- 1Department of Agricultural, Forest and Food Sciences (DISAFA), University of Turin, Turin, Italy
- 2Department of Plant and Environmental Science, University of Copenhagen, Copenhagen, Denmark
Organo-mineral fertilizers (OMFs) can have higher efficiency than mineral fertilizers. In Europe, peat is commonly used as an organic matrix in OMFs, as it is a highly stable organic material. However, peat extraction releases long-term stored organic C. Stabilized biowaste materials could replace peat in OMFs. Thus, this study aimed to understand how the variety of chemical properties in biowastes can influence OMF-soil interactions and nutrient availability to plants. Peat, green compost (GC), municipal solid waste compost (MSWC), and vermicompost (VC) were used as the organic matrix of OMFs with a C-N-P2O5 content of 7.5, 10, and 5%, respectively. OMFs were tested first in a ten-day plant-free incubation to measure Hedley P fractionation, nitrate, ammonium, total N in the soil, and CO2 and NH3 emissions. Further, a 30-day greenhouse trial measured maize yield and N and P use efficiencies. Controls included no fertilization (N0P0) and mineral N and P fertilization (MFNP). No differences were found in the incubation experiment for mineral N fractions in the soil. Fertilization significantly increased CO2 emissions, which were slightly higher in OMFs over MFNP, whereas OMFs significantly increased ammonia volatilization compared to MFNP. Available P had consistent results in the incubation and the greenhouse trials. Peat and MFNP had the highest water-and bicarbonate-P pools in the first experiment and higher yield and P use efficiency by maize. Therefore, OMFs from biowaste materials exhibited limited suitability for short growth cycles due to lower P use efficiency and higher ammonia volatilization.
1 Introduction
Organo-mineral fertilizers (OMFs) combine organic and mineral components designed to provide a balanced supply of nutrients to plants, harnessing the benefits of both organic materials and inorganic minerals (Smith et al., 2020). The degree of influence of the organic material on the mineral fertilizer depends on the proportion between organic and mineral fractions (Pare et al., 2010). Therefore, comparisons across different OMFs are rather complex when OMFs are made with different mineral fertilizers, mixtures of organic and mineral nutrients, and organic materials proportions. Low organic C (Corg) OMFs primarily utilize the organic material as a carrying matrix for mineral nutrients rather than a primary source of nutrients. In Europe, the standard establishes a minimum of 7.5% of Corg in solid OMFs (EC, 2019). Thus, low Corg OMFs have almost negligible amounts of macronutrients provided by the organic material, while they can be a source of some microelements such as Fe, Cu, and Zn (Sitzmann et al., 2023). However, the organic material ranges ideally between 30 to 50% of the total OMF volume (Pare et al., 2010). Consequently, organic materials with a medium Corg content are considered desirable as they allow sufficient addition of mineral fertilizers while providing an optimal organic matrix for efficient nutrient delivery.
In low Corg OMFs, the combination of an organic matrix with mineral nitrogen (N) and phosphorus (P) has proven effective in reducing N losses and enhancing N use efficiency (Richards et al., 1993; Antille et al., 2014; Florio et al., 2016). Additionally, OMF application increases plant P uptake by increasing the availability of mineral P (Antille et al., 2013b). The increase of nutrient use efficiency can be attributed to a variety of underlying processes: (i) the reduction of mineral sorption of P to soil minerals (Parent et al., 2003), thereby facilitating absorption by plant roots while simultaneously inhibiting the transformation of available P into forms that are inaccessible to plants (Khiari and Parent, 2005); (ii) an increase in microbial immobilization of both N and P, leading to a gradual nutrient release (Mandal et al., 2007) and a reduced N leaching (Richards et al., 1993); (iii) a nutrient electrostatic attraction onto the charged surface of the organic material, thereby reducing nutrient mobility in soil (Gwenzi et al., 2018; Luo et al., 2021); (iv) chemical interactions between the inorganic nutrients and the organic fraction that lead to the formation of new compounds with lower solubility (Mazeika et al., 2016; Carneiro et al., 2021; Luo et al., 2021); (v) the OMF acts as a physical barrier to soil humidity, thereby decreasing fertilizer solubility (Limwikran et al., 2018).
Traditionally, OMFs with humic C content have been considered high quality fertilizers (Alianiello et al., 1999; Florio et al., 2016). Humic-related substances have been reported to influence microbial and physicochemical properties of soil increasing crop growth (Li et al., 2019), having a biostimulant effect on plant metabolism (Asli and Neumann, 2010; Vujinović et al., 2020), and ameliorated soil contaminants thanks to a high sorption capacity (Piccolo, 2002; Conte et al., 2005). Therefore, in low Corg OMFs, Peat has been appointed as a high-quality organic material for OMF (Alianiello et al., 1999; Florio et al., 2016) as it is a material typically rich in humic C substances and with a high humification degree (Cavani et al., 2003). Among other geogenic materials, peat in OMFs is allowed in Europe (EC, 2019). However, there is a rising interest in replacing peat with affordable local alternatives that do not require long-distance transportation (Taparia et al., 2021) to protect peatlands to avoid the release of long-term stored C as CO2 and the emissions of other greenhouse gasses (Saarikoski et al., 2019; Humpenöder et al., 2020). Among possible alternatives to peat, biowaste materials have been proposed to replace peat substrates for horticultural production (Taparia et al., 2021) and mushroom cultivation (Schmilewski, 2008).
Biowaste are easily accessible and local resources, thereby offering the benefit of reducing transportation expenses and costs related to peat extraction. Indeed, the incorporation of biowaste materials into OMFs promotes a circular value proposition, as it establishes a closed-loop system that repurposes and recycles waste materials to create agricultural benefits (Barros et al., 2020; Chojnacka et al., 2020; Zeller et al., 2020). For that, biowaste materials should be degraded through digestion, composting, or vermicomposting to achieve the biochemical stability that will allow the production of a homogeneous OMF with stable interactions between the mineral and organic fractions over time (Sakurada et al., 2016; Bouhia et al., 2022). Therefore, using biowaste materials as an organic matrix seems promising for OMF manufacturing (Chojnacka et al., 2020, 2022) and, thus, as peat replacement.
Although multiple interactions between organic and mineral fractions have been described, there is still limited knowledge about the main influences of a biowaste material as the organic matrix on the mineral fertilizer in a blended OMF.
Based on this background, we hypothesized that the close contact of an organic material and mineral fertilizers in a blended OMF will cause:
(H1) slower nutrient release patterns related to the granule integrity in soil due to a higher recalcitrant Corg content.
(H2) an increase of the organic immobilization of nutrients related to a more labile Corg fraction boosting the microbial activity.
(H3) changes in N losses through ammonia volatilization either by reducing it because microbial immobilization into organic N (Norg) or increasing it because the interaction between the organic matrix pH and the mineral fertilizer in the OMF.
These hypotheses were tested by analyzing changes in soil respiration, ammonia emissions, and N and P forms in incubated soils fertilized with granular OMFs made with organic materials from different origins We also tested if potential changes in nutrient availability in soil affect the initial growth and nutrient use efficiency by maize.
This study aimed to contribute to a deeper understanding of the interactions between organic and mineral fractions in low Corg OMFs while also validating alternatives to peat for more sustainable agricultural practices.
2 Materials and methods
This study conducted two independent experiments: (i) a soil incubation trial and (ii) a plant growth trial with maize as the selected crop. For both trials four organo-mineral fertilizers (OMFs) with different organic matrices were used: peat, green compost (GC), municipal solid waste compost (MSWC), and vermicompost (VC). Two control treatments were also included: (1) no fertilization (N0P0); (2) mineral fertilization (MFNP), consisting of a blend of ammonium sulfate, urea, and triple superphosphate (TSP) at an N:P ratio of 10–2.1. In the pot trial, two additional control treatments were used: (3) fertilization with only mineral N (MFN), using ammonium sulfate and urea, and (4) fertilization with only mineral P (MFP), utilizing TSP.
2.1 Organo-mineral fertilizers production
Four different organo-mineral fertilizers (OMFs) were produced using organic materials collected from industries across Italy. The chosen organic materials were green compost based on pruning residues (GC), municipal solid waste compost (MSWC), peat (Peat), and manure-based vermicompost (VC). The organic materials organic C, heavy metals, degrees of stability, and other additional chemical parameters are presented in Table 1. For more details about the characterization of the organic materials, see Sitzmann et al. (2023).
The OMFs were provided by SCAM S.p.a. (Modena, Italy). The OMFs were obtained by a granulation process (Figure 1). The organic material was mixed with mineral N – ammonium sulfate + urea - and water into a granulator. The water was added to facilitate a partial dissolution and impregnation of mineral N into the organic material. The dissolved urea acted as a binder between the organic material and the undissolved mineral N. Diammonium phosphate (DAP) granules is soon thereafter added to the granulator. DAP granules serve as the core for the OMF granules. No additional binders were added. After the granulation, the granules were dried in an oven during 24 h at 60°C to reduce their moisture content to approximately 4%. The OMFs were sieved to give a product of 2 to 5 mm in diameter. The organic material and mineral fertilizers mixture produced OMFs with a Corg-N-P2O5 of 7.5–10-5 (2.1% of total P).
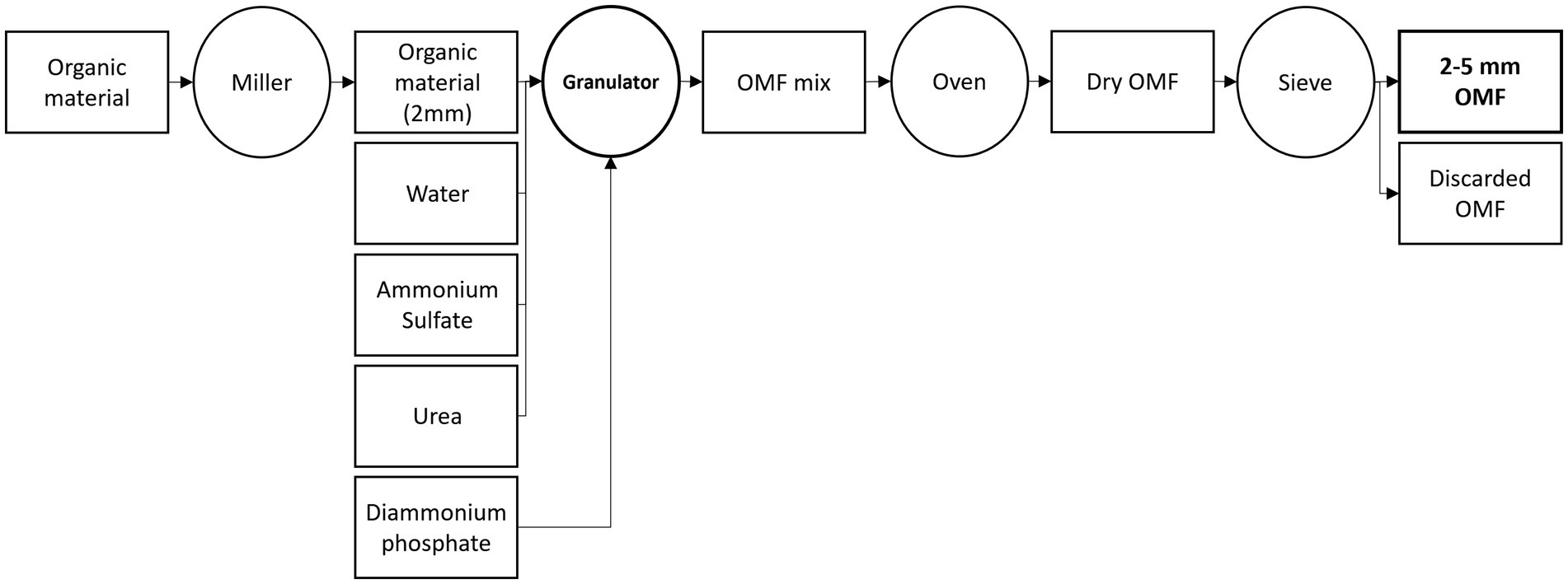
Figure 1. Organo-mineral fertilizer (OMF) manufacturing scheme. The rectangles indicate the materials used during the OMF manufacturing and the circles are the equipment used to transform the materials.
2.2 Soil
The soil used for this study was collected from the unfertilized treatment, serving as the negative control, within the CRUCIAL long-term fertilization trial that was initiated in 2003 at the University of Copenhagen’s experimental farm in Taastrup, Denmark (55°40′N, 12°16′E). This trial predominantly involved the cultivation of spring cereals. The soil is a low-P (Olsen-P: 5 mg kg−1) sandy loam, characterized as a Luvisol according to the FAO classification, comprising 12.6% clay, 14.3% silt, and 69.8% sand. The soil pH was 6.8 (measured at a 1:5 ratio with water), with a water holding capacity (WHC) of 31 g per 100 g of dry soil and a water-extractable P content of 1.78 mg kg−1 in terms of dry soil weight. Further comprehensive insights into the CRUCIAL long-term trial and the specific soil employed in our investigation can be found in López-Rayo et al. (2016) and Lemming et al. (2019). After collection, the soil was air-dried and sieved to a size of 4 mm.
The soil was pre-fertilized with all the essential nutrients (except N and P), independently of its corresponding treatment with nutrient solutions. These were the nutrient application rates: 150 mg K, 50 mg Ca, 40 mg Mg, 1.2 mg Zn, 0.1 mg Mo, 3 mg Fe, 0.3 mg B, 2 mg Mn, 1.5 mg Cu, and 0.1 mg Co per kg−1 soil to avoid any possible complementary nutrient deficiency. The application rates for macro and micronutrients were determined based on preliminary studies conducted with the soil used in this study, and based on Sica et al. (2023). Nitrogen and phosphorus were only provided by the fertilization treatments used in this study.
For both experiments in this study the soil was mixed with quartz sand (0.4 to 0.8 mm) in a proportion of 3 to 1. The sand was added to increase the water infiltration rate and facilitate root growth throughout the experiment. The soil and sand mixture are hereafter called ‘soil.’ Then, the soil was humidified to 40% of water holding capacity and pre-incubated for 7 days at room temperature aiming to reactivate the microbial community, as described by Oehl et al. (2004).
2.3 Incubation setup
For the incubation, 120 mL plastic containers, with a surface area of 18 cm2, were filled with the equivalent of 100 g of dry soil packed to a bulk density of 1.00 g cm−3. Before being added to the plastic containers, each experimental unit was fertilized homogeneously with 600 mg N kg−1 soil and 131 mg P kg−1 soil and humidified to 50% of its water-holding capacity. The following treatments were used in this experiment: (1) no P, no N; (2) N and P mineral fertilizers (MFNP); (3) peat OMF; (4) GC OMF; (5) MSWC OMF; (6) VC OMF.
Thereafter, containers were placed into a 750 mL glass jar with an airtight lid. Also, in the same jar two open traps were placed consisting of (1) 5 mL of 0.2 M sulfuric acid (H2SO4) in an open plastic container (26 mL airtight plastic container, Frisenette ApS, Knebel, Denmark) to trap ammonia (NH3) that could potentially volatilize (Ndegwa et al., 2009); (2) 10 mL of 1 M sodium hydroxide (NaOH) to trap the CO2 released by the soil. In addition, 10 mL of deionized water was placed into a plastic shot glass to maintain a high humidity inside the jar preventing desiccation of the fertilized soils. The jars were incubated in a dark room set at approximately 15°C for 10 days. Thereafter, fresh soil samples for inorganic analysis were collected avoiding OMF granules that may not have dissolved completely.
2.4 Plant trial setup
The pot trial was carried out with the same soil, bulk density, and pre-fertilization as the incubation. Soils were homogeneously fertilized with OMFs and controls to achieve 300 mg N kg−1 soil and 65.5 mg P kg−1 soil and added to 1-liter pots with the equivalent of 1 kg of dry soil packed to a bulk density of 1.00 g cm−3. The following treatments were used in this study: (1) no P, no N (N0P0); (2) no P, mineral N (MFN); (3) no N, mineral P (MFP); (4) mineral N and P (MFNP); (5) peat OMF; (6) GC OMF; (7) MSWC OMF; (8) VC OMF.
One day after fertilization, one pre-germinated seed of maize (Zea mays) cv. “Ambition” (Limagrain SE) was transplanted to each pot, ensuring that all seedlings were similar height. The pots were rotated randomly four times a week to mitigate the potential impact of temperature or light gradients within the greenhouse. Additionally, watering was conducted based on weight measurements to sustain the soil moisture content at 60% of the soil water holding capacity (WHC). Throughout the experiment, the daytime (14 h) temperatures fluctuated between a minimum of 13°C and a maximum of 28°C, while the nighttime (10 h) temperatures ranged from 6°C to 18°C.
2.5 Analyses
2.5.1 Incubation
For the incubation, all treatments were done in triplicates. After 10 days, the fresh soil was homogenized to analyze N-NH4+, N-NO3−, and Hedley sequential extraction fractionation.
For the ammonium and nitrate, the fresh soil was extracted with KCl at a ratio of 1:5, by shaking in an end-over-end shaker for 1 h. The samples were filtered using Whatman filter papers and analyzed for N-NH4+ and N-NO3-by a flow-injection analyzer (FIAstar 500, FOSS, Denmark).
A simplified Hedley P fractionation scheme (Hedley et al., 1982) was used to evaluate the impact of OMFs on distinct soil inorganic P pools. This adapted fractionation technique consisted of four sequential extractions from an equivalent of 0.5 g of dry soil. The first extraction was with 30 mL of deionized water (at a 1:60 ratio) in the soil, followed by 16 h of extraction using an end-over-end shaker and subsequent centrifugation (15 min, 5,000 rpm). The supernatant was filtered, and the residual solids were employed for the subsequent extraction stage, with 30 mL of 0.5 M NaHCO3. The same procedure — 30 mL of extractant, 16 h of shaking, 15 min of centrifugation at 5,000 rpm, and supernatant filtration — was repeated for the subsequent extractions employing 0.1 M NaOH and 1 M HCl. The ortho-P content in all extracted solutions was determined using the molybdenum blue method on a flow injection analyzer (FIAstar 5,000, Foss Analytical, Denmark).
Norg was calculated as the difference between total N and N-NH4+ and N-NO3−. Residual P was measured as the differences between the soil total P and the P extracted by water, bicarbonate, NaOH, and HCl fractions.
The microbial respiration was determined by adding a saturated barium chloride solution to the 1 M NaOH trap solutions and back titrating it with 1 M HCl, adapted from Alef (1995). The ammonia emissions were determined by measuring the NH4+ contents in the H2SO4 traps.
2.5.2 Plant analyses
For the plant trial, all treatments were performed with four replicates. Plant shoots were harvested 33 days after transplanting and dried at 60°C for 48 h to determine the dry matter (DM). After that, shoots were placed in a container with three zirconia balls and milled by shaking for 5 min. A subsample was collected to determine the total N content in the shoots using an Elemental Analyzer (Vario macro cube, Elementar Analysensysteme GmbH, Germany).
The total P was determined as follows: around 100 ± 10 mg of the sample powder was precisely weighed into crucibles. The crucibles with the samples underwent incineration at 550°C for 1 h. Following incineration, the resulting ash was extracted using 50 mL of 0.5 M sulfuric acid, utilizing an end-over-end shaker overnight. The subsequent step involved filtering the extract through Whatman No. 5 ashless filter papers to eliminate any residual solid particles. Ortho-P content in the extract was quantified using ammonium molybdate by a flow-injection analyzer (FIAstar 500, FOSS, Denmark).
2.6 Calculations
The N and P uptake, in mg kg−1 soil, were calculated by multiplying the shoots dry matter, in g DM kg−1 soil, by the N and P content, respectively, in mg g−1 DM.
The nutrient index is calculated according to the Eq. (1):
These values indicate the plant’s nutrition level, with 100 indicating an ideal situation, whereas a higher value indicates a luxurious consumption by the crop, and a value below 100 indicates a deficiency (Duru and Ducrocq, 1996).
For N, the critical content was calculated according to Eq. (2), a model obtained by Ziadi et al. (2008) for maize:
Where N critical content is the N content at which below the plant will be under deficiency of N (in %) and DM is the plant dry matter (in g DM kg−1 soil).
The P critical content was calculated according to Eq. (3), based on the model developed by Gagnon et al. (2020) for maize:
Where the P critical content is the P content at which below the plant will be under P deficiency and the N content is the N measured concentration in the maize shoots in mg g−1.
The N or P use efficiency (NUE, PUE respectively) were calculated according to Eq. (4):
2.7 Statistical analyses
The pot trial was set up in a randomized complete block design, while the incubation had a complete randomized design. A General linear model (GLM) procedure was used to analyze variables considering the treatment as a categorical variable. A post hoc Tukey’s HSD test (<0.05) was performed when differences were found between treatments. Analyses were performed using the statistical software R, version 4.3.1.
3 Results and discussion
3.1 Organic matter stability effect on OMF coating (hypothesis I)
We hypothesized that the stability of the organic material used to coat the mineral fertilizer within the OMF would increase the granule’s resistance to degradation in soil, thereby prolonging nutrient release. After 10 days of incubation, N-NH4+ remained higher than N-NO3− in all treatments. The N-NH4+ in fertilized treatments was 3 times higher than in the control N0P0, while the N-NO3− content was only 1.5 to 2 times higher than in N0P0. Fertilization increased the total mineral N content in soil by an average of 28 to 42%. No significant differences in the Norg content were found between treatments (Table 2).
Using the Alkyl C/O Alkyl C ratio (Baldock et al., 1997; Pizzanelli et al., 2023) and the humification degree (Zaccone et al., 2018) as indicators of Corg stability, it is shown that Peat would be more difficult to degrade in soil than GC, MSWC and VC. However, with no differences in N-NH4+ and N-NO3− concentrations it was not possible to confirm the hypothesis that the granule integrity will slow down the N release. Additionally, in the pot trial, among the OMFs and the control MFNP, NNI (>100%) demonstrated that the N dosage used was sufficient to sustain plant needs through the growth trial, not limiting growth (Table 3).
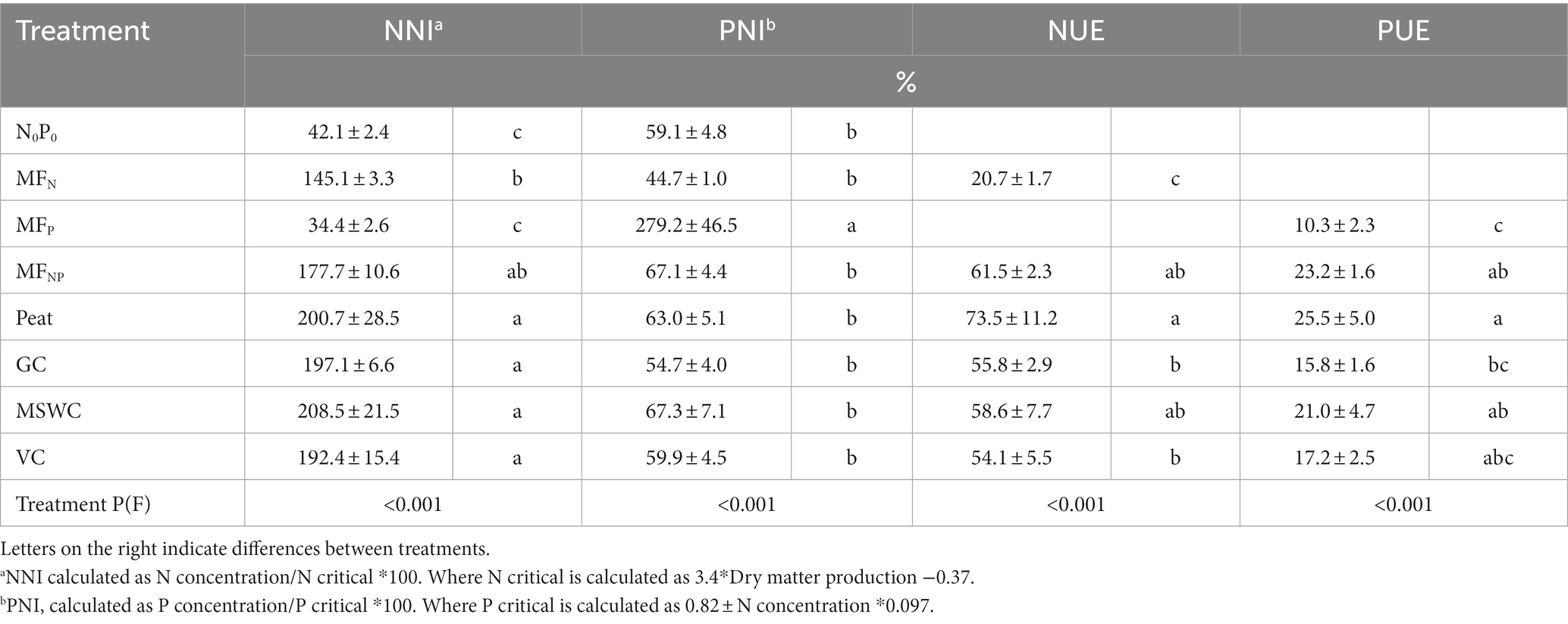
Table 3. N and P nutrient index (NNI and PNI, respectively), and N and P use efficiency (NUE and PUE, respectively) average and standard deviation.
Adding P increased the different P fractions in the soil compared to the control N0P0. Treatments MSWC and VC had on average half of the water-extractable P (Water P) than the control MFNP, GC, and Peat (Table 2). Among the fertilizers, the bicarbonate-P pool was significantly higher for peat OMFs, compared to MSWC and VC. The NaOH P pool was significantly higher in VC than in GC and MSWC. Compared to the control N0P0, the HCl P pool was higher only in Peat (31%), with intermediate values in MFNP (29%), GC (12%), MSWC (17%), and VC (21%). The residual P was significantly lower in the mineral control MFNP (−10%) than in MSWC, with intermediate values for the rest of the treatments.
Despite differences in P availability, the initial hypothesis can also be dismissed for P. Peat had the highest Corg stability among all samples (Table 1); however, water and bicarbonate P concentrations in soil – considered indicators of plant-available P (Hedley et al., 1982; Teng et al., 2020) – were higher for peat OMF compared to the other OMFs (Table 2). This higher plant-available P was consistent in both experiments, as peat OMF also had the highest PUE (Table 3) among the fertilizers in the plant trial experiment.
It has been reported that physical forms that promote compacted proximity between the organic and mineral components in OMFs, such as pellets and grains, exhibit a more gradual release of nutrients compared to mineral controls or powdered mixtures of these constituents (Rodrigues et al., 2021; Fachini et al., 2022). However, this observation was not corroborated by the present study. Despite the granulated peat-based OMF’s physical form, there were no discernible differences in the availability of N and P in the soil and their uptake by maize compared to the mineral control MFNP. Consequently, the differences in nutrient availability observed in the cases of GC, MSWC, and VC OMFs are more closely linked to interactions between the soil and the OMF rather than the organic matrix stability in the OMF.
3.2 Organic matter effect on nutrient organic immobilization (hypothesis II)
We also hypothesized that adding a less recalcitrant Corg source through the OMF in the soil might enhance nutrient immobilization by stimulating microbial activity. Soil CO2 emission was used as a microbial activity indicator (Barnard et al., 2020). The fertilization was the primary driver for higher respiration compared to the control N0P0 (Figure 2), as it increased it by 34 to 43 times, indicating nitrogen deficiency in the turnover of native soil carbon. A modest yet consistent increase (11 to 23%) in CO2 emissions was observed in OMFs compared to the mineral control MFNP. This increase may have been caused by adding Corg to C-limited microorganisms in the soil (Traoré et al., 2016; Zheng et al., 2020). Although some authors point out that the fertilizer-soil interface can immobilize N (Moritsuka et al., 2004) and P (Sica et al., 2023) in organic forms, this has not been observed in this study.
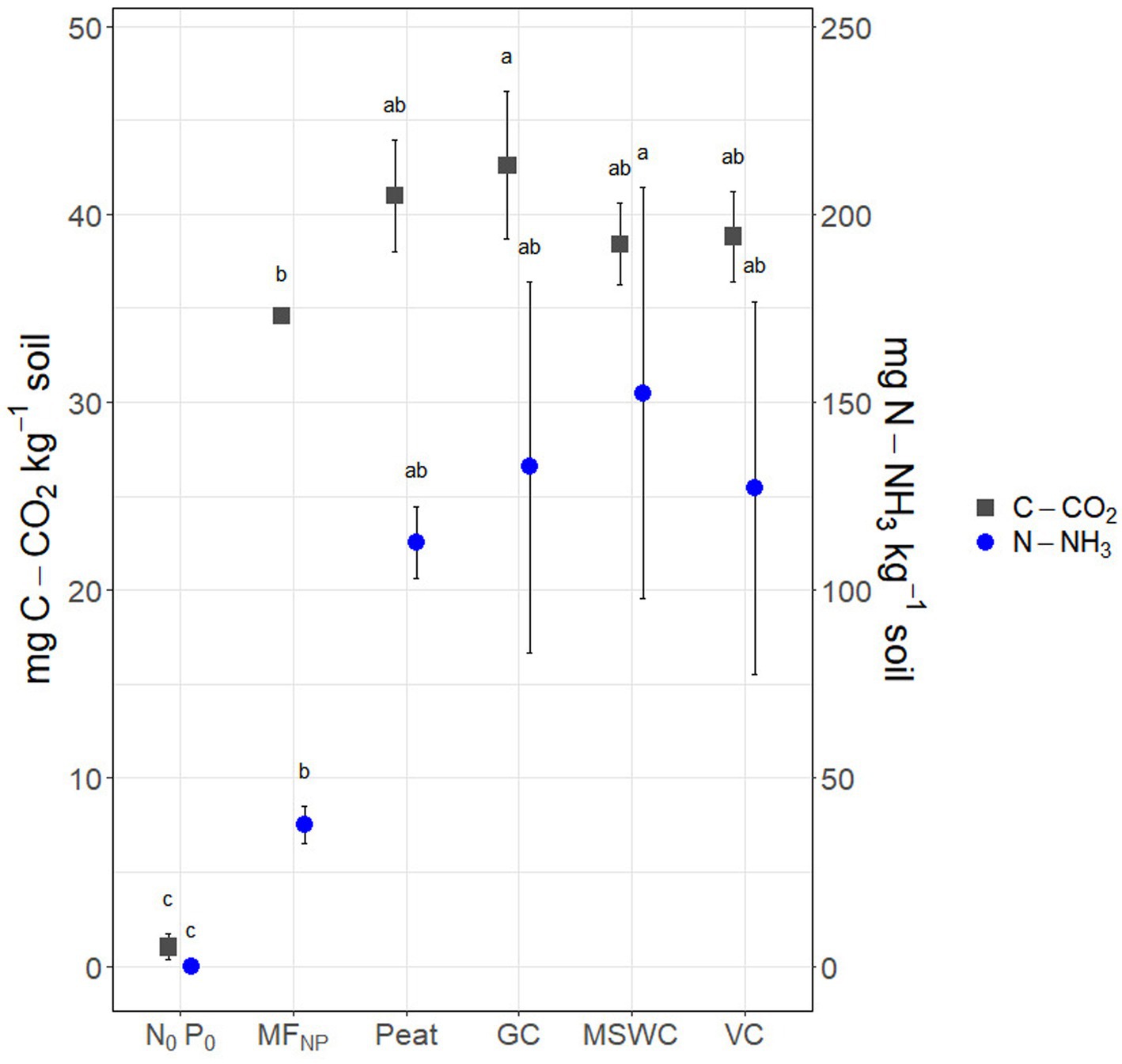
Figure 2. Average CO2 and NH4 emissions in an incubation of 10 days. Bars indicate standard deviation. Letters above the bars indicate differences between treatments.
On the other hand, in the case of P, it was observed that the GC, MSWC, and VC OMFs had higher residual P fractions in soil compared to the mineral control and the Peat OMF (Table 2). In the simplified Hedley extraction method, the residual P also accounts for organic P fractions in the soil (Alvarenga et al., 2017; Sica et al., 2023). Differences between the OMFs on the increase of residual P can be linked to the stability of the organic material and its resistance to microbial breakdown (Bernal et al., 1998; Grigatti et al., 2020). However, no statistical differences exist for the residual P between the control N0P0 and the biowaste OMFs, suggesting that adding low Corg quantities does not immobilize P into the organic fraction.
Nevertheless, it is possible to estimate that the addition of Corg alone will cause an almost neglectable nutrient immobilization compared to the other nutrients added. An indicative microbial nutrient immobilization can be calculated assuming that 20% of the respired C-CO2 from the OMFs treatments was incorporated into the microbial biomass C (Holland and Coleman, 1987; Sistla et al., 2012) and a stoichiometric relation for soil microbial biomass C:N:P of 60:7:1 (Cleveland and Liptzin, 2007). During the incubation, the soil respiration was between 4 to 8 mg C-CO2 kg−1 soil higher in the OMFs treatments than in the mineral control MFNP (Figure 2). Therefore, adding Corg through the OMFs would immobilize only 0.3 to 0.6 mg N kg−1 soil and 0.02 to 0.04 mg P kg−1 soil into the microbial biomass. The differences in water-and bicarbonate-extractable P between GC, MSWC, and VC and the mineral control MFNP ranged between 8 to 37 and 10 to 19 mg P kg−1 soil, respectively. This indicates that differences in available P between low Corg biowaste OMFs and MFNP and Peat OMF are related to chemical interactions between the organic material and the mineral fertilizer rather than microbial immobilization.
3.3 Organic matter effect on nutrient chemical immobilization
As the organic matrix stability does not significantly influence the granule integrity nor the microbial immobilization of nutrients, it is necessary to examine further chemical properties that may influence nutrient availability. Unexpectedly no link was found between the organic matrix properties and N availability in soil. A slower N release by OMFs was reported in studies using urea combined with potash to coat a biosolid granule used as a P source (Antille et al., 2013a, 2014) and ammonium nitrate pelletized with peat (Richards et al., 1993). However, comparing those studies with our results is rather complex due to differences in organic materials, elaboration process, and formulation. In a study by Florio et al. (2016), a peat OMF similar to the one used in this study, with 7.5% Corg produced with 20% N (12% ammoniacal N, 8% ureic N, and 1% Norg), reported a reduction of N release into the soil after measuring at different days less nitrate content in leachates but similar N-NH4+ emissions and N plant uptake than a mineral control. Differences between that study and ours can be related to time differences (10 vs. 36 days of incubation).
A potential explanation for the lack of differences in soil N fractions was that the soil used in the incubation showed a low nitrification capacity for the duration of the experiment as most of the N was found as N-NH4+. The low nitrification capacity could be caused by the high N fertilization used during the incubation. High N inputs have been associated with a lower soil quality that decreases the soil microbial functional diversity, inhibiting the N transformation in soil (Shen et al., 2010). Differences between fertilizers’ release patterns can be observed in soils with a high nitrification capacity (Sahrawat, 2008). Shortly after fertilization, fertilizers with an earlier release of N will have a lower N-NH4+ concentration and a higher N-NO3− concentration than a fertilizer with a slow N release (Sahrawat, 2008).
On the other hand, a plausible explanation for the differences in P availability can be related to the higher alkalinity of the biowaste materials compared to Peat. Biowaste materials surpassed pH 9 (Table 1), which may reduce P availability to plants (Hartemink and Barrow, 2023). Additionally, a potential chemical interaction between OMF and soil that reduces plant available P is the formation of bonds between Ca and phosphate (Lindsay and Stephenson, 1959; Penn and Camberato, 2019). Peat and VC had a similar Ca content, while GC and MSWC have a slightly higher Ca content (Table 1); however, the higher pH in GC, MSWC, and VC may have stimulated the formation of Ca-phosphates bonds compared to Peat (Devau et al., 2011). Although no differences in N soil fractions were found, the acid–base properties of the organic material can limit the plant available P fractions in soil.
3.4 Organic material acid–base properties influence N losses (hypothesis III)
We hypothesized that the organic matrix of an OMF can influence ammonia volatilization, reducing or increasing it. The average ammonia volatilization in the soil after 10 days was 3 to 4 times higher in the OMF treatments than in the mineral control MFNP (Figure 2). It is worth noting that for NH3 soil emissions, the standard deviation in the OMFs was considerably higher (2 to 10 times higher) than in the MFNP.
Our findings of OMFs increasing the ammonia volatilization are similar to the results from Florio et al. (2016), who found that OMFs with low Corg quantity made with peat as an organic matrix tend to increase the ammonia volatilization compared to a mineral control. Florio et al. (2016) hypothesized that the increase in ammonia volatilization was caused by a rising pH in the soil surrounding the OMF granules caused by the hydrolyzation of labile Norg by soil urease enzymes. The Norg values of the organic materials were correlated to the ammonia emissions since the highest ammonia values were found in MSWC, which was the organic material with the higher Norg content. At the same time, Peat had the lowest Norg content and the lower ammonia volatilization among OMFs. It is unclear if OMFs with less than 0.5% of total Norg will cause a discernible pH increase in the fertilizer hotspot.
In addition, the pH of the organic material itself could cause higher ammonia volatilization. It is well known that a high pH in the soil area where the fertilizer is present can increase the N losses through ammonia volatilization (Fenn and Hossner, 1985; Fan and Mackenzie, 1993); shifting the ammonium-ammonia equilibriums toward the gaseous form (Körner et al., 2001). All organic materials used in this experiment were alkaline, but peat had one to two points less pH than other organic materials (Table 1). The organic material alkalinity explains a trend of lower volatilization in Peat than in the rest of the OMFs. Further, the organic materials used in this experiment would provide more than 1% of CaO to 7.5% Corg OMFs, which may cause a localized liming effect (Maguire et al., 2006; de Campos et al., 2022), increasing the NH3 volatilization. On this base, an acidification process of the organic matrix can be suggested (Mari et al., 2005; Turan, 2008; Fangueiro et al., 2015) as adding small quantities of organic materials to the mineral fertilizer promotes ammonia volatilization.
3.5 OMF effect on maize nutrient use efficiency
The sum of the hypothesized interactions between the organic matrix and the mineral fertilizers in the OMF and soil can cause an apparent slow release of nutrients by the fertilizers. Therefore, we tested if nutrients differences will cause an effect on early growth of maize plants. Differences in yield and nutrient uptake were linked to differences in plant available P in the incubation experiment. Maize dry matter ranged between 1.2 and 7.9 g (Figure 3A). Fertilization with only N (MFN) or P (MFP) did not increase the yield compared to the control N0P0, suggesting a co-limitation of nutrients in the soil used in this experiment. The lower maize yield in N0P0, MFN, and MFP was expected due to previous reports of low soil N and P availability (Gómez-Muñoz et al., 2018; Lemming et al., 2019). For the treatments receiving combined mineral N and P, the yield increased 3 to 5 times compared to N0P0, with Peat showing a similar yield to the mineral control MFNP. However, all three biowaste materials OMFs had significantly lower DM compared to peat OMF and MFNP.
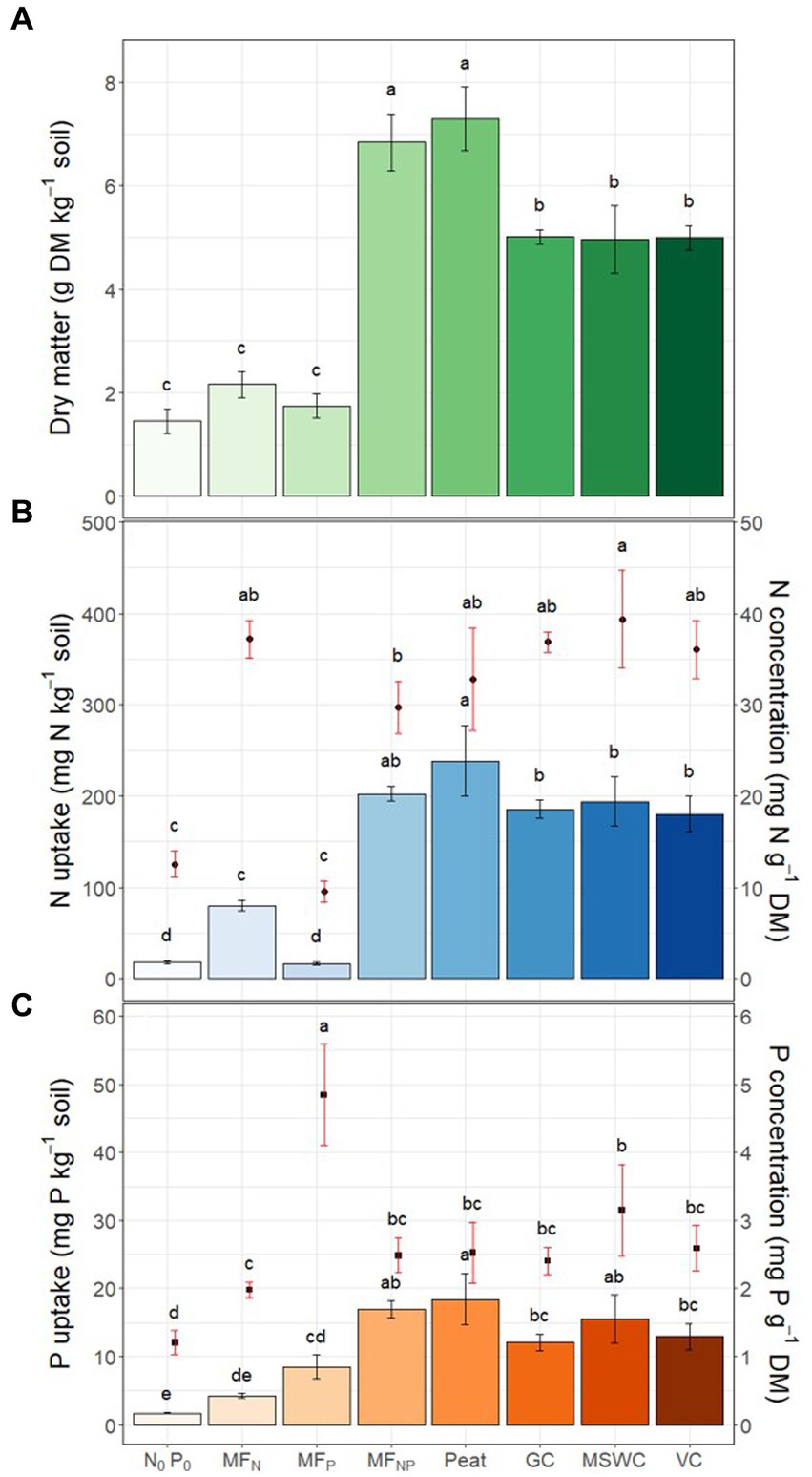
Figure 3. OMF influence in crop: (A) Above ground dry matter production; (B) N concentration in tissues (as points) and N uptake (as bars) by maize shoots; (C) P concentration in tissues (as points) and P uptake (as bars) by maize shoots. Letters above the standard deviation bars indicate differences between treatments.
The addition of N and P also increased the N and P contents in the shoots (Figure 3B). The addition of only mineral P did not influence the plant N concentration. However, when mineral P was added in MFP, MFNP, Peat, GC, MSWC, and VC, the P concentration was 2 to 4 times higher than in control N0P0, being higher in MFP and with no differences between treatments with combined mineral N and P (Figure 3C). Using the concentrations to calculate the N and P nutrient indexes, it was observed that after one month, the limiting nutrient for plant growth was P, except for MFP, which was strongly limited by N, whereas the N reserves in shoots surpassed 1.5 to 2 times the minimum content required for its plant growth, except for the control N0P0 and MFP (Table 3).
The N uptake by shoots followed a similar trend as biomass production. Adding combined N and P (MFNP and OMFs) increased N uptake 10 to 13 times compared to the control N0P0 (Figure 3B). Compared to other OMFs, Peat significantly increased N uptake by 23 to 32%. The P uptake was significantly higher in Peat than in GC and VC by 53 and 42%, respectively. The nutrient uptake reflected the efficiency of the different fertilizers, with higher NUE and PUE found for Peat and the lowest NUE and PUE efficiency in GC and VC (Table 3).
Treatments with both mineral N and P (MFNP, Peat, GC, MSWC, and VC) had the same content of mineral nutrients; however, the organic matrix could provide additional N and P to the OMFs (Rady, 2012; Antille et al., 2013a,b; Anetor and Omueti, 2014). However, with a content of 7.5% Corg in OMFs, the Norg in the organic matrix would represent less than 0.5% of the total N in fertilizer while the P addition is practically neglectable and does not influence P uptake. Thus, as N was not limiting plant growth in this study, the additional Norg may not have affected the plant growth.
In soils receiving N and P fertilization, the nutrient index values revealed that P (PNI < 70%) rather than N (NNI > 170%) acted as a growth-limiting factor. Consequently, plant growth and N uptake differences could be attributed to variations in P release or immobilization (Hertzberger et al., 2020). Also, although OMF had higher N losses through ammonia volatilization, it did not impact the N uptake in 30-day-old maize plants. The biowaste organic matrix effect on P availability in 10 days caused plant differences after 30 days, indicating that crops require longer periods of time to recover this immobilized P.
4 Conclusion
The results obtained in this study show that even small quantities of organic material with high pH should not be used in OMFs containing mineral N. It is necessary to reduce N losses in biowaste OMFs before using them as effective peat OMF replacements. In addition, biowaste-based OMFs used in this experiment are not recommendable for short-term growing crops because of a lower initial P availability that reduces the P use efficiency. These biowaste OMFs require to be tested in long-term trials to determine if the unavailable P can be taken up by plants over extended periods.
The main differences between organo-mineral fertilizer (OMF) treatments are related to whether the organic material in OMFs originated from geogenic sources or from biowastes that underwent a composting process. However, our hypotheses of the organic carbon stability influencing the granule integrity and the microbial immobilization were discarded. The main factors influencing the P availability in soil are related to the Ca and pH of the organic matrixes. Our third hypothesis was confirmed as the pH of the organic matrix from the OMFs increased the ammonia volatilization. The organic matrix influence on the mineral fertilizers remained after 30 days, as the initial P immobilization correlated to a lower P use efficiency by early maize plants. However, N losses may not have affected the fertilizer use efficiency, as for all OMFs, plants were not limited by N uptake as indicated by the Nitrogen Nutrient Index.
Data availability statement
The raw data supporting the conclusions of this article will be made available by the authors, without undue reservation.
Author contributions
TS: Conceptualization, Data curation, Formal analysis, Investigation, Methodology, Writing – original draft. PS: Conceptualization, Investigation, Methodology, Writing – review & editing. CG: Funding acquisition, Writing – review & editing. JM: Conceptualization, Supervision, Writing – review & editing.
Funding
The author(s) declare financial support was received for the research, authorship, and/or publication of this article. This project has received funding from the European Union’s Horizon 2020 research and innovation program under the Marie Sklodowska-Curie grant agreement no. 860127.
Acknowledgments
The authors appreciate the collaboration of Stefano Tagliavini from SCAM S.p.A. for manufacturing the organo-mineral fertilizers used in this experiment. The authors thank Laura Zavattaro for her help in correcting the manuscript.
Conflict of interest
The authors declare that the research was conducted in the absence of any commercial or financial relationships that could be construed as a potential conflict of interest.
Publisher’s note
All claims expressed in this article are solely those of the authors and do not necessarily represent those of their affiliated organizations, or those of the publisher, the editors and the reviewers. Any product that may be evaluated in this article, or claim that may be made by its manufacturer, is not guaranteed or endorsed by the publisher.
References
Alef, K. (1995). “Soil respiration” in Methods in soil microbiology and biochemistry. eds. K. Alef and P. Nannipieri (San Diego: Academic Press Inc)
Alianiello, F., Dell’Orco, S., Benedetti, A., and Sequi, P. (1999). Identification of primary substrates in organo-mineral fertilizers by means of isoelectric focusing. Commun. Soil Sci. Plant Anal. 30, 2169–2181. doi: 10.1080/00103629909370363
Alvarenga, E., Øgaard, A. F., and Vråle, L. (2017). Effect of anaerobic digestion and liming on plant availability of phosphorus in iron-and aluminium-precipitated sewage sludge from primary wastewater treatment plants. Water Sci. Technol. 75, 1743–1752. doi: 10.2166/WST.2017.056
Anetor, M. O., and Omueti, J. A. I. (2014). Organo-mineral fertilizer effects in some phosphorus-unresponsive soils of southwestern Nigeria: 1. Effects on maize growth response and soil properties. Agric. Biol. J. N. Am. 5, 265–280. doi: 10.5251/abjna.2014.5.6.265.280
Antille, D. L., Sakrabani, R., and Godwin, R. J. (2014). Nitrogen release characteristics from biosolids-derived Organomineral fertilizers. Commun. Soil Sci. Plant Anal. 45, 1687–1698. doi: 10.1080/00103624.2014.907915
Antille, D. L., Sakrabani, R., Tyrrel, S. F., Le, M. S., and Godwin, R. J. (2013a). Characterisation of organomineral fertilisers derived from nutrient-enriched biosolids granules. Appl. Environ. Soil Sci. 2013, 1–11. doi: 10.1155/2013/694597
Antille, D. L., Sakrabani, R., Tyrrel, S. F., Le, M. S., and Godwin, R. J. (2013b). Development of organomineral fertilisers derived from nutrient-enriched biosolids granules: product specification. Am. Soc. Agric. Biol. Eng. 5, 4152–4170. doi:10.13031/aim.20131620153
Asli, S., and Neumann, P. M. (2010). Rhizosphere humic acid interacts with root cell walls to reduce hydraulic conductivity and plant development. Plant Soil 336, 313–322. doi: 10.1007/s11104-010-0483-2
Baldock, J. A., Oades, J. M., Nelson, P. N., Skene, T. M., Golchir, A., and Clarke, P. (1997). Assessing the extent of decomposition of natural organic materials using solid-state 13C NMR spectroscopy. Soil Res. 35, 1061–1084. doi: 10.1071/S97004
Barnard, R. L., Blazewicz, S. J., and Firestone, M. K. (2020). Rewetting of soil: revisiting the origin of soil CO2 emissions. Soil Biol. Biochem. 147:107819. doi: 10.1016/j.soilbio.2020.107819
Barros, M. V., Salvador, R., de Francisco, A. C., and Piekarski, C. M. (2020). Mapping of research lines on circular economy practices in agriculture: from waste to energy. Renew. Sust. Energ. Rev. 131:109958. doi: 10.1016/j.rser.2020.109958
Bernal, M. P., Sánchez-Monedero, M. A., Paredes, C., and Roig, A. (1998). Carbon mineralization from organic wastes at different composting stages during their incubation with soil. Agric. Ecosyst. Environ. 69, 175–189. doi: 10.1016/S0167-8809(98)00106-6
Bouhia, Y., Hafidi, M., Ouhdouch, Y., Boukhari, M. E. M.El, Mphatso, C., Zeroual, Y., et al. (2022). Conversion of waste into organo-mineral fertilizers: current technological trends and prospects. Rev. Environ. Sci. Biotechnol. doi: 10.1007/s11157-022-09619-y, 21, 425–446
Carneiro, J. S. D. S., Ribeiro, I. C. A., Nardis, B. O., Barbosa, C. F., Lustosa Filho, J. F., and Melo, L. C. A. (2021). Long-term effect of biochar-based fertilizers application in tropical soil: agronomic efficiency and phosphorus availability. Sci. Total Environ. 760:143955. doi: 10.1016/J.SCITOTENV.2020.143955
Cavani, L., Ciavatta, C., and Gessa, C. (2003). Identification of organic matter from peat, leonardite and lignite fertilisers using humification parameters and electrofocusing. Bioresour. Technol. 86, 45–52. doi: 10.1016/S0960-8524(02)00107-4
Chojnacka, K., Mikula, K., Skrzypczak, D., Izydorczyk, G., Gorazda, K., Kulczycka, J., et al. (2022). Practical aspects of biowastes conversion to fertilizers. Biomass Conv. Bioref. 1, 1–19. doi: 10.1007/S13399-022-02477-2/TABLES/5
Chojnacka, K., Moustakas, K., and Witek-Krowiak, A. (2020). Bio-based fertilizers: a practical approach towards circular economy. Bioresour. Technol. 295:122223. doi: 10.1016/j.biortech.2019.122223
Cleveland, C. C., and Liptzin, D. (2007). C:N:P stoichiometry in soil: is there a “Redfield ratio” for the microbial biomass? Biogeochemistry 85, 235–252. doi: 10.1007/s10533-007-9132-0
Conte, P., Agretto, A., Spaccini, R., and Piccolo, A. (2005). Soil remediation: humic acids as natural surfactants in the washings of highly contaminated soils. Environ. Pollut. 135, 515–522. doi: 10.1016/J.ENVPOL.2004.10.006
de Campos, M., Rossato, O. B., Marasca, I., Martello, J. M., de Siqueira, G. F., Garcia, C. P., et al. (2022). Deep tilling and localized liming improve soil chemical fertility and sugarcane yield in clayey soils. Soil Tillage Res. 222:105425. doi: 10.1016/j.still.2022.105425
Devau, N., Hinsinger, P., Le Cadre, E., Colomb, B., and Gérard, F. (2011). Fertilization and pH effects on processes and mechanisms controlling dissolved inorganic phosphorus in soils. Geochim. Cosmochim. Acta 75, 2980–2996. doi: 10.1016/J.GCA.2011.02.034
Duru, M., and Ducrocq, H. (1996). A nitrogen and phosphorus herbage nutrient index as a tool for assessing the effect of N and P supply on the dry matter yield of permanent pastures. Nutr. Cycl. Agroecosyst. 47, 59–69. doi: 10.1007/bf01985719
EC (2019). Regulation (EU) 2019/1009 of the European Parliament and of the council of 5 June 2019 laying down rules on the making available on the market of EU fertilising products and amending regulation (EC) no 1069/2009 and (EC) no 1107/2009 and repealing Regulat. Off. J. Eur. Union.
Fachini, J., De Figueiredo, C. C., Vale, A. T., and Do,. (2022). Assessing potassium release in natural silica sand from novel K-enriched sewage sludge biochar fertilizers. J. Environ. Manag. 314:115080. doi: 10.1016/J.JENVMAN.2022.115080
Fan, M. X., and Mackenzie, A. F. (1993). Urea and phosphate interactions in fertilizer microsites: Ammonia volatilization and pH changes. Soil Sci. Soc. Am. J. 57, 839–845. doi: 10.2136/sssaj1993.03615995005700030034x
Fangueiro, D., Hjorth, M., and Gioelli, F. (2015). Acidification of animal slurry– a review. J. Environ. Manag. 149, 46–56. doi: 10.1016/J.JENVMAN.2014.10.001
Fenn, L. B., and Hossner, L. R. (1985). Ammonia volatilization from ammonium or ammonium-forming nitrogen fertilizers. New York, NY: Springer
Florio, A., Felici, B., Migliore, M., Dell’Abate, M. T., and Benedetti, A. (2016). Nitrogen losses, uptake and abundance of ammonia oxidizers in soil under mineral and organo-mineral fertilization regimes. J. Sci. Food Agric. 96, 2440–2450. doi: 10.1002/jsfa.7364
Gagnon, B., Ziadi, N., Bélanger, G., and Parent, G. (2020). Validation and use of critical phosphorus concentration in maize. Eur. J. Agron. 120:126147. doi: 10.1016/j.eja.2020.126147
Gómez-Muñoz, B., Jensen, L. S., de Neergaard, A., Richardson, A. E., and Magid, J. (2018). Effects of Penicillium bilaii on maize growth are mediated by available phosphorus. Plant Soil 431, 159–173. doi: 10.1007/s11104-018-3756-9
Grigatti, M., Barbanti, L., Hassan, M. U., and Ciavatta, C. (2020). Fertilizing potential and CO2 emissions following the utilization of fresh and composted food-waste anaerobic digestates. Sci. Total Environ. 698:134198. doi: 10.1016/j.scitotenv.2019.134198
Gwenzi, W., Nyambishi, T. J., Chaukura, N., and Mapope, N. (2018). Synthesis and nutrient release patterns of a biochar-based N–P–K slow-release fertilizer. Int. J. Environ. Sci. Technol. 15, 405–414. doi: 10.1007/S13762-017-1399-7/FIGURES/6
Hartemink, A. E., and Barrow, N. J. (2023). Soil pH - nutrient relationships: the diagram. Plant Soil 486, 209–215. doi: 10.1007/s11104-022-05861-z
Hedley, M. J., Stewart, J. W. B., and Chauhan, B. S. (1982). Changes in inorganic and organic soil phosphorus fractions induced by cultivation practices and by laboratory incubations. Soil Sci. Soc. Am. J. 46, 970–976. doi: 10.2136/SSSAJ1982.03615995004600050017X
Hertzberger, A. J., Cusick, R. D., and Margenot, A. J. (2020). A review and meta-analysis of the agricultural potential of struvite as a phosphorus fertilizer. Soil Sci. Soc. Am. J. 84, 653–671. doi: 10.1002/saj2.20065
Holland, E. A., and Coleman, D. C. (1987). Litter placement effects on microbial and organic matter dynamics in an agroecosystem. Ecology 68, 425–433. doi: 10.2307/1939274
Humpenöder, F., Karstens, K., Lotze-Campen, H., Leifeld, J., Menichetti, L., Barthelmes, A., et al. (2020). Peatland protection and restoration are key for climate change mitigation. Environ. Res. Lett. 15:104093. doi: 10.1088/1748-9326/ABAE2A
Khiari, L., and Parent, L. E. (2005). Phosphorus transformations in acid light-textured soils treated with dry swine manure. Can. J. Soil Sci. 85, 75–87. doi: 10.4141/S03-049
Körner, S., Das, S. K., Veenstra, S., and Vermaat, J. E. (2001). The effect of pH variation at the ammonium/ammonia equilibrium in wastewater and its toxicity to Lemna gibba. Aquat. Bot. 71, 71–78. doi: 10.1016/S0304-3770(01)00158-9
Lemming, C., Oberson, A., Magid, J., Bruun, S., Scheutz, C., Frossard, E., et al. (2019). Residual phosphorus availability after long-term soil application of organic waste. Agric. Ecosyst. Environ. 270-271, 65–75. doi: 10.1016/j.agee.2018.10.009
Li, Y., Fang, F., Wei, J., Wu, X., Cui, R., Li, G., et al. (2019). Humic acid fertilizer improved soil properties and soil microbial diversity of continuous cropping Peanut: a three-year experiment. Sci. Rep. 9, 12014–12019. doi: 10.1038/s41598-019-48620-4
Limwikran, T., Kheoruenromne, I., Suddhiprakarn, A., Prakongkep, N., and Gilkes, R. J. (2018). Dissolution of K, ca, and P from biochar grains in tropical soils. Geoderma 312, 139–150. doi: 10.1016/J.GEODERMA.2017.10.022
Lindsay, W. L., and Stephenson, H. F. (1959). Nature of the reactions of Monocalcium phosphate monohydrate in soils: II. Dissolution and precipitation reactions involving Iron, aluminum, manganese, and calcium. Soil Sci. Soc. Am. J. 23, 18–22. doi: 10.2136/SSSAJ1959.03615995002300010013X
López-Rayo, S., Laursen, K. H., Lekfeldt, J. D. S., Delle Grazie, F., and Magid, J. (2016). Long-term amendment of urban and animal wastes equivalent to more than 100 years of application had minimal effect on plant uptake of potentially toxic elements. Agric. Ecosyst. Environ. 231, 44–53. doi: 10.1016/j.agee.2016.06.019
Luo, W., Qian, L., Liu, W., Zhang, X., Wang, Q., Jiang, H., et al. (2021). A potential mg-enriched biochar fertilizer: excellent slow-release performance and release mechanism of nutrients. Sci. Total Environ. 768:144454. doi: 10.1016/J.SCITOTENV.2020.144454
Maguire, R. O., Hesterberg, D., Gernat, A., Anderson, K., Wineland, M., and Grimes, J. (2006). Liming poultry manures to decrease soluble phosphorus and suppress the Bacteria population. J. Environ. Qual. 35, 849–857. doi: 10.2134/jeq2005.0339
Mandal, A., Patra, A. K., Singh, D., Swarup, A., and Ebhin Masto, R. (2007). Effect of long-term application of manure and fertilizer on biological and biochemical activities in soil during crop development stages. Bioresour. Technol. 98, 3585–3592. doi: 10.1016/j.biortech.2006.11.027
Mari, I., Ehaliotis, C., Kotsou, M., Chatzipavlidis, I., and Georgakakis, D. (2005). Use of sulfur to control ph in composts derived from olive processing by-products. Comp. Sci. Utiliz. 13, 281–287. doi: 10.1080/1065657X.2005.10702252
Mazeika, R., Dambrauskas, T., Baltakys, K., Mikolajunas, M., Staugaitis, G., Virzonis, D., et al. (2016). Molecular and morphological structure of poultry manure derived Organo-mineral fertilizers (OMFs). ACS Sustain. Chem. Eng. 4, 4788–4796. doi: 10.1021/acssuschemeng.6b00984
Moritsuka, N., Yanai, J., Mori, K., and Kosaki, T. (2004). Biotic and abiotic processes of nitrogen immobilization in the soil-residue interface. Soil Biol. Biochem. 36, 1141–1148. doi: 10.1016/J.SOILBIO.2004.02.024
Ndegwa, P. M., Vaddella, V. K., Hristov, A. N., and Joo, H. S. (2009). Measuring concentrations of Ammonia in ambient air or exhaust air stream using acid traps. J. Environ. Qual. 38, 647–653. doi: 10.2134/JEQ2008.0211
Oehl, F., Frossard, E., Fliessbach, A., Dubois, D., and Oberson, A. (2004). Basal organic phosphorus mineralization in soils under different farming systems. Soil Biol. Biochem. 36, 667–675. doi: 10.1016/j.soilbio.2003.12.010
Pare, M. C., Allaire, S. E., Khiari, L., and Parent, L.-E. (2010). Improving physical properties of Organo-mineral fertilizers: substitution of peat by pig slurry composts. Appl. Eng. Agric. 26, 447–454. doi: 10.13031/2013.29945
Parent, L. E., Khiari, L., and Pellerin, A. (2003). The P fertilization of potato: increasing agronomic efficiency and decreasing environmental risk. Acta Hortic. 627, 35–41. doi: 10.17660/ActaHortic.2003.627.3
Penn, C. J., and Camberato, J. J. (2019). A critical review on soil chemical processes that control how soil ph affects phosphorus availability to plants. Agriculture (Switzerland) 9:120. doi: 10.3390/agriculture9060120
Piccolo, A. (2002). The supramolecular structure of humic substances: a novel understanding of humus chemistry and implications in soil science. Adv. Agron. 75, 57–134. doi: 10.1016/s0065-2113(02)75003-7
Pizzanelli, S., Calucci, L., Forte, C., and Borsacchi, S. (2023). Studies of organic matter in composting, vermicomposting, and anaerobic digestion by 13C solid-state NMR spectroscopy. Appl. Sci. 13:2900. doi: 10.3390/app13052900
Rady, M. M. (2012). A novel organo-mineral fertilizer can mitigate salinity stress effects for tomato production on reclaimed saline soil. S. Afr. J. Bot. 81, 8–14. doi: 10.1016/j.sajb.2012.03.013
Richards, J. E., Daigle, J.-Y., LeBlanc, P., Paulin, R., and Ghanem, I. (1993). Nitrogen availability and nitrate leaching from organo-mineral fertilizers. Can. J. Soil Sci. 73, 197–208. doi: 10.4141/cjss93-022
Rodrigues, M. M., Viana, D. G., Oliveira, F. C., Alves, M. C., and Regitano, J. B. (2021). Sewage sludge as organic matrix in the manufacture of organomineral fertilizers: physical forms, environmental risks, and nutrients recycling. J. Clean. Prod. 313:127774. doi: 10.1016/j.jclepro.2021.127774
Saarikoski, H., Mustajoki, J., Hjerppe, T., and Aapala, K. (2019). Participatory multi-criteria decision analysis in valuing peatland ecosystem services—trade-offs related to peat extraction vs. pristine peatlands in southern Finland. Ecol. Econ. 162, 17–28. doi: 10.1016/j.ecolecon.2019.04.010
Sahrawat, K. L. (2008). Factors affecting nitrification in soils. Commun. Soil Sci. Plant Anal. 39, 1436–1446. doi: 10.1080/00103620802004235
Sakurada, R., Batista, L. M. A., Inoue, T. T., Muniz, A. S., and Pagliar, P. H. (2016). Organomineral phosphate fertilizers: agronomic efficiency and residual effect on initial corn development. Agron. J. 108, 2050–2059. doi: 10.2134/agronj2015.0543
Schmilewski, G. (2008). The role of peat in assuring the quality of growing media. Mires Peat 3, 1–8.
Shen, W., Lin, X., Shi, W., Min, J., Gao, N., Zhang, H., et al. (2010). Higher rates of nitrogen fertilization decrease soil enzyme activities, microbial functional diversity and nitrification capacity in a Chinese polytunnel greenhouse vegetable land. Plant Soil 337, 137–150. doi: 10.1007/s11104-010-0511-2
Sica, P., Kopp, C., Müller-Stöver, D. S., and Magid, J. (2023). Acidification and alkalinization pretreatments of biowastes and their effect on P solubility and dynamics when placed in soil. J. Environ. Manag. 333:117447. doi: 10.1016/j.jenvman.2023.117447
Sistla, S. A., Asao, S., and Schimel, J. P. (2012). Detecting microbial N-limitation in tussock tundra soil: implications for Arctic soil organic carbon cycling. Soil Biol. Biochem. 55, 78–84. doi: 10.1016/j.soilbio.2012.06.010
Sitzmann, T., Zavattaro, L., Tagliavini, S., Celi, L., Padoan, E., Moretti, B., et al. (2023). Suitability of recycled organic materials in Organo-mineral fertilizers manufacturing: a discussion about organic Geogenic material replacement. SSRN. doi: 10.2139/SSRN.4580695
Smith, W. B., Wilson, M., and Pagliari, P. (2020). “Organomineral fertilizers and their application to field crops” in Animal manure: Production, characteristics, environmental concerns, and management. eds. H. M. Waldrip, P. H. Pagliari, and Z. He (New York: John Wiley & Sons, Ltd.)
Taparia, T., Hendrix, E., Nijhuis, E., de Boer, W., and van der Wolf, J. (2021). Circular alternatives to peat in growing media: a microbiome perspective. J. Clean. Prod. 327:129375. doi: 10.1016/J.JCLEPRO.2021.129375
Teng, Z., Zhu, J., Shao, W., Zhang, K., Li, M., and Whelan, M. J. (2020). Increasing plant availability of legacy phosphorus in calcareous soils using some phosphorus activators. J. Environ. Manag. 256:109952. doi: 10.1016/j.jenvman.2019.109952
Traoré, O. Y. A., Kiba, D. I., Arnold, M. C., Fliessbach, A., Oberholzer, H. R., Nacro, H. B., et al. (2016). Fertilization practices alter microbial nutrient limitations after alleviation of carbon limitation in a ferric Acrisol. Biol. Fertil. Soils 52, 177–189. doi: 10.1007/s00374-015-1061-9
Turan, N. G. (2008). The effects of natural zeolite on salinity level of poultry litter compost. Bioresour. Technol. 99, 2097–2101. doi: 10.1016/j.biortech.2007.11.061
Vujinović, T., Zanin, L., Venuti, S., Contin, M., Ceccon, P., Tomasi, N., et al. (2020). Biostimulant action of dissolved humic substances from a conventionally and an organically managed soil on nitrate Acquisition in Maize Plants. Front. Plant Sci. 10:652. doi: 10.3389/fpls.2019.01652
Zaccone, C., Plaza, C., Ciavatta, C., Miano, T. M., and Shotyk, W. (2018). Advances in the determination of humification degree in peat since Achard (1786): applications in geochemical and paleoenvironmental studies. Earth Sci. Rev. 185, 163–178. doi: 10.1016/j.earscirev.2018.05.017
Zeller, V., Lavigne, C., D’Ans, P., Towa, E., and Achten, W. M. J. (2020). Assessing the environmental performance for more local and more circular biowaste management options at city-region level. Sci. Total Environ. 745:140690. doi: 10.1016/j.scitotenv.2020.140690
Zheng, L., Chen, H., Wang, Y., Mao, Q., Zheng, M., Su, Y., et al. (2020). Responses of soil microbial resource limitation to multiple fertilization strategies. Soil Tillage Res. 196:104474. doi: 10.1016/J.STILL.2019.104474
Keywords: organo-mineral fertilizers, biosolid, bio-waste, peat replacement, nutrient immobilization, pruning compost, municipal solid waste, vermicompost
Citation: Sitzmann TJ, Sica P, Grignani C and Magid J (2024) Testing biowaste materials as peat replacement in organo-mineral fertilizers. Front. Sustain. Food Syst. 8:1330843. doi: 10.3389/fsufs.2024.1330843
Edited by:
Rachel E. Thorman, Agricultural Development Advisory Service (United Kingdom), United KingdomReviewed by:
James Steve Robinson, University of Reading, United KingdomRuben Sakrabani, Cranfield University, United Kingdom
Copyright © 2024 Sitzmann, Sica, Grignani and Magid. This is an open-access article distributed under the terms of the Creative Commons Attribution License (CC BY). The use, distribution or reproduction in other forums is permitted, provided the original author(s) and the copyright owner(s) are credited and that the original publication in this journal is cited, in accordance with accepted academic practice. No use, distribution or reproduction is permitted which does not comply with these terms.
*Correspondence: Tomas Javier Sitzmann, dG9tYXMuc2l0em1hbm5AdW5pdG8uaXQ=