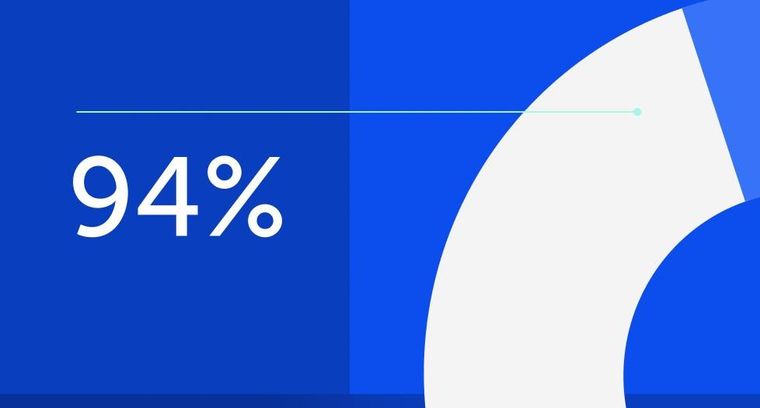
94% of researchers rate our articles as excellent or good
Learn more about the work of our research integrity team to safeguard the quality of each article we publish.
Find out more
ORIGINAL RESEARCH article
Front. Sustain. Food Syst., 01 March 2024
Sec. Sustainable Food Processing
Volume 8 - 2024 | https://doi.org/10.3389/fsufs.2024.1284069
This article is part of the Research TopicAlternative Protein Source For A Sustainable And Healthy NutritionView all 12 articles
Probiotics, which offer various health benefits can face challenges in terms of stability during food processing, storage, and gastrointestinal digestion. Therefore, this study aimed to improve the stability and survival of probiotics during various processing conditions and storage. To address this issue, the study was designed to microencapsulate Lactobacillus reuteri DSM 17938 within plant proteins (specifically rice protein (RP) and pea protein (PeP)) and their Maillard reaction conjugated with inulin by spray-drying. The encapsulation efficiency (EE%), stability during storage and temperature, and the viability after simulated gastrointestinal digestion of the microcapsules were examined. The results demonstrate that individual proteins exhibited lower EE%; however, the Maillard conjugates showed increased EE%, with RC (rice protein conjugates) displaying a higher EE% (96.99%) than PC (pea protein conjugates) (92.87%) (p < 0.05). Fourier Transform Infrared Spectroscopy verified the interaction between different functional groups of the proteins and Maillard conjugated and indicated the successful encapsulation of Lactobacillus reuteri DSM 17938 cells. The results also suggested that RC-encapsulated probiotic cells exhibited maximum survival upon gastrointestinal transit, with a decline of only 1.24 and 1.52 log CFU/g after gastric and complete simulated gastrointestinal digestion, respectively. The viability of probiotics encapsulated with RC and PeC showed improvement compared to those encapsulated with RP and PeP, particularly during refrigerated and room temperature storage, thermal challenge, and simulated gastrointestinal transit. Overall, these findings suggest that plant proteins and prebiotic inulin conjugates could serve as promising new encapsulation matrices for the encapsulation of probiotics in food applications.
Probiotics are a group of microorganisms that exert positive biological effects on the host when consumed in appropriate quantities (Hill et al., 2014). According to the previous reports, probiotic strains can treat constipation, decrease cholesterol levels, regulate the immune system, and maintain human gut health by preventing the growth of harmful bacteria (Kaur et al., 2021). A minimum of 108 colony forming units (CFU) per mL or g of probiotic food is required to exert a positive effect (Hill et al., 2014). However, several factors affect the survivability of probiotic strains during processing, storage, and gastrointestinal transit, ultimately limiting their beneficial effects at the targeted area (Arslan et al., 2015; Markowiak and Śliżewska, 2017). Therefore, maintaining the viability of probiotics during all stages of production until consumption is of paramount importance to food producers and scientists worldwide.
Microencapsulation is a promising technique for bacterial cell protection. Several studies have investigated the protective role of microencapsulation against the adverse conditions faced by probiotics (Ashwar et al., 2018; Fu et al., 2021; Devarajan et al., 2022; Hadidi et al., 2022; Yeung et al., 2023). The successful application of microencapsulation plays a major role in improving the viability and availability of cells throughout the stages of preparation and extends through the human gastrointestinal tract (Kailasapathy, 2002; Sarao and Arora, 2017). The viability of probiotics in food processing and formulations can be affected by the type of encapsulation material used (Loyeau et al., 2018; Ahmad et al., 2019; de Araújo Etchepare et al., 2020; Devarajan et al., 2022). Different food materials, such as milk proteins, plant proteins, and polysaccharides, have been used as wall materials for the encapsulation of probiotics (Afzaal et al., 2021; Rajam and Subramanian, 2022; Xu et al., 2022). Complex polysaccharides with prebiotic properties and various dietary proteins are the most frequently used wall materials for the microencapsulation of probiotic microorganisms (Fernanda et al., 2016). The food industry has been using spray-drying microencapsulation successfully for many years. The process generally involves the dispersion or dissolution of a core material in a solution of wall material to form a fluid mixture. This mixture is then sprayed into a heated chamber. As the solvent of the wall material evaporates, the small droplets transform into solid particles with the core material entrapped within the wall material matrix (Arslan et al., 2015; Burger et al., 2022). Spray drying is one of the oldest, most common, and economical techniques for producing large quantities of viable cells. Spray drying is frequently used as the optimal method for microencapsulation (Vaniski et al., 2021).
Protein-based delivery systems, in addition to their disadvantages of precipitation and aggregation (pH and ionic strength effects), exhibit poor stability against digestive enzymes in the gastrointestinal tract and are thus easily degraded, ultimately leading to a burst release of encapsulated bioactive compounds in the gastric environment instead of in the intestine (Marson et al., 2020; Nooshkam and Varidi, 2020). Although crosslinking proteins with other molecules could address the above issue, owing to health concerns, the application of chemicals as crosslinkers is limited (Li and Huang, 2015). Similarly, prebiotic oligosaccharides are known to have weak physical interactions with probiotics; therefore, microcapsules exert limited improvement in encapsulation efficiency (EE), and insufficient protective effects are delivered to encapsulated probiotic cells (Zhong et al., 2021). Therefore, the conjugation of proteins with carbohydrates via the Maillard reaction could improve protein stability and prebiotic interactions with proteins, improving the overall EE and microcapsule stability (Zhong et al., 2021). Various dietary proteins and polysaccharides and their conjugates have been used as wall materials for microencapsulation (Ahmad et al., 2019; Devarajan et al., 2022). However, researchers are now focusing on new sources of the wall matrix, specifically from plant proteins, owing to their sustainability and low cost of production compared to animal proteins (Hadidi et al., 2023; Islam et al., 2023). Plant protein isolates can be used to increase the nutritional value and functional properties of food (Lam et al., 2018). Moreover, combining plant proteins with prebiotics can improve the stability of the encapsulated probiotics (Gharibzahedi and Smith, 2021).
Pea and rice are good protein sources owing to their high nutritional value and bioavailability (Pietrysiak et al., 2018; Kiran et al., 2023). Pea and rice proteins are suitable for encapsulation due to their high solubility, ability to absorb water and fat, capacity for emulsion stabilization, and capability to form gels (Burger et al., 2022). The globulins of pea and rice proteins possess all the functional properties necessary for their successful incorporation into microencapsulation systems as wall materials (Hadidi et al., 2023; Islam et al., 2023). Recent studies have reported the utilization of pea and rice proteins for probiotic encapsulation (Varankovich et al., 2017; Vaniski et al., 2021). Nevertheless, to the best of our knowledge, the application of rice and pea proteins and prebiotic conjugates as wall materials for probiotics and their comparison with native proteins have not been reported. Therefore, this study aimed to investigate the utility of rice protein (RP), pea protein (PeP), their conjugates (RC and PeC), and prebiotics as wall materials for encapsulating the probiotic strain, Lactobacillus reuteri DSM 17938. L. reuteri DSM 17938 was chosen because it has been extensively researched, shows promising health benefits for gastrointestinal and immune health, has a well-known survival profile in the gut, and has a proven safety record in clinical trials. The physicochemical characteristics, morphology, and impact of RP, PeP, RC, and PeC on probiotic survival during gastrointestinal digestion were evaluated. Furthermore, the viability and stability of the probiotic organisms during storage at different processing temperatures and during transit via simulated gastrointestinal digestion (SGID) were investigated.
RP (Protein content = 91.2%) and PeP (Protein content = 85.93%) were procured from ET Proteins (Public Republic of China). The prebiotic, inulin, was procured from the NOW Foods Company (Bloomingdale, IL, United States). DeMan, Rogosa, and Sharpe (MRS) medium were obtained from HiMedia Laboratories (Mumbai, India). The enzymes used to simulate gastrointestinal digestion, such as pepsin, trypsin, pancreatin, and bile salts, and other analytical chemicals and reagents were purchased from Sigma Aldrich (St. Louis, MO, USA) and were of analytical grade. Lactobacillus reuteri DSM 17938 https://www.sciencedirect.com/topics/food-science/lactobacillus was obtained from the Department of Food Science, UAEU.
The rice and pea protein conjugates (RC and PeC) were produced via the Maillard reaction according to a previously optimized protocol in our laboratory (data not shown) following the protocols as described by Guo et al. (2022). Briefly, RP and PeP were mixed with inulin in a ratio of 1:1.25 (w/w) in deionized water. The pH was then adjusted to 9.0 with 1 M NaOH and the mixtures were stored at 4°C overnight for complete hydration. After this hydrated solutions were freeze dried overnight, and were heated at 80°C for 16 h (as per previously optimized conditions) under a controlled relative humidity of 79%. The resulting RC and PeC were stored under refrigerated conditions until further use.
Encapsulation of the probiotic bacterial culture, L. reuteri DSM 17938, was performed according to the methodology described by Algaithi et al. (2022). Briefly, the L. reuteri DSM 17938 strain was inoculated in sterile MRS broth and incubated for 18 h at 37°C. The cells were harvested via centrifugation (Digicen 21 R, Ortoalresa, Madrid, Spain) at 5000 × g for 5 min at room temperature and then washed twice with sterile peptone water to remove any lingering traces of spent broth. Thereafter, the solution was resuspended in sterile saline (0.9%) to obtain a bacterial cell suspension of 1011–1012 CFU/mL. RP, PeP, and their conjugates (RC and PeC) were rehydrated in sterile deionized water at 5% (w/v) and L. reuteri DSM 17938 probiotic cells were added to obtain a final cell count of approximately 108–9 CFU/mL. To facilitate the microencapsulation process, the mixture was stirred under sterile conditions for 2 h at 200 rpm and then spray dried using a pilot-scale spray dryer (Mini Spray Dryer B-290; BUCHI, Switzerland). The following parameters were used: an inlet air temperature of 180°C, an outlet temperature of 91°C, and a feed flow rate of 8.0 mL/min. The resulting powders were kept in airtight containers and stored under two storage conditions, refrigerated storage (4–8°C) and room temperature storage (25°C), for further analysis.
The EE was determined according to the methodology described by Mudgil et al. (2022). Briefly, the viability of the L. reuteri DSM 17938 probiotic strain upon encapsulation using RP, PeP, RC, and PeC as wall materials was determined before and after the spray–drying process based on dry weight. Appropriate serial dilutions were prepared and pour plating was performed using sterile MRS agar. The colony forming units (CFU) for the free and encapsulated probiotic strains were determined after 48 h of incubation at 37°C. The EE was determined using the following equation (Eq. 1):
The microstructures of L. reuteri DSM 17938-loaded RP, PeP, RC, and PeC were analyzed after spray drying using scanning electron microscopy (SEM) (JSM-6010PLUS/LA scanning electron microscope, JEOL). Briefly, the samples were placed on an aluminum stub using a double-sided carbon tape and coated with a layer of gold through sputtering (108 Auto Sputter Coater, TED PELLA, INC). Micrographs were recorded under high vacuum to obtain digital images at the desired magnification.
Structural changes in the L. reuteri DSM 17938-loaded RP, PeP, RC, and PeC samples after spray drying were further analyzed via Fourier transform infrared spectroscopy (FTIR) (Spectrum Two UATR, PerkinElmer, Waltham, MA, United States) over the range 450–4,000 cm−1. For each spectrum, 32 scans were recorded. The spectral resolution was set at 4 cm−1.
As mentioned in Section 2.3, the spray-dried bacterial cells were stored under refrigerated (4°C) and room temperature (25°C) conditions for 28 days (4 weeks). Viability was assessed weekly throughout the 28 days of storage via serial dilution and plating onto MRS agar, as described above. Probiotic viability (%) was calculated using Eq. (2).
The probiotic viability of free and encapsulated cells under SGID was determined according to the methods by Ahmad et al. (2019) and Devarajan et al. (2022). Gastric fluid was made to pH 2.0 using 1 M HCl and contained the following chemicals; sodium chloride, potassium chloride and pepsin in concentrations of 94 mM, 13 mM and 2000 IU/mL of the fluid. Similarly, SIF (pH; 8.0) contained calcium chloride, potassium chloride, sodium bicarbonate, sodium chloride, bile salt, along with 4,000 mg pancreatin and 261 units of pancreatic lipase in a concentration of 3 mM, 10 mM. 85 mM 164 mM and 3.1 mM, respectively.
Briefly, free cells and encapsulated L. reuteri DSM 17938-loaded RP, PeP, RC, and PeC samples (approximately 8.0 log CFU/mL) were incubated with simulated gastric fluid (SGF) for 2 h followed by simulated intestinal fluid (SIF) for 3 h at 37°C. An aliquot of the sample was collected at each digestion stage, and cell viability was determined after pour plating with MRS agar, as described in Section 2.6.1. Viable cells were counted via plating onto MRS agar after 48 h of incubation at 37°C. The effects of simulated gastric digestion (SGD) and gastrointestinal digestion (SGID) processes on the viability of L. reuteri DSM 17938 in the free form and when encapsulated in the RP, PeP, RC, and PeC matrices were determined by calculating the Log CFU/g of free L. reuteri DSM 17938 and the encapsulated cells using Eq. (3):
Thermal stability tests were performed according to Guo et al. (2022). Briefly, powdered encapsulated materials were suspended in sterile peptone water at approximately 8.0 Log CFU/g. The mixture was then heated at 50°C and 80°C for 5 and 10 min, respectively. Cell viability before and after thermal treatment was calculated as described in Section 2.6.1 and 2.6.2, by plating appropriate dilutions of cells onto MRS agar. Cell viability was calculated as previously described.
Microencapsulation of the probiotic was performed in three batches using the protein and prebiotic conjugates. Data analysis was carried out using SPSS 28.0 (IBM, Chicago, IL, United States), with one-way analysis of variance (ANOVA) and Tukey’s multiple range test for separating the means between samples (p < 0.05).
The effectiveness of microencapsulating L. reuteri DSM 17938 probiotic cells using plant-based proteins (i.e., RP and PeP) and their inulin-based conjugates (i.e., RC and PeC) was assessed according to their cell viability; the results are presented in Figure 1. No significant differences were found between the EEs of the native rice and pea proteins, with EE values of 80.16 and 83.80%, respectively. However, the Maillard conjugates (RP + inulin and PeP + inulin) exhibited increased EE, with RC displaying a better EE% (96.99%) than PC (92.87%) (p < 0.05). Therefore, the encapsulation of probiotics in protein microparticles was less effective than encapsulation in conjugated protein microparticles. Protein/polysaccharide complexes or conjugates have been reported to be useful for the microencapsulation of probiotics due to their good flexibility while entrapping various types of probiotic cells, ultimately improving their survivability (Praepanitchai et al., 2019; Sun et al., 2022). For instance, whey proteins and isomalto-oligosaccharide-based Maillard reaction conjugates displayed higher EE for Lactobacillus rhamnosus than their native mixture, which aligns with the results of the current study (Liu et al., 2016). Similar results have been reported by other researchers, where Maillard reaction product (MRP) conjugates served as better wall materials than native proteins for the encapsulation of probiotics (Fu et al., 2021; Yeung et al., 2023). As reported previously, MRP conjugation leads to the creation of glycoproteins with balanced hydrophobic and hydrophilic interactions, and is better at lowering interfacial tension than native proteins, resulting in high encapsulation yields (Liu et al., 2016).
Figure 1. Encapsulation efficiency (%) of L. reuteri DSM 17938 into RP, PeP, and RC, PeC conjugate microparticles, after spray drying. Keynotes: RP: Rice protein; PeP: Pea proteins; RC: Rice conjugate; PeC: Pea conjugate. Different letters over the bars indicate statistically significant differences among samples (p < 0.05) (n = 3).
SEM was used to examine the surface morphology of L. reuteri DSM 17938 free cells and cells encapsulated in RP, RC, PeP, and PeC. As shown in Figure 2A, free-cell micrographs revealed the typical morphological features of L. reuteri DSM 17938 cells: rod shaped, colonized in groups, and attached to each other. In contrast, native inulin particles had an irregular spherical morphology with a slightly smooth surface (Figure 2B). Figures 2C,F show the native rice and pea proteins, respectively. RP had round globular structures with rough surfaces and some cavities, while PeP had smaller globular structures with smooth margins. Figures 2D,E show the probiotics entrapped in RP and RC, respectively; L. reuteri DSM 17938 could not be seen even at higher magnifications (data not shown), indicating that the probiotic cells were successfully entrapped within the wall materials. Interestingly, upon encapsulation, the RP and RC particles exhibited smooth surfaces, contrary to those of the native rice proteins, RP-pro (Figure 2D) and RC-pro (Figure 2E); this could be attributed to the spray drying procedure, which permitted the rapid evaporation of water. The L. reuteri DSM 17938-loaded microparticles appeared to be stable in the interior cavities of the protein-prebiotic conjugate matrix. Moreover, prebiotic conjugation with RP and PeP decreased the porosity of the wall materials by filling the pores of the matrix, ultimately providing better coverage for the probiotics. The use of prebiotic-based wall materials in the encapsulation process can result in the formation of a protective layer around the probiotic bacteria, which can affect the overall morphology of the encapsulated particles. Similar entrapment results were obtained when Lactobacillus plantarum and Lactobacillus casei were loaded into soybean protein microparticles via spray drying (González-Ferrero et al., 2018). Furthermore, Mao et al. (2018) reported that the encapsulation of Bifidobacterium longum in Maillard reaction conjugates of soy protein isolates and carrageenan resulted in spherical cavity-like structures upon spray drying, providing better protection to probiotic cells.
Figure 2. SEM images of L. reuteri DSM 17938 free cells (A), Inulin (B), native RP (C) RP-pro (D), and RC-pro (E), native PeP (F) PeP-pro (G), and PeC-pro (H) microparticles after spray drying. Keynotes: RP: Rice protein; RP- pro: Rice protein with probiotics; RC-pro: Rice conjugate with probiotics; PeP: Pea protein; PeP- pro: Pea protein with probiotics; PeC-pro: Pea conjugate with probiotics.
To interpret the interaction between the probiotics and wall materials, Fourier transform infrared spectra were recorded in the range 450–4,000 cm−1. The infrared spectra of the wall materials before and after probiotic encapsulation are presented in Figure 3A for RP and RC, and Figure 3B for PeP and PeC. Inulin sample displayed a characteristic peak between 3,267 and 3,340 cm−1, which was attributed to stretching in the OH region (Olech et al., 2023). Proteins display unique spectral characteristics, with the amide I and II bands being the most prominent and occurring in the approximate wavenumber ranges of 1,600–1,500 cm−1 and 1700–1,600 cm−1, respectively. In both the PeP and RP samples, the distinct bands at 1645 cm−1, 1,652 cm−1, 1,542 cm−1, and 1,529 cm−1 originated from the stretching of C=O bonds in amide I and the vibrations of N-H bonds in amide II, respectively (Devarajan et al., 2022). In addition, the Maillard reaction consumed some carbonyl and amino groups, resulting in the creation of Schiff bases (C=N), pyrazines (C–N), and Amadori compounds (C=O). These changes led to variations in the intensity and positioning of peaks related to amide A, amide I, and amide II indicating conjugation of proteins with carbohydrates (Li et al., 2023). The intense absorption peak around 2,925 cm−1 for free cells could be mainly attributed to antisymmetric stretching vibration and bending vibrations of the C–H groups, and bending and vibrations of lipid molecules in the cell membrane (Chen et al., 2023). After conjugation, the intensity of the peak at 1034 cm−1 for RP and RC and 1,029 cm−1 for PeP and PeC increased, indicating the occurrence of structural and conformational changes upon conjugation. As the peak at 1301–1034 cm − 1 was attributed to the stretching vibrations of C– O and C– C bonds, together with the bending of C– H bonds, and identified absorption peaks serve for accurate indications for carbohydrates. Which are organic compounds composed of carbon, hydrogen, and oxygen atoms and the presence of C– O and C– C bonds in the absorption peaks suggests the presence of carbohydrates in the protein sample or complex protein further confirming conjugation of proteins with carbohydrates. Moreover, as observed in both spectra upon encapsulation, the characteristic peak of free cells at 2925 cm−1 decreased, suggesting their entrapment in the wall materials. Such findings suggest no interaction occurred between the wall materials and probiotics during microencapsulation; hence, the encapsulated probiotics could be easily released during gastrointestinal transit. Overall, upon encapsulation, no noticeable change was observed, except a slight peak shift.
Figure 3. FTIR spectra of Prebiotic inulin, native RP (A), native RC (B), Prebiotic, L. reuteri DSM 17938 free cells, and the different L. reuteri DSM 17938 -loaded RP-pro, and RC-pro conjugate microparticles, after spray drying. For keynotes, please see legend for Figure 2.
The harsh condition of the gastrointestinal tract serves as the main challenge in the successful delivery and survival of probiotics. Therefore, the survivability of free and encapsulated L. reuteri DSM 17938 probiotic cells in the RP, PeP, RC, and PeC microparticles after the gastric and complete-SGID phases was analyzed according to cell viability (%), and the obtained data are presented in Figure 4. The viability of free L. reuteri DSM 17938 displayed a significant decline of 4.52 log cells from an initial log count of 8.3 to 3.78 log CFU/g. However, the pea protein and conjugate did not result in any significant differences in probiotic cell survivability. Overall, cells encapsulated in PeP showed a 2.1 log reduction after gastric transit and 3.98 log reduction after complete SGID. Similarly, PeC-encapsulated probiotic cells showed a decline of 1.67 log CFU/g and 3.84 log reduction upon completion of SGID. Of note, PeC-encapsulated probiotic cells survived better under gastric conditions than PeP-encapsulated probiotic cells (Figure 4). Furthermore, probiotic cells encapsulated in the RP and RC matrices showed better survival than those encapsulated in PeP and its conjugate. Overall, RP-encapsulated probiotic cells displayed 1.57 and 2.09 log reductions after gastric and complete SGID, respectively. These results suggest that RC-encapsulated probiotic cells exhibited maximum survival upon gastrointestinal transit, with a decline of only 1.24 and 1.52 log CFU/g, respectively after gastric and complete SGID. Overall, encapsulation in protein-prebiotic conjugates, such as in RC, can protect L. reuteri DSM 17938 under both gastric and complete-SGID conditions for their establishment in the intestine as well as their proliferation. The improved survival of probiotic cells encapsulated in the RC matrix could be ascribed to the fact that RC-microencapsulated probiotic cells were released in a controlled manner and maintained their viability and functionality during gastrointestinal transit. The results of the current study are consistent with those of a previous study that confirmed the enhanced viability of L. plantarum cells when encapsulated in whey protein-dextran conjugates were obtained via the Maillard reaction (Guo et al., 2022). Similarly, Loyeau et al. (2018) reported the enhanced viability of Bifidobacterium animalis subsp. lactis INL1 using whey proteins and dextran conjugates. Another study reported similar SGID findings when the Maillard reaction between soy protein isolate (SPI) and Ι-carrageenan (IC) was used to encapsulate Bifidobacterium longum (Mao et al., 2018); probiotic viability decreased by 2.38 log under simulated gastric conditions. Gunzburg et al. (2020) reported 8 logs stomach acid protection of L. casei that had been encapsulated by cellulose sulphate. These authors confirm that this improved survival artificial gastric juice also translates into the in vivo situation in mice that have been gavaged with cellulose sulphate encapsulated bacteria showing better survival and colonization. Overall, the conjugation of rice protein with the prebiotic, inulin, resulted in a conjugate with enhanced EE and enhanced protection under harsh SGID conditions.
Figure 4. Viability of L. reuteri DSM 17938 free and encapsulated cells into RP, PeP, and RC, PeC conjugate microparticles, upon undergoing in vitro simulated gastrointestinal digestion. Pea conjugate. The level of different * at different phases indicate statistical difference among samples (p < 0.05) (n = 3). For keynotes, please see legend for Figure 2.
The success of probiotic supplements or products is entirely related to the viable counts maintained during the storage of food products. Therefore, a high number of live cells must be maintained throughout the shelf-life of probiotic food products. Microencapsulation has been reported to effectively increase probiotic survival during storage (Feng et al., 2020). To comparatively evaluate the effects of native proteins and their conjugates on probiotic survival, studies at room temperature (25°C) and refrigeration temperature (4°C) were performed weekly for up to 28 days (4 weeks) (Figure 5). Storage at 25°C for 28 days resulted in a decline in viable count among the encapsulated samples. The maximum decline was observed in samples encapsulated in the PeP wall matrix, with a reduction of 2.68 log CFU/g after 28 days of storage. However, the reduction in the PeC sample was less than that in the native protein-encapsulated sample, with the viable cell count reduced by 1.78 log CFU/g after 28 days of storage. Probiotic cells encapsulated in native RP were better protected than those encapsulated in native PeP based on a total reduction of only 0.9 log CFU/g, which is markedly better than that obtained with PeP conjugates and PeC. Overall, RC-encapsulated probiotic cells displayed the maximum survival rate throughout storage, and only a decline of 0.73 log CFU/g after 8 days of storage. The RP and RC microparticles showed significantly higher protective effects based on the slower decline in L. reuteri DSM 17938 with increasing storage time. Such findings suggest that encapsulation in these matrices is a beneficial strategy to increase cell viability during storage. The results obtained in this study were comparable to those obtained in another study, where L. plantarum 21,805 was encapsulated in whey protein–dextran conjugates. Notably, a decline of approximately 4.86 log CFU/mL was found after 30 days of storage at room temperature (Guo et al., 2022). Similar results were reported for L. reuteri DSM 17938 encapsulated in camel milk proteins, where a better survival was observed after continuous storage for up to 90 days (Algaithi et al., 2022). Similarly, a study on the encapsulation of B. animalis in whey protein-dextran conjugates revealed substantial protection of the encapsulated cells even after 12 months of storage at room temperature (Loyeau et al., 2018). According to a study on the encapsulation of B. animalis in soy protein isolate and carrageenan conjugates, better protection was achieved with the conjugate than the native soy protein-carrageenan mixture at 20°C (Mao et al., 2018). Allahdad et al. (2022) reported that a mixture of pea and rice protein maintained a high concentration of viable probiotics during a 143 days storage period. Overall, these results indicate that plant proteins, upon conjugation with inulin, provide a better encapsulating matrix for the efficient protection and delivery of probiotic bacteria.
Figure 5. Storage stability of L. reuteri DSM 17938 into RP, PeP, and RC, PeC conjugate microparticles, during 28 days of storage at (A) room temperature 25°C; (B) refrigerator 4°C. The level of different * at different phases indicate statistical difference among samples (p < 0.05) (n = 3). For keynotes, please see legend for Figure 2.
The results regarding the stability of encapsulated probiotic cells under refrigerated storage (4°C) for 28 days suggested better protection than storage at room temperature based on the higher cell viability (Figure 5B). During storage at room temperature, a maximum decline of 2.09 log CFU/g was observed in PeP-encapsulated samples, followed by RP-, PeC-, and RC-encapsulated samples, with declines of 1.23, 1.22, and 1.18 log CFU/g, respectively. In general, conjugated proteins induced significantly (p < 0.05) better protection of probiotic cells than the native proteins. Furthermore, higher viability of samples was observed at 4°C compared to room temperature. These findings align with those of other studies that suggested better survival of encapsulated cells at refrigeration temperatures than at room temperature (Loyeau et al., 2018; Mao et al., 2018; Guo et al., 2022; Yeung et al., 2023).
The continuous decline in the number of cells over prolonged storage at room temperature is largely attributed to injuries induced by the high temperature of spray drying, intrinsic temperature and desiccation resistance of the probiotic strain used, the characteristics of the encapsulated material, moisture content of the particles produced, and packaging conditions (Vaniski et al., 2021). The higher protection by conjugated proteins could be due to their better moisture retention capacity imparted by prebiotics, which prevented excessive drying and improved cell viability during storage (González-Ferrero et al., 2018). Overall, the results of this study suggest that conjugated proteins can be used for probiotic microencapsulation to increase the viability of probiotic bacterial cells.
Microencapsulation of probiotic bacteria is not only used to increase the long-term survival of cells during storage, but also to overcome the thermal conditions applied during food processing (González-Ferrero et al., 2018). The microencapsulation technology has proven to be a highly preferred option for protecting probiotics, boosting a decrease in thermal stress and improving the thermal tolerance of probiotic bacteria (Devarajan et al., 2022). The effect of thermal heating on the viability of probiotic-free and encapsulated L. reuteri DSM 17938 was assessed after heating for 10 min at 50 and 80°C (Figure 6). The microencapsulation of L. reuteri DSM 17938 in the RP, PeP, RC, and PeC groups were found to have significantly different effects on bacterial viability. Further, L. reuteri DSM 17938 -loaded PeP, PC, and RP microparticles could not withstand extreme heat treatments, with RP showing a decline of 1.36 and 1.73 log CFU/g after 5 and 10 min of heating at 50°C (Figure 6A). Interestingly, the ability of probiotic cells to tolerate heat was markedly improved after encapsulation in RC based on a loss of only 0.37 and 0.35 log CFU/g after 5 and 10 min, respectively (Figure 6A). Further, probiotic cells encapsulated in PeP showed a decline of 0.99 and 1.25 log CFU/g after 5 and 10 min of heating, respectively, whereas PeC-encapsulated cells showed a reduction of 0.85 and 1.19, respectively, after 5 and 10 min of heating, indicating better protection conferred by the conjugated proteins compared to native proteins. Overall, RC encapsulation of L. reuteri DSM 17938 provided greater protection than RP, PeP, or PeC alone, effectively reducing heat transfer from the surrounding environment to the cell interior (Ahmad et al., 2019; Algaithi et al., 2022).
Figure 6. Thermal stability of L. reuteri DSM 17938 into RP, PeP, and RC, PeC conjugate microparticles, during 10 min of heat treatment at (A) 50°C; (B) 80°C. Different symbols on the lined at particular time period means statistically significant differences as analyzed via ANOVA (p < 0.05) (n = 3). For keynotes, please see legend for Figure 2.
Figure 6B displays the effect of high temperature treatment (80°C) on the survivability of encapsulated probiotics. PeP-encapsulated probiotics could not withstand high heat treatments, with a reduction of 2.64 and 4.81 log CFU/g after 5 and 10 min of heat treatment at 80°C. Similarly, substantial alterations in the viability of the probiotic were also observed in RP- and PeC-encapsulated cells based on a decline of 1.94 and 1.34 log CFU/g after 10 min, respectively. However, probiotic cells could tolerate high heat treatment (80°C) after encapsulation in the RC matrix, with only a decline of 0.58 log CFU/g after 10 min. These results align with those obtained by Ahmad et al. (2019), who found that encapsulation of probiotics in camel whey protein matrices led to enhanced protection of probiotics from thermal stress induced by 10 min of treatment. Similar results were obtained in a prior study as dextran conjugated whey microparticle led to enhanced thermal protection of L. plantarum probiotic cells compared to free cells (Guo et al., 2022). Loyeau et al. (2018) reported similar results for the viability of Bifidobacterium when encapsulated in whey protein dextran conjugates produced via the Maillard reaction. Based on these results, microencapsulation is an alternative for ensuring good stability of probiotics, as MRP-based protein carbohydrate conjugates are known to possess higher thermal stability than native proteins and can offer better protection to probiotics during processing and in the digestion process (Zhong et al., 2021; Guo et al., 2022).
This research explored the effect of native proteins (pea and rice) and their Maillard conjugates with inulin, formed by wet heating, on encapsulation of L. reuteri DSM 17938 cells by spray drying. All encapsulated L. reuteri DSM 17938 cells exhibited superior viability during simulated gastrointestinal transit. All encapsulated L. reuteri DSM 17938 cells exhibited superior viability upon SGID in these in-vitro studies. RC-encapsulated probiotic cells showed maximum survival with a decline of only 1.24 and 1.52 log CFU/g, respectively after gastric and complete SGID phase. Probiotics loaded in RC exhibited higher levels of storage survivability, which could be attributed to the advantageous microstructure of RC, ultimately offering higher protection. Furthermore, the thermal treatment and storage stability results indicated that RC-encapsulated probiotics tolerated harsh environments and provided superior protection to probiotic cells. Hence, microencapsulation of probiotic bacteria using rice protein-prebiotic (inulin) conjugates may be a useful and effective approach for the encapsulation of probiotic bacteria for further biofortification applications. Overall, this study presents a new source of matrices for probiotic carriers with high stability and protective effects.
The original contributions presented in the study are included in the article/supplementary material, further inquiries can be directed to the corresponding author.
PM: Methodology, Validation, Formal analysis, Investigation, Writing – original draft. FA: Formal analysis, Investigation, Methodology, Writing – original draft. HK: Data curation, Software, Validation, Writing – review & editing. MJ: Software, Writing – review & editing. AH: Writing – review & editing, Data curation, Formal Analysis. FH: Data curation, Formal analysis, Investigation, Writing – review & editing. SM: Conceptualization, Funding acquisition, Methodology, Project administration, Supervision, Validation, Writing – review & editing.
The author(s) declare financial support was received for the research, authorship, and/or publication of this article. The funding has been received from United Arab Emirates University through Summer Undergraduate Research Experience-Plus (SURE-Plus) project with grant code G00004484.
Authors are thankful to United Arab Emirates University for funding this research through Summer Undergraduate Research Experience-Plus (SURE-Plus) project with grant code G00004484. awarded to PI-Sajid Maqsood.
The authors declare that the research was conducted in the absence of any commercial or financial relationships that could be construed as a potential conflict of interest.
The author(s) declared that they were an editorial board member of Frontiers, at the time of submission. This had no impact on the peer review process and the final decision.
All claims expressed in this article are solely those of the authors and do not necessarily represent those of their affiliated organizations, or those of the publisher, the editors and the reviewers. Any product that may be evaluated in this article, or claim that may be made by its manufacturer, is not guaranteed or endorsed by the publisher.
Afzaal, M., Saeed, F., Aamir, M., Usman, I., Ashfaq, I., Ikram, A., et al. (2021). Encapsulating properties of legume proteins: recent updates & perspectives. Int. J. Food Prop. 24, 1603–1614. doi: 10.1080/10942912.2021.1987456
Ahmad, M., Mudgil, P., and Maqsood, S. (2019). Camel whey protein microparticles for safe and efficient delivery of novel camel milk derived probiotics. LWT 108, 81–88. doi: 10.1016/j.lwt.2019.03.008
Algaithi, M., Mudgil, P., Hamdi, M., Redha, A. A., Ramachandran, T., Hamed, F., et al. (2022). Lactobacillus reuteri-fortified camel milk infant formula: effects of encapsulation, in vitro digestion, and storage conditions on probiotic cell viability and physicochemical characteristics of infant formula. J. Dairy Sci. 105, 8621–8637. doi: 10.3168/jds.2022-22008
Allahdad, Z., Manus, J., Aguilar-Uscanga, B. R., Salmieri, S., Millette, M., and Lacroix, M. (2022). Physico-chemical properties and sensorial appreciation of a new fermented probiotic beverage enriched with pea and Rice proteins. Plant Foods Hum. Nutr. 77, 112–120. doi: 10.1007/s11130-021-00944-1
Arslan, S., Erbas, M., Tontul, I., and Topuz, A. (2015). Microencapsulation of probiotic Saccharomyces cerevisiae var. boulardii with different wall materials by spray drying. LWT Food Sci. Technol. 63, 685–690. doi: 10.1016/j.lwt.2015.03.034
Ashwar, B. A., Gani, A., Gani, A., Shah, A., and Masoodi, F. A. (2018). Production of RS4 from rice starch and its utilization as an encapsulating agent for targeted delivery of probiotics. Food Chem. 239, 287–294. doi: 10.1016/j.foodchem.2017.06.110
Burger, T. G., Singh, I., Mayfield, C., Baumert, J. L., and Zhang, Y. (2022). The impact of spray drying conditions on the physicochemical and emulsification properties of pea protein isolate. LWT Food Sci. Technol. 153:112495. doi: 10.1016/j.lwt.2021.112495
Chen, X., Zhang, K., Wang, Y., Wu, X., and Wan, H. (2023). Green tea polysaccharide conjugates and gelatin enhanced viability of L. acidophilus by layer-by-layer encapsulation. Food Biosci. 52:102471. doi: 10.1016/j.fbio.2023.102471
de Araújo Etchepare, M., Nunes, G. L., Nicoloso, B. R., Barin, J. S., Moraes Flores, E. M., de Oliveira Mello, R., et al. (2020). Improvement of the viability of encapsulated probiotics using whey proteins. LWT Food Sci. Technol. 117:108601. doi: 10.1016/j.lwt.2019.108601
Devarajan, A., Mudgil, P., Aldhaheri, F., Hamed, F., Dhital, S., and Maqsood, S. (2022). Camel milk-derived probiotic strains encapsulated in camel casein and gelatin complex microcapsules: stability against thermal challenge and simulated gastrointestinal digestion conditions. J. Dairy Sci. 105, 1862–1877. doi: 10.3168/jds.2021-20745
Feng, K., Huang, R.-M., Wu, R.-Q., Wei, Y.-S., Zong, M.-H., Linhardt, R. J., et al. (2020). A novel route for double-layered encapsulation of probiotics with improved viability under adverse conditions. Food Chem. 310:125977. doi: 10.1016/j.foodchem.2019.125977
Fernanda, B. H., Roudayna, D., and Andreea, P. (2016). Encapsulation of probiotics: Insights into academic and industrial approaches AIMS Materials Science. 3, 114–136. doi: 10.3934/matersci.2016.1.114
Fu, G.-M., Xu, Z.-W., Luo, C., Xu, L.-Y., Chen, Y.-R., Guo, S.-L., et al. (2021). Modification of soy protein isolate by Maillard reaction and its application in microencapsulation of Limosilactobacillus reuteri. J. Biosci. Bioeng. 132, 343–350. doi: 10.1016/j.jbiosc.2021.06.007
Gharibzahedi, S. M. T., and Smith, B. (2021). Legume proteins are smart carriers to encapsulate hydrophilic and hydrophobic bioactive compounds and probiotic bacteria: a review. Compr. Rev. Food Sci. Food Saf. 20, 1250–1279. doi: 10.1111/1541-4337.12699
González-Ferrero, C., Irache, J., and González-Navarro, C. (2018). Soybean protein-based microparticles for oral delivery of probiotics with improved stability during storage and gut resistance. Food Chem. 239, 879–888. doi: 10.1016/j.foodchem.2017.07.022
Gunzburg, W. H., Aung, M. M., Toa, P., Ng, S., Read, E., Tan, W. J., et al. (2020). Efficient protection of microorganisms for delivery to the intestinal tract by cellulose sulphate encapsulation. Microb. Cell Factories 19:216. doi: 10.1186/s12934-020-01465-3
Guo, Q., Tang, J., Li, S., Qiang, L., Chang, S., Du, G., et al. (2022). Lactobacillus plantarum 21805 encapsulated by whey protein isolate and dextran conjugate for enhanced viability. Int. J. Biol. Macromol. 216, 124–131. doi: 10.1016/j.ijbiomac.2022.06.207
Hadidi, M., Boostani, S., and Jafari, S. M. (2022). Pea proteins as emerging biopolymers for the emulsification and encapsulation of food bioactives. Food Hydrocoll. 126:107474. doi: 10.1016/j.foodhyd.2021.107474
Hadidi, M., Tan, C., Assadpour, E., Kharazmi, M. S., and Jafari, S. M. (2023). Emerging plant proteins as nanocarriers of bioactive compounds. J. Control. Release 355, 327–342. doi: 10.1016/j.jconrel.2023.01.069
Hill, C., Guarner, F., Reid, G., Gibson, G. R., Merenstein, D. J., Pot, B., et al. (2014). The international scientific Association for Probiotics and Prebiotics consensus statement on the scope and appropriate use of the term probiotic. Nat. Rev. Gastroenterol. Hepatol. 11, 506–514. doi: 10.1038/nrgastro.2014.66
Islam, F., Amer Ali, Y., Imran, A., Afzaal, M., Zahra, S. M., Fatima, M., et al. (2023). Vegetable proteins as encapsulating agents: recent updates and future perspectives. Food Sci. Nutr. 11, 1705–1717. doi: 10.1002/fsn3.3234
Kailasapathy, K. (2002). Microencapsulation of probiotic bacteria: technology and potential applications. Curr. Issues Intest. Microbiol. 3, 39–48. Available at: https://www.ncbi.nlm.nih.gov/pubmed/12400637.
Kaur, J., Singh, B. P., Chaudhary, V., Elshaghabee, F. M. F., Singh, J., Singh, A., et al. (2021). “Probiotics as live bio-therapeutics: prospects and perspectives” in Advances in probiotics for sustainable food and medicine. eds. G. Goel and A. Kumar (Singapore: Springer Singapore), 83–120.
Kiran, F., Afzaal, M., Shahid, H., Saeed, F., Ahmad, A., Ateeq, H., et al. (2023). Effect of protein-based nanoencapsulation on viability of probiotic bacteria under hostile conditions. Int. J. Food Prop. 26, 1698–1710. doi: 10.1080/10942912.2023.2228514
Lam, A., Can Karaca, A., Tyler, R., and Nickerson, M. (2018). Pea protein isolates: structure, extraction, and functionality. Food Rev. Intl. 34, 126–147. doi: 10.1080/87559129.2016.1242135
Li, Y., and Huang, Q. (2015). “Protein-polysaccharide complexes for effective delivery of bioactive functional food ingredients” in Nano-and micro-scale vehicles for effective delivery of bioactive ingredients in functional foods, John Wiley & Sons, Ltd. eds. C. M. Sabliov, H. Chen, and R. Y. Yada 224–246.
Li, W., Zhao, Y., Zhao, Y., Li, S., Yun, L., Zhi, Z., et al. (2023). Improving the viability of Lactobacillus plantarum LP90 by carboxymethylated dextran-whey protein conjugates: the relationship with glass transition temperature. Food Hydrocoll. 134:108102. doi: 10.1016/j.foodhyd.2022.108102
Liu, L., Li, X., Zhu, Y., Bora, A. F. M., Zhao, Y., Du, L., et al. (2016). Effect of microencapsulation with Maillard reaction products of whey proteins and isomaltooligosaccharide on the survival of Lactobacillus rhamnosus. LWT Food Sci. Technol. 73, 37–43. doi: 10.1016/j.lwt.2016.05.030
Loyeau, P. A., Spotti, M. J., Braber, N. V., Rossi, Y. E., Montenegro, M. A., Vinderola, G., et al. (2018). Microencapsulation of Bifidobacterium animalis subsp. lactis INL1 using whey proteins and dextrans conjugates as wall materials. Food Hydrocoll. 85, 129–135. doi: 10.1016/j.foodhyd.2018.06.051
Mao, L., Pan, Q., Hou, Z., Yuan, F., and Gao, Y. (2018). Development of soy protein isolate-carrageenan conjugates through Maillard reaction for the microencapsulation of Bifidobacterium longum. Food Hydrocoll. 84, 489–497. doi: 10.1016/j.foodhyd.2018.06.037
Markowiak, P., and Śliżewska, K. (2017). Effects of probiotics, prebiotics, and synbiotics on human health. Nutrients 9:1021. doi: 10.3390/nu9091021
Marson, G. V., Saturno, R. P., Comunian, T. A., Consoli, L., da Costa Machado, M. T., and Hubinger, M. D. (2020). Maillard conjugates from spent brewer’s yeast by-product as an innovative encapsulating material. Food Res. Int. 136:109365. doi: 10.1016/j.foodres.2020.109365
Mudgil, P., Aldhaheri, F., Hamdi, M., Punia, S., and Maqsood, S. (2022). Fortification of Chami (traditional soft cheese) with probiotic-loaded protein and starch microparticles: characterization, bioactive properties, and storage stability. LWT Food Sci. Technol. 158:113036. doi: 10.1016/j.lwt.2021.113036
Nooshkam, M., and Varidi, M. (2020). Maillard conjugate-based delivery systems for the encapsulation, protection, and controlled release of nutraceuticals and food bioactive ingredients: a review. Food Hydrocoll. 100:105389. doi: 10.1016/j.foodhyd.2019.105389
Olech, M., Cybulska, J., Nowacka-Jechalke, N., Szpakowska, N., Masłyk, M., Kubiński, K., et al. (2023). Novel polysaccharide and polysaccharide-peptide conjugate from Rosa rugosa Thunb. Pseudofruit – structural characterisation and nutraceutical potential. Food Chem. 409:135264. doi: 10.1016/j.foodchem.2022.135264
Pietrysiak, E., Smith, D. M., Smith, B. M., and Ganjyal, G. M. (2018). Enhanced functionality of pea-rice protein isolate blends through direct steam injection processing. Food Chem. 243, 338–344. doi: 10.1016/j.foodchem.2017.09.132
Praepanitchai, O.-A., Noomhorm, A., and Anal, A. K. (2019). Survival and behavior of encapsulated probiotics (Lactobacillus plantarum) in calcium-alginate-soy protein isolate-based hydrogel beads in different processing conditions (pH and temperature) and in pasteurized mango juice. Biomed Res. Int. 2019:9768152. doi: 10.1155/2019/9768152
Rajam, R., and Subramanian, P. (2022). Encapsulation of probiotics: past, present and future. Beni-Suef Univ. J. Basic Appl. Sci. 11, 1–18. doi: 10.1186/s43088-022-00228-w
Sarao, L. K., and Arora, M. (2017). Probiotics, prebiotics, and microencapsulation: a review. Crit. Rev. Food Sci. Nutr. 57, 344–371. doi: 10.1080/10408398.2014.887055
Sun, X., Wang, H., Li, S., Song, C., Zhang, S., Ren, J., et al. (2022). Maillard-type protein-polysaccharide conjugates and electrostatic protein-polysaccharide complexes as delivery vehicles for food bioactive ingredients: formation, types, and applications. Gels 8:135. doi: 10.3390/gels8020135
Vaniski, R., da Silva, S. C., da Silva-Buzanello, R. A., Canan, C., and Drunkler, D. A. (2021). Improvement of Lactobacillus acidophilus La-5 microencapsulation viability by spray-drying with rice bran protein and maltodextrin. Journal of Food Process. Preserv. 45:e15364. doi: 10.1111/jfpp.15364
Varankovich, N., Martinez, M. F., Nickerson, M. T., and Korber, D. R. (2017). Survival of probiotics in pea protein-alginate microcapsules with or without chitosan coating during storage and in a simulated gastrointestinal environment. Food Sci. Biotechnol. 26, 189–194. doi: 10.1007/s10068-017-0025-2
Xu, C., Ban, Q., Wang, W., Hou, J., and Jiang, Z. (2022). Novel nano-encapsulated probiotic agents: encapsulate materials, delivery, and encapsulation systems. J. Control. Release 349, 184–205. doi: 10.1016/j.jconrel.2022.06.061
Yeung, Y. K., Kang, Y.-R., and Chang, Y. H. (2023). Synbiotic encapsulation with Maillard reaction product and okra Pectic oligosaccharide to enhance the survival of Lactiplantibacillus Plantarum. Food Bioprocess Technol. 17, 409–423. doi: 10.1007/s11947-023-03141-x
Zhong, S.-R., Li, M.-F., Zhang, Z.-H., Zong, M.-H., Wu, X.-L., and Lou, W.-Y. (2021). Novel antioxidative wall materials for lactobacillus casei microencapsulation via the maillard reaction between the soy protein isolate and prebiotic oligosaccharides. J. Agric. Food Chem. 69, 13744–13753. doi: 10.1021/acs.jafc.1c02907
Keywords: probiotics, protein-prebiotic conjugate, viability, encapsulation, simulated gastrointestinal digestion
Citation: Mudgil P, Alkaabi F, Khan H, Javed M, Hajamohideen AR, Hamed F and Maqsood S (2024) Enhanced viability and stability of the Lactobacillus reuteri DSM 17938 probiotic strain following microencapsulation in pea and rice protein-inulin conjugates. Front. Sustain. Food Syst. 8:1284069. doi: 10.3389/fsufs.2024.1284069
Received: 27 August 2023; Accepted: 14 February 2024;
Published: 01 March 2024.
Edited by:
Carla Cavallo, University of Naples Federico II, ItalyReviewed by:
Kathleen L. Hefferon, Cornell University, United StatesCopyright © 2024 Mudgil, Alkaabi, Khan, Javed, Hajamohideen, Hamed and Maqsood. This is an open-access article distributed under the terms of the Creative Commons Attribution License (CC BY). The use, distribution or reproduction in other forums is permitted, provided the original author(s) and the copyright owner(s) are credited and that the original publication in this journal is cited, in accordance with accepted academic practice. No use, distribution or reproduction is permitted which does not comply with these terms.
*Correspondence: Sajid Maqsood, c2FqaWQubUB1YWV1LmFjLmFl
†These authors have contributed equally to this work
Disclaimer: All claims expressed in this article are solely those of the authors and do not necessarily represent those of their affiliated organizations, or those of the publisher, the editors and the reviewers. Any product that may be evaluated in this article or claim that may be made by its manufacturer is not guaranteed or endorsed by the publisher.
Research integrity at Frontiers
Learn more about the work of our research integrity team to safeguard the quality of each article we publish.