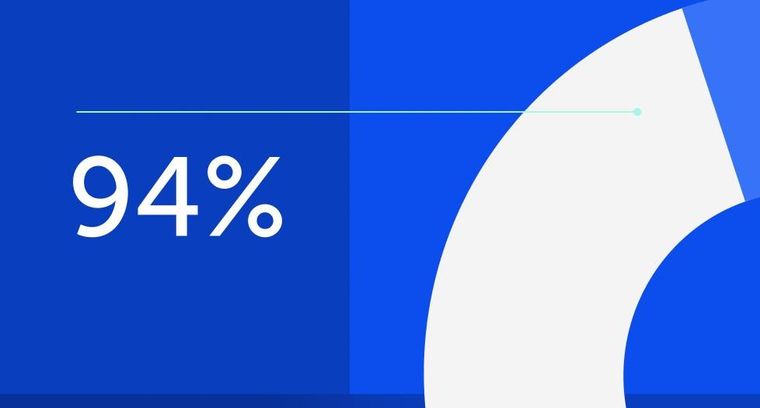
94% of researchers rate our articles as excellent or good
Learn more about the work of our research integrity team to safeguard the quality of each article we publish.
Find out more
ORIGINAL RESEARCH article
Front. Sustain. Food Syst., 26 October 2023
Sec. Nutrition and Sustainable Diets
Volume 7 - 2023 | https://doi.org/10.3389/fsufs.2023.1288136
This article is part of the Research TopicNutrition and Sustainable Development Goal 11: Sustainable Cities and CommunitiesView all 7 articles
Urban agriculture (UA) serves as an essential aspect of local food production that could promote the urban resilience enhancement of food system. This study applies a “farm-to-table” emergy method to analyze the environmental sustainability of two typical urban farms in Beijing, China, based on the field investigation. One is a suburban greenhouse farm that sells food through supermarkets, and the other is an aquaponic farm that delivers fresh food to consumers’ tables by express delivery. The results showed that compared with traditional greenhouse farming, aquaponics farm has a lower environmental loading ratio and higher emergy sustainability index, but requires more emergy inputs. The research findings would provide scientific references for the future planning of urban agriculture from the perspective of both production and sales.
Projections say that 68% of the global population will live in cities and towns in 2050 (UN-Habitat, 2022), as zero hunger is highlighted as an essential target of the sustainable development goals (SDGs; Jarzebski et al., 2021), an urgency to satisfy the increasing food demands within cities shows consequently (Li et al., 2023; Taylor et al., 2023). In particular, with the shocks from global COVID-19, the issue of the urban food supply has received broader attention (Garnett et al., 2020). Urban agriculture (UA), an emerging form of agricultural planning, is gaining popularity to buffer the latent risks brought by the post-epidemic, and achieve the sustainable development goals of zero hunger and sustainable cities and communities (Dian et al., 2019).
Urban agriculture includes agriculture in small areas within cities, such as vacant urban lots, gardens, balconies, and agriculture located in small towns, cities, and on the edge of metropolitan areas (suburbs; Food and Agriculture Organization of the United Nations, 1999; Viljoen and Bohn, 2014; Russo and Cirella, 2019). Urban agriculture can be divided into two types depending on the site. One is building-related agriculture called Zero-Acreage Farming, also called ZFarming (Thomaier et al., 2015). ZFarming specifically includes open rooftop farms, rooftop greenhouses, productive façades, and agricultural activities in existing or new urban buildings, where the form of cultivation can be either soil-or hydroponic and may involve livestock. The building use can be either mixed or single agricultural use. Another type of agriculture is carried out on vacant urban lands, such as city farms, allotment gardens, and urban farm parks (Goldstein et al., 2016; Koegler et al., 2017). In order to achieve efficient food production on limited land, most of the agriculture carried out on vacant urban land is protected agriculture, also called controlled environmental agriculture (Weidner et al., 2022).
UA is an intensive and specialized agricultural activity using urban resources (Viljoen and Bohn, 2014). The primary objective of urban agriculture development is to make full use of its function of food production and thus help improve the resilience of urban food systems. The results of urban agriculture in mitigating urban food crises and securing food supply in special times demonstrate its great potential for food production (Zezza and Tasciotti, 2010; Martellozzo et al., 2014). Scholars and research institutions have been assessing this capacity more systematically and precisely in recent years. Grewal and Grewal (2012) showed that developing urban agriculture activities on 80% of Cleveland’s vacant lots, 9% of each occupied residential area, and 62% of industrial and commercial rooftops could satisfy between 46 and 100% of Cleveland’s fresh produce needs, as well as 94% of its poultry and shell egg and 100% of its honey needs. If rooftop agriculture were developed in public residential areas throughout Singapore, it could increase domestic vegetable production by 700% and meet 35.5% of domestic demand (Astee and Kishnani, 2010). Orsini et al. (2014) found that the rooftop gardens in Bologna could provide an estimated 12,000 tons of vegetables per year, which is equal to 77% of the residents’ demands. In addition to alleviating the food risks within cities, urban agriculture also generates environmental benefits such as the mitigation of urban heat islands (Ackerman et al., 2014), biodiversity conservation, and climate change adaptation (Astee and Kishnani, 2010; Kulak et al., 2013; Meng et al., 2023a). For socio-economic development, urban agriculture also plays a unique role in generating income and providing employment opportunities (Ayenew et al., 2011; Hamilton et al., 2014), educating teenagers about environmental protection, improving the well-being of residents (Mourão et al., 2019), building harmonious communities (Reynolds and Cohen, 2016), and thus fostering inclusive cities.
Current research on the environmental sustainability of urban agriculture is mainly based on life cycle assessment (Kulak et al., 2013; Zhang et al., 2013; Sanyé-Mengual et al., 2015; Benis and Ferrão, 2017; Beltran et al., 2023) and emergy analysis (McDougall et al., 2019, 2020; Cristiano, 2021; David et al., 2022), and focuses on the impact of urban agriculture on food miles and whether it has a role in alleviating the pressure on urban resources and the environment. A study of hydroponic urban growing plants in Japan showed that per kg of lettuce and spinach generate 6.4 kg CO2 and 2.3 kg CO2, respectively, despite the high vegetable yields of this type of urban agriculture model (Shiina et al., 2010). Considering the hydroponically grown lettuce and conventionally grown lettuce systems in a greenhouse in Yuma, Arizona, United States, it was revealed that the lettuce yield was 41 ± 6.1 kg/m2/year in the former condition, and the corresponding water and energy requirements were 20 ± 3.8 L/kg/y and 90,000 ± 11,000 kJ/kg/y. While the conventional growing system produced a lettuce yield of 3.9 ± 0.21 kg/m2/year, and its water and energy requirements were 250 ± 25 L/kg/y and 1,100 ± 75 kJ/kg/y, respectively (Barbosa et al., 2015).
UA can not only make innovations in production but also in how food is sold as the consumption patterns of modern urban dwellers have changed. More and more urban farms are choosing to sell their food through “farm-to-table” means such as online ordering and express shipping, thus avoiding the potential environmental impacts of traditional food sales. However, current research on UA mainly focuses on greenhouse agriculture and the single perspective of production, but lacks a view of the integrated assessment of both the production and marketing or delivery process.
As a megacity, Beijing’s urban food supply relies heavily on outside sources. UA can improve the city’s self-sufficiency in food production. Currently, the predominant form of urban agriculture in Beijing is protected agriculture, mainly based on greenhouse agriculture. Aquaponics has been introduced in Beijing as a new type of mixed farming technology and will be a crucial focus of future UA development (Beijing Municipal Agriculture and Rural Affairs Bureau, 2020). Based on the above, this study builds a “farm-to-table” emergy method to analyze the environmental sustainability of UA, and selected two typical urban agriculture cases in Beijing, which are traditional greenhouse farms and aquaponics farms. We explored the sustainability of these two urban agriculture cases including food production and sales, aiming to provide a theoretical reference for the planning and management of urban agriculture in the future.
The Xin Xin He Yuan Agricultural Plantation in Beijing was selected as case 1. It is a typical suburban greenhouse farm located in Changping District, Beijing, the main production activities there are greenhouse cultivation of vegetables and fruits. The products include white radish, celery, cabbage, spinach, lettuce, begonia fimbristipula, Artemi mainly sia stem, kohlrabi, cucumber, tomato, and strawberry. There are wholly 30 greenhouses in the park, each covering an area of 1 ha. The planting adopts the form of crop rotation. Vegetables are mostly harvested twice a year, and fruits are harvested once a year. The farm produces nearly 1,200 tons of vegetables and fruits annually, uniformly distributed to supermarkets and purchased by consumers.
Case 2 is an aquaponics farm. This aquaponics project involves hydroponic cultivation, high-density aquaculture, microbial water treatment technology, and an automobile water circular control system. This study selected a typical aquaponics covering an area of 0.067 ha as a specific research unit. The unit’s products include sturgeon, tomatoes, and leafy vegetables (lettuce). In that system, the sturgeon are taken as core products, and the excrement of the sturgeon is subject to bacterial decomposition treatment. It is then used as a fertilizer for crops such as tomatoes and lettuce for hydroponic planting. The entire production process does not involve the use of pesticides and fertilizers. In order to enable consumers to obtain fresher food, fish, and vegetables, symbiotic products will be sent directly from the distribution center to farm members’ homes, thus avoiding resource consumption and environmental impacts in the supermarket process. Further explanations for the different stages of these two cases are shown in the supplementary information.
As shown in Figure 1, the system of case 1 specifically includes six phases: farm construction, food production, packaging, cold-chain transportation, sales in supermarkets, and consumer purchasing. Case 2 explicitly includes four phases: farm construction, food production, packaging, and express delivery. The on-farm stage includes farm construction and food production, while the off-farm stage is the subsequent process how bringing the food from farms to consumers’ tables. The input and output of various materials in each stage are systematically summarized and calculated.
The greenhouse farm and aquaponics farm produce different types of food, including vegetables, fruits, and fish. To compare the environmental benefits of different systems, this study analyzes the environmental and economic benefits of the two farms with per joule of food as the functional unit.
In this study, the emergy analysis (EA) method created by Odum was used as a quantitative method to study the efficiency of material and energy flows such as resource inputs and food outputs of the system. The EA method converts various types and properties of energy and materials flowing and stored in an eco-economic system into a uniform standard solar energy value, thus achieving a quantitative evaluation of the system’s resource use efficiency, environmental load and sustainability. Studies have shown that EA can systematically assess the ecological services of agricultural systems and thus test the sustainability of agricultural systems (Shah et al., 2019).
Odum originally defined Emergy as the amount of energy of another category contained in a flow or accumulation of energy. In other words, Emergy is the sum of other categories of energy applied directly or indirectly by a product or human activity in its formation (Odum, 1996). Any energy that flows or accumulates on Earth is derived from solar energy. Therefore, “solar emergy” is often a uniform metric in practical studies. The amount of solar energy contained in all other energy is the “solar emergy” of that energy. Its unit is solar emjoule, abbreviated as sej.
In emergy analysis studies, a conversion factor is needed to convert one type of energy to another, and this is the emergy transformity. The transformity follows the thermodynamic principle and is usually used as solar transformity in practical applications. The units are usually solar emjoules per joule, or solar emjoules per gram, abbreviated as sej/J or sej/g, which represents the amount of solar energy contained in a unit of matter or energy. A higher value of transformity means that the energy has a higher emergy value and is in a higher rank in the energy system. During the flow of energy in nature, through various interactions, low-quality energy is converted into high-quality energy with a decrease in the amount of energy, an increase in the quality of energy, and a corresponding increase in the transformity (Odum, 1996).
The Emergy Yield Ratio (EYR) characterizes the system’s ability to utilize local resources and contribute to the external economic system. The higher the EYR value, the stronger the system’s ability to utilize local resources and contribute to the external system. The EYR can be calculated according to the following formula:
Where U represents the total emergy input, and PR represents the emergy input of purchased resources.
The Environmental Loading Ratio (ELR) indicates the impact of the product’s production process on the environment or the pressure on the system. The higher the ELR value, the higher the load caused by the system on the environment. The ELR can be calculated according to the following formula:
Where N represents the non-renewable natural resources, FN represents the non-renewable purchased resources, R represents the renewable natural resources, and FR represents the renewable purchased resources.
This parameter measures the stress and stressful environmental effects due to the input and use of non-renewable resources. It is an indicator to examine the environmental stress of energy transfer and transfer processes, which can also be seen as a measure of the ecological stress of production. A lower ELR indicates that the system is less dependent on non-renewable inputs and is, therefore, usually more sustainable. In general, systems with ELR >10 are considered to be highly environmentally loaded, ELR < 2 is considered sustainable, and in between is moderately sustainable (Brown and Ulgiati, 1997).
The Emergy Sustainability Index (ESI) reflects the system’s sustainability; the higher the ESI value, the more sustainable the system is. The ESI can be calculated according to the following formula:
Emergy analysis table is the basis for calculating the system’s emergy value input and output. The emergy value analysis table mainly includes a project description, unit, updatable scale factor, emergy transformity, original energy (matter) flow, solar emergy value, and output. The emergy transformity in this study uses the benchmark GEB2016 (12.0 × 1024 sej/a), and all the previous emergy transformity with 9.44 × 1024 sej/a and 15.83 × 1024 sej/a as benchmarks are converted to the corresponding values under the GEB2016 benchmark in the study to ensure the consistency of parameter selection (Brown and Ulgiati, 2016; Liu and Yang, 2018). Refer to the following formula:
Where j represents the jth input item, Energy (Material)j represents the amount of raw energy or material input to the system. The prepared emergy analysis table is shown in the Supplementary Information. After this, the energy consumption of the production and distribution processes of the two cases is calculated, and thus the two cases are compared and analyzed.
Since profit is the main pursuit of farmers, it is necessary to consider the economic benefits when assessing the environmental impact of the cases. In this study, a cost–benefit analysis was conducted by systematically accounting for the costs and benefits of each of the two cases, and the emergy inputs required to generate a unit of profit were calculated (Figure 2).
In summary, case 1 has higher food production and profit than case 2 for the same scale. The food production and profit of the aquaponics farm are 1.51 and 1.54 times higher than that of the greenhouse farm. As shown in Figure 3, in the production and operation model of case 1, the total emergy input per joule of food is 1.28E+08 sej, of which the on-farm and off-farm stages occupy 3.13% and 96.87%, respectively. The total emergy input per joule of food in case 2 is 1.66E+07 sej, only 13.39% of that in case 1, of which the on-farm and off-farm phases occupy 44.47% and 55.53%, respectively. For both cases, the emergy inputs are almost all from purchased resources. Renewable and non-renewable purchased resources in case 1 account for 87.18% and 12.81%, respectively. Renewable purchased resources and non-renewable purchased resources account for 74.16% and 25.82%, respectively, in case 2.
As shown in Figure 4, for the on-farm stage, the total emergy input per joule of food produced in the greenhouse farm is 3.88E+06 sej, most of which come from non-renewable purchased resources, accounting for 92.74%. The total emergy input per joule of food produced in the on-farm stage of the aquaponics farm is 7.37E+06 sej, which is about 1.90 times higher than that of the greenhouse farm, of which renewable purchased resources and non-renewable purchased resources account for 54.58% and 45.37%, respectively. Comparing only the vegetable production processes of the two farms, the emergy value input per joule of vegetables produced in the aquaponics farm is 2.21 times higher than that in the greenhouse farm.
For the greenhouse farm in case 1, 91.14% of the emergy inputs come from farm construction and subsequent facility maintenance. All the resources for this process are non-renewable purchased resources. Renewable purchased resources are mainly labor and services, which account for 7.12% of the total emergy inputs. For the aquaponics farm in case 2, 24.68% of the emergy inputs come from farm construction and subsequent facility maintenance and 14.15% from purchased fish feed, which are non-renewable purchased resources. Renewable purchased resources are mainly labor and services, which account for 57.20% of the total emergy inputs.
The EYR of both farms is nearly 1.00, which indicates that both farms hardly used any local resources and relied more on economic systems to drive them, which is in line with the high input characteristics of facility farming. The ELR for greenhouse farming is 13.24, much higher than the standard value of 2, which indicates that the greenhouse farm in case 1 imposed a high environmental load on the surrounding environment. In contrast, the ELR of aquaponics farming is only 0.83, which is significantly more environmentally friendly than greenhouse farming. The ESIs of 0.08 and 1.20 for the greenhouse and aquaponics farms, respectively, indicate the better sustainability of the aquaponics farm. In contrast, the greenhouse farm is less sustainable and needs more optimization and management in the future development process.
For the off-farm stage, the emergy input per joule of food reaching the consumer in case 1 is1.26E+08 sej, while the emergy input per joule of food reaching the consumer by express delivery in case 2 was only 9.21E+06 sej, which is 7.65% of that in case 1. As presented in Figure 5, both food delivery methods are more dependent on renewable purchased resources. The supermarket sale method’s 89.77% of the total resource input comes from renewable purchased resources, and the express delivery method has 89.74%.
In case 1, 89.87% of the emergy input in the food distribution method comes from the supermarket selling stage and 9.26% from the cold chain transportation stage. The emergy inputs of labor and services in these two stages account for almost all the emergy inputs (98.86%) of the entire food-selling process. In case 2, 93.10% of the emergy input comes from the express delivery process and 6.90% from packaging. Labor and services are also the most important, accounting for 98.66% of all emergy inputs.
The EYR of both food distribution methods is about 1.00, which indicates that both methods are more dependent on external resource inputs and less able to utilize local resources. The ELR of both food distribution methods is about 0.11, indicating that both methods have a relatively low environmental impact. The ESI for the supermarket sale method is 8.87, and the ESI for the express delivery method is 8.88. This result indicates that both delivery methods are relatively sustainable and environmentally friendly.
Aquaponics farms have higher economic efficiency than greenhouse farms. The profit of the aquaponics farm was about 3,432,000 RMB/ha. The profit for greenhouse farms was about 2,233,000 RMB/ha, which is 65.08% of that for aquaponics. For aquaponics farms, the emergy input per unit of profit was 2.32E+12 sej, while for greenhouse farms, the emergy input per unit of profit was 1.59E+14 sej, which indicates that the emergy input of greenhouse farms is about 68.54 times higher than that of aquaponics farms to obtain the same profit.
According to the findings, the emergy input per joule of food produced in greenhouse agriculture in Beijing and the ESI is consistent with the results obtained from the systematic emergy analysis of protected agriculture in China. The EYR value of 1.00 for both greenhouses and aquaponics in this study is similar to that of Chinese facility agriculture (1.09; Guo et al., 2023), indicating that the facility vegetable production system relies more on the economic system to drive it, and does not efficiently exploit local resources during its operation, which is also characteristic of high input of facility vegetable production in general. The ELR of aquaponics agriculture is much lower than that of greenhouse agriculture, which demonstrates the environmental friendliness of aquaponics systems. The ESI indicator further reflects that the aquaponics system is more sustainable than the greenhouse. Notably, the emergy input per joule of food produced by aquaponics is higher than that of the greenhouse farm, which is inextricably linked to aquaponics farms requiring more water and electricity management and labor inputs. The high input of the aquaponics system also brings its high outputs. For the full advantage of the high productivity and sustainability of aquaponics systems, more prudent management of aquaponics is needed in the future, such as replacing non-renewable energy sources, reducing labor costs, and introducing automated management facilities (McDougall et al., 2019).
The results of the two schemes of food distribution show that the “farm-to-table” model could reduce the number of energy input. The farm-to-consumer online trading platform and the delivery of goods by express delivery have become increasingly popular among urban residents in recent years, which would reduce the cost of money and time for consumers, and enable consumers to obtain fresh food promptly. However, the “farm-to-table” delivery mode is probably more suitable for short-distance food delivery, and the issue of food preservation in long-distance delivery is difficult to solve by express transportation at present.
The research on the sustainability of UA will develop from a single perspective to a nexus perspective (Meng et al., 2023b). The development of UA involves the application and integration of multi-disciplinary knowledge, including agriculture, architecture, economy, public health, resources and environment, urban planning, and so on. The future development of UA should strengthen the combination of social investigation, geographic information technology, environmental remote sensing technology, and other technologies and do better planning for the development of urban agriculture through the combination of various scientific means (Zhu et al., 2023).
Currently China’s UA is still in the primary stage of development, and the development mode of urban agricultural activities is relatively single, which is still dominated by farm planting activities in the suburbs of the city, and urban residents participate in urban agricultural activities mainly through visiting suburban farms. Compared with the active practice of roof agriculture, community farms, and other urban agricultural models carried out by urban residents in Europe and the United States (Kirby et al., 2021), urban residents in China are limited by the crowded urban living space and the lack of urban agricultural land norms. They cannot personally participate in urban agricultural planting activities. In the future, it is advocated to gradually improve the corresponding laws, regulations, and standards system of urban agriculture construction and promote the integration of planning and management of urban agriculture (Orsini et al., 2020).
The UA model of the green cycle represented by the aquaponics farm shows strong sustainability. The future development of urban agriculture should vigorously promote the development model of circular agriculture and “farm-to-table” (Baganz et al., 2022). Greenhouse agriculture should reduce the use of pesticides, fertilizers, and other substances, strengthen resource utilization efficiency, and promote the development of the food system in the direction of intensification. The food delivery mode of “farm-to-table” can not only make ordinary families get new things directly through express delivery but also help to build the food transportation chain of farm-community and farm-restaurant, shorten the urban food mileage, and promote the sustainable development of the urban food system (Daftary-Steel et al., 2015).
Due to the limitation of data collection, the sample size of this study is small, and although the selected cases are highly representative, no general conclusions can be drawn about the development of urban agriculture in Beijing, and this study does not discuss the social benefits of two urban agriculture models, traditional suburban farms and hydroponic farms. This study currently focuses only on the energy value analysis of the two UA production and marketing/delivery models, and further work will be carried out in the future on the corresponding energy consumption, water consumption, carbon emissions, and other specific environmental impacts. UA development brings important implications for improving the city’s food supply capacity and reducing the flow of resources across borders. In the future, more research needs to move forward to the development potential of urban agriculture in Beijing and other megacities to find the optimal UA development model, helping reduce the environmental impacts led by food imports beyond city boundaries.
This study built a “farm-to-table” emergy method and assessed the sustainability of two typical urban agriculture modes in Beijing, China based on the field investigation, which are traditional greenhouse farming and aquaponics farming. The results show that the total emergy input of the aquaponics farm is higher than that of the greenhouse farm, but the ELR and ESI of the aquaponics farm are smaller. Therefore, compared with the greenhouse farm, the food production mode of the aquaponics farm is more sustainable. The “farm-to-table” food distribution method is more sustainable and eliminates cold-chain transportation, supermarket sales, and consumer purchase processes. The total emergy input of food delivery is only 7.65% of that of case 1. Compared with traditional open-field agriculture, protected agriculture requires more energy input and artificial maintenance. In the future, UA will form a more intensive, modernized, and mechanized production model, combined with the “farm-to-table” sales model that eliminates middlemen, which can better meet the needs of modern urban consumers and help alleviate urban food supply problems and contribute to sustainable urban development.
The original contributions presented in the study are included in the article/Supplementary material, further inquiries can be directed to the corresponding authors.
QY: Data curation, Formal analysis, Investigation, Methodology, Software, Visualization, Writing – original draft. SM: Data curation, Formal analysis, Investigation, Methodology, Software, Visualization, Writing – original draft. FM: Conceptualization, Funding acquisition, Project administration, Supervision, Validation, Writing – review & editing. JH: Data curation, Investigation, Software, Visualization, Writing – review & editing. YS: Investigation, Resources, Visualization, Writing – review & editing. HL: Conceptualization, Funding acquisition, Project administration, Supervision, Writing – review & editing. AS: Software, Visualization.
The author(s) declare financial support was received for the research, authorship, and/or publication of this article. This work was supported by the National Natural Science Foundation of China (Nos. 72174028, 71804023, and 72004035).
The authors declare that the research was conducted in the absence of any commercial or financial relationships that could be construed as a potential conflict of interest.
All claims expressed in this article are solely those of the authors and do not necessarily represent those of their affiliated organizations, or those of the publisher, the editors and the reviewers. Any product that may be evaluated in this article, or claim that may be made by its manufacturer, is not guaranteed or endorsed by the publisher.
The Supplementary material for this article can be found online at: https://www.frontiersin.org/articles/10.3389/fsufs.2023.1288136/full#supplementary-material
Ackerman, K., Conard, M., Culligan, P., Plunz, R., Sutto, M. P., and Whittinghill, L. (2014). Sustainable food systems for future cities: the potential of urban agriculture. Econ Soc Rev. 45, 189–206.
Astee, L. Y., and Kishnani, N. T. (2010). Building integrated agriculture: Utilising rooftops for sustainable food crop cultivation in Singapore. J. Green Build. 5, 105–113. doi: 10.3992/jgb.5.2.105
Ayenew, Y. A., Wurzinger, M., Tegegne, A., and Zollitsch, W. (2011). Socioeconomic characteristics of urban and peri-urban dairy production systems in the north western Ethiopian highlands. Trop. Anim. Health Prod. 43, 1145–1152. doi: 10.1007/s11250-011-9815-3
Baganz, G. F., Timpe, A., Baganz, D., Staaks, G., Hunger, B., Kloas, W., et al. (2022). City or hinterland–site potentials for upscaled aquaponics in a Berlin case study. npj Urban Sustain. 2:29. doi: 10.1038/s42949-022-00072-y
Barbosa, G. L., Gadelha, F. D., Kublik, N., Proctor, A., Reichelm, L., Weissinger, E., et al. (2015). Comparison of land, water, and energy requirements of lettuce grown using hydroponic vs. conventional agricultural methods. Int. J. Environ. Res. Public Health 12, 6879–6891. doi: 10.3390/ijerph120606879
Beijing Municipal Agriculture and Rural Affairs Bureau. (2020). Beijing agricultural and rural development plan. [Webpage]. Retrieved from http://nyncj.beijing.gov.cn/nyj/snxx/gqxx/10905539/index.html.
Beltran, A. M., Padró, R., La Rota-Aguilera, M. J., Marull, J., Eckelman, M. J., Cirera, J., et al. (2023). Displaying geographic variability of peri-urban agriculture environmental impacts in the metropolitan area of Barcelona: a regionalized life cycle assessment. Sci. Total Environ. 858:159519. doi: 10.1016/j.scitotenv.2022.159519
Benis, K., and Ferrão, P. (2017). Potential mitigation of the environmental impacts of food systems through urban and peri-urban agriculture (UPA)–a life cycle assessment approach. J. Clean. Prod. 140, 784–795. doi: 10.1016/j.jclepro.2016.05.176
Brown, M. T., and Ulgiati, S. (1997). Emergy-based indices and ratios to evaluate sustainability: monitoring economies and technology toward environmentally sound innovation. Ecol. Eng. 9, 51–69. doi: 10.1016/S0925-8574(97)00033-5
Brown, M. T., and Ulgiati, S. (2016). Emergy assessment of global renewable sources. Ecol. Model. 339, 148–156. doi: 10.1016/j.ecolmodel.2016.03.010
Cristiano, S. (2021). Organic vegetables from community-supported agriculture in Italy: Emergy assessment and potential for sustainable, just, and resilient urban-rural local food production. J. Clean. Prod. 292:126015. doi: 10.1016/j.jclepro.2021.126015
Daftary-Steel, S., Herrera, H., and Porter, C. M. (2015). The unattainable trifecta of urban agriculture. J. Agri. Food Syst. Commun Develop. 6, 19–32. doi: 10.5304/jafscd.2015.061.014
David, L. H., Pinho, S. M., Agostinho, F., Costa, J. I., Portella, M. C., Keesman, K. J., et al. (2022). Sustainability of urban aquaponics farms: an emergy point of view. J. Clean. Prod. 331:129896. doi: 10.1016/j.jclepro.2021.129896
Dian, T. A., Guinée, J. B., and Tukker, A. (2019). The second green revolution: innovative urban agriculture's contribution to food security and sustainability–a review. Global Food Sec. 22, 13–24. doi: 10.1016/j.gfs.2019.08.002
Food and Agriculture Organization of the United Nations. (1999). Two-thirds of city and peri-urban households involved in farming. Retrieved from https://sswm.info/sites/default/files/reference_attachments/FAO%201999%20Two%20thirds%20of%20city%20and%20peri%20urban%20households%20involved%20in%20farming.pdf
Garnett, P., Doherty, B., and Heron, T. (2020). Vulnerability of the United Kingdom’s food supply chains exposed by COVID-19. Nat Food. 1, 315–318. doi: 10.1038/s43016-020-0097-7
Goldstein, B., Hauschild, M., Fernández, J., and Birkved, M. (2016). Urban versus conventional agriculture, taxonomy of resource profiles: a review. Agron. Sustain. Dev. 36, 1–19. doi: 10.1007/s13593-015-0348-4
Grewal, S. S., and Grewal, P. S. (2012). Can cities become self-reliant in food? Cities 29, 1–11. doi: 10.1016/j.cities.2011.06.003
Guo, Y., Wang, H., Zhang, W., Chen, B., and Song, D. (2023). Sustainability evaluation of protected vegetables production in China based on emergy analysis. J. Clean. Prod. 388:135928. doi: 10.1016/j.jclepro.2023.135928
Hamilton, A. J., Burry, K., Mok, H. F., Barker, S. F., Grove, J. R., and Williamson, V. G. (2014). Give peas a chance? Urban agriculture in developing countries. A review. Agron Sustain Dev. 34, 45–73. doi: 10.1007/s13593-013-0155-8
Jarzebski, M. P., Elmqvist, T., Gasparatos, A., Fukushi, K., Eckersten, S., Haase, D., et al. (2021). Ageing and population shrinking: implications for sustainability in the urban century. npj Urban Sustain. 1:17. doi: 10.1038/s42949-021-00023-z
Kirby, C. K., Specht, K., Fox-Kämper, R., Hawes, J. K., Cohen, N., Caputo, S., et al. (2021). Differences in motivations and social impacts across urban agriculture types: case studies in Europe and the US. Landsc. Urban Plan. 212:104110. doi: 10.1016/j.landurbplan.2021.104110
Koegler, M., Grard, B. J. P., and Christine, A. (2017). Climate innovation potentials of urban agriculture (CIPUrA) geographic pathfinder. Climate KIC report.
Kulak, M., Graves, A., and Chatterton, J. (2013). Reducing greenhouse gas emissions with urban agriculture: a life cycle assessment perspective. Landsc. Urban Plan. 111, 68–78. doi: 10.1016/j.landurbplan.2012.11.007
Li, Y., Zhong, H., Shan, Y., Hang, Y., Wang, D., Zhou, Y., et al. (2023). Changes in global food consumption increase GHG emissions despite efficiency gains along global supply chains. Nat Food. 4, 483–495. doi: 10.1038/s43016-023-00768-z
Liu, G., and Yang, Z. (2018). Emergy analysis theory and practice: ecological economic accounting and urban green management. Beijing: Science Press.
Martellozzo, F. E., Landry, J. S., Plouffe, D., Seufert, V., Rowhani, P., and Ramankutty, N. (2014). Urban agriculture: a global analysis of the space constraint to meet urban vegetable demand. Environ. Res. Lett. 9:064025. doi: 10.1088/1748-9326/9/6/064025
McDougall, R., Kristiansen, P., and Rader, R. (2019). Small-scale urban agriculture results in high yields but requires judicious management of inputs to achieve sustainability. Proc. Natl. Acad. Sci. U. S. A. 116, 129–134. doi: 10.1073/pnas.1809707115
McDougall, R., Rader, R., and Kristiansen, P. (2020). Urban agriculture could provide 15% of food supply to Sydney, Australia, under expanded land use scenarios. Land Use Policy. 94:104554. doi: 10.1016/j.landusepol.2020.104554
Meng, F., Yuan, Q., Bellezoni, R. A., de Oliveira, J. A. P., Cristiano, S., Shah, A. M., et al. (2023a). Quantification of the food-water-energy nexus in urban green and blue infrastructure: a synthesis of the literature. Resour. Conserv. Recycl. 188:106658. doi: 10.1016/j.resconrec.2022.106658
Meng, F., Yuan, Q., Bellezoni, R. A., Puppim de Oliveira, J. A., Hu, Y., Jing, R., et al. (2023b). The food-water-energy nexus and green roofs in Sao Jose dos Campos, Brazil, and Johannesburg. South Africa. npj Urban Sustain. 3:12. doi: 10.1038/s42949-023-00091-3
Mourão, I., Moreira, M. C., Almeida, T. C., and Brito, L. M. (2019). Perceived changes in well-being and happiness with gardening in urban organic allotments in Portugal. Int. J. Sustain. Dev. World Eco. 26, 79–89. doi: 10.1080/13504509.2018.1469550
Odum, H. T. (1996). Environmental accounting: emergy and environmental decision making. New York: John Wiley and Sons.
Orsini, F., Gasperi, D., Marchetti, L., Piovene, C., Draghetti, S., Ramazzotti, S., et al. (2014). Exploring the production capacity of rooftop gardens (RTGs) in urban agriculture: the potential impact on food and nutrition security, biodiversity and other ecosystem services in the city of Bologna. Food Secur. 6, 781–792. doi: 10.1007/s12571-014-0389-6
Orsini, F., Pennisi, G., Michelon, N., Minelli, A., Bazzocchi, G., Sanyé-Mengual, E., et al. (2020). Features and functions of multifunctional urban agriculture in the global north: a review. Front in Sustain Food Syst. 4:562513. doi: 10.3389/fsufs.2020.562513
Reynolds, K., and Cohen, N. (2016). Beyond the kale: Urban agriculture and social justice activism in new York City (Vol 28). Georgia: University of Georgia Press.
Russo, A., and Cirella, G. T. (2019). Edible urbanism 5.0. Palgrave Commun. 5, 1–9. doi: 10.1057/s41599-019-0377-8
Sanyé-Mengual, E., Oliver-Solà, J., Montero, J. I., and Rieradevall, J. (2015). An environmental and economic life cycle assessment of rooftop greenhouse (RTG) implementation in Barcelona, Spain. Assessing new forms of urban agriculture from the greenhouse structure to the final product level. Int. J. Life Cycle Assess. 20, 350–366. doi: 10.1007/s11367-014-0836-9
Shah, S. M., Liu, G., Yang, Q., Wang, X., and Giannetti, B. F. (2019). Emergy-based valuation of agriculture ecosystem services and dis-services. J. Clean. Prod. 239:118019. doi: 10.1016/j.jclepro.2019.118019
Shiina, T., Hosokawa, D., Roy, P., Nakamura, N., Thammawong, M., and Orikasa, T. (2010). Life cycle inventory analysis of leafy vegetables grown in two types of plant factories. In XXVIII international horticultural congress on science and horticulture for people (IHC 2010): International symposium on 919 (pp. 115–122). Available at: https://10.17660/ActaHortic.2011.919.14.
Taylor, I., Bull, J. W., Ashton, B., Biggs, E., Clark, M., Gray, N., et al. (2023). Nature-positive goals for an organization’s food consumption. Nat Food. 4, 96–108. doi: 10.1038/s43016-022-00660-2
Thomaier, S., Specht, K., Henckel, D., Dierich, A., Siebert, R., Freisinger, U. B., et al. (2015). Farming in and on urban buildings: present practice and specific novelties of zero-acreage farming (ZFarming). Renew. Agric. Food Syst. 30, 43–54. doi: 10.1017/S1742170514000143
UN-Habitat. (2022). World cities report 2022: Envisaging the future of cities. United Nations Publications. Available at: https://unhabitat.org/wcr/#
Viljoen, A., and Bohn, K. (2014). Second nature urban agriculture: Designing productive cities. UK: Routledge.
Weidner, T., Yang, A., Forster, F., and Hamm, M. W. (2022). Regional conditions shape the food–energy–land nexus of low-carbon indoor farming. Nat Food. 3, 206–216. doi: 10.1038/s43016-022-00461-7
Zezza, A., and Tasciotti, L. (2010). Urban agriculture, poverty, and food security: empirical evidence from a sample of developing countries. Food Policy 35, 265–273. doi: 10.1016/j.foodpol.2010.04.007
Zhang, S., Bi, X. T., and Clift, R. (2013). A life cycle assessment of integrated dairy farm-greenhouse systems in British Columbia. Bioresour. Technol. 150, 496–505. doi: 10.1016/j.biortech.2013.09.076
Keywords: urban agriculture, sustainable development, emergy analysis, greenhouse agriculture, aquaponics agriculture
Citation: Yuan Q, Mi S, Meng F, Hou J, Sun Y, Li H and Shah AM (2023) An emergy analysis of environmental sustainability in urban agriculture: evidence from protected agriculture in Beijing, China. Front. Sustain. Food Syst. 7:1288136. doi: 10.3389/fsufs.2023.1288136
Received: 03 September 2023; Accepted: 09 October 2023;
Published: 26 October 2023.
Edited by:
Rui Jing, Xiamen University, ChinaReviewed by:
Jianchuan Qi, Tsinghua University, ChinaCopyright © 2023 Yuan, Mi, Meng, Hou, Sun, Li and Shah. This is an open-access article distributed under the terms of the Creative Commons Attribution License (CC BY). The use, distribution or reproduction in other forums is permitted, provided the original author(s) and the copyright owner(s) are credited and that the original publication in this journal is cited, in accordance with accepted academic practice. No use, distribution or reproduction is permitted which does not comply with these terms.
*Correspondence: Fanxin Meng, ZmFueGluLm1lbmdAYm51LmVkdS5jbg==; Hui Li, bGlodXVpQGJudS5lZHUuY24=
†These authors have contributed equally to this work
Disclaimer: All claims expressed in this article are solely those of the authors and do not necessarily represent those of their affiliated organizations, or those of the publisher, the editors and the reviewers. Any product that may be evaluated in this article or claim that may be made by its manufacturer is not guaranteed or endorsed by the publisher.
Research integrity at Frontiers
Learn more about the work of our research integrity team to safeguard the quality of each article we publish.