- 1Agricultural and Environmental Research Station, West Virginia State University, Institute, WV, United States
- 2Posgrado en Ingeniería Agrícola y Uso Integral del Agua, Universidad Autónoma Chapingo, Chapingo, Mexico
- 3Department of Biology, West Virginia State University, Institute, WV, United States
This study aimed to assess the microbiological quality of microgreen seeds purchased online, including the levels of total aerobic plate counts (APC), Escherichia coli/coliforms, mold & yeast, and the presence of Salmonella spp. and Listeria monocytogenes. Additionally, seed decontamination practices commonly found online were evaluated, involving soaking in water only (SDW), 3% hydrogen peroxide (H), and a “poor man's solution” (HV; a mixture of 3% hydrogen peroxide and 5% acidity white vinegar with water), with or without heat treatment at 50 and 60°C for 20 and 40 h. The effectiveness of these treatments was evaluated using Amaranth (AM), Borage (BO), and Carrot (CA) seeds in reducing Enterobacteriaceae, coliforms, and mold & yeast, along with examining the germination rate. A total of 102 samples composed of 14 species were purchased from online vendors, and the microbial levels tested were significantly different among the seed species (p < 0.05). Among the species, BO, CA, and Onion (ON) seeds exhibited the highest APC levels (4.99–5.37 log CFU/g), which was significantly higher than the APC of Arugula, Cabbage, Mustard, Kale, and Pea seeds (p < 0.05). The coliform population of BO (3.77 ± 0.68 log CFU/g) was significantly higher than in other species. The 10-min soaking of AM, BO, and CA in H significantly reduced the microbial levels (p < 0.05), and the subsequent heat treatment at 60°C for 20 h further enhanced microbial reduction (total 1.2–3.4 log reduction by heat and soaking). The germination rates were not significantly affected by the different treatment combinations (p > 0.05). This study provides scientifically-validated data for small-scale and home growers to ensure the safety and improve the quality of microgreens.
Introduction
Microgreens, the young and tender greens from vegetables, herbs, legumes, or grain seeds, are recognized as a new specialty crop for being grown in a controlled environment among small-scale growers (Kyriacou et al., 2016; Turner et al., 2020). Moreover, the popularity of home cultivation of microgreens has increased during and after the COVID-19 pandemic due to various reasons (Teng et al., 2023): firstly, microgreens offer a suitable option for home gardening, contributing to the enhancement of physical and mental wellbeing. Secondly, microgreens may address food security concerns in households with limited access to nutrient-rich food by utilizing user-friendly microgreen growing kits or methods. Indeed, purchasing microgreen seeds, substrates, and growing kits online offers convenience for individuals. However, no available reports exist regarding the microbiological quality of microgreen seeds and growing kits obtained through online purchases.
Microgreens differ from sprouts; Sprouts are typically harvested when the cotyledons (or seed leaves) are still undeveloped or underdeveloped, and true leaves have not yet begun to emerge [Food and Drug Administration (FDA), 2017]. The key distinction between sprouts and microgreens lies in their cultivation methods. Commercially, sprouts grow from seeds within rotating drums or sprouting containers, maintaining warm, and humid conditions. In contrast, microgreens are usually grown hydroponically or in soil substitutes. For sprouts, elevated humidity and the absence of light can create conditions more conducive to the proliferation of bacterial pathogens compared to microgreens (Xiao, 2013). It is worth noting that sprouts are consumed raw with the root system intact, whereas microgreens are typically eaten without the roots (Galieni et al., 2020), potentially reducing the risk of foodborne illness. Nevertheless, it is essential to ensure the safety of microgreens, as they, like other fresh produce, could be a potential source of foodborne illness (Riggio et al., 2019). While there have been no confirmed outbreaks associated with microgreens, there have been recalls due to the presence of Salmonella and Listeria monocytogenes in microgreens (Hamilton et al., 2023; Yeargin et al., 2023). Moreover, microgreens growers often face the microbiological quality issue such as damping-off (McGehee et al., 2019).
Like other fresh produce, microgreens are considered as covered produce under the FDA Produce Safety Rule (PSR) Part 112 (Federal Register, 2015), with voluntary compliance to subpart M, which specifically applies to sprouts (Misra and Gibson, 2021; Hewage et al., 2023). However, small-scale microgreen growers are not covered by the PSR if their selling is <$25,000 worth of produce annually. Nevertheless, growers should not overlook food safety practices in their production and handling processes (Riggio et al., 2019). Given the relative novelty of the microgreen industry, especially among small-scale growers, they often rely on empirical knowledge acquired from the internet (e.g., websites and online videos) or fellow growers (Misra and Gibson, 2021). In a survey conducted across the United States, Hamilton et al. (2023) discovered that the internet was the most popular source of educational resources for first-time microgreen growers.
The potential sources of microbial contamination in microgreens include contaminated seeds, growing media, and irrigation water during production (Işik et al., 2020). Additionally, poor worker hygiene, contaminated equipment/tools, cross-contamination, and inappropriate storage during harvest/post-harvest can contribute to contamination. The contaminated seed is one of the primary sources of pathogenic microorganism proliferation in microgreen production, particularly in the hydroponic culture system (Wright and Holden, 2018; Liu et al., 2019). It is worth mentioning that contaminated seeds have been identified as the most common source of sprout-related outbreaks, as recognized by the Food and Drug Administration (FDA) (2020). Regarding sprouts, the FDA Produce Safety Rule (PSR) mandates the inclusion of a scientifically-based seed treatment protocol in the seed treatment record to assess its effectiveness, which must be maintained. An example of a standard chemical decontamination method involves soaking seeds in a solution of 20,000 ppm calcium hypochlorite for 10–15 min [Food and Drug Administration (FDA), 1999]. However, there is currently no established standard or requirement for microgreen seed decontamination. In a recent study, Hewage et al. (2023) conducted an assessment of microgreen training materials available on the internet, totaling 223 (comprising 86 from Google and 137 from YouTube), to gauge their alignment with the PSR. Out of these 223 training materials, only 27 made mention of the purchase, reception, storage, and treatment of seeds. Moreover, 25 out of the 223 materials provided information on procedures involving seed rinsing, seed sanitization/disinfection, and the utilization of various chemicals such as hydrogen peroxide (H2O2), white vinegar, H2O2, bleach, and other antifungal agents, including essential oils. However, the materials did not indicate any science/evidence-based food safety guidelines or references with the information (Hewage et al., 2023).
The overall goal of this study to provide reliable and scientifically-valid information to small-scale and/or home growers. This study aims to assess the microbiological quality of microgreen seeds purchased online, including the total aerobic plate counts, coliforms, mold, and yeast, as well as the presence of Salmonella spp. and L. monocytogenes. Additionally, the study collected metadata such as vendor type, packaging type, organic vs. conventional seeds, and various microgreen seed species, to examine the effects of these variables on the microbiological quality. Furthermore, the study aims to evaluate seed decontamination practices commonly found online among growers.
Materials and methods
Part 1. Microbiological quality of microgreen seeds purchased from online vendors
Collection of microgreen seed samples
A total of 102 microgreen seed samples, comprising 14 species, Amaranth (AM), Arugula (AU), Borage (BO), Buckwheat (BW), Chard (C), Carrot (CA), Cabbage (CB), Kale (K), Mild mix (MM), Mustard (MS), Onion (ON), Pea (P), Sunflower (SL), and Spicy mix (SM), were acquired from 26 online vendors between August and November of 2022. The online vendors were identified through keyword searches using the terms “microgreen seed” and “variety.” Upon receipt in the laboratory, the seeds were randomly assigned codes and stored at a cabinet at room temperature. Processing occurred within 3 d of receipt. Product information, vendor type (seed company or individual seller), organic or non-organic status, packaging type (clear zip bag, paper-based without zipper, or sealed zipper pouch), GMO or non-GMO status, lot number, and any additional information (germination rate, storage recommendation, packed date, and growing recommendation, if available) were recorded.
Microbial load of microgreen seeds
A 25-g seed sample was aseptically transferred to a filter bag containing 125 mL of sterile 0.1% peptone water (SPW; BD, Sparks, MD) and pummeled for 1 min using a stomacher (Stomacher® 400, Seward, UK). The sample was subjected to 10-fold serial dilution using sterile 0.1% SPW and plated in duplicate onto Tryptic Soy Agar (TSA; BD, Sparks, MD), Dichloran 18% glycerol (DG18) agar (Neogen, Lansing, MI), and 3MTM PetrifilmTM coliform/E. coli Count Plates (3MTM, St. Paul, MN, USA) to enumerate total aerobic bacteria, mold and yeast, and coliform/Escherichia coli, respectively. The TSA and 3MTM PetrifilmTM plates were incubated at 37°C for 24 ± 1 h, while DG18 plates were stored at room temperature for 5 d for enumeration.
Detection of Salmonella spp. and Listeria monocytogenes
A 25-g seed sample was enriched with 225 mL of Buffered Peptone Water (BPW; Biorad, France) and Listeria Special Broth (LSB; Biorad, France) for 24 ± 1 h at 37 and 30°C, respectively. The enriched samples were dispensed to a deep well plate for bacterial genomic DNA extraction using iQ-Check™ Salmonella II and L. monocytogenes PCR Detection Kits (Hercules, CA). The extracted DNA were then processed in a thermal cycler (Bio-Rad, CFX), following the manufacturer's instructions (Bio-Rad Laboratories, Inc. Hercules, CA). The CFX manager™ IDE software (Bio-rad Laboratories, Inc. Hercules, CA) was used to run the thermal cycler and interpret the results, which involved analyzing the quantification cycle [Cq] values of each sample using an algorithm.
Part 2. The efficacy of seed treatment to reduce Enterobacteriaceae, coliform, and mold and yeast
Seed treatment
Three species, Amaranth (AM), Borage (BO), and Carrot (CA), which had the highest coliform population in the first part of the experiment, were selected. Approximately 1,400 g of each microgreen seed species were purchased from a seed supplier and thoroughly mixed using aseptic technique to ensure uniform distribution. Twelve grams of seed samples was placed into a stainless steel strainer, which was then placed inside a 4-ounce glass jar (10 g portion for microbial analysis and 2 g portion for the germination test). Subsequently, the seeds were subjected to a 10-min soaking using one of the following 50 mL solutions: sterilized distilled water (SDW), 3% hydrogen peroxide (H; Vaxxen Labs, Canada), or poor man's solution (HV; a mixture of 3% hydrogen peroxide, 5% acidity household white vinegar (Heinz, Pittsburgh, PA), and SDW (1:1:48, v/v). Following the treatment, the seeds were drained and rinsed three times with 50 mL of SDW. The drained seeds in the strainer were then transferred to a drying oven (Heratherm™, ThermoFisher). The seeds were subjected to dry heat treatments at temperatures of either 50 or 60°C for durations of 0, 20, and 40 h. Since microgreen seeds are typically evenly scattered on the growing substrate, heat treatment for drying purposes was included in the experimental design. For the mixture of 3% hydrogen peroxide and 5% vinegar, the level of peracetic acid was measured using a titration method with a peracetic acid test kit (Aquaphoenix Scientific, Hanover, PA). Untreated seeds without any soaking were used as the control (C).
Microbiological analysis
The untreated (C) and treated seeds (SDW, H, and HV) were analyzed on 0, 20, and 40 h of heat treatment, following the method outlined by Sharma and Demirci (2003). Briefly, a 10-g of the treated or untreated seed sample was transferred to 40 mL of 0.1% SPW for pummeling seeds for 1 min in a stomacher (Stomacher® 400, Seward, UK). The samples were serially diluted (1:10) in 0.1% SPW and plated in duplicate on MacConkey agar (BD, Sparks, MD), DG18 agar (Neogen, Lansing, MI), or 3MTM PetrifilmTM coliform/E. coli Count Plates (3MTM, St. Paul, MN) for enumerating Enterobacteriaceae, mold & yeast, or coliform, respectively. The detection limit for Enterobacteriaceae and mold & yeast or coliform/E. coli was 1.4 or 0.4 log CFU/g, respectively.
Germination test
Following the seed treatment process, a total of 100 seeds, whether treated or untreated, were subsequently placed on seed germination paper (Φ150 mm, Ahlstrom, Finland) moistened with ~15 g of SDW in sterile Petri dishes (Φ150 mm, VWR, Radnor, PA). Any visibly defective seeds were excluded. The Petri dishes were placed in a dark environment at room temperature for a period of 3–7 d, with regular watering as required. The germination rate was determined by calculating the percentage of seeds that exhibited visible radicle emergence.
Statistical analysis
All relevant seed metadata, including vendor type (seed company or individual seller), organic or non-organic status, packaging type (single zip, paper-based without zipper, or barrier pouch), and GMO or non-GMO status, were meticulously recorded in an Excel spreadsheet (Microsoft, Redmond, WA). The microbial data were presented as mean ± standard deviation and/or visually represented using an Excel spreadsheet. To assess the microbial quality of the seeds in Part 1 of the study, the duplicated counts of APC (aerobic plate count), coliform, and mold & yeast were recorded as logarithms of the number of colony-forming units (log CFU/g). In cases where no colonies were detected on the plates, indicating values below the detection limits (1.4 log CFU/g for mold and yeast, and 0.4 log CFU/g for coliform), those values were treated as missing values during statistical analysis. The microbial population (APC, mold & yeast, and coliform) associated with these metadata categories were analyzed using PROC Mixed with LSMEANS SIDAK test (SAS Institute, Cary, NC). Subsequently, the mean values of the variable(s) displaying a significance level of p < 0.05 were further compared using PROC GLM with the Duncan post-hoc test at a significance level of p < 0.05. For evaluating the seed decontamination treatment (Part 2), the values of coliform and mold & yeast below the detection limits were entered as 0.39 and 1.30 log CFU/g to simulate the worst case scenario. The seed treatments and germination tests were triplicated independently, and the means were compared based on heat treatment time and 10-min soaking treatment using a two-way ANOVA with the Duncan post-hoc test at a significance level of p < 0.05 (SAS Institute, Cary, NC).
Results and discussion
Microbiological quality of microgreens seeds
Table 1 presents the profile of microgreen seeds obtained from online vendors. A total of 102 samples, comprising 14 species, were purchased from 17 individual sellers on third-party marketplaces (n = 53) and nine seed companies (n = 49). The primary criterion for selecting samples was the variety and type of vendor. However, the desired sample count of 10 per variety was not able to be achieved due to limited availability. Out of the samples, 30 (29.4%) were classified as non-organic. Approximately 92% of the seed samples were categorized as non-GMO according to their labels. A mixed-effects model was employed to compare the mean values of the microbial population, taking into account factors such as vendor type, organic status, packaging type, and species. However, only the species factor showed a significant impact on the microbial population of the seeds (p < 0.05). Consequently, the microbial populations of aerobic plate count (APC), coliform/E. coli, and mold & yeast were compared across different seed species.
Figure 1 shows the APC obtained from the microgreen seed samples. Among the species, BO, CA, and ON exhibited the highest APC levels (4.99–5.37 log CFU/g). However, no significant differences were observed among BO, CA, ON, AM, MM, and SM (p > 0.05). On the other hand, the APC of AU, CB, MS, K, and P were significantly lower than those of BO, CA, and ON (p < 0.05). It should be noted that the APC level is primarily used to estimate the number of viable microbes and has limited relevance to food safety considerations. Table 2 presents the populations of coliform and mold & yeast, along with the screening results of Salmonella spp. and L. monocytogenes. Notably, the coliform population in BO seeds (3.77 ± 0.68 log CFU/g) was significantly higher than in other species. Additionally, while no coliforms were found in any P seeds, 100% of CA seeds tested positive for coliform. Enterobacteriaceae, coliforms, generic E. coli, and Listeria spp. are commonly used as indicator organisms to monitor fecal contamination in various food processing environments (Ruiz-Llacsahuanga et al., 2021). Although no E. coli was detected in any of the samples (with a detection limit of 0.4 log CFU/g), coliforms were still present in 49 samples collected (48% of 102). Additionally, real-time PCR was performed to screen for Salmonella spp. and L. monocytogenes in this study. Results revealed that one ON seed sample tested positive for Salmonella spp., while one CA seed sample tested presumptive positive for L. monocytogenes. However, no viable cells of these microorganisms were recovered from the samples.
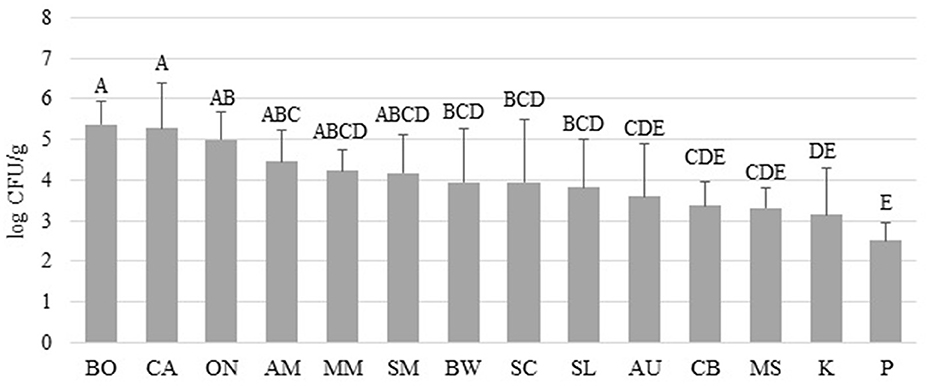
Figure 1. Total aerobic plate counts (APC) recovered from microgreen seed samples collected from online vendors by seed species. Different uppercase letters among the bars denotes significant differences (p < 0.05).
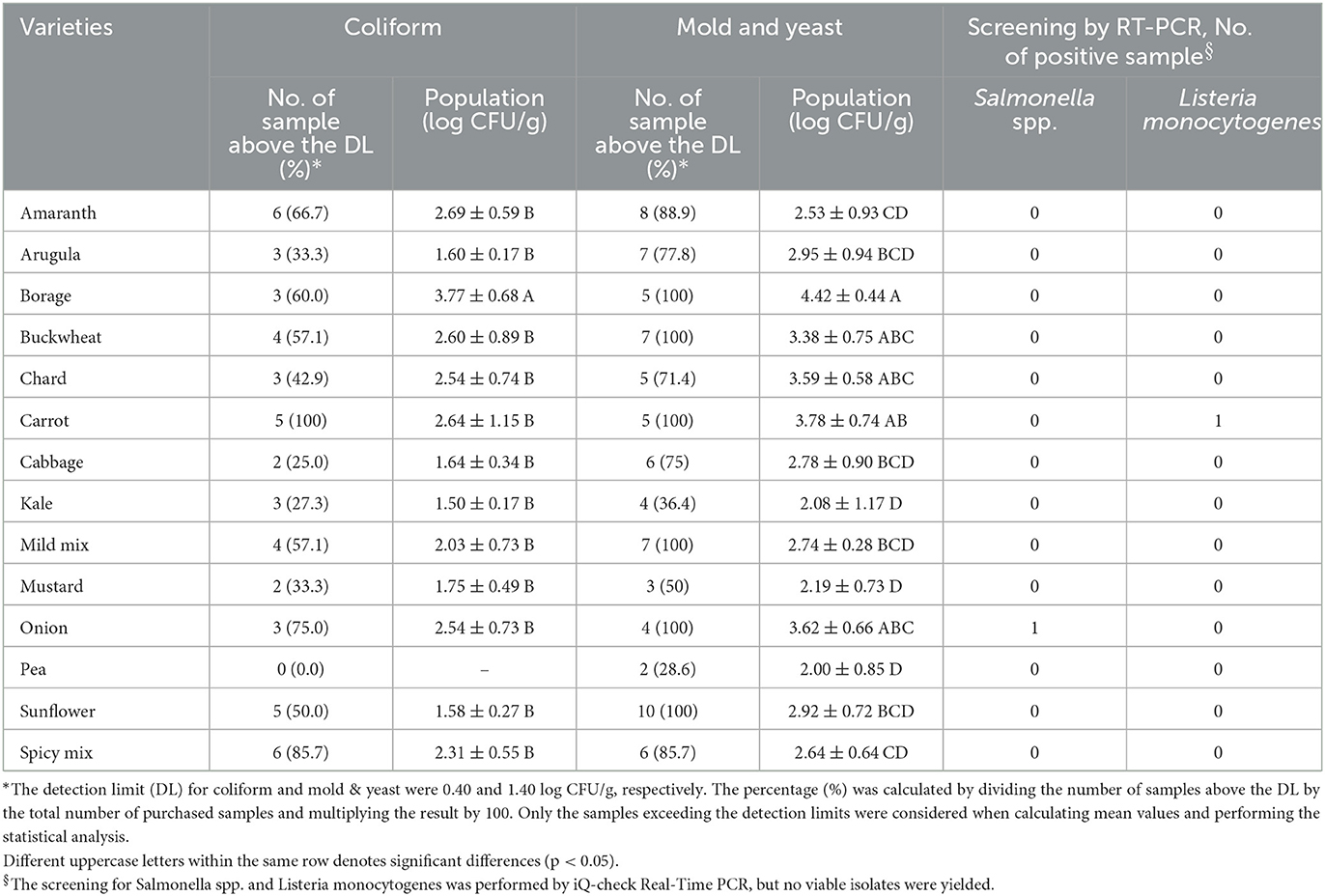
Table 2. Population of coliform and mold and yeast and screening for Salmonella spp. and Listeria monocytogenes in a variety of microgreen seed samples.
Pao et al. (2005) examined the microbiological quality of 30 organic and 30 conventional sprout seeds (alfalfa, broccoli, lentil, mung bean, and radish) sourced from the internet. The total plate counts ranged from 2.8 to 3.6 log CFU/g, and the counts for Bacillus cereus and coliforms were 0.7–1.0 log CFU/g and −0.5 to 0.0 log MPN/g, respectively. No Salmonella, E. coli O157, and Staphylococcus aureus were detected. The researchers found that organic seeds had lower counts for the detected microorganisms, but this current study did not find any significant difference in the counts of microbes tested between organic and non-organic seeds. In another study by Prokopowich and Blank (1991), the levels of total and fecal coliforms, APC, and Staphylococcus aureus were determined in dry alfalfa, onion, and mixed seeds (including alfalfa, radish, clover, mustard, and lentil seeds). The APC and coliform counts ranged from 3.5 to 6.6 log CFU/g and from zero to 4.0 log CFU/g, respectively. Fecal coliforms were only detected in the onion seeds, ranging from 0.9 to 3.0 log CFU/g. Bergšpica et al. (2020) collected nine microgreen seed samples from retail stores and local producers in Riga, Latvia to evaluate the occurrence of Shiga Toxin-Producing E. coli (STEC), Salmonella spp., and Listeria spp.; Among the samples, one was found to be positive for Salmonella spp. using MALDI-TOF mass spectrometry. Although this study did not recover any viable Salmonella spp. or L. monocytogenes, similar to previous research, some seeds were found to be contaminated with coliforms and other microbes. This contamination indicates a poor hygienic condition.
Microgreen seed decontamination practices
Seed contamination by undesirable microbes can lead to the persistence and proliferation of these microorganisms on the edible parts of microgreens throughout cultivation, thereby compromising both their quality and safety. Xiao et al. (2014) demonstrated that the inoculation of seeds with E. coli O157:H7 and O104:H4 led to the proliferation of these pathogens during microgreen growth. Similarly, the presence of Shiga toxin-producing E. coli (STEC; Wright and Holden, 2018) and Salmonella enterica (Reed et al., 2018) on microgreens seeds resulted in the colonization of these foodborne pathogens on the edible parts of the microgreens. Wright and Holden (2018) suggested that the same pre-germination treatment to that used for sprouts is necessary when growing microgreens, particularly hydroponically.
Hewage et al. (2023) assessed microgreen training materials available on the internet, specifically 86 from Google and 137 from YouTube. Among the 223 materials reviewed, nine training materials mentioned seed rinsing, while 16 materials mentioned sanitizing/disinfecting the seeds and using chemicals such as hydrogen peroxide (H2O2), bleach, and other antifungal agents (essential oil). Some growers empirically use food-grade hydrogen peroxide (3% H2O2) or a mixture of vinegar and 3% H2O2 with water, known as the “poor man's solution,” for sanitizing/disinfecting (Farmer Rex, 2019). Thus, this study evaluated the efficacy of soaking seeds in 3% H2O2, a “poor man's solution,”1 and water alone to reduce the microbial load. The “poor man's solution” is the mixture of vinegar (acetic acid, CH3CO2H) and hydrogen peroxide (H2O2), which generates peracetic acid (CH3CO3H +H2O). Indeed, peracetic acid is widely used in postharvest washing. Therefore, this study also measured the concentration of peracetic acid (PAA), which was found to be 43 ± 8 ppm.
Nonetheless, it is not common practice to soak or decontaminate seeds before sowing microgreen. For example, among the 14 species of microgreen seeds purchased for Part 1 of this study, only ON, BW, SL, and P were recommended for presoaking, as per the growing guidelines provided by the seed company. Notably, these seeds (ON, BW, SL, and P) are larger in size compared to the others (Figure 2). The main reason why soaking small seed species is not recommended among microgreen growers is the difficulty of evenly spreading them on the growing substrate after soaking. Therefore, a dry heat treatment was incorporated by drying the seeds at 50 and 60°C after the soaking. This heat treatment aimed to dry the previously wet seeds and enhance additional effects on reducing the microbial load. The germination rate was also evaluated post-treatment to determine the resulting seed viability.
Seed decontamination techniques: soaking and heating
Figure 3 shows the population of Enterobacteriaceae after soaking treatment followed by heat treatment for seed decontamination. Additionally, the Enterobacteriaceae load of the seeds subjected to no soaking (C) and no heat treatment after soaking (No heat) was determined to assess the effects of heat and washing alone. Regarding the AM seeds, the Enterobacteriaceae levels were reduced to near or below detection limits (1.4 log CFU/g) through the application of H and HV soaking treatments and/or 60°C heat treatment. It is worth noting that the initial Enterobacteriaceae level in AM seeds was significantly lower compared to BO and CA seeds (p < 0.05). The Enterobacteriaceae levels of AM seeds soaked in H and HV without heat treatment were found to be 1.99 and 2.18 log CFU/g, respectively, which were significantly lower than seeds soaked in SDW (3.34 log CFU/g; p < 0.05). No significant difference in Enterobacteriaceae populations was observed among C, SDW, H, and HV treatments for BO seeds without heat treatment (p > 0.05). Additionally, only the Enterobacteriaceae levels of H-treated CA seeds were significantly lower than those of C, SDW, and HV treatments when heat was not applied (p < 0.05). Heat treatment at 60°C for 20 and 40 h resulted in a significant reduction in Enterobacteriaceae levels compared to the “No heat” treated seeds in SDW, H, and HV-treated CA seeds (p < 0.05). However, the 50°C heat treatment did not yield any significant effect in reducing Enterobacteriaceae population, regardless of soaking treatment, for either the 20 or 40 h duration when compared to the “No heat” seeds; in fact, there was a slight increase in the Enterobacteriaceae level of CA seeds through the 50°C heat treatment compared to “No heat” seeds. Also, for BO and CA seeds, the Enterobacteriaceae populations exhibited an increase following a 40 h heat treatment compared to a 20 h heat treatment. Specifically, BO seeds subjected to HV soaking and CA seeds treated with SDW and H soaking demonstrated significantly higher populations after 40 h of heating at 60°C compared to the 20 h treatment (p < 0.05). This could be attributed to certain survival mechanisms of some Enterobacteriaceae that enable them to withstand heat stress, especially in the context of sub-lethal heat exposure (Arku et al., 2011). In low-moisture foods, the thermal tolerance of microorganisms is known to increase as water activity decreases (Podolak et al., 2017). Consequently, these bacteria may trigger adaptive responses, thereby bolstering their resistance and overall survival. Overall, the most effective reduction of Enterobacteriaceae was achieved through a 10-min soaking with 3% H2O2 (H) following a heat treatment at 60°C for 20 h; the seeds soaked in H and subjected to 60°C for 20 h resulted in a reduction of 1.8 log (AM), 1.46 log (BO), and 2.83 log (CA) per g of seeds, compared to the “No heat” and C seeds.
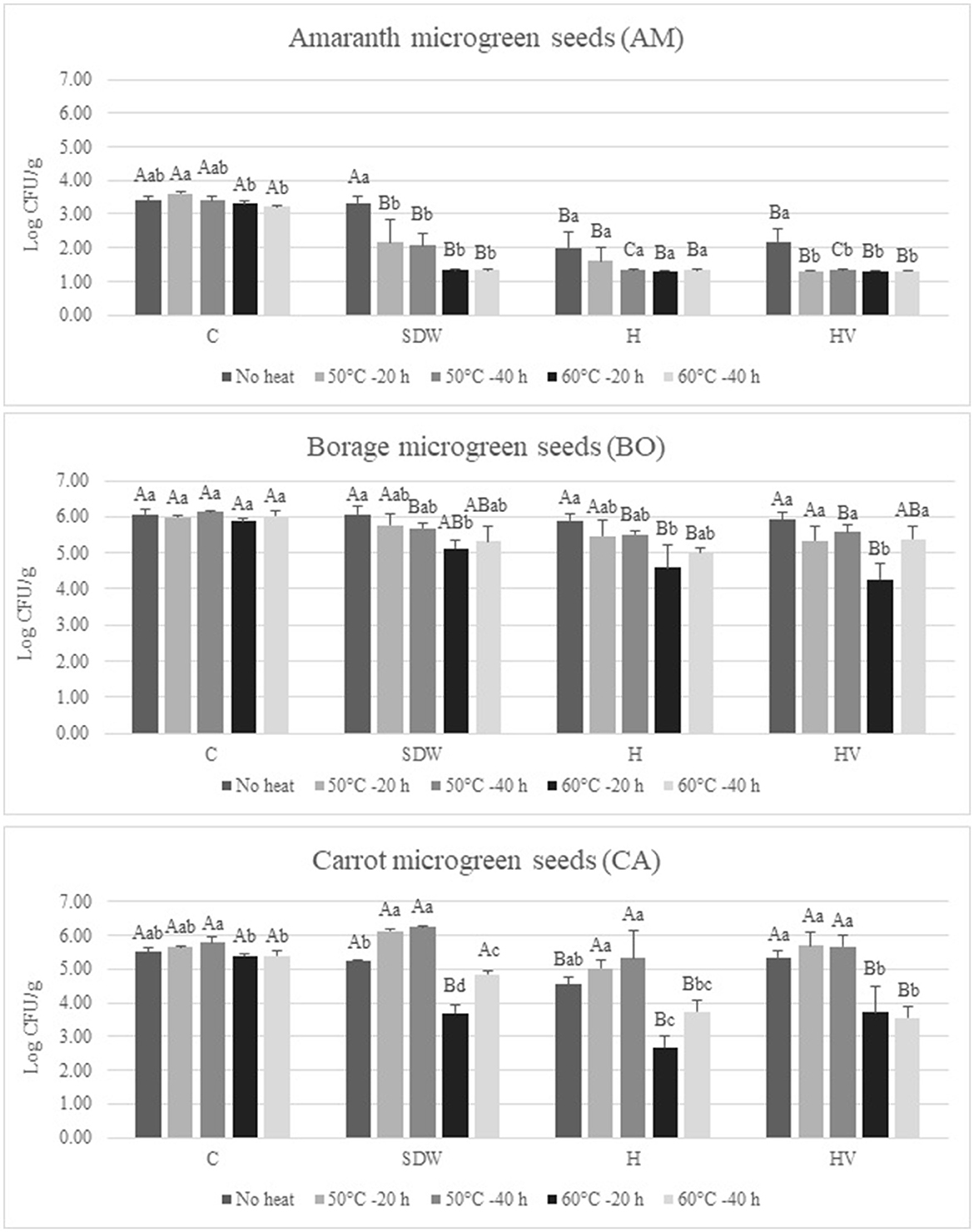
Figure 3. Enterobacteriaceae population after 10-min soaking treatment with or without heat treatment of microgreen Onion (ON), Borage (BO), and Carrot (CA) seed samples. Values represent the mean ± standard deviation (n = 3); C, no soaking; SDW, soaking in sterilized distilled water; H, Soaking in 3% hydrogen peroxide; HV, soaking in a mixture of 3% hydrogen peroxide and 5% acidity white vinegar in SDW (1:1:48); Different lowercase letters of each seed species represents significant differences among different heat treatments with a particular soaking treatment (p < 0.05); Different uppercase letters indicates significant differences for a particular heat treatment on different seed soaking treatment (p < 0.05).
Tables 3, 4 present the population of coliforms and molds & yeasts, respectively, after soaking treatment followed by heat treatment. The initial coliform population of BO was significantly higher than that of CA (p < 0.05). However, no significant difference was observed in the population of molds & yeasts between BO and CA (p > 0.05). The H and HV soaking treatments of the AM seeds, followed by a heat treatment at 60°C, reduced the coliform level below the detection limit. For BO seeds, the H and HV soaking, along with a heat treatment at 60°C for 20 h, significantly reduced the coliform level compared to the “No heat” seeds after soaking (p < 0.05). No significant effect was observed in reducing the coliform level of CA by heat treatment after SDW, H, and HV soaking (p > 0.05). However, seeds soaked in SDW, H, and HV without heat treatment (No heat) showed significantly lower coliform levels compared to the non-soaked seeds (C). This indicates that there was an effect on reducing coliforms in CA seeds by applying a 10-min soaking. Similar with Enterobacteriaceae and coliform, H and HV soaking with 60°C were significantly lower the mold & yeast population (p < 0.05). The H soaking with heat treatment at 60°C for 20 h reduced the population of mold & yeast compared to no soaking (C) and “No heat” seeds, having ~2.31 log reduction on BO and 3.4 log reduction on CA. Phornvillay et al. (2022) evaluated the efficacy of advanced oxidation process (H2O2 +UV-C) on decontamination, seed viability, and enhancing phytonutrients of Roselle Microgreens. The study found 5% H2O2 alone and H2O2 +UV-C showed significantly reduced the total coliform levels by 1.5–2 log reduction and enhanced germination rate compared to no washed seeds.
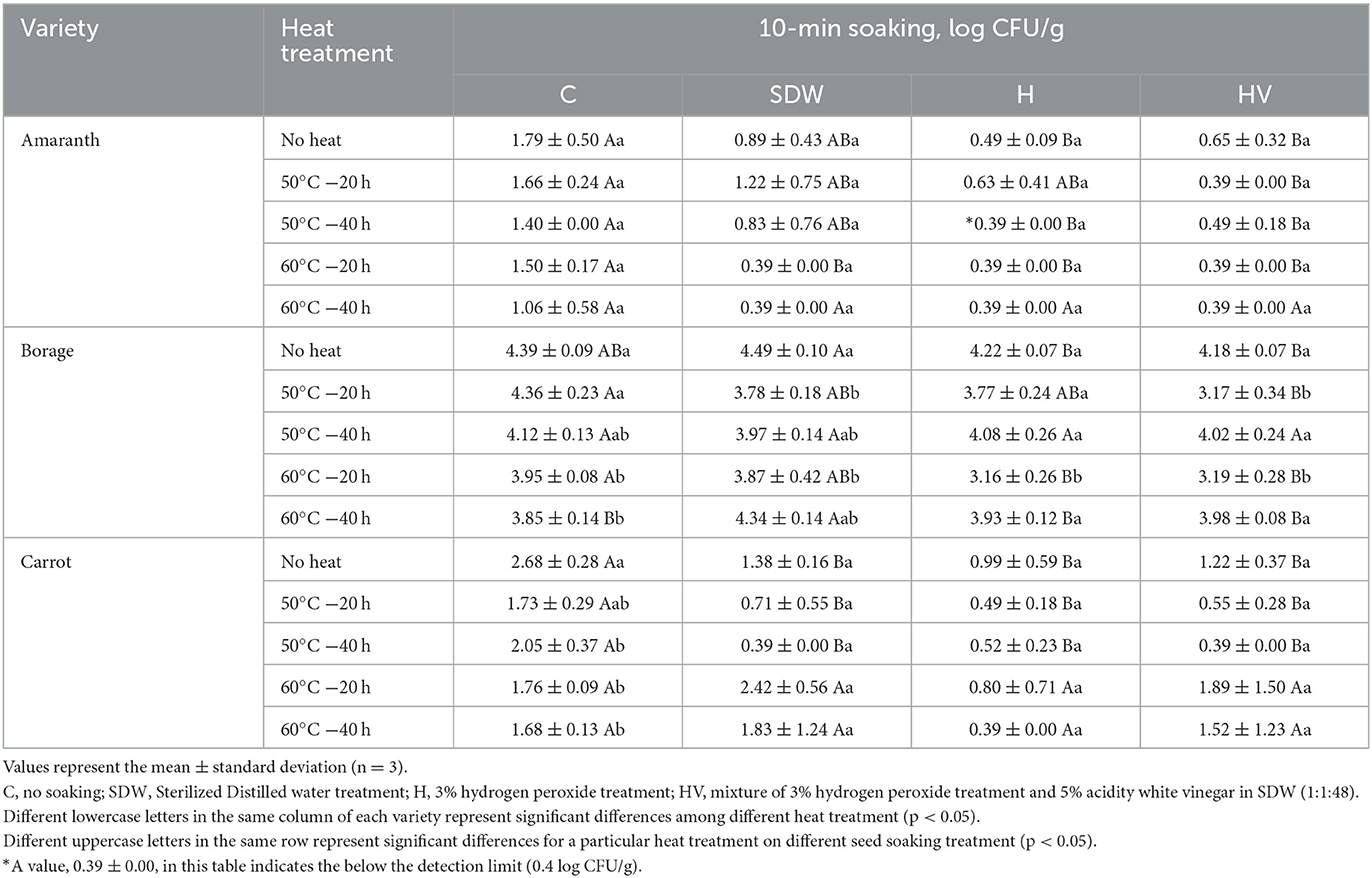
Table 3. Coliform population after soaking treatment with or without heat treatment of microgreen seed samples.
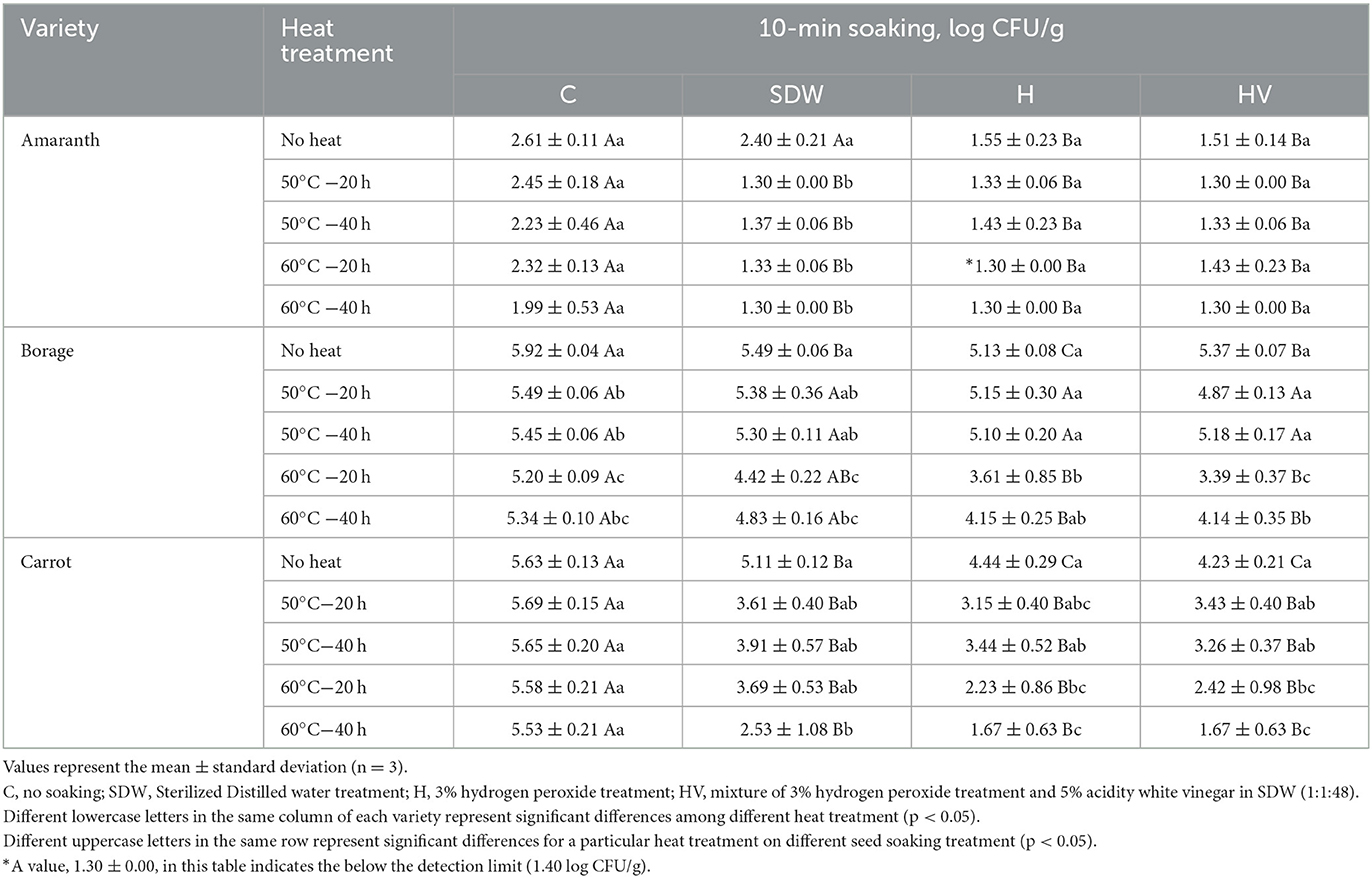
Table 4. Mold and yeast population after soaking treatment with or without heat treatment of microgreen seed samples.
Seed decontamination techniques of sprouts have been extensively studied, but not for microgreen seeds (Riggio et al., 2019). For example, the FDA recommended method with 20,000 ppm calcium hypochlorite achieved 3.08 ± 2.03 log CFU/g reduction on sprout seeds (Riggio et al., 2019). Hong and Kang (2016) investigated the effect of sequential dry heat (60, 70, and 80°C) and H2O2 on the inactivation of Salmonella Typhimurium on alfalfa seeds. They found that dry heat only reduced Salmonella Typhimurium by 0.26–2.76 log and sequential dry heat and H2O2 achieved 1.66–3.60 log reduction on alfalfa seeds. This study also revealed the germination rate of treated seeds by heat and H2O2 was significantly enhanced compared to untreated seeds. It is known that heat treatment can potentially have a negative impact on seed viability. However, certain seed species, such as alfalfa, exhibit heat tolerance (Hong and Kang, 2016). The germination rates of AM, BO, and CA without any soaking and heating treatment in this study were 96.0 ± 1.0, 94.3 ± 0.6, and 94.7 ± 0.6%, respectively (Table 5); however, the germination rates of the seeds subjected to the 10-min soaking and heat treatment were not significantly different (p > 0.05), regardless of the different soaking and heat temperature and duration, indicating that these treatments did not have a significant effect on the germination rate.
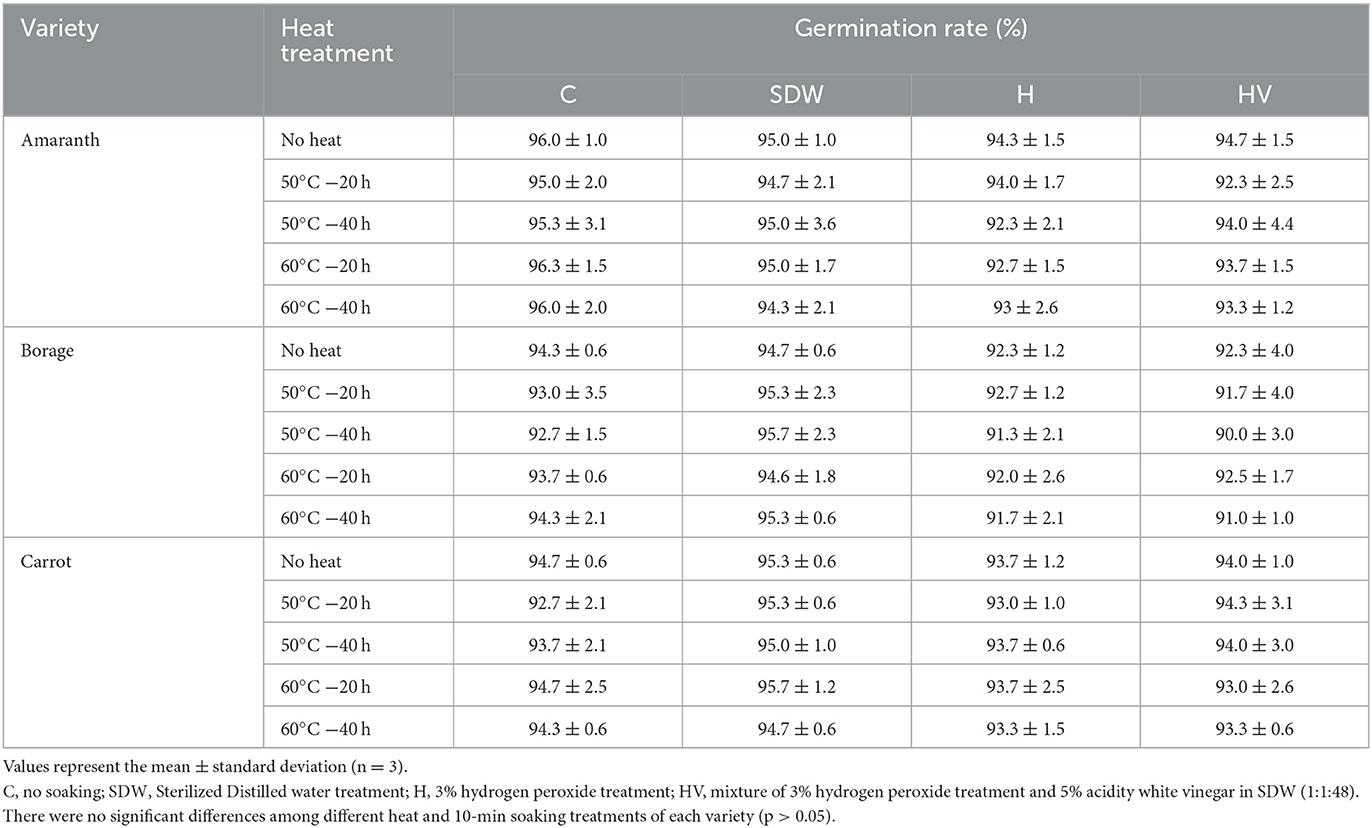
Table 5. Germination rate after soaking treatment with or without heat treatment of microgreen seed samples.
Seed decontamination techniques play a crucial role in enhancing the quality and safety of sprouts and microgreens. Researchers have investigated various techniques to reduce microbial populations, including foodborne pathogens. For example, studies have explored the application of aqueous and gaseous plasma on mung bean seeds (Darmanin et al., 2021), the use of alginate-based antimicrobial coatings on alfalfa seeds (Fu et al., 2022), and the application of gaseous chlorine dioxide and heat treatment on radish and cabbage seeds (Yeom et al., 2021). However, it is important to note that the effectiveness of seed treatment can vary depending on the types, textures, and surface characteristics of the seeds (Riggio et al., 2019). These variations in seed characteristics can impact the efficacy of different decontamination techniques. Therefore, it is essential to consider the specific characteristics of the seeds when selecting and implementing appropriate seed treatment methods. For example, C and BO seeds exhibit irregular and rough surfaces, while pea, buckwheat, and sunflower seeds have smooth and larger surfaces compared to others (Figure 2). These differences in surface characteristics may impact the efficacy of the decontamination techniques. Therefore, it is necessary to investigate and tailor the efficacy of different techniques to the specific species of microgreens.
It is also worth mentioning that this study did not evaluate the efficacy of the treatment with specific foodborne pathogens. Therefore, further research is needed to determine the efficacy of these treatments in reducing pathogenic microbes. Additionally, the efficacy of the treatment could vary depending on the initial microbial population and the quantity of seeds subjected to the treatment. Furthermore, when assessing the effectiveness of seed treatments, factors such as germination rate, production yield, and nutritional concentration should be taken into consideration to ensure that the treatments do not have any adverse effects on the overall quality and characteristics of the microgreens.
Conclusion
In conclusion, this study had two primary objectives: evaluating the microbiological quality of microgreen seeds from various sources and assessing the effectiveness of seed decontamination techniques. The findings revealed several important insights. First, E. coli was not detected in any of the samples, with a detection limit of 0.4 log CFU/g. However, coliforms were found in 48% of the 102 samples, and Borage seeds exhibited the highest coliform population at 3.77 ± 0.68 log CFU/g. Interestingly, Pea seeds had no coliform, while 100% of Carrot seeds tested positive for coliform. It is worth noting that no viable cells of Salmonella spp. or L. monocytogenes were recovered during the study. Regarding seed decontamination techniques, the 10-min soaking of Amaranth, Borage, and Carrot seeds in 3% H2O2 (H) significantly reduced the levels of Enterobacteriaceae, coliforms, and molds & yeasts (p < 0.05), except for the Enterobacteriaceae population of Borage. Additionally, a heat treatment at 60°C for 20 h, combined with the 10-min soaking, contributed an additional effect in reducing the microbial load in the tested microgreen seeds without adversely affecting germination rates. The findings from this study provide valuable insights for small growers, offering guidance on measures to enhance the safety and quality of microgreens. This is especially relevant given the potential for microgreens to support local growers through cost-effective production and their increasing popularity due to their numerous benefits.
Data availability statement
The raw data supporting the conclusions of this article will be made available by the authors, without undue reservation.
Author contributions
TO: Investigation, Methodology, Writing—original draft, Data curation. NL: Data curation, Investigation, Writing— review & editing. YJ: Investigation, Writing—review & editing, Conceptualization, Formal analysis, Funding acquisition, Methodology, Project administration, Resources, Software, Supervision, Validation, Visualization, Writing—original draft.
Funding
The author(s) declare financial support was received for the research, authorship, and/or publication of this article. This research was supported by funding from the USDA National Institute of Food and Agriculture (NIFA)'s Evans-Allen Capacity Grant.
Conflict of interest
The authors declare that the research was conducted in the absence of any commercial or financial relationships that could be construed as a potential conflict of interest.
Publisher's note
All claims expressed in this article are solely those of the authors and do not necessarily represent those of their affiliated organizations, or those of the publisher, the editors and the reviewers. Any product that may be evaluated in this article, or claim that may be made by its manufacturer, is not guaranteed or endorsed by the publisher.
Footnotes
1. ^Poor man's sanitizer for microgreens, https://riverforkfarms.com/fresh/river-fork-farms/poor-mans-sanitizer-for-microgreens/.
References
Arku, B., Fanning, S., and Jordan, K. (2011). Heat adaptation and survival of Cronobacter spp. (formerly Enterobacter sakazakii). Foodborne Pathog. Dis. 8, 975–981. doi: 10.1089/fpd.2010.0819
Bergšpica, I., Ozola, A., Miltina, E., Alksne, L., Meistere, I., Cibrovska, A., et al. (2020). Occurrence of pathogenic and potentially pathogenic bacteria in microgreens, sprouts, and sprouted seeds on retail market in Riga, Latvia. Foodborne Pathog. Dis. 17, 420–428. doi: 10.1089/fpd.2019.2733
Darmanin, M., Fröhling, A., Bußler, S., Durek, J., Neugart, S., Schreiner, M., et al. (2021). Aqueous and gaseous plasma applications for the treatment of mung bean seeds. Sci. Rep. 11:19681. doi: 10.1038/s41598-021-97823-1
Farmer Rex (2019). Poor Man's Sanitizer for Microgreens. Retrieved from: https://riverforkfarms.com/fresh/river-fork-farms/poor-mans-sanitizer-for-microgreens (accessed July 17, 2023).
Federal Register (2015). Standards for the Growing, Harvesting, Packing, and Holding of Produce for Human Consumption. Retrieved from: https://www.govinfo.gov/content/pkg/FR-2015-11-27/pdf/2015-28159.pdf (accessed July 17, 2023).
Food and Drug Administration (FDA) (1999). Guidance for Industry: Reducing Microbial Food Safety Hazards for Sprouted Seeds. US Food and Drug Administration.
Food and Drug Administration (FDA) (2017). Compliance With and Recommendations for Implementation of the Standards for the Growing, Harvesting, Packing, and Holding of Produce for Human Consumption for Sprout Operations: Guidance for Industry. Food and Drug Administration.
Food Drug Administration (FDA) (2020). Memorandum of Record: 2012 – Sprout-Related Outbreak Data. Retrieved from: https://www.regulations.gov/document/FDA-2014-N-0053-0096 (accessed July 17, 2023).
Fu, Y., Bhunia, A. K., and Yao, Y. (2022). Alginate-based antimicrobial coating reduces pathogens on alfalfa seeds and sprouts. Food Microbiol. 103:103954. doi: 10.1016/j.fm.2021.103954
Galieni, A., Falcinelli, B., Stagnari, F., Datti, A., and Benincasa, P. (2020). Sprouts and microgreens: trends, opportunities, and horizons for novel research. Agronomy 10:1424. doi: 10.3390/agronomy10091424
Hamilton, A. N., Fraser, A. M., and Gibson, K. E. (2023). Barriers to implementing risk management practices in microgreens growing operations in the United States: thematic analysis of interviews and survey data. Food Control 152:109836. doi: 10.1016/j.foodcont.2023.109836
Hewage, S. C. N., Makawita, A., Chandran, S., Gibson, K. E., and Fraser, A. M. (2023). Evaluating the alignment and quality of microgreens training materials available on the internet: a content analysis. J. Food Protect. 86:100021. doi: 10.1016/j.jfp.2022.100021
Hong, E. J., and Kang, D. H. (2016). Effect of sequential dry heat and hydrogen peroxide treatment on inactivation of Salmonella Typhimurium on alfalfa seeds and seeds germination. Food Microbiol. 53, 9–14. doi: 10.1016/j.fm.2015.08.002
Işik, H., Topalcengiz, Z., Güner, S., and Aksoy, A. (2020). Generic and Shiga toxin-producing Escherichia coli (O157: H7) contamination of lettuce and radish microgreens grown in peat moss and perlite. Food Control 111:107079. doi: 10.1016/j.foodcont.2019.107079
Kyriacou, M. C., Rouphael, Y., Di Gioia, F., Kyratzis, A., Serio, F., Renna, M., et al. (2016). Micro-scale vegetable production and the rise of microgreens. Trends Food Sci. Technol. 57, 103–115. doi: 10.1016/j.tifs.2016.09.005
Liu, D., Cui, Y., Walcott, R., Díaz-Pérez, J., Tishchenko, V., and Chen, J. (2019). Transmission of human enteric pathogens from artificially-inoculated flowers to vegetable sprouts/seedlings developed via contaminated seeds. Food Control 99, 21–27. doi: 10.1016/j.foodcont.2018.12.023
McGehee, C. S., Raudales, R. E., Elmer, W. H., and McAvoy, R. J. (2019). Efficacy of biofungicides against root rot and damping-off of microgreens caused by Pythium spp. Crop Protect. 121, 96–102. doi: 10.1016/j.cropro.2018.12.007
Misra, G., and Gibson, K. E. (2021). Characterization of microgreen growing operations and associated food safety practices. Food Protect. Trends 41:56. doi: 10.4315/1541-9576-41.1.56
Pao, S., Khalid, M. F., and Kalantari, A. (2005). Microbial profiles of on-line–procured sprouting seeds and potential hazards associated with enterotoxigenic Bacillus spp. in homegrown sprouts. J. Food Protect. 68, 1648–1653. doi: 10.4315/0362-028X-68.8.1648
Phornvillay, S., Yodsarn, S., Oonsrithong, J., Srilaong, V., and Pongprasert, N. (2022). A novel technique using advanced oxidation process (UV-C/H2O2) combined with micro-nano bubbles on decontamination, seed viability, and enhancing phytonutrients of Roselle microgreens. Horticulturae 8:53. doi: 10.3390/horticulturae8010053
Podolak, R., Lucore, L., and Harris, L. J. (2017). “Heat resistance of Salmonella and other bacterial pathogens in low-moisture foods,” in Control of Salmonella and Other Bacterial Pathogens in Lowmoisture Foods, eds R. Podolak and D. G. Black (Chichester: WileyBlackwell), 121–148. doi: 10.1002/9781119071051.ch6
Prokopowich, D., and Blank, G. (1991). Microbiological evaluation of vegetable sprouts and seeds. J. Food Protect. 54, 560–562. doi: 10.4315/0362-028X-54.7.560
Reed, E., Ferreira, C. M., Bell, R., Brown, E. W., and Zheng, J. (2018). Plant-microbe and abiotic factors influencing Salmonella survival and growth on alfalfa sprouts and Swiss chard microgreens. Appl. Environ. Microbiol. 84, e02814–e02817. doi: 10.1128/AEM.02814-17
Riggio, G. M., Wang, Q., Kniel, K. E., and Gibson, K. E. (2019). Microgreens—A review of food safety considerations along the farm to fork continuum. Int. J. Food Microbiol. 290, 76–85. doi: 10.1016/j.ijfoodmicro.2018.09.027
Ruiz-Llacsahuanga, B., Hamilton, A., Zaches, R., Hanrahan, I., and Critzer, F. (2021). Utility of rapid tests to assess the prevalence of indicator organisms (aerobic plate count, Enterobacteriaceae, coliforms, Escherichia coli, and Listeria spp.) in apple packinghouses. Int. J. Food Microbiol. 337:108949. doi: 10.1016/j.ijfoodmicro.2020.108949
Sharma, R. R., and Demirci, A. (2003). Treatment of Escherichia coli O157: H7 inoculated alfalfa seeds and sprouts with electrolyzed oxidizing water. Int. J. Food Microbiol. 86, 231–237. doi: 10.1016/S0168-1605(02)00549-4
Teng, Z., Luo, Y., Pearlstein, D. J., Wheeler, R. M., Johnson, C. M., Wang, Q., et al. (2023). Microgreens for home, commercial, and space farming: a comprehensive update of the most recent developments. Annu. Rev. Food Sci. Technol. 14, 539–562. doi: 10.1146/annurev-food-060721-024636
Turner, E. R., Luo, Y., and Buchanan, R. L. (2020). Microgreen nutrition, food safety, and shelf life: a review. J. Food Sci. 85, 870–882. doi: 10.1111/1750-3841.15049
Wright, K. M., and Holden, N. J. (2018). Quantification and colonisation dynamics of Escherichia coli O157: H7 inoculation of microgreens species and plant growth substrates. Int. J. Food Microbiol. 273, 1–10. doi: 10.1016/j.ijfoodmicro.2018.02.025
Xiao, Z. (2013). Nutrition, sensory, quality and safety evaluation of a new specialty produce: microgreens (Doctoral dissertation). University of Maryland, College Park, MD, United States.
Xiao, Z., Nou, X., Luo, Y., and Wang, Q. (2014). Comparison of the growth of Escherichia coli O157: H7 and O104: H4 during sprouting and microgreen production from contaminated radish seeds. Food Microbiol. 44, 60–63. doi: 10.1016/j.fm.2014.05.015
Yeargin, T. A., Lin, Z., do Prado, I., Sirsat, S. A., and Gibson, K. E. (2023). Consumer practices and perceptions regarding the purchasing and handling of microgreens in the United States. Food Control 145:109470. doi: 10.1016/j.foodcont.2022.109470
Keywords: microgreens, seeds, decontamination, microbial quality, soaking, online
Citation: Ocho Bernal TG, Lyttle N and Jung Y (2023) Microbiological quality of microgreen seeds purchased from online vendors and evaluating seed decontamination techniques available online. Front. Sustain. Food Syst. 7:1264472. doi: 10.3389/fsufs.2023.1264472
Received: 20 July 2023; Accepted: 30 October 2023;
Published: 17 November 2023.
Edited by:
M. Leonor Faleiro, University of Algarve, PortugalReviewed by:
Antonio Martínez, Spanish National Research Council (CSIC), SpainTongyin Li, Mississippi State University, United States
Célia Quintas, University of Algarve, Portugal
Wenjun Deng, Qingdao University, China
Copyright © 2023 Ocho Bernal, Lyttle and Jung. This is an open-access article distributed under the terms of the Creative Commons Attribution License (CC BY). The use, distribution or reproduction in other forums is permitted, provided the original author(s) and the copyright owner(s) are credited and that the original publication in this journal is cited, in accordance with accepted academic practice. No use, distribution or reproduction is permitted which does not comply with these terms.
*Correspondence: Yangjin Jung, eWFuZ2ppbi5qdW5nJiN4MDAwNDA7d3ZzdGF0ZXUuZWR1