- 1Dr. Kalam Agricultural College, Arrabari, Bihar, India
- 2ICAR-Central Research Institute for Dryland Agriculture, Hyderabad, Telangana, India
- 3Division of Agronomy, ICAR-Indian Agricultural Research Institute, New Delhi, India
- 4Visva Bharati University, Shantiniketan, West Bengal, India
Accelerated energy use, negative environmental outcomes, and poor economic returns questioned the sustainability of contemporary agricultural production systems globally. The task is much more daunting in the northwestern part of India where the over exploitation of natural resources is a major concern for sustainable agricultural planning. An integrated farming system (IFS) encompasses various enterprises such as crops, dairy, poultry, and fisheries can offer a myriad of benefits in terms of enhanced farm productivity, profitability, and environmental sustainability. Hence, the study hypothesized that the complementary interaction between the different enterprises would improve food production and reduce negative environmental outcomes. Therefore, production potential and environmental sustainability in terms of energy efficiency, greenhouse gas emissions, and eco-efficiency of nine IFS models, namely, crop enterprise (M2); crop + dairy (M3); crop + dairy + fishery (M4); crop + dairy + fishery + poultry (M5); crop + dairy + fishery + poultry + duckery (M6); crop + dairy + fishery + poultry + duckery + apiary (M7); crop + dairy + fishery + poultry + duckery + apiary + boundary plantation (M8); crop + dairy + fishery + poultry + duckery + apiary + boundary plantation + biogas unit (M9); crop + dairy + fishery + poultry + duckery + apiary + boundary plantation + biogas unit + vermicompost (M10), were compared with the rice–wheat system (M1; the existing system). All the IFS models were tested between 2018 and 2021. The results revealed that the highest food production (61.5 Mg ha−1) was recorded under M10 followed by M9 (59.9 Mg ha−1). Concerning environmental sustainability, the combination of crop + dairy + fishery + poultry + duckery + apiary + boundary plantation + biogas unit + vermicompost (M10) recorded considerably higher energy output (517.6 × 103 MJ ha−1), net energy gain (488.5 × 103 MJ ha−1), energy ratio (17.8), and energy profitability (16.8 MJ MJ−1) followed by M9. Furthermore, the M10 had the lowest greenhouse gas (GHG) intensity (0.164 kg CO2 eq per kg food production). However, M9 had the highest eco-efficiency index (44.1 INR per kg GHG emission) followed by M10. Hence, an appropriate combination of diversified and complementary enterprises in a form of IFS model is a productive and environmentally robust approach for sustainable food production in the northwestern part of India.
1. Introduction
Environmental crises, resource dwindling, and poor economic returns have jeopardized the sustainability of current agricultural production systems (Babu et al., 2020; Yadav et al., 2021). Climate change due to increased greenhouse gas concentration has emerged as a serious global environmental issue, as it may threaten global and regional food and nutritional security (Yadav et al., 2017). Currently, global food system sector accounts for ~21–37% of total annual global greenhouse gas (GHG) emissions (Mbow et al., 2019). Therefore, there is an urgent need to reduce this emission by adopting environmentally robust agricultural production technologies. The linear economy-based agricultural production model has increased food production considerably but at the same time causes negative environmental outcomes (Babu et al., 2023).
Contemporary linear economy-based agricultural approaches such as the rice–wheat system maximize farm profitability but, at the same time, it has resulted in increased natural resource demands and pollution loads. In addition, contemporary agricultural practices have generated a huge amount of heterogeneous bio-waste (Babu et al., 2020, 2022). Open dumping of heterogeneous waste, in addition to environmental degradation, causes potential hazards to human health and livelihood security. Hence, to sustain food and nutritional security without compromising environmental quality, there is an urgent need to make agriculture more resilient to environmental degradation and climate change. Environmentally robust agricultural production technologies such as integrated farming systems (IFS), bio-intensive cropping, conservation agriculture, and organic farming offer efficient resource recycling and promote a circular economy that can potentially improve food and nutritional security without compromising environmental quality. The circular economy-based agricultural production model such as IFS relies on “take-make-waste” principle (Rathore et al., 2022; Babu et al., 2023). The IFS model focuses on minimizing external input and maximizing resource recycling, which reduces negative environmental outcomes (Babu et al., 2019). Moreover, the circular economy-led agricultural production model offers a multitude of societal, environmental, and financial benefits (Babu et al., 2023).
The best way to lower the environmental hazard of energy use is to increase energy use efficiency (Esengun et al., 2007). Hence, to maximize the efficiency of modern agricultural technology of an individual farm, the existing farming system must be characterized to capture the overall farm biodiversity (Yadav et al., 2013). It has been concluded in many studies that the yield and economic parameters increased linearly as the level of fertility increased, while the reverse trend was observed with energy use efficiency and energy productivity (Tuti et al., 2012). Furthermore, increase energy use amplified the GHG emission, as energy use and GHG emission have a positive correlation (Yadav et al., 2017). An input–output energy analysis provides farm planners and policymakers an opportunity to evaluate the economic intersection of energy use (Ozkan et al., 2004).
The IFS is a complex interrelated matrix of soil, plants, animals, implements, power, labor, capital, and other inputs controlled by farming families and influenced to varying degrees by political, economic, institutional, and other factors that operate at the farm level. It represents the integration of farm enterprises such as crops, animal husbandry, fisheries, forestry, sericulture, and poultry for optimum resource utilization (Paramesh et al., 2022). Diversified agricultural systems including livestock and crops are an ideal approach to build resilience in agricultural production systems (Sahoo et al., 2019; Babu et al., 2023). Developing climate–resilient agriculture through an integrated approach is also an ideal solution to ensure the food security of the ever-increasing global population at a time when there are twin problems of land degradation and climate change (Bhatt Sheeraz, 2016). Through diversified enterprises, IFS provides a stable and sustainable production system, and this helps in risk minimization and resilience to climate change (Behera and France, 2016). Integrating crops with livestock increases ecosystem services, minimizes environmental impact, and sustains farm profitability (Sulc and Franzluebbers, 2014). Integration of livestock with areca nut improves the ecosystem services and reduces ecological imbalance arising due to climate change scenarios in coastal agro-ecosystem (Sujatha and Bhat, 2015).
Assessment of farm productivity through energy analysis is essential to make efficient use of the naturally available resources (Soni et al., 2013). In recent times, due to the advancement of agricultural practices especially in mixed farming systems, energy consumption has increased in the form of animal feed, concentrates, minerals, fossil fuels, fertilizers, chemicals, electric power, and modern machinery causing environmental degradation (Kumar et al., 2019). Co-culturing of rice, turtle, and fish was found to be an energy and economically efficient system compared with rice monoculture (Liu et al., 2019). An integrated production system encompasses diverse enterprises and is a complex entity that needs precise estimation of energy input–output relationships and economic and environmental sustainability. Therefore, it was hypothesized that concurrent cultivation of diversified cropping along with other enterprises such as dairy, poultry, fisheries, and apiary can potentially enhance food production without compromising the environmental quality, which can mitigate the problem of food, nutritional, economic, and environmental insecurities. Hence, comparative assessments of 10 production models were undertaken in field conditions during 2018–2021 in the northwestern region of India with the following objectives: (i) to find out the effect of different enterprise's integration on food production, (ii) to quantify the effect of diverse enterprise's integration on energy dynamics and greenhouse gas emissions, and (iii) to assess the eco-efficiency index of designed IFS models over the rice–wheat system (the existing system).
2. Materials and methods
2.1. Experimental site
The field trials were executed during 2018–2021 at the ICAR-Indian Agricultural Research Institute, New Delhi, situated at 28°38′N latitude, 77°10′E longitude, and at an altitude of about 228.6 m above mean sea level. The experimental location is characterized by a sub-tropical, semi-arid climate with prominent hot dry summer and cold winter and falls under trans-Gangetic plains. New Delhi experiences hot summers (April–July) and very cold winters (December–January). The temperature ranges between 25–45°C during summer and 22–5°C during the winter season. The soil (Inceptisol, Mehruli series) was sandy clay loam in texture, and the baseline analysis of soil samples from 0 to 15 cm depth indicated 0.38% of soil organic carbon, 251.8 kg ha−1 of available nitrogen (N), 11.2 kg ha−1 of available phosphorus (P), 254 kg ha−1 of available potassium (K), and a pH of 7.6 (1:2.5 soil:water ratio).
2.2. Experimental design and management
The study objective was to assess the food production capacity, and environmental competency in terms of energy input–output relationship, greenhouse gas emissions, and eco-efficiency of designed IFS models over the conventional system. The nine IFS models, namely, M2—crop enterprise; M3—crop + dairy; M4—crop + dairy + fishery; M5—crop + dairy + fishery + poultry; M6—crop + dairy + fishery + poultry + duckery; M7—crop + dairy + fishery + poultry + duckery + apiary; M8—crop + dairy + fishery + poultry + duckery + apiary + boundary plantation; M9—crop + dairy + fishery + poultry + duckery + apiary + boundary plantation + biogas unit; and M10—crop + dairy + fishery + poultry + duckery + apiary + boundary plantation + biogas unit + vermicompost, were designed and tested against the M1 (the existing system) in field conditions between 2018 and 2021.
The M2 model comprising of crop enterprise consisted of different cropping systems, such as baby corn–berseem–baby corn, maize–mustard–sunflower, maize–vegetable pea–okra, multi-cut sorghum–potato–onion, maize–wheat–cowpea, rice–wheat–cowpea, bottle gourd–marigold–multi-cut sorghum, red gram–wheat–baby corn, and brinjal–ratoon–cowpea (dual purpose). The crops were sown with recommended seed rates and fertilizers. The details are given in Supplementary Table 1. Farmyard manure (FYM) was applied to all the Kharif season crops which were available at the farm itself. The pond water was applied as irrigation to the crops to supplement rainfall. The grain yield and straw/stover yield of all the crops in the cropping systems were recorded after the harvest of each crop. The crop residues apart from those used as cattle feed were recycled in the system.
The dairy enterprise consisted of three cross-bred cows, and the cattle shed was attached to the farmhouse. The feed and fodder requirement of the cattle was met through the fodder crops included under different cropping systems. The stalks of baby corn and maize and straw of rice and wheat were given as dry fodder, whereas the vines of vegetable pea and biomass of green cowpea were fed as green fodder to the animals. Cattle feed concentrate was given as per the recommended dose. Manure output from the dairy unit was measured through regular weighing, and each cow produced ~25 ± 3 kg of fresh cow dung per day. The cow dung and cowshed waste were recycled in the system through composting. A fishpond of 0.1 ha (50 × 20 × 2 m diameter) was constructed in the model. A total of 50 poultry birds (CARI-Devendra) (41 female and 9 male) and 32 ducks (Khaki Campbell) (female 22 and male 10) were reared above the fishpond in a low-cost house. Broken maize grains and wheat bran were fed to the birds. The number of eggs laid per annum and birds' weight were recorded. Fingerlings of freshwater fish, Rohu (Labeo rohita) as a column feeder (30%), Catla (Catla catla) as a surface feeder (30%), and Mrigal (Cirrhinus mrigala) as a bottom feeder (40%), were stocked in the ponds. The poultry and duck droppings acted as raw materials for the growth of plankton in the pond. As and when required, wheat bran and mustard cake (60:40) available at the farm were also fed to the fish, but no outside purchased feed was provided to the fish. In total, 10 kg of lime was applied before each stocking of fingerlings. The Fishes were harvested three times, and their live weight was recorded. The apiary unit was composed of 10 boxes consisting of 10 colonies of Apis mellifera which were placed at field bunds. The honey extracted from each box was weighed and recorded. The boundary plantation of flat beans as a fence crop along with 10 lemon trees, 20 kinnow trees, and 18 moringa trees was implemented on the borders of the IFS model. No fertilizers were applied to the flat bean crop, whereas the recommended dose of fertilizers was applied to lemon, kinnow, and moringa trees. Irrigation to these trees was given as and when required. The economic yield from boundary plantation crops was recorded. A biogas unit (KVIC model) of 2 m3 capacity was established along with the cattle shed, and ~25 kg of dung was added every day along with 25 L of water. The biogas produced was used for cooking and lighting purposes at the farmhouse, whereas the biogas slurry obtained was dried and applied to the crops in the system. Four vermicomposting units (3 × 1 × 1 m) were also established in the IFS model. In general, ~25% of the total dung that was left after the addition to the biogas plant was used for the vermicomposting unit, whereas farmyard manure (FYM) was prepared with remaining dung and animal wastes.
2.3. Food production estimation
The food production potential of different enterprises was assessed in terms of rice equivalent yield (REY). The REY was determined by converting the economic production of different enterprises such as crops, milk, egg, meat, and fish based on their prevailing market price (INR: Indian rupees) for each product and expressed in Mg ha−1.
2.4. Assessment of environmental sustainability
Environmental impact of different crops and enterprises in particular models was assessed by calculating the energy dynamics, global warming potential (GWP), greenhouse gas intensity (GHGI), and eco-efficiency index (EEI).
2.4.1. Energy budgeting
Energy accounting is an imperative step for designing environmentally robust production systems. In the current study, operation-wise energy was calculated based on the input energy consumed in field preparation, sowing, fertilizer application, irrigation, intercultural operation weeding, plant protection, harvesting, threshing and other operations. Similarly, the total output from each system was converted into energy output in a particular system. Energy output and input were estimated by multiplying the particular input/output with the studded energy coefficient (Supplementary Table 3). The energy competency of different IFS models was judged by following energy indices.
where NEG = net energy gain,
where ER = energy ratio,
where PE = energy profitability
where EP = energy productivity.
2.4.2. Estimation of global warming potential (GWP) and greenhouse gas intensity (GHGI)
The total GHG emissions (CO2, N2O, and CH4) during the cropping period were estimated in terms of CO2 equivalent. The CO2 equivalent is also known as GWP. The CO2, N2O, and CH4 were converted into CO2 equivalent by using GWP equivalent factors of 1, 265, and 28 for CO2, N2O, and CH4, respectively, for the timeframe of 100 years (Intergovernmental Panel on Climate Change, 2013). The GHG emissions from farm operations (tillage, herbicide, and insecticide application, planting and fertilizer application, as well as harvest) and inputs such as fertilizer and seeds were calculated by multiplying the input with its corresponding emission coefficient (West and Marland, 2002; Lal, 2004; Yadav et al., 2017).
The CH4, N2O, and emissions from the rice and applied manure and biomass, respectively, were calculated by the following equation (Yadav et al., 2017):
where EF = 10 g m−2 year−1 for India, Aj = area under rice paddy, ha year−1, SF0 = 1.4 organic manures correction factor, and SFj = 0.7 scaling factor for A.
To calculate the N2O emission, the 0.01 emission factor was multiplied by the total N supplied by organic means and expressed in N2O kg N input−1 (Tubiello et al., 2015) as follows:
GWP from all crops in the cropping system except rice was estimated with the following formula:
However, GWP from the rice was calculated as follows:
The GHG emission from dairy components was estimated using the Tier 1 method (Intergovernmental Panel on Climate Change, 2006). CO2 emissions from livestock were not estimated because annual net CO2 emissions were assumed to be zero—the CO2 photosynthesized by plants is returned to the atmosphere as respired CO2. Livestock production can result in methane (CH4) emissions from enteric fermentation and both CH4 and nitrous oxide (N2O) emissions from livestock manure management systems. The GHG emissions from the poultry and duckery plantations were mainly CH4 and N2O emissions from manure management. The CH4 and N2O emission from manure management was calculated based on the Tier 1 method outlined in Intergovernmental Panel on Climate Change (2006) guidelines. The emission intensity for fish feeds (wheat bran and mustard oil cake) was obtained from the data available for India (Food Agriculture Organization, 2017) and was expressed as kg CO2 eq kg DM−1. The rates of electricity used per Mg of live weight (LW) were multiplied by the emission factor (EFs) to determine the emission intensity in fishery components. Regional EFs were used for grid electricity (BEIS (Department for Business Energy Industrial Strategy)., 2016). The N2O emissions from the water body on the fish farm arise from microbial nitrification and denitrification, the same as in terrestrial or other aquatic ecosystems (Hu et al., 2012). The amount of N2O per species group was determined by multiplying the production by the N2O emission factor per kg of production (Hu et al., 2012), i.e., 1.69 g N2O-N per kg of production, or 0.791 kg CO2 eq. per kg LW production. The functional unit taken for the calculation of carbon footprint was 1 kg LW fish and expressed as kg CO2 eq. year−1 kg−1 LW fish.
The total global warming potential from different enterprises was calculated with the following formula:
GHGI was estimated by dividing the total GWP by total food production in terms of REY and expressed as kg CO2 eq. kg food production.
2.4.3. Estimation of eco-efficiency
Estimation of the eco-efficiency index (EEI) is imperative while designing environmentally robust production systems. EEI measures the economic return ability of the designed system concerning environmental distraction. An environmentally sound production system always had higher EEI by reducing negative environmental outcomes and enhancing the net economic gain (Babu et al., 2020). In this study of the ecological impact of different IFS, models were measured in terms of economic gain concerning total GHG emission (kg CO2 eq.). EEI was calculated with the following formula:
2.5. Statistical analysis
The data collected on different parameters were subjected to appropriate statistical analysis following the procedure described by Gomez and Gomez (1984). Standard deviation (SD) and standard error (SE) were used to measure the degree of variability between the individual data values.
3. Results
3.1. Food production
In the present study, the food production capacity of different integrated farming system models was assessed in terms of rice equivalent yield (REY). Food production differed considerably among different IFS models (Figure 1).
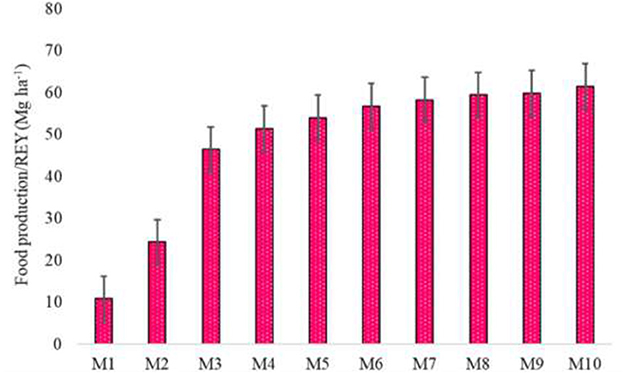
Figure 1. Food production potential of different IFS models. The error bars indicate the standard error between the treatment. M1—rice–wheat system; M2—crop enterprise; M3—crop + dairy; M4—crop + dairy + fishery; M5—crop + dairy + fishery + poultry; M6—crop + dairy + fishery + poultry + duckery; M7—crop + dairy + fishery + poultry + duckery + apiary; M8—crop + dairy + fishery + poultry + duckery + apiary + boundary plantation; M9—crop + dairy + fishery + poultry + duckery + apiary + boundary plantation + biogas unit; M10—crop + dairy + fishery + poultry + duckery + apiary + boundary plantation + biogas unit + vermicompost.
All the designed IFS models outperformed over the rice–wheat system (the existing system). Designed IFS models registered ~2–6 times higher food production over the existing production system (M1) of northwest India. Among the designed IFS models, concurrent rearing of crop + dairy + fishery + poultry + duckery + apiary + boundary plantation along with biogas unit and vermicomposting (M10) resulted in the highest food production (61.5 Mg ha−1) followed by M9 (60.0 Mg ha−1), M7 (59.5 Mg ha−1), and M6 (58.2 Mg ha−1).
3.2. Energy budgeting
Energy budgeting is crucial for designing an environmentally efficient production system. The energy input and energy output were influenced by the integration of different enterprises in integrated farming system models (Table 1). It was assumed that increasing the enterprises correspondingly increases the energy input. This was the case in the current study as well, where all the designed systems had higher energy requirements as compared with the existing farming practice (rice–wheat). In the designed system, maximum energy (29.1 × 103 MJ ha−1) was incurred under M10, while M2 had the least energy demand (25.0 × 103 MJ ha−1).
Concerning energy output, M10 registered the highest gross energy returns (517.6 × 103 MJ ha−1) followed by M9 (513.9 × 103 MJ ha−1). Similarly, the net energy gain was also considerably influenced by integration of enterprises in different IFS models, and the maximum net energy gain was obtained in M10 (488.5 × 103 MJ ha−1) followed by M9 (484.9 × 103 MJ ha−1). The energy ratio (ER) indicates net energy gain per unit of energy investment. In the current study, the IFS model comprises more enterprises that yielded more energy per unit of energy investment. All the designed systems had a higher energy ratio than the conventional farming system (rice–wheat). M10 had ~2.5 times higher energy ratio over M1 (the existing systems). The M1 was the least energy-efficient system (Figure 2). Among the tested system, M10 registered the highest energy ratio (17.8) followed by M9 (17.7).
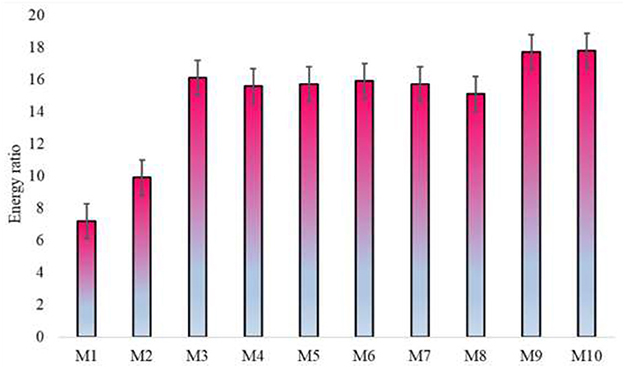
Figure 2. Effect of enterprise integration on energy ratio under different IFS models. The error bars indicate the standard error between the treatments. M1—rice–wheat system; M2—crop enterprise; M3—crop + dairy; M4—crop + dairy + fishery; M5—crop + dairy + fishery + poultry; M6—crop + dairy + fishery + poultry + duckery; M7—crop + dairy + fishery + poultry + duckery + apiary; M8—crop + dairy + fishery + poultry + duckery + apiary + boundary plantation; M9—crop + dairy + fishery + poultry + duckery + apiary + boundary plantation + biogas unit; M10—crop + dairy + fishery + poultry + duckery + apiary + boundary plantation + biogas unit + vermicompost.
Energy productivity indicates the food production per unit of energy investment. In the current study, all the designed systems recorded higher energy productivity than the conventional system. The designed system had ~3–4 times higher energy productivity over M1. Among the designed systems, M10 recorded the maximum energy productivity (3.6 kg MJ−1) and was closely followed by M9 (3.2 kg MJ−1). A similar trend was noticed with energy profitability; all the designed systems recorded higher energy profitability over the conventional system. M10 was recorded to be the highest energy profitability (16.8 MJ MJ−1) followed by M9 (16.7 MJ MJ−1) (Figure 3). M10 registered ~2.7 times more energy profitability over M1, and this indicates that complementary interaction of different enterprises is confirmed to make conventional production system energy efficient in northwest India.
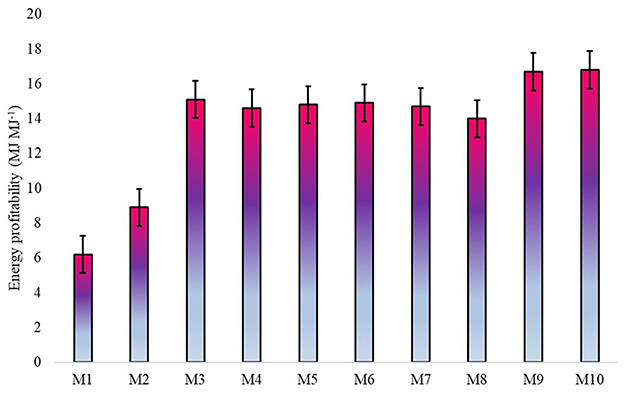
Figure 3. Effect of enterprise integration on energy profitability under different IFS models. The error bars indicate the standard error between the treatments. M1—rice–wheat system; M2—crop enterprise; M3—crop + dairy; M4—crop + dairy + fishery; M5—crop + dairy + fishery + poultry; M6—crop + dairy + fishery + poultry + duckery; M7—crop + dairy + fishery + poultry + duckery + apiary; M8—crop + dairy + fishery + poultry + duckery + apiary + boundary plantation; M9—crop + dairy + fishery + poultry + duckery + apiary + boundary plantation + biogas unit; M10—crop + dairy + fishery + poultry + duckery + apiary + boundary plantation + biogas unit + vermicompost.
3.3. Global warming potential, greenhouse gas intensity, and eco-efficiency index
The global warming potential (GWP), greenhouse gas intensity (GHGI), and eco-efficiency index (EEI) were significantly influenced by enterprise integration in different IFS models (Table 2). Integration of more enterprises increased the GWP of different IFS models. M2 had the lowest GWP (7.8 Mg CO2 eq ha−1); however, M10 had the highest GWP (10.1 Mg CO2 eq ha−1), which was almost similar to M9 and M8. On the other hand, increase in the number of enterprises considerably reduced the GHGI over M1. The M10 had the lowest GHGI (0.164 kg CO2 eq kg−1 food production) followed by M9 (0.169 kg CO2 eq kg−1 food production). The designed system had ~ 2–5 times less GHGI over M1 (the existing system). Concerning EEI, the lowest EEI (13.2 INR kg GHG−1) was reported in M1. All the designed IFS models recorded 63–70% higher EEI over M1. Among the tested IFS models, M9 registered the maximum EEI (44.1 INR kg GHG−1) closely followed by M5, M6, M7, M8, and M10.
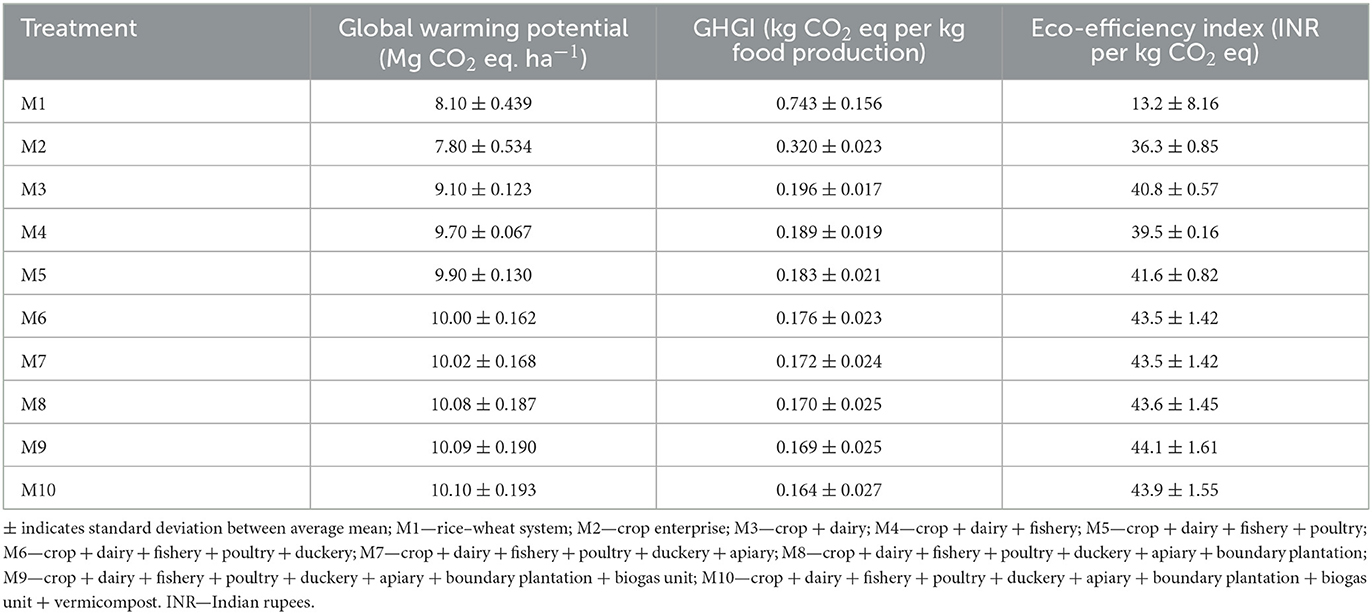
Table 2. Global warming potential, greenhouse gas intensity (GHGI), and eco-efficiency index (EEI) of different IFS models.
4. Discussion
4.1. Food production potential
The food production (rice equivalent yield) varied significantly among the IFS models, and it was maximum with the integration of more diverse enterprises, whereas lower food production was recorded in the rice–wheat system and crop enterprise alone. It is pertinent to mention here that even a simple integration of crop + dairy has the potential to enhance total food production as compared with sole cropping. The increased productivity may be ascribed to synergisms among the enterprises and the wastes or by-products from one enterprise used as inputs in another enterprise (Babu et al., 2023). Moreover, with the simultaneous application of recycled pond silt, poultry manure, nutrient-rich pond water for irrigation, and cow dung as FYM and vermicompost, crop residues provided a congenial situation to increase the enterprise's productivity. When a fishery unit was combined with a duckery, such as in models M6–M10, fish production improved as well because duck droppings served as a source of food for the fish. In the current study, total food production was the highest under M10, but it was statistically at par with M9, M7, and M6. Thus, it can be inferred that even with the integration of a few diverse and more productive enterprises such as crop + dairy + fishery + poultry + duckery (M6), the same level of food production can be attained as those achieved by integration of more enterprises such as crop + dairy + fishery + poultry + duckery + apiary + boundary plantation + biogas unit + vermicompost (M10). Gill et al. (2009) also reported statistically at par farm productivity with crops + dairy and crops + dairy + poultry production systems but significantly superior productivity over sole cropping. Enterprises such as apiary, biogas unit, and vermicompost are complementary enterprises, and their inclusion into the integrated farming systems may not achieve a significant increase in productivity though they have associated benefits such as nutrient recycling, improving the nutritional security of marginal landholders and smallholders. Farm-based crop and animal integration promote resource recycling (Soussana and Lemaire, 2014), which may improve soil fertility. Concurrent rearing of crops and livestock improves productivity and resource use efficiency (Domiciano et al., 2016; Babu et al., 2019). The complementary integration of crops with livestock and other enterprises promoted efficient resource recycling and reduces GHG emissions and follows the circular and/or green economy principles. Hence, the integration of crops with animal systems at the field level could minimize environmental pollution and increase farm production (Sartor et al., 2014; Martins et al., 2017). Integration of high-value components such as fish/poultry/duck/goat/cattle can contribute to better crop productivity (Kumar et al., 2012). Bio-intensification with complementary integration of different enterprises including crop, horticultural, livestock, fishery, poultry, and agroforestry leads to higher system productivity (Singh et al., 2007; Dhyani et al., 2016). Multiple increases in farm production under IFS over cropping alone in the lower Gangetic plains of Bihar was also reported by Kumar et al. (2012). Approximately three times higher farm productivity under field crops + fish + goat system was recorded over a crop enterprise alone in Tamil Nadu (Jayanthi et al., 2002). However, in Karnataka, India, integration of different farm enterprises resulted in ~6-fold higher system productivity over rice–rice alone (Channabasavanna and Biradar, 2007). Hence, it can be inferred that the benefits of IFS in terms of improving productivity largely depend upon the number and kind of enterprises and their management.
4.2. Environmental sustainability
The ecological sustainability of any agricultural production system mainly depends on its carbon footprint (Dubey and Lal, 2009), soil health (Babu et al., 2020), and input use efficiency (Yadav et al., 2021). In the current study, the energy input was the highest with concurrent cultivation of crop + dairy + fishery + poultry + duckery + apiary + boundary plantation + biogas unit + vermicompost (M10). The highest energy consumption in M10 is due to the maximum number of enterprises, which requires higher input energy (Babu et al., 2023). Similarly, Kumar et al. (2018) found that combining poultry and mushroom with a rice–brinjal system required higher energy input, whereas sole rice cropping required the least energy. Similarly, higher energy output was recorded under M10 due to more food production. The integration of multiple enterprises with crops resulted in higher energy output indicating the necessity of an integrated farming system for efficient utilization of the scarce and costly resource (Korikanthimath and Manjunath, 2009).
The net energy gain, energy ratio, energy productivity, and energy profitability were also higher in diversified IFS models with more enterprises, which can be attributed to the fact that these models also recorded significantly higher output energy, farm productivity, and economic returns as compared with the rice–wheat system and crop enterprise. The higher energy efficiency might be due to higher energy savings in these systems. The energy efficiency was higher in M10 and other models having diverse enterprises since there was synergism among the diverse enterprises, and the output of one enterprise served as input for another (Paramesh et al., 2019; Babu et al., 2023). At the same time, chemical fertilizers were supplemented by farm-available organic manures and nutrient-rich pond water, which further reduced the input energy that otherwise would have been required for the production and transport of the chemical fertilizers (Singh et al., 2021). Hence, it is pertinent to mention that the use of more organic nutrient sources, improved irrigation technology, and precision agriculture can enhance the energy use efficiency of the IFS model (Jackson et al., 2010; Mohammadi et al., 2014). The integration of dairy with crops significantly increased the net energy gain and energy efficiency, which was mainly due to the efficient recycling of green fodder cultivated at the IFS itself and using by-products of crops such as maize, baby corn, and cowpea as animal feed. The energy efficiency further increased with the integration of a fishery, poultry, and duckery due to more food production without the purchase of feed from the market. Broken wheat and maize grains, and rice bran were fed to the ducks and poultry, whereas the integration of ducks and poultry with the fishery supplements the feed requirement of fish by poultry and duck droppings. Furthermore, the droppings of poultry and ducks enhances the nutritional quality of pond water, which was used for irrigating the crops. In addition, a duckery ensures effective aeration in the fishpond when the duckery is integrated with the fishery under IFS, which may increase fish productivity and reduce the energy requirements. Thus, it can be emphasized that the integration of complementary enterprises in IFS increases energy efficiency. Behera et al. (2014) suggested that in an IFS, energy efficiency can be achieved by exploring renewable forms of energy such as biogas to meet the energy requirements of the farm household. Higher energy efficiencies under IFS were reported by several researchers (Rahman and Sarkar, 2012; Kumar et al., 2019; Babu et al., 2023).
Concerning GWP, the models having a greater number of enterprises registered higher GWP over the rice–wheat, crop enterprise, and crop + dairy systems primarily due to higher resource consumption in their integrated fashion. There was a marked increase in the GWP of the crop + dairy system on account of higher CH4 and N2O emissions from the dairy component. The models that were integrated with animal components (dairy, fishery, poultry, and duckery) recorded higher GWP due to increased emissions of GHG from these components. On the other hand, the greenhouse gas intensity (GHGI) of the models integrated with a greater number of complementary enterprises was lower, suggesting that the adoption of IFS with a higher number of complementary enterprises has lower GHG emissions per unit of food production. GHG emissions and energy use had a direct relationship. GHGI and energy productivity are positively correlated (Rathore et al., 2022). GHGI was lower in these models on account of higher food production than in other models. Hence, the GHGI of the monoculture system can be decreased by good agronomic measures and sustainable intensification (Gan et al., 2014). Hence, emphasis should be given to selecting those enterprises in the IFS model that require fewer inputs and have high conversion efficiency, which contributes less to GHG emissions.
The eco-efficiency index is used to express the efficiency of a system concerning its impact on nature. Eco-efficiency encompasses both the economic and ecological dimensions of a production system (Keating et al., 2010). The models having diverse enterprises recorded higher eco-efficiency index when compared with the rice–wheat system and crop enterprise signifying that the IFS is environmentally efficient with more economic returns per unit of GHG emission. The rice–wheat system had the lowest eco-efficiency index in terms of GHG emissions, which implied that this system has more negative impact on environment than designed IFS models. Sustainable agriculture aims at increasing the eco-efficiency of a system by lowering impacts such as energy use and GHG emissions while, at the same time, increasing the economic output (Cicek et al., 2011). Thus, it can be emphasized that the adoption of the IFS model with diverse complementary enterprises can lead the way toward environmentally clean production systems.
5. Conclusion
The findings prove the hypothesis that the co-culturing of different enterprises in a complementary fashion at the farm level is an environmentally robust food production system in northwest India. The integrated farming system promotes close-loop nutrient recycling, which minimizes the external input use and enhances waste recycling thereby reducing the pollution load in the ecosystem with improved food production. However, in IFS, the enterprises should be chosen in such a way that there should be a high degree of complementarity among them and that the by-product of one enterprise should act as an input for another enterprise for enhancing resource use efficiency. Through the integration of diversified cropping systems and diverse enterprises, the IFS model can be identified as a climate–resilient system with lower GHG intensity and a higher eco-efficiency index, which makes the system environmentally friendly. The IFS model encompassing crop + dairy + fishery + poultry + duckery + apiary + boundary plantation + biogas unit + vermicompost (M10) and crop + dairy + fishery + poultry + duckery + apiary + boundary plantation + biogas unit (M9) were found to be the most productive and eco-friendly production systems. M10 and M9 enhanced food production by ~6 times while reducing the GHGI by ~78%compared to M1. Hence, M10 and M9 can be promoted as environmentally robust production systems in the northwestern part of India for ensuring the food and nutritional security of small and marginal farmers. However, enterprise selection in an IFS model is very individualistic and location-specific and, hence, needs proper planning and understanding of the interactions among the different enterprises to harness their synergies. This means that the same IFS model cannot be replicated in all agroecologies. Moreover, the IFS is a multi-product production system, which needs multi-specialty and marketing.
Data availability statement
The original contributions presented in the study are included in the article/Supplementary material, further inquiries can be directed to the corresponding authors.
Author contributions
AF, VS, SB, SR, RS, and PU: conceptualization, resources, supervision, and software. AF: investigation and data recording. AF and SB: writing the original draft. BK, MH, and HP: reviewing and editing. All authors contributed to the article and approved the submitted version.
Acknowledgments
The authors are grateful to the Division of Agronomy, ICAR-Indian Agricultural Research Institute, New Delhi, for providing the necessary facilities for the conduct of the experiment. The support of VS, Director, ICAR-Central Research Institute for Dryland Agriculture, Hyderabad, is highly acknowledged for overall supervision. The authors also expresses their sincere thanks to the support and technical staff of the Agronomy Division, ICAR-IARI, New Delhi, for their help in data recording and maintaining the different components of integrated farming systems.
Conflict of interest
The authors declare that the research was conducted in the absence of any commercial or financial relationships that could be construed as a potential conflict of interest.
Publisher's note
All claims expressed in this article are solely those of the authors and do not necessarily represent those of their affiliated organizations, or those of the publisher, the editors and the reviewers. Any product that may be evaluated in this article, or claim that may be made by its manufacturer, is not guaranteed or endorsed by the publisher.
Supplementary material
The Supplementary Material for this article can be found online at: https://www.frontiersin.org/articles/10.3389/fsufs.2023.959464/full#supplementary-material
References
Babu, S., Das, A., Mohapatra, K. P., Yadav, G. S., Singh, R., Tahashildar, M., et al. (2019). Pond dyke utilization: an innovative means for enhancing productivity and income under integrated farming system in North East Hill Region of India. Indian J. Agric. Sci. 89, 117–122. doi: 10.56093/ijas.v89i1.86190
Babu, S., Das, A., Singh, R., Mohapatra, K. P., Kumar, S., Rathore, S. S., et al. (2023). Designing an energy-efficient, economically feasible, and environmentally robust integrated farming system model for sustainable food production in the Indian Himalayas. Sustain. Food. Technol. 1, 126–142. doi: 10.1039/D2FB00016D
Babu, S., Mohapatra, K. P., Das, A., Yadav, G. S., Tahasildar, M., Singh, R., et al. (2020). Designing energy-efficient, economically sustainable and environmentally safe cropping system for the rainfed maize–fallow land of the Eastern Himalayas. Sci. Total Environ. 722, 137874. doi: 10.1016/j.scitotenv.2020.137874
Babu, S., Rathore, S. S., Singh, R., Kumar, S., Singh, V. K., Yadav, S. K., et al. (2022). Exploring agricultural waste biomass for energy, food and feed production and pollution mitigation: a review. Bioresour. Technol. 48, 127566. doi: 10.1016/j.biortech.2022.127566
Behera, U. K., Babu, A., Kasechele, H., and France, J. (2014). “Energy self-sufficient sustainable integrated farming systems for livelihood security under changing climate scenario,” in Proceedings of National Symposium on Agricultural Diversification for Sustainable Livelihood and Environmental Security, Held During 18–20 November 2014 at Ludhiana, Punjab, 576.
Behera, U. K., and France, J. (2016). Integrated farming systems and the livelihood security of small and marginal farmers in India and other developing countries. Adv.Agron. 138, 234–274. doi: 10.1016/bs.agron.2016.04.001
BEIS (Department for Business Energy and Industrial Strategy). (2016). Government GHG Conversion Factors for Company Reporting: Methodology Paper for Emission Factors. London: Department of Business, Energy and Industrial Strategy, 112.
Bhatt Sheeraz, S. (2016). Integrated farming can fight climate change. Down to earth. Soc. Environ. Commun. 90, 1378–1388.
Channabasavanna, A. S., and Biradar, D. P. (2007). Relative performance of different rice-fish-poultry integrated farming system models with respect to system productivity and economics. Karnataka J. Agric. Sci. 20, 706–709.
Cicek, A., Altintas, G., and Erdal, G. (2011). Energy consumption patterns and economic analysis of irrigated wheat and rain-fed wheat production: case study for Tokat Region, Turkey. Bulg. J. Agric. Sci.17, 378–388.
Dhyani, S. K., Ram, A., and Dev, I. (2016). Potential of agroforestry systems in carbon sequestration in India. Indian J. Agric. Sci. 86, 1103–1112.
Domiciano, L., Mombach, M., Carvalho, P., Da Silva, N., Pereira, D., Cabral, L., et al. (2016). Performance and behaviour of Nellore steers on integrated systems. Anim. Prod. Sci. 58, 920–929. doi: 10.1071/AN16351
Dubey, A., and Lal, R. (2009). Carbon footprint and sustainability of agricultural production systems in Punjab, India, and Ohio, USA. J. Crop Improv. 23, 332–350. doi: 10.1080/15427520902969906
Esengun, K., Erdal, G., Gündüz, O., and Erdal, H. (2007). An economic analysis and energy use in stake-tomato production in Tokat province of Turkey. Renew. Energy 32, 1873–1881. doi: 10.1016/j.renene.2006.07.005
Food and Agriculture Organization (2017). FAOSTAT Food Balance Sheets. Rome: Food and Agriculture Organization. Available online at: http://www.fao.org/faostat/en/ (accessed January 10, 2021).
Gan, Y., Liang, C., Chai, Q., Lemke, R. L., Campbell, C. A., and Zentner, R. P. (2014). Improving farming practices reduces the carbon footprint of spring wheat production. Nat. Commun. 5, 1–13. doi: 10.1038/ncomms6012
Gill, M. S., Singh, J. P., and Gangwar, K. S. (2009). Integrated farming system and agriculture sustainability. Ind J. Agron. 54, 128–139.
Gomez, K. A., and Gomez, A. A. (1984). Statistical Procedures for Agricultural Research, 2 edn. New York, NY: John Wiley and Sons, 188–207.
Hu, Z., Lee, J. W., Chandran, K., Kim, S., and Khanal, S. K. (2012). Nitrous oxide (N2O) emission from aquaculture: a review. Environ. Sci. Technol. 46, 6470–6480. doi: 10.1021/es300110x
Intergovernmental Panel on Climate Change (2006). Guidelines for National Greenhouse Inventories. Vol. 4: Agriculture, Forestry and Other Land Use. Geneva: Intergovernmental Panel on Climate Change. Available online at: http://www.ipcc-nggip.iges.or.jp/public/2006gl/vol4 (accessed January 10, 2021).
Intergovernmental Panel on Climate Change (2013). “Climate change 2013: the physical science basis,” in Contribution of Working Group I to the Fifth Assessment Report of the Intergovernmental Panel on Climate Change, eds T. F. Stocker, D. Qin, G. K. Qin, M. Tignor, S. K. Allen, J. Boschung, A. Nauels, Y. Xia, V. Bex, and P. M. Midglev (Cambridge and New York, NY: Cambridge University Press), 710–716. doi: 10.1017/CBO9781107415324
Jackson, T. M., Khan, S., and Hafeez, M. (2010). A comparative analysis of water application and energy consumption at the irrigated field level. Agric. Water Manag. 97, 1477–1485. doi: 10.1016/j.agwat.2010.04.013
Jayanthi, C., Balusamy, M., Chinnusamy, C., and Mythili, S. (2002). Integrated nutrient supply system of linked components in lowland integrated farming system. Ind J. Agron. 48, 241–246.
Keating, B. A., Carberry, P. S., Bindraban, P. S., Asseng, S., Meinke, H., and Dixon, J. (2010). Eco-efficient agriculture: concepts, challenges, and opportunities. Crop Sci. 50, 109–119. doi: 10.2135/cropsci2009.10.0594
Korikanthimath, V. S., and Manjunath, B. L. (2009). Integrated farming systems for sustainability in agricultural production. Indian J. Agron. 54, 140–148.
Kumar, R., Patra, M. K., Thirugnanavel, A., Deka, B. C., Chatterjee, D., Borah, T. R., et al. (2018). Comparative evaluation of different integrated farming system models for small and marginal farmers under the eastern Himalayas. Indian J. Agric. Sci. 88, 1722–1729. doi: 10.56093/ijas.v88i11.84913
Kumar, S., Kumar, R., and Dey, A. (2019). Energy budgeting of crop–livestock–poultry integrated farming system in irrigated ecologies of Eastern India. Indian J. Agric. Sci. 89, 1017–1022. doi: 10.56093/ijas.v89i6.90826
Kumar, S., Singh, S. S., Meena, M. K., Shivani, S., and Dey, A. (2012). Resource recycling and their management under integrated farming system for lowlands of Bihar. Indian J. Agric. Sci. 82, 504–510.
Lal, R. (2004). Carbon emission from farm operations. Environ. Int. 30, 981–990. doi: 10.1016/j.envint.2004.03.005
Liu, G., Huang, H., and Zhou, J. (2019). Energy analysis and economic assessment of a rice-turtle-fish co-culture system. Agroecol. Sustain. Food Syst. 43, 299–309. doi: 10.1080/21683565.2018.1510870
Martins, M., Sant'Anna, S., Zaman, M., Santos, R., Monteiro, R., Alves, B., et al. (2017). Strategies for the use of urease and nitrification inhibitors with urea: Impact on N2O and NH3 emissions, fertilizer-15N recovery and maize yield in a tropical soil. Agric. Ecosyst. Environ. 247, 54–62. doi: 10.1016/j.agee.2017.06.021
Mbow, C., Rosenzweig, C., Barioni, L. G., Benton, T. G., Herrero, M., and Krishnapillai, M. (2019). “Food security,” in Climate Change Land: An IPCC Special Report on Climate Change, Desertification, Land Degradation, Sustainable Land Management, Food Security, Greenhouse Gas Fluxes in Terrestrial Ecosystems, eds P. R. Shukla, J. Skea, E. Calvo Buendia, V. Masson-Delmotte, H.-O. Pörtner, D. C. Roberts (Ginevra: IPCC). Available online at: https://policycommons.net/artifacts/458644/food-security/1431487/ (accessed February 7, 2023).
Mohammadi, A., Rafiee, S., Jafari, A., Keyhani, A., Mousavi–Avval, S. H., and Nonhebel, S. (2014). Energy use efficiency and greenhouse gas emissions of farming systems innorth Iran. Renew. Sust. Energ. Rev. 30, 724–733. doi: 10.1016/j.rser.2013.11.012
Ozkan, B., Kurklu, A., and Akcaoz, H. (2004). An input–output energy analysis in greenhouse vegetable production: a case study for Antalya region of Turkey. Biomass Bioenergy 26, 89–95. doi: 10.1016/S0961-9534(03)00080-1
Paramesh, V., Kumar, P., Shamim, M., Ravisankar, N., Arunachalam, V., Nath, A. J., et al. (2022). Integrated farming systems as an adaptation strategy to climate change: case studies from diverse agro-climatic zones of India. Sustainability 14, 11629. doi: 10.3390/su141811629
Paramesh, V., Parajuli, R., Chakurkar, E., Sreekanth, G., Kumar, H. C., Gokuldas, P., et al. (2019). Sustainability, energy budgeting, and life cycle assessment of crop-dairy-fish-poultry mixed farming system for coastal lowlands under humid tropic condition of India. Energy 188, 116101. doi: 10.1016/j.energy.2019.116101
Rahman, F. H., and Sarkar, S. (2012). “Efficient resource utilization through integrated farming system approach in the farmers' field at Burdwan district of West Bengal,” in Extended Summaries Vol 3: 3rd International Agronomy Congress, held during 26–30 November 2012 at New Delhi, 997–8.
Rathore, S. S., Babu, S., Shekhawat, K., Singh, R., Yadav, S. K., Singh, V. K., et al. (2022). Designing energy cum carbon-efficient environmentally clean production system for achieving green economy in agriculture. Sustain. Energy Technol. Assess. 52, 102190. doi: 10.1016/j.seta.2022.102190
Sahoo, A. K., Pattanaik, P., Haldar, D., and Mohanty, U. C. (2019). Integrated farming system: a climate smart agriculture practice for food security and environment resilience. Int. J. Trop. Agric. 37, 193–201.
Sartor, K., Restivo, Y., Ngendakumana, P., and Dewallef, P. (2014). Prediction of SOx and NOx emissions from a medium size biomass boiler. Biomass Bioenerg. 65, 91–100. doi: 10.1016/j.biombioe.2014.04.013
Singh, J. P., Gill, M. S., and Tripathi, D. (2007). “Development of integrated farming system models for marginal and small farmers,” in Extended Summaries of 3rd National Symposium on Integrated Farming System and Its Role Towards Livelihood Improvement Held at ARS, Durgapura, Jaipur, 51–3.
Singh, R., Babu, S., Avasthe, R. K., Yadav, G. S., Das, A., Mohapatra, K. P., et al. (2021). Crop productivity, soil health, and energy dynamics of Indian Himalayan intensified organic maize-based systems. Int. Soil Water Conserv. Res. 9, 260–270. doi: 10.1016/j.iswcr.2020.11.003
Soni, P., Taewichit, C., and Salokhe, V. M. (2013). Energy consumption and CO2 emissions in rainfed agricultural production systems of Northeast Thailand. Agric. Syst. 116, 25–36. doi: 10.1016/j.agsy.2012.12.006
Soussana, J. F., and Lemaire, G. (2014). Coupling carbon and nitrogen cycles for environmentally sustainable intensification of grasslands and crop-livestock systems. Agric. Ecosyst. Environ. 190, 9–17. doi: 10.1016/j.agee.2013.10.012
Sujatha, S., and Bhat, R. (2015). Resource use and benefits of mixed farming approach in arecanut ecosystem in India. Agric. Syst. 141, 126–137. doi: 10.1016/j.agsy.2015.10.005
Sulc, R. M., and Franzluebbers, A. J. (2014). Exploring integrated crop-livestock systems indifferent eco-regions of the United States. Eur. J. Agron. 57, 21–30. doi: 10.1016/j.eja.2013.10.007
Tubiello, F. N., Condor-Golec, R. D., Salvatore, M., Piersante, A., Federici, S., Ferrara, A., et al. (2015). Estimating Greenhouse Gas Emissions in Agriculture: A Manual to Address Data Requirements for Developing Countries. Food and Agriculture Organization of the United Nations, Rome, Italy.
Tuti, M. D., Vedprakash, B. M., Pandey, R., Bhattacharyya, D., Mahanta, J. K., Bisht, M. K., et al. (2012). Energy budgeting of colocasia-based cropping systems in the Indian sub-Himalayas. Energy 45, 986–993. doi: 10.1016/j.energy.2012.06.056
West, T. O., and Marland, G. A. (2002). Synthesis of carbon sequestration, carbon emissions, and net carbon flux in agriculture: comparing tillage practices in the United States. Agric. Ecosyst. Environ. 91, 217–232. doi: 10.1016/S0167-8809(01)00233-X
Yadav, G. S., Chandan, D., Datta, M., Ngachan, S. V., Yadav, J. S., and Babu, S. (2013). Comparative evaluation of traditional and improved farming practices in Tripura. Indian J. Agric. Sci. 83, 310–314. doi: 10.1155/2013/718145
Yadav, G. S., Das, A., Kandpal, B., Babu, S., Lal, R., Datta, M., et al. (2021). The food-energy-water-carbon nexus in a maize-maize-mustard cropping sequence of the Indian Himalayas: An impact of tillage-cum-live mulching. Renew. Sustain. Energy Rev. 151, 111602. doi: 10.1016/j.rser.2021.111602
Keywords: energy productivity, eco-efficiency index, greenhouse gas intensity, productivity, sustainability
Citation: Fatima A, Singh VK, Babu S, Singh RK, Upadhyay PK, Rathore SS, Kumar B, Hasanain M and Parween H (2023) Food production potential and environmental sustainability of different integrated farming system models in northwest India. Front. Sustain. Food Syst. 7:959464. doi: 10.3389/fsufs.2023.959464
Received: 01 June 2022; Accepted: 22 February 2023;
Published: 24 March 2023.
Edited by:
Venkatesh Paramesh, Indian Council of Agricultural Research (ICAR), IndiaReviewed by:
Ebrahim Tilahun, Addis Ababa University, EthiopiaRevappa Mohan Kumar, University of Agricultural Sciences, Bangalore, India
Copyright © 2023 Fatima, Singh, Babu, Singh, Upadhyay, Rathore, Kumar, Hasanain and Parween. This is an open-access article distributed under the terms of the Creative Commons Attribution License (CC BY). The use, distribution or reproduction in other forums is permitted, provided the original author(s) and the copyright owner(s) are credited and that the original publication in this journal is cited, in accordance with accepted academic practice. No use, distribution or reproduction is permitted which does not comply with these terms.
*Correspondence: Vinod Kumar Singh, dmt1bWFyc2luZ2hfMDEmI3gwMDA0MDt5YWhvby5jb20=; Subhash Babu, c3ViaGlhcmkmI3gwMDA0MDtnbWFpbC5jb20=; Pravin Kumar Upadhyay, cHJhdmluLm5kdSYjeDAwMDQwO2dtYWlsLmNvbQ==