cows replaced each year) is negatively correlated with total GHG emissions (Table 4), which contrasts with other studies reporting no correlation with replacement rate and carbon footprint (O'Brien et al., 2014; Lorenz et al., 2019). An explanation for this is that a higher herd replacement results in higher meat production from the farm, and there is therefore a higher allocation of emissions to meat production in these farming systems, as indicated by the negative correlation between GHG emissions allocation to meat production and GHG emissions from enteric fermentation (Table 4). In addition, herd replacement rate was positively correlated with milk production efficiency (liters and solids per 100 kg liveweight) which may serve as an indication that the dairy farmers with lower herd replacement rates may not be culling inefficient cows, while farming systems with higher production efficiency showcase a greater rate at removing unproductive and inefficient cows.
There was a positive correlation between concentrates fed (g L−1 milk produced) and total GHG emissions (Table 4). Also, there was a negative correlation between concentrates fed and all milk production efficiency measures. Importantly, farming systems should not aim to increase milk production efficiency by feeding higher amounts of concentrates. From Table 2 it is clear that farms with lower emissions are more efficient at converting concentrates into milk (310 g L−1 to CiM) than farms with higher emissions (391 g L−1 to CiM; P < 0.001). Interestingly there were no significant correlations observed between total GHG emissions and pasture percentage, bought roughage (g L−1) and home-grown roughage (g L−1; Table 4). Also, no differences were found between the lowest and highest GHG emissions farms for pasture, concentrate and home-grown roughage percentage in the diet (Table 2). The only significant difference was found for the percentage of bought roughage between the lowest (12%) and highest (7%) emission farms. This was associated with the positive correlation between bought roughage (g L−1 and % of diet) and milk production efficiency measures as stated in Table 4. It is imperative to provide lactating animals with sufficient and well-balanced diet (de Boer et al., 2011). Efficient feed conversion is also affected by the daily storage and handling of feeds, where feed losses should be limited. From the animal perspective, many factors influence effective feed conversion (Clark et al., 2007; Capper et al., 2009) such as selective breeding to improve the feed conversion of pasture biomass.
No correlation was found between stocking rate and total GHG emissions, although a positive correlation was found between stocking rate and GHG emissions per hectare (r = 0.95, P < 0.001; Table 4). Since the focus of this assessment is GHG emissions per unit of milk production and not per hectare, this will be discussed in a separate paper.
Inorganic nitrogen management
Despite no significant correlation between inorganic nitrogen (N) fertilizer and total GHG emissions (Table 3), a difference (P < 0.05) was found for inorganic N fertilizer between dairy farming systems with the lowest and highest GHG emissions (Table 2). On average, the farms with the highest GHG emissions used 30% more inorganic N fertilizers per hectare than the farms with the lowest GHG emissions. In addition, a positive correlation was found between inorganic N fertilizer usage and GHG emissions from crop and pasture, and fertilizer production. Dairy farmers use high amounts of nitrate- and urea-based N fertilizers to increase pasture productivity (Swanepoel et al., 2014a) with greater amounts of GHG emissions associated with higher N fertilizer usage. The primary aim of these pasture systems is to achieve high biomass yields and allowing the expression of the genetic production potential of the species found in the pasture in response to the prevailing environmental conditions. In addition, soil quality and health play a pivotal role to ensure high production rates and quality herbage in dairy pastures over the long term (Swanepoel et al., 2014b), which is directly influenced by soil tillage practices (Swanepoel et al., 2015) and nitrogen fertilizer management (Galloway et al., 2018a; Phohlo et al., 2022). Improved soil health can therefore provide pathways to lower N fertilizer usage and the carbon footprint of a pasture-based dairy farming system. Healthier soils can provide ecosystem services such as efficient nutrient cycling, sustainable N mineralization and sustain soil microbial activities (Verhulst et al., 2010). These ecosystem roles can support productive crop and pasture growth, negating the need for high amounts of inorganic N fertilizers on a regular basis (Bolan et al., 2004; Monaghan et al., 2007).
As this study focused on the carbon footprint of South African pasture-based dairy farming systems, it is important to note that additional management practices, which are not discussed in this paper, can further promote the sustainability of these systems. Examples of these practices are improved effluent and water management (Zonderland-Thomassen and Ledgard, 2012; Sobhi et al., 2021), and optimized grazing and pasture management (O'Brien et al., 2016; Ledgard et al., 2020). These practices influence the abovementioned factors directly and are therefore important to consider when optimizing the sustainability of dairy farming systems.
Outlook for sustainable dairy production and future research agenda
Sustainable dairy production is important to address the high dairy demands in South Africa. Pasture-based dairy farming systems are intensively managed and demand high inputs to ensure a favorable cost-to-income ratio. These complex farming systems require adaptable management approaches whereby all soil, crop and livestock in-field activities and challenges are considered (Van der Laan et al., 2017). The soil and crop management principles of regenerative agriculture have been proposed as an adaptable approach to address the soil erosion and degradation effects of high-input conventional management practices (Haarhoff et al., 2020; Musto et al., 2023). These regenerative agriculture practices include minimum tillage, growing multi-species perennial pastures, effective inorganic fertilizer management and optimal grazing management (Khangura et al., 2023). Building soil organic carbon is central to the regenerative agriculture management approach, thereby inter alia increasing the soil water holding capacity and improving overall microbial activity which in turn promotes the available ecosystem services and functions (Verhulst et al., 2010).
To address sustainability challenges for South Africa pasture-based dairy farming systems, scientific research is needed to provide dairy farmers, researchers, and extension workers with an improved decision-making roadmap. Current knowledge gaps were identified, and the following future research agenda is proposed for pasture-based dairy farming systems in South Africa.
• Explore modeling approaches to assess the economic and environmental impact of dairy production in South Africa, offering a holistic understanding of the system dynamics while also quantifying net carbon emissions or sinks, including the evaluation of carbon storage potential and sequestration capacities within the dairy production systems.
• Assess GHG emissions not only per unit area (hectare), but also use alternative metrics such as emissions per unit of milk produced, per cow, per kilogram of forage consumed, per unit of milk product, per operational output, per unit of nutrient cycled on the farm (e.g., N), or per productivity or efficiency metric (e.g., milk per hectare). These various metrics can provide a comprehensive understanding of GHG emissions efficiency and sustainability in dairy production systems, contributing to a more holistic assessment of environmental impact and management strategies.
• Formulate management guidelines for regenerative agriculture practices for low carbon economy dairy pasture systems, such as no-tillage and multi-species pastures. Nitrogen management and judicious use of agrochemicals should receive specific attention in the context of regenerative agriculture guidelines.
• Explore alternative feed sources and precision feeding techniques to reduce reliance on inorganic fertilizers.
• Investigate advanced grazing management strategies, such as holistic grazing management of multispecies pastures, to maximize forage utilization, herbage productivity and persistence of multiple species in a mix, while maintaining animal welfare.
• Develop a framework to assess the ecological impact of current and alternative pasture and livestock management practices, with particular emphasis on biodiversity, water quality of nearby water bodies, soil organic carbon levels and ecosystem services.
• Analyse consumer preferences and market opportunities for sustainably produced dairy products, emphasizing regenerative agriculture practices.
Conclusion
In this study we have assessed the carbon footprint of 82 pasture-based dairy farming systems across South Africa representing data from 2012 until 2022. These dairy farming systems are highly complex and require intensive livestock, soil and pasture management to sustain profitable production. The average carbon footprint across all farming systems was 1.36 ± 0.21 kg CO2eq kg−1 FPCM, which is slightly greater compared to dairy systems outside South Africa. Enteric fermentation had the largest influence on the carbon footprint, as high volumes of CH4 are associated with ruminants. Other significant contributors to the carbon footprint included manure management, crop and pasture production, electricity and purchased feed production, highlighting the opportunities for improving the carbon footprint of these systems. It was clear that efficient milk production is a critical factor to reduce GHG emissions from pasture-based dairy farming systems. Farming systems with high GHG emissions were characterized by greater N fertilizer usage, indicating alternative N fertilizer management strategies are required to improve pasture N uptake and utilization. In order to address all the complexities and demands within pasture-based dairy farming systems, an adaptable management system is required where each management practice can be tailored according to the prevailing soil and climatic conditions, farming system challenges and advantages.
Data availability statement
The raw data supporting the conclusions of this article will be made available by the authors, without undue reservation.
Author contributions
PS: Investigation, Validation, Visualization, Writing – review & editing. CG: Conceptualization, Data curation, Formal analysis, Funding acquisition, Investigation, Methodology, Project administration, Software, Visualization, Writing – original draft. SH: Conceptualization, Formal analysis, Methodology, Visualization, Writing – review & editing.
Funding
The author(s) declare that no financial support was received for the research, authorship, and/or publication of this article.
Acknowledgments
The role of the Trace and Save and Woodlands Dairy Sustainability Project teams in collecting and screening the data is acknowledged and appreciated.
Conflict of interest
CG was employed by Trace and Save. SH was employed by Climate Neutral Group South Africa.
The remaining author declares that the research was conducted in the absence of any commercial or financial relationships that could be construed as a potential conflict of interest.
Publisher's note
All claims expressed in this article are solely those of the authors and do not necessarily represent those of their affiliated organizations, or those of the publisher, the editors and the reviewers. Any product that may be evaluated in this article, or claim that may be made by its manufacturer, is not guaranteed or endorsed by the publisher.
References
Audsley, E., Stacey, K. F., Parsons, D. J., and Williams, A. G. (2009). Estimation of the Greenhouse Gas Emissions From Agricultural Pesticide Manufacture and Use. Cranfield; Bedford: Cranfield University.
Badgery, W. B., Simmons, A. T., Murphy, B. W., Rawson, A., Andersson, K. O., and Lonergan, V. E. (2014). The influence of land use and management on soil C levels for crop pasture systems in Central New South Wales, Australia. Agric. Ecosyst. Environ. 196, 147–157. doi: 10.1016/j.agee.2014.06.026
Bai, T., Wang, P., Qiu, Y., Zhang, Y., and Hu, S. (2023). Nitrogen availability mediates soil carbon cycling response to climate warming: a meta-analysis. Glob. Change Biol. 29, 2608–2626. doi: 10.1111/gcb.16627
Bartl, K., Gómez, C. A., and Nemecek, T. (2011). Life cycle assessment of milk produced in two smallholder dairy systems in the highlands and the coast of Peru. J. Clean Prod. 19, 1494–1505. doi: 10.1016/j.jclepro.2011.04.010
Beever, D. E., and Doyle, P. T. (2007). Feed conversion efficiency as a key determinant of dairy herd performance: a review. Austr. J. Exp. Agri. 47, 645–657. doi: 10.1071/EA06048
Blignaut, A., Vienings, E., Saywood, K., Nel, A., Smith, H., and Fourie, P. (2019). Appendix 6: Annual Progress Report: Carbon Footprint of Maize, Phase 1. Project title: Determining the Carbon Footprint of Maize for Different Farming Systems in South Africa's Summer Rainfall Regions. Pretoria: The Maize Trust.
Bodas, R. N., Prieto, N. R., Garcia-Gonzalez, R. S., Andres, S., Giraldez, F. J., and Lopez, S. (2012). Manipulation of rumen fermentation and methane production with plant secondary metabolites. Anim. Feed Sci. Technol. 176, 78–93. doi: 10.1016/j.anifeedsci.2012.07.010
Bolan, N. S., Saggar, S., Luo, J., Bhandral, R., and Singh, J. (2004). Gaseous emissions of nitrogen from grazed pastures: processes, measurements and modeling, environmental implications, and mitigation. Adv. Agron. 84:120. doi: 10.1016/S0065-2113(04)84002-1
Botha, P. R., Meeske, R., and Snyman, H. A. (2008a). Kikuyu over-sown with ryegrass and clover: dry matter production, botanical composition and nutritional value. Afri. J. Range Forage Sci. 25, 93–101. doi: 10.2989/AJRF.2008.25.3.1.598
Botha, P. R., Meeske, R., and Snyman, H. A. (2008b). Kikuyu over-sown with ryegrass and clover: grazing capacity, milk production and milk composition. Afri. J. Range Forage Sci. 25, 103–110. doi: 10.2989/AJRF.2008.25.3.2.599
Brewer, K. M., Muñoz-Araya, M., Martinez, I., Marshall, K. N., and Gaudin, A. C. (2023). Long-term integrated crop-livestock grazing stimulates soil ecosystem carbon flux, increasing subsoil carbon storage in California perennial agroecosystems. Geoderma 438:116598. doi: 10.1016/j.geoderma.2023.116598
Capper, J. L., Cady, R. A., and Bauman, D. E. (2009). The environmental impact of dairy production: 1944 compared with 2007. J. Anim. Sci. 87, 2160–2167. doi: 10.2527/jas.2009-1781
Chobtang, J., Ledgard, S. F., McLaren, S. J., and Donaghy, D. J. (2017). Life cycle environmental impacts of high and low intensification pasture-based milk production systems: a case study of the Waikato region, New Zealand. J. Clean. Prod. 140, 664–674. doi: 10.1016/j.jclepro.2016.06.079
Christie, K. M. (2019). Greenhouse Gas Emissions and Potential Mitigation Options for the Australian Dairy Industry (Ph.D. thesis). University of Tasmania, Hobart, TAS, Australia.
Cieslak, A., Szumacher-Strabel, M., Stochmal, A., and Oleszek, W. (2013). Plant components with specific activities against rumen methanogens. Animal 7, 253–265. doi: 10.1017/S1751731113000852
Clark, D. A., Caradus, J. R., Monaghan, R. M., Sharp, P., and Thorrold, B. S. (2007). Issues and options for future dairy farming in New Zealand. N. Zeal. J. Agri. Res. 50, 203–321. doi: 10.1080/00288230709510291
de Boer, I. J., Cederberg, C., Eady, S., Gollnow, S., Kristensen, T., Macleod, M., et al. (2011). Greenhouse gas mitigation in animal production: towards an integrated life cycle sustainability assessment. Curr. Opin. Environ. Sustain. 3, 423–431. doi: 10.1016/j.cosust.2011.08.007
Department of Environment, Food and Rural Affairs and Department of Energy and Climate Change. (2022). Greenhouse Gas Reporting: Conversion Factors 2022. London.
Department of Environmental Affairs (2017). Technical Guidelines for Monitoring, Reporting and Verification of Greenhouse Gas Emissions by Industry. A Companion to the South African National GHG Emission Reporting Regulations. Pretoria.
Department of Forestry, Fisheries and the Environment (2021). Republic of South Africa. South Africa's 4th Biennial Update Report to the United Nations Framework Convention on Climate Change. Pretoria.
Du Toit, C. J., Meissner, H. H., and Van Niekerk, W. A. (2013). Direct methane and nitrous oxide emissions of South African dairy and beef cattle. South Afri. J. Anim. Sci. 43, 320–339. doi: 10.4314/sajas.v43i3.7
Fageria, N. K. (2012). Role of soil organic matter in maintaining sustainability of cropping systems. Commun. Soil Science Plant Anal. 43, 2063–2113. doi: 10.1080/00103624.2012.697234
Flysjö, A, Cederberg, C., Henriksson, M., and Ledgard, S. F. (2011). How does co-product handling affect the carbon footprint of milk? Case study of New Zealand and Sweden. Int. J. Life Cycle Assess. 16, 420–430. doi: 10.1007/s11367-011-0283-9
Food and Agriculture Organization (2010). Greenhouse Gas Emissions From the Dairy Sector. A Life Cycle Assessment. In: Report: Animal Production and Health Division. Rome: FAO.
Food and Agriculture Organization (2018). Global Livestock Environmental Assessment Model. Version 2.0. Model Description. Rome: FAO.
Food and Agriculture Organization (2020a). GLEAM App. Available online at: https://gleami.apps.fao.org/input (accessed June 15, 2023).
Food and Agriculture Organization (2020b). Global Database of GHG Emissions Related to Feed Crops. Available online at: http://www.fao.org/partnerships/leap/database/ghg-crops/en/ (accessed June 15, 2023).
Food and Agriculture Organization (2021). Global Livestock Environmental Assessment Model (GLEAM). Available online at: http://www.fao.org/gleam/modeldescription/en/ (accessed May 8, 2023).
Food and Agriculture Organization and Global Dairy Platform (2018). Climate Change and the Global Dairy Cattle Sector - The Role of the Dairy Sector in a Low-Carbon Future. Food and Agriculture Organization and Global Dairy Platform. Available online at: https://dairysustainabilityframework.org/wp-content/uploads/2019/01/Climate-Change-and-the-Global-Dairy-Cattle-Sector.pdf (accessed September 29, 2022).
Fourth Quadrant (2023). Available online at: http://fourthq.co.za/ (accessed May 20, 2023).
Frasier, I., Barbero, F., Perez Brandan, C., Gómez, M. F., Fernandez, R., Quiroga, A., et al. (2022). Roots are the key for soil C restoration: a comparison of land management in the semiarid argentinean pampa. Soil Tillage Res. 235:105918. doi: 10.1016/j.still.2023.105918
Galloway, C., Conradie, B., Prozesky, H., and Esler, K. (2018a). Are private and social goals aligned in pasture-based dairy production? J. Clean. Prod. 175, 402–408. doi: 10.1016/j.jclepro.2017.12.036
Galloway, C., Conradie, B., Prozesky, H., and Esler, K. (2018b). Opportunities to improve sustainability on commercial pasture-based dairy farms by assessing environmental impact. Agricult. Syst. 166, 1–9. doi: 10.1016/j.agsy.2018.07.008
Guest, G., Smith, W., Grant, B., VanderZaag, A., Desjardins, R., and McConkey, B. (2017). A comparative life cycle assessment highlighting the trade-offs of a liquid manure separator-composter in a Canadian dairy farm system. J. Clean. Prod. 143, 824–835. doi: 10.1016/j.jclepro.2016.12.041
Haarhoff, S. J., Kotzé, T. N, and Swanepoel, P. A. (2020). A prospectus for sustainability of rainfed maize production systems in South Africa. Crop Sci. 60, 14–28. doi: 10.1002/csc2.20103
Hanrahan, L., McHugh, N., Hennessy, T., Moran, B., Kearney, R., Wallace, M., et al. (2018). Factors associated with profitability in pasture-based systems of milk production. J. Dairy Sci. 101, 5474–5485. doi: 10.3168/jds.2017-13223
Haque, M. N. (2018). Dietary manipulation: a sustainable way to mitigate methane emissions from ruminants. J. Anim. Sci. Technol. 60, 1–10. doi: 10.1186/s40781-018-0175-7
Hietala, S., Heusala, H., Katajajuuri, J. M., Järvenranta, K., Virkajärvi, P., Huuskonen, A., et al. (2021). Environmental life cycle assessment of Finnish beef-cradle-to-farm gate analysis of dairy and beef breed beef production. Agric. Syst. 194:103250. doi: 10.1016/j.agsy.2021.103250
International Dairy Federation (2015). A Common Carbon Footprint Approach for the Dairy Sector. The IDF Guide to Standard Life Cycle Assessment Methodology. Brussels: Bulletin of the International Dairy Federation 479/2015.
International Panel on Climate Change (2006a). Guidelines for National Greenhouse Gas Inventories. Chapter 10: Emissions From Livestock and Manure Management. Volume 4: Agriculture, Forestry and Other Land Use. Geneva.
International Panel on Climate Change (2006b). Guidelines for National Greenhouse Gas Inventories. Chapter 11: N2O Emissions From Managed Soils, and CO2 Emissions From Lime and Urea Application. Volume 4: Agriculture, Forestry and Other Land Use. Geneva.
International Panel on Climate Change (2006c). Guidelines for National Greenhouse Gas Inventories. Chapter 2: Stationary Combustion and Chapter 3: Mobile Combustion. Volume 2: Energy. Geneva.
International Panel on Climate Change (2019a). Guidelines for National Greenhouse Gas Inventories. Chapter 10: Emissions From Livestock and Manure Management. Volume 4: Agriculture, Forestry and Other Land Use. Refinement to the 2006 IPCC Guidelines for National Greenhouse Gas Inventories. Geneva.
International Panel on Climate Change (2019b). Guidelines for National Greenhouse Gas Inventories. Chapter 11: N2O Emissions From Managed Soils, and CO2 Emissions From Lime and Urea Application. Volume 4: Agriculture, Forestry and Other Land Use. Refinement to the 2006 IPCC Guidelines for National Greenhouse Gas Inventories. Geneva.
Kagan, I. A. (2021). Soluble phenolic compounds of perennial ryegrass (Lolium perenne L.): potential effects on animal performance, and challenges in determining profiles and concentrations. Anim. Feed Sci. Technol. 277:114960. doi: 10.1016/j.anifeedsci.2021.114960
Khangura, R., Ferris, D., Wagg, C., and Bowyer, J. (2023). Regenerative agriculture - a literature review on the practices and mechanisms used to improve soil health. Sustainability 15:2338. doi: 10.3390/su15032338
Kool, A., Marinussen, M., and Blonk, H. (2012). LCI Data for the Calculation Tool Feedprint for Greenhouse Gas Emissions of Feed Production and Utilization. Gouda: GHG Emissions of N, P and K Fertiliser Production, Blonk Consultants.
Ku-Vera, J. C., Jiménez-Ocampo, R., Valencia-Salazar, S. S., Montoya-Flores, M. D., Molina-Botero, I. C., Arango, J., et al. (2020). Role of secondary plant metabolites on enteric methane mitigation in ruminants. Front. Vet. Sci. 7:584. doi: 10.3389/fvets.2020.00584
Lal, R. (2015). Restoring soil quality to mitigate soil degradation. Sustainability 7, 5875–5895. doi: 10.3390/su7055875
Ledgard, S. F., Falconer, S. J., Abercrombie, R., Philip, G., and Hill, J. P. (2020). Temporal, spatial, and management variability in the carbon footprint of New Zealand milk. J. Dairy Sci. 103, 1031–1046. doi: 10.3168/jds.2019-17182
Lorenz, H., Reinsch, T., Hess, S., and Taube, F. (2019). Is low-input dairy farming more climate friendly? A meta-analysis of the carbon footprints of different production systems. J. Clean. Prod. 211, 161–170. doi: 10.1016/j.jclepro.2018.11.113
Mazzetto, A. M., Falconer, S., and Ledgard, S. (2022). Mapping the carbon footprint of milk production from cattle: a systematic review. J. Dairy Sci. 105, 9713–9725. doi: 10.3168/jds.2022-22117
Meissner, H., and Ohlhoff, C. (2022). Sustainability in the SA Dairy Industry: A Status and Progress Report. Pretoria: Milk SA.
Monaghan, R. M., Hedley, M. J., Di, H. J., McDowell, R. W., Cameron, K. C., and Ledgard, S. F. (2007). Nutrient management in New Zealand pastures-recent developments and future issues. N. Zeal. J. Agri. Res. 50, 181–201. doi: 10.1080/00288230709510290
Morais, T. G., Teixeira, R. F., Rodrigues, N. R., and Domingos, T. (2018). Carbon footprint of milk from pasture-based dairy farms in Azores, Portugal. Sustainability 10:3658. doi: 10.3390/su10103658
Musto, G. A., Swanepoel, P. A., and Strauss, J. A. (2023). Regenerative agriculture v. conservation agriculture: potential effects on soil quality, crop productivity and whole-farm economics in Mediterranean-climate regions. J. Agri. Sci. 2023, 1–11. doi: 10.1017/S0021859623000242
O'Brien, D., Brennan, P., Humphreys, J., Ruane, E., and Shalloo, L. (2014). An appraisal of carbon footprint of milk from commercial grass-based dairy farms in Ireland according to a certified life cycle assessment methodology. Int. J. Life Cycle Assess. 19, 1469–1481. doi: 10.1007/s11367-014-0755-9
O'Brien, D., Geoghegan, A., McNamara, K., and Shalloo, L. (2016). How can grass-based dairy farmers reduce the carbon footprint of milk? Anim. Prod. Sci. 56, 495–500. doi: 10.1071/AN15490
O'Brien, D., Shalloo, L., Grainger, C., Buckley, F., Horan, B., and Wallace, M. (2010). The influence of strain of Holstein-Friesian cow and feeding system on greenhouse gas emissions from pastoral dairy farms. J. Dairy Scie. 93, 3390–3402. doi: 10.3168/jds.2009-2790
Paustian, K., Lehmann, J., Ogle, S., Reay, D., Robertson, G. P., and Smith, P. (2016). Climate-smart soils. Nature 532, 49–57. doi: 10.1038/nature17174
Phohlo, M. P., Swanepoel, P. A., and Hinck, S. (2022). Excessive nitrogen fertilization is a limitation to herbage yield and nitrogen use efficiency of dairy pastures in South Africa. Sustainability 14:4322. doi: 10.3390/su14074322
Pirlo, G., Terzano, G., Pacelli, C., Abeni, F., and Carè, S. (2014). Carbon footprint of milk produced at Italian buffalo farms. Livestock Sci. 161, 176–184. doi: 10.1016/j.livsci.2013.12.007
Porwollik, V., Rolinski, S., Heinke, J., von Bloh, W., Schaphoff, S., and Müller, C. (2022). The role of cover crops for cropland soil carbon, nitrogen leaching, and agricultural yields-a global simulation study with LPJmL. (V. 5.0-tillage-cc). Biogeosciences 19, 957–977. doi: 10.5194/bg-19-957-2022
Roldan, M. B., Cousins, G., Muetzel, S., Zeller, W. E., Fraser K Salminen, J. P., Blanc, A., et al. (2022). Condensed tannins in white clover (Trifolium repens). foliar tissues expressing the transcription factor TaMYB14-1 bind to forage protein and reduce ammonia and methane emissions in vitro. Front. Plant Sci. 12:777354. doi: 10.3389/fpls.2021.777354
Rotz, A., Stout, R., Leytem, A., Feyereisen, G., Waldrip, H., Thoma, G., et al. (2021). Environmental assessment of United States dairy farms. J. Clean. Prod. 315, 128153. doi: 10.1016/j.jclepro.2021.128153
Rutledge, S., Mudge, P. L., Campbell, D. I., Woodward, S. L., Goodrich, J. P., Wall, A. M., et al. (2015). C balance of an intensively grazed temperate dairy pasture over four years. Agric. Ecosyst. Environ. 206, 10–20. doi: 10.1016/j.agee.2015.03.011
Smit, H. P., Reinsch, T., Swanepoel, P. A., Loges, R., Kluß, C, and Taube, F. (2021). Environmental impact of rotationally grazed pastures at different management intensities in South Africa. Animals 11:1214. doi: 10.3390/ani11051214
Sobhi, M., Gaballah, M. S., Han, T., Cui, X., Li, B., Sun, H., et al. (2021). Nutrients recovery from fresh liquid manure through an airlift reactor to mitigate the greenhouse gas emissions of open anaerobic lagoons. J. Environ. Manag. 294:112956. doi: 10.1016/j.jenvman.2021.112956
Soussana, J. F., Tallec, T., and Blanfort, V. (2010). Mitigating the greenhouse gas balance of ruminant production systems through carbon sequestration in grasslands. Animal 4, 334–350. doi: 10.1017/S1751731109990784
Swanepoel, P. A., Botha, P. R., Snyman, H. A., and du Preez, C. C. (2014a). Impact of cultivation method on productivity and botanical composition of a kikuyu-ryegrass pasture. Afri. J. Range Forage Sci. 9, 1–6. doi: 10.2989/10220119.2014.903999
Swanepoel, P. A., du Preez, C. C., Botha, P. R., Snyman, H. A., and Habig, J. (2014b). Soil quality characteristics of kikuyu-ryegrass pastures in South Africa. Geoderma 232–234, 589–599. doi: 10.1016/j.geoderma.2014.06.018
Swanepoel, P. A., du Preez, C. C., Botha, P. R., Snyman, H. A., and Habig, J. (2015). Assessment of tillage effects on soil quality of pastures in South Africa with indexing methods. Soil Res. 53, 274–285. doi: 10.1071/SR14234
Terrer, C., Jackson, R. B., Prentice, I. C., Keenan, T. F., Kaiser, C., Vicca, S., et al. (2019). Nitrogen and phosphorus constrain the CO2 fertilization of global plant biomass. Nat. Clim. Change 9, 684–689. doi: 10.1038/s41558-019-0545-2
Tongwane, M. I., and Moeletsi, M. E. (2020). Emission factors and carbon emissions of methane from enteric fermentation of cattle produced under different management systems in South Africa. J. Clean Prod. 265:121931. doi: 10.1016/j.jclepro.2020.121931
Trace and Save (2023). Available online at: http://traceandsave.com/woodlands-dairy-partners-with-trace-save/ (accessed October 25, 2023).
Van der Colf, J. (2011). Kikuyu Over-sown with Italian, Westerwolds or Perennial Ryegrass: Dry Matter Production, Botanical Composition and Nutritional Value. The Production Potential of Kikuyu (Pennisetum clandestinum) Pastures Oversown with Ryegrass (Lolium spp.) (MSc. thesis). University of Pretoria, Pretoria, South Africa.
Van der Laan, M., Bristow, K. L., Stirzaker, R. J., and Annandale, J. G. (2017). Towards ecologically sustainable crop production: a South African perspective. Agri. Ecosyst. Environ. 236, 108–119. doi: 10.1016/j.agee.2016.11.014
Verhulst, N., Govaerts, B., Verachtert, E., Castellanos-Navarrete, A., Mezzalama, M., Wall, P., et al. (2010). Conservation agriculture, improving soil quality for sustainable production systems. Adv. Soil Sci. 17, 137–208. doi: 10.1201/EBK1439800577-7
Viljoen, C., Van der Colf, J., and Swanepoel, P. A. (2020). Benefits are limited with high nitrogen fertiliser rates in kikuyu-ryegrass pasture systems. Land 9:173. doi: 10.3390/land9060173
Wall, E., Simm, G., and Moran, D. (2009). Developing breeding schemes to assist mitigation of greenhouse gas emissions. Animal 4, 366–376. doi: 10.1017/S175173110999070X
Yan, T., Mayne, C. S., Gordon, F. G., Porter, M. G., Agnew, R. E., Patterson, D. C., et al. (2010). Mitigation of enteric methane emissions through improving efficiency of energy utilization and productivity in lactating dairy cows. J. Dairy Sci. 93, 2630–2638. doi: 10.3168/jds.2009-2929
Zehetmeier, M., Hoffmann, H., Sauer, J., Hofmann, G., Dorfner, G., and O'Brien, D. (2014). A dominance analysis of greenhouse gas emissions, beef output and land use of German dairy farms. Agri. Syst. 129, 55–67. doi: 10.1016/j.agsy.2014.05.006
Keywords: life cycle assessment, climate change, milk production, livestock, meat production, global warming
Citation: Galloway C, Swanepoel PA and Haarhoff SJ (2024) A carbon footprint assessment for pasture-based dairy farming systems in South Africa. Front. Sustain. Food Syst. 8:1333981. doi: 10.3389/fsufs.2024.1333981
Received: 06 November 2023; Accepted: 22 January 2024;
Published: 07 February 2024.
Edited by:
David Boansi, Kwame Nkrumah University of Science and Technology, GhanaReviewed by:
Thomas M. R. Maxwell, Lincoln University, New ZealandNugun P. Jellason, Teesside University, United Kingdom
Copyright © 2024 Galloway, Swanepoel and Haarhoff. This is an open-access article distributed under the terms of the Creative Commons Attribution License (CC BY). The use, distribution or reproduction in other forums is permitted, provided the original author(s) and the copyright owner(s) are credited and that the original publication in this journal is cited, in accordance with accepted academic practice. No use, distribution or reproduction is permitted which does not comply with these terms.
*Correspondence: Craig Galloway, Y3JhaWdAdHJhY2VhbmRzYXZlLmNvbQ==