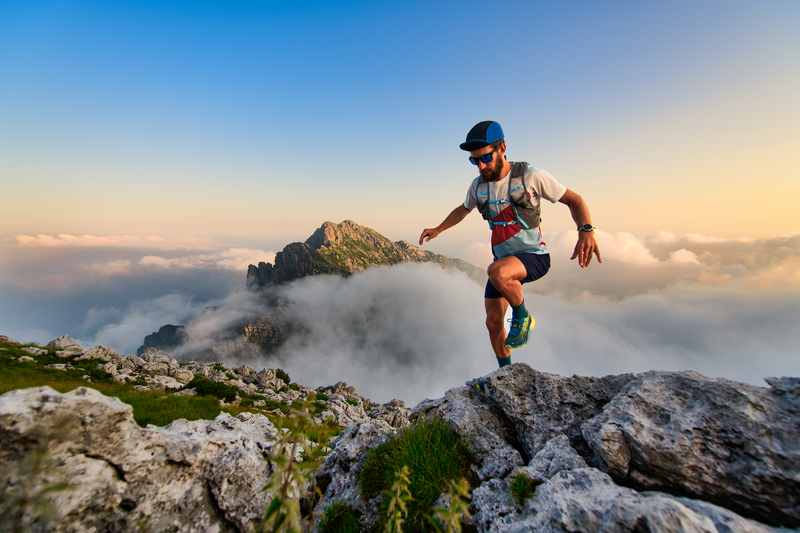
95% of researchers rate our articles as excellent or good
Learn more about the work of our research integrity team to safeguard the quality of each article we publish.
Find out more
ORIGINAL RESEARCH article
Front. Sustain. Food Syst. , 02 October 2023
Sec. Crop Biology and Sustainability
Volume 7 - 2023 | https://doi.org/10.3389/fsufs.2023.1237206
This article is part of the Research Topic Biostimulants for Climate-Smart and Sustainable Agriculture View all 12 articles
Plant growth-promoting microorganisms (PGPMs) and the specific compounds they produce have the capacity to mitigate the adverse effects of stressors on plants. An example in this regard is Thuricin 17 (Th17), a signal molecule produced by Bacillus thuringiensis NEB17 (Bt NEB17), a plant growth-promoting rhizobacterium. In this study, we aimed to determine the efficacy of Th17 in mitigating drought and the combination of drought and heat stress in canola [Brassica napus (L.)]. Two of the best Th17 concentrations, 10−9 (Th1) M and 10−11 (Th2) M, were used either as seed treatment plus root drenching or foliar spray. Leaf area and biomass accumulation was increased by both application methods of Th1 under moderate and severe drought stress, whereas more promising results were seen from Th2-treated plants under the combination of stressors. Additionally, root length, root surface, and root volume were increased by 21%, 22%, and 23%, respectively, for plants grown from Th1 seed treatment plus root drenching compared to controls under severe drought conditions. Moreover, SOD, POD, and CAT contents were increased by spraying Th1 and Th2 under individual stresses and the combination of heat and drought, respectively. Accordingly, increases in physiological variables were observed for sprayed plants, which also had higher antioxidant contents. These results indicated that plant responses to the compound varied with concentration of Th17 and plant growth conditions. Specifically, when plants were grown under an individual stress condition, either drought or heat, the higher level of Th17 was more effective, whereas the lower dose demonstrated higher positive impacts under the combination of heat and drought. Regarding application method, both seed treatment plus root drenching and foliar spray had the ability to assist plants in alleviating stresses through growth stimulatory mechanisms. Therefore, Th17 has potential to become an environmentally friendly biostimulant, particularly under stressful environmental conditions.
Crops are often simultaneously exposed to a variety of abiotic stresses in natural environments, which negatively impacts field crop development and productivity. Extreme temperatures and water deficit are two of the abiotic stressors posing the greatest threats to crop growth and yield, and consequently food security, under constantly changing climate conditions. Results of a meta-analysis from 120 studies regarding the combination of heat and drought demonstrates considerable negative influences on yield components and crop production (Cohen et al., 2021). The climatic estimates for the year 2100 anticipate a 50% rise in the number of regions affected by drought, hence, a significant reduction in agricultural production seems almost certain. Heat waves and drought periods have both increased in frequency and severity, with more detrimental effects on agriculture than other climatic extremes (IPCC et al., 2022). Plants can tolerate, avoid, or escape abiotic stresses through evolved abiotic stress adaptation mechanisms or deliberate selection in agricultural breeding programs. However, the responses to the individual stresses could not be directly extrapolated to a combinations of stresses. Heat and drought responses are commonly regulated by several genes, and the underlying mechanisms are more complex than other stressors, such as biotic stress, which is largely defined by monogenic resistance. Moreover, other abiotic and/or biotic factors frequently have an additive effects on heat and drought responses, which causes studies in this field to be more complex (Deutsch et al., 2018; Lamaoui et al., 2018). Indications of drought stress, when soil water drops below a specific threshold, as detected through plants roots, results in ABA hormonal-signal transduction. Transduction of this hormonal signal within xylem sap causes stomatal closure, to reduce transpiration. A decrease in the stomatal conductance and carbon dioxide (CO2) diffusion tremendously decreases carbohydrate production, leading to less crop production (Chatterjee and Solankey, 2015; Giordano et al., 2021). Heat waves, which are typically associated with drought, make this effect worse by hastening soil drying and worsening water vapor pressure deficit. Heat stress also hinders photosynthesis in plants, largely by interfering with metabolic processes including denaturation of proteins, enzymes, nucleic acids, and cell membranes (Nadeem et al., 2018; Janni et al., 2020). Additionally, the equilibrium of reactive oxygen species (ROS) production and scavenging could be disrupted by abiotic stressors, causing accumulation of these extremely reactive and toxic materials. Accordingly, plants have evolved defense mechanisms through activation of antioxidant enzymes such as superoxide dismutase (SOD), peroxidase (POD), and catalase (CAT) to scavenge ROS in cells (Zhou et al., 2019; Zhanassova et al., 2021). Hence, sustainable technologies are essential to overcome the challenges of increasing agriculture production under rapidly changing climatic conditions. In the past three decades, a number of technical innovations have been proposed to boost crop production. In this regard, the development of plant biostimulants, including microorganisms and/or substances they produce, could be a promising approach for addressing these pressing issues (Yakhin et al., 2017; Chiaiese et al., 2018; Antar et al., 2021; Shah et al., 2021). Among biostimulants, microbial-derived compounds could provide plants with necessary features to develop and grow by enhancing access to nutrients (Matse et al., 2020; Tang et al., 2020), producing phytohormones (Cassán et al., 2014; Moon and Ali, 2022), improving antioxidant defense system functionality (Khalilzadeh et al., 2018; Neshat et al., 2022), and/or inhibiting harmful microorganisms (Suryadi et al., 2019; Xia et al., 2020; Aioub et al., 2022) under both non-stressful or stressful conditions. Regarding the extracellular substances of PGPMs, some of these can be bacteriocins, which can control the dynamics of the plant-associated microbial population (phytomicrobiome) by acting against microbial strains closely related to the producer strain. Numerous bacterial taxa, including those found in the rhizosphere microbiome, have been shown to produce at least one bacteriocin, and Bacillus species were one of the first groups to be examined for the production of various bacteriocins. Bt NEB17 was isolated from soybean root nodules by our laboratory, which was since determined to produce and excrete a bacteriocin named Thuricin 17 (Th 17); none of the other discovered bacteriocins have been studied as extensively as Th17 (Gray et al., 2006a). This signal molecule was partially sequenced by Gray et al. (2006b) and its full sequence was eventually determined (Lee et al., 2009). The molecular weight of Th17 is low, 3.162 kDa, and this compound is highly resistant to heat and pH (range of 1.0–9.25) with anti-microbial activity and plant growth promotion capacity, particularly under stressful conditions (Jung et al., 2011; Prudent et al., 2015; Schwinghamer et al., 2016; Subramanian et al., 2016; Nazari and Smith, 2020; Nazari et al., 2022). We should add that the most recent characterization of Th17 has revealed differences from our initial understating; hence we are considering renaming it Bacillin 20. The use of bacteriocins in the food industry is of great importance, but little research has been done on their agronomic potential. Therefore, we are here attempting to determine the potential role of Th 17 in growth and development of canola, a valuable crop producing one of the healthiest oils for human consumption, under drought and the combination of heat and drought. This study is the perquisite experiment to examine two concentrations and application methods of Th17 for further field studies to develop a biostimulant to make crops more resilient to climate change.
Th 17 was extracted and purified according to Gray et al. (2006a,b). In brief, King's B medium was used to culture Bt NEB17 as previously mentioned (Gray et al., 2006a). Bacterial cells were collected from plated material and cultured in 250 ml flasks with 50 ml medium for the initial broth inoculum. The bacterium was grown for 48 h on an orbital shaker revolving at 150 rev min−1 at 28 ± 2°C. Next, 5 ml of subculture were added to 2 liters of broth as an initial culture, and the culture was grown under the same conditions on the shaker. Bacterial populations were measured after 96 h with a Pro UV/Visible Spectrophotometer at 600 nm. Bacterial cultures were grown to an O.D.600nm of at least 1.4 or ~5.5 log CFU (colony forming units) cells ml−1 (Gray, 2005). Bacterial culture samples were centrifuged at 13,000 g for 10 min, then cell-free supernatants (CFS) were used for analytical-HPLC identification. For partial purification of Th17, 0.8 L of butanol was added to 2 L of the culture for 12 h, after which the upper layer was collected for rotary evaporation. The resulting viscose extract was diluted with 12 ml of 30% acetonitrile (ACN). The sample was centrifuged at 13,000 g for 10 minutes, followed by serial fractionation with ACN and HPLC identification. The chromatographic conditions were set as follows: column—Vydac C18 reversed-phase column (0.46 × 25 cm; 5μ), 25°C temperature, 1 ml/min flow rate, 214 nm detector wavelength, and a gradient of 18%−95% throughout the 18-min run. By comparing the retention time of a standard Th17 sample, the corresponding peak to Th17 was found (Figure 1). According to chromatographs, the highest peak of Th17 was observed once the peptide collected in 60% ACN. This fraction was lyophilized and then stored at −20°C for dilution to the required levels. Here, Th17 at 10−9 M (Thu1) and 10−11 M (Thu2) were used in all experiments.
Figure 1. HPLC chromatograms for butanol extraction of the bacterial culture (A) Th17 standard (B) and Th17 collected in 60% ACN (C).
Untreated canola seeds of cultivar (cv.) InVigor L233P were used; this hybrid is recommended for short to mid-length growing season zones. For seed disinfection, 20% bleach (6% sodium hypochlorite, NaOCl) was used; seeds were then rinsed with distilled water until odorless. Th17 levels were applied either as a pre-planting seed treatment followed by root drench or spray at the time of stress. For the seed treatment experiments, seeds were soaked in 10 ml of two concentrations of Th17, 10−9 and 10−11 M, suggested from germination data analysis (Nazari et al., 2022), and distilled water as a control, which was followed by weekly root drenching of the compound (three times in total) until stress induction time (based on the initial experiments), while Th17 solutions and distilled water were sprayed on leaves with an atomizer just prior to stress induction for foliar experiments. For leaf sprays, Tween 20 (0.01%) was added as a surfactant to treatment solutions, including the control. To prevent dripping of the treatment solutions onto the soil, vinyl plastic was placed over the top surfaces of the pots. Canola seeds were placed in 10 cm pots filled with AGRO MIX® G10 media. The plant growth chamber (Conviron R, Canada) conditions were as follows: 22/18°C day/night, photoperiod of 14/10 h light/darkness cycle, 60%−70% relative humidity, and photosynthetic irradiance of 350–400 μmol m−2 s−1 (Nazari et al., 2022). The plants were trimmed to one seedling per container after a week. Plants were grown until the end of the third week and regularly watered using half strength Hoagland's solution. Then, 3-week-old plants were exposed to four levels of the stressors, where one level was the control. To induce uniform water stress, polyethylene glycol 8000 (PEG) was used; levels were control −0.25 MPa (half strength Hoagland solution for control), mild drought −0.5 MPa (197 g L−1 PEG), moderate drought −0.9 MPa (270 g L−1 PEG), or severe drought −1.3 MPa (330 g L−1 PEG) (Michel, 1983). For foliar experiments, the leaves were sprayed with Th17 solutions and water as the control, until the leaves were uniformly wet at the onset of stress induction. The temperature levels were 22/18°C day/night for drought stress experiments, whereas for the heat and drought combination, the same levels of drought stress were applied, but at 30/18°C day/night (Elferjani and Soolanayakanahally, 2018). In each case, the plants were allowed to grow for 2 weeks following the onset of stress treatment, and then sampled for data collection. For determination of plant physiology responses to the treatments, photosynthetic rate, transpiration rate, and stomatal conductance inside the leaves were measured (LI-COR 6400 portable photosynthesis meter at a constant CO2 concentration of 400 ppm and light intensity of 500 μmol m−2 s−1); readings were taken from the upper-most fully expanded leaf of each plant 1 day before stress onset, 1 day after, 1 week after, and just prior to harvesting. Harvested plants at the end of the experiment were used for leaf area, fresh weight, dry weight, and root trait data collection. For measuring root variables, after harvesting, soil was gently shaken off from roots followed by washing and using WinRHIZO™ in order to scan and measure total root length, total root surface, root volume, and root diameter.
Five hundred milligrams of fresh leaf tissue were ground to a fine powder in liquid nitrogen and then homogenized in 1.2 ml of 0.2 M sodium phosphate buffer (pH 7.8 with 0.1 mM EDTA). The samples were centrifuged at 13,000 g × for 10 min at 4°C and the supernatant was then subjected to enzyme activity assays. All spectrophotometric analyses were conducted on an Ultrospec (4,300 pr) UV/visible spectrophotometer. SOD activities were using the method employed by Giannopolitis and Ries (1977). The reaction mixture contained 50 ml of extract, 50 mM Tris-HCl (pH 8.0), 63 mM NBT, 1.3 mM riboflavin, 13 mM methionine, and 0.1 mM EDTA. One unit of SOD was determined as the amount of enzyme required to produce 50% inhibition of the rate of nitro blue tetrazolium photoreduction measured at 560 nm. POD activities were determined using the Britton and Mehley (1955) method; the reaction mixture contained 0.1 ml of extract, 50 L of 20 mM tetraguaiacol, and 2.8 ml of 50 mM Tris-HCl buffer (pH 8.0). By adding 20 μl of 40 mM H2O2 the reaction started, and after recording the change in absorbance at 470 nm for 1 min, POD activity was calculated using the extinction coefficient of tetraguaiacol. One unit of activity was determined by the required amount of enzyme for the formation of 1 μmol of tetraguaiacol min−1. The CAT assay method described by Hugo and Lester (1984) were used to measure enzyme activities by monitoring oxidation of H2O2. The enzyme activity was calculated using extinction coefficient of H2O2 (40 M−1 cm−1 at 240 nm), which is expressed as the amount of enzyme activity required to decompose 1 μmol of H2O2 per minute.
The experiments were organized following a factorial randomized design with four replicates. Each experiment was repeated twice, and the data pooled for analysis using SAS 9.4; differences between means were considered statistically significant at p < 0.05, using Tukey's test. All analyses were conducted separately for seed treatment plus root drenching and foliar spray experiments since comparison of application methods is not a goal for this study. Two-way variance analysis was conducted, where Th17 treatment was one factor and stressful conditions was another. When the interaction of the compound and stressors was absent, one-way ANOVA was performed to indicate the main effects. For physiological variables, two-way repeated measures ANOVA was applied at each stress level.
Leaf area is an important indicator of vegetative growth and strongly responded to Th17 and stressors. For seed treatment plus root drenching experiments, leaf area significantly decreased across all drought levels for controls without Th17 treatment, where it dropped by ~50% under severe drought, whereas significant reductions for Th17-treated plants occurred only under severe stress. Under moderate and severe drought stress alone, Th1-treated plants had 12.5 and 14.5% more leaf area than controls, respectively (Figure 2A). Similarly, spraying Th1 significantly increased leaf area by 13% compared to non-sprayed plants under severe drought (Figure 2B). Under non-stressful conditions, no stimulatory effects of Th17 were reported. For heat and drought experiments, significant decreases in leaf area occurred across heat and drought combinations. Seed treatment plus root drenching of Th2 significantly enhanced leaf area (by 27%) under the combination of moderate drought and heat stress (Figure 2C). Interestingly, the interaction of spray application and stressful conditions was significant (p = 0.03) in that foliar spraying of Th2 could significantly offset decreases in leaf area, by ~19%, when moderate drought and heat occurred simultaneously (Figure 2D). However, neither seed treatment nor foliar spray could substantially offset leaf area reduction under severe drought and heat combination.
Figure 2. Effect of Th17 application on leaf area under drought (A, B) and combination of drought and heat (C, D). (A, C) Seed treatment plus root drenching and (B, D) foliar spray of distilled water (Control), Th1 (10−9 M) and Th2 (10−11M). Combination of lower and capital letters represents one-way ANOVA results for main effects; capital letters represent differences between different growth conditions at the same level of Th17 whereas lower letters indicate differences between different levels of Th17 at the same growth conditions. The absence of lower and capital letter combination indicates significant interaction of Th17 and stressful conditions by two-way ANOVA. Each bar represents mean ± standard error (n = 8). Means with the same letters are not significantly different (p < 0.05).
The interaction of drought stress and Th17 seed treatment plus root drenching was significant (p = 0.04) in that Th17 increased fresh biomass accumulation under either stressful or normal conditions. The highest fresh biomass resulted from seed treatment with Th17 plus root drenching, for Th2, at 37 g under normal conditions; however, severe drought decreased fresh biomass accumulation by 40% compared to optimal conditions, while Th1 offset this reduction by 27% (Figure 3A). Similar results were observed for plants sprayed with Th1; it significantly increased fresh biomass accumulation by 23 and 21% under moderate and severe drought conditions, respectively (Figure 3B). A drastic decline of fresh biomass was observed when a combination of drought and heat stress was imposed, although application of Th2 had significant stimulatory effects, resulting in 24 and 28% more biomass accumulation under moderate stresses for seed treatment plus root drenching (Figure 3C) and foliar spray (Figure 3D) experiments, respectively. Moreover, those plants grown with Th17 treatments, both application methods, could produce more fresh biomass under heat stress than controls, but the differences were not significant (Figures 3C, D).
Figure 3. Effect of Th17 application on fresh biomass under drought (A, B) and combination of drought and heat (C, D). (A, C) Seed treatment plus root drenching and (B, D) foliar spray of distilled water (Control), Th1 (10−9 M) and Th2 (10−11 M). Combination of lower and capital letters represents one-way ANOVA results for main effects; capital letters represent differences between different growth conditions at the same level of Th17 whereas lower letters indicate differences between different levels of Th17 at the same growth conditions. The absence of lower and capital letter combination indicates significant interaction of Th17 and stressful conditions by two-way ANOVA. Each bar represents mean ± standard error (n = 8). Means with the same letters are not significantly different (p < 0.05).
For seed treatment plus root drenching experiments, significant decreases in biomass accumulation were observed under severe drought stress while application of Th1 caused 16 and 14% more biomass production under conditions of moderate and severe drought, respectively, compared to the controls (Figure 4A). However, the interaction of drought stress and Th17 leaf spraying was significant (p = 0.02); Th1 increased biomass accumulation with greater increases at more severe drought stress levels (Figure 4B). When the two stressors were combined, considerable biomass reductions were reported across all combinations. However, Th17 applications, either seed treatment plus root drenching (Figure 4C) or spraying (Figure 4D), could enhance dry biomass compared to controls, although those increases were not depicted statistically significant, except for the effect of spraying of Th2 where dry biomass increased by 19% compared to control under moderate drought and heat combination.
Figure 4. Effect of Th17 application on dry biomass under drought (A, B) and combination of drought and heat (C, D). (A, C) Seed treatment plus root drenching and (B, D) foliar spray of distilled water (Control), Th1 (10−9 M) and Th2 (10−11 M). Combination of lower and capital letters represents one-way ANOVA results for main effects; capital letters represent differences between different growth conditions at the same level of Th17 whereas lower letters indicate differences between different levels of Th17 at the same growth conditions. The absence of lower and capital letter combination indicates significant interaction of Th17 and stressful conditions by two-way ANOVA. Each bar represents mean ± standard error (n = 8). Means with the same letters are not significantly different (p < 0.05).
The interaction of Th17 seed treatment plus root drenching and drought stresses was significant for all root variables (Figure 5) except diameter: root length (p < 0.04), root surface (p < 0.01), and root volume (p < 0.01) were significantly increased. At increased drought stress levels, Th1 remarkably increased root length, presumably to help plants gain better access to water; this variable ranged from 2,882 cm for untreated seeds under well-watered conditions to 5,090 cm in response to Th1 seed treatment plus root drenching under severe drought conditions. Similarly, the greatest root volume (2.9 cm3) and root surface (410 cm2) were reported for plants grown with Th1 seed treatment plus root drenching. However, neither seed treating plus root drenching nor leaf spraying resulted in significant impacts under control (unstressed) conditions. Likewise, spraying Th17 did not significantly stimulate root development under drought conditions; roots merely responded to drought levels in which the greatest level of root attributes occurred under severe drought conditions (Table 1). Root diameter did not respond to growing conditions or Th17 applications.
Figure 5. 5-week-old Canola root images of seed treatment plus root drenching with distilled water (A), 10 −9 M Th17 (B), C: 10−11 M Th17 (C) under control, mild, moderate, and severe drought stress, respectively.
In contrast to individual stresses, root variables considerably decreased under the combination of both stresses. Table 2 indicates that increasing the severity of stresses had negative effects on root growth and development where neither watering with Th 17 supplements nor foliar spray caused significant changes compared to controls. The greatest amounts, 3,664 cm for root length, 306 cm2 for surface, and 2.13 cm3 for volume, were for Th2 seed treatment plus root drenching under the combination of mild drought and heat. On the contrary, Th17 seed treatment plus root drenching treatments greatly assisted in developing better under-ground development under heat conditions; root length, root surface, and root volume increased by 30%, 27%, and 25%, respectively, for 10−9 M Th17 (Th1) seed treatment plus root drenching. In terms of root diameter, no significant changes were reported across all treatments and stressful circumstances.
Plants under PEG-induced drought stress showed significant increases in the amount of SOD, POD, and CAT. For seed treatment plus root drenching experiments, antioxidant enzyme contents were considerably affected by drought stress levels; the greatest numbers, 181 unit mg−1 fw for SOD (Th2), 119 units mg−1 fw for POD (Control), and 24 units mg−1 fw for CAT (Th2), were recorded under severe drought stress levels (Table 3). However, for foliar spray, the interaction of spraying the compound and drought levels was significant for SOD (p < 0.05), POD contents (p < 0.02), and CAT (p < 0.04); they were increased by 56%−29%, 31%−18%, and 56%−58% respectively in Th1 foliar sprayed plants compared to controls, under moderate to severe drought levels. When drought and heat were combined, antioxidant enzyme contents enhanced by increasing the severity of drought level, where the highest number of enzymes were reported under the combination of severe drought and heat conditions for both Th17 application experiments. Specifically, the antioxidant enzyme levels in plants grown from treated seeds plus root drenching were quite similar to untreated plants in a way that stress levels seem to be the stimulant for changes. For foliar experiments, enzymes did not meaningfully respond to Th17 under heat or the combination of heat and mild drought but did respond under the moderate and severe combination with heat (Table 4). Accordingly, the greatest increases, 33 and 20%, were observed with Th2 foliar sprayed plants over the controls for SOD, once heat was combined with moderate and severe drought, respectively. Similarly, spraying Th2 significantly increased the amount of POD and CAT by 26 and 56% under the combination of heat and severe drought stress, respectively.
Figure 6 indicates that photosynthetic rate was considerably reduced by all stresses; drought aggravated it to a greater extent than heat, and it was greatly inhibited by the heat and drought stress combination. Under optimal growing conditions, no significant effect of treatments, either seed treatment plus root drenching or foliar spraying of Th17, was observed. Photosynthetic rate of plants grown from treated seeds and drenched roots did not show meaningful changes compared to untreated plants under drought and the combination of drought and heat (Figures 6A, C). However, spraying Th17 could alleviate reductions related to stresses compared to unsprayed plants. Assimilation rates in those plants which were supplementary sprayed with Th1 demonstrated increases during all measurement times across drought levels; particularly mean photosynthetic rate significantly increased by 14%, 23%, and 32% compared to controls under mild, moderate, and severe drought conditions, respectively, 1 day after spraying, whereas this significant stimulatory effect lasted until 1 week after spraying under severe drought conditions (Figure 6B). Likewise, spraying the supplement, in particular Th2 one day after spraying, caused meaningful responses in carbon assimilation rate under heat stress alone and the combinations of heat with drought levels (Figure 6D). Interestingly, the effect of the sprayed supplements could significantly last until 2 weeks and 1 week under individual heat and combination of mild drought and heat stresses, respectively.
Figure 6. Effect of Th17 application on photosynthetic rate under drought (A, B) and combination of heat and drought (C, D) at 1 day before stress induction (T1), 1 day (T2), 1 week (T3), and two weeks (T4) after stress induction. (A, C) Seed treatment plus root drenching and (B, D) foliar spray of distilled water (Control), Th1 (10−9 M) and Th2 (10−11M). A black line within the box marks the mean (n = 8), respectively. Boxes with an asterisk are significantly different at each measurement time and stress level (p < 0.05).
Stomatal conductivity was greatly decreased by drought, but the decreases were smaller when both stresses were applied simultaneously; instead, heat stress increased stomatal conductance. No statistically significant changes in the stomatal conductivity resulted from Th17 applications either when the supplement was applied to seeds plus root drenching or spraying across all conditions. Specifically, plants developed from Th17 treated seeds plus root drenching had insignificantly greater stomatal conductivity during almost all measurements for drought, heat and the combination of heat and drought (Figures 7A, C). Equally, for foliar experiments, stomatal conductance for sprayed plants was slightly higher than controls, particularly for Th1 under drought and Th2 when drought stress was accompanied by heat stress, but they were not significant (Figures 7B, D).
Figure 7. Effect of Th17 application on stomatal conductivity under drought (A, B) and combination of heat and drought (C, D) at 1 day before stress induction (T1), 1 day (T2), 1 week (T3), and two weeks (T4) after stress induction. (A, C) Seed treatment plus root drenching and (B, D) foliar spray of distilled water (Control), Th1 (10−9 M) and Th2 (10−11M). A black line within the box marks the mean (n = 8), respectively. Boxes without an asterisk are not significantly different at each measurement time and stress level (p < 0.05).
The results of measuring transpiration rate at four different times for both seed treatment plus root drenching and spraying experiments demonstrated that compared to optimal conditions, it was slighlty reduced by heat while sharp declines were observed when the plants were subjected to drought and a combination of drought and heat stresses; however, the decreases were greater under drought alone (Figures 8A, B) where the lowest transpiration rate resulted from severe drought stress. Both treated plants, either seed plus root drenching (Figures 8A, C) or sprayed ones (Figures 8B, D), had marginally higher transpiration rates than controls across all stressful conditions, although they were not statistically distingushable.
Figure 8. Effect of Th17 application on transpiration rate under drought (A, B) and combination of heat and drought (C, D) at 1 day before stress induction (T1), 1 day (T2), 1 week (T3), and two weeks (T4) after stress induction. (A, C) Seed treatment plus root drenching and (B, D) foliar spray of distilled water (Control), Th1 (10−9 M) and Th2 (10−11M). A black line within the box marks the mean (n = 8), respectively. Boxes without an asterisk are not significantly different at each measurement time and stress level (p < 0.05).
Plants will be encountering higher average temperatures and more extreme drought episodes due to climate change, which will potentially cause marked declines in their productivity. Hence, studies regarding the discovery of potential approaches to assist crops in combating stressful conditions are a primary concern. Various research findings have proven the stimulatory effect of PGPMs under stressful conditions (Sarkar et al., 2018; Khan et al., 2019; Lin et al., 2020; Shah et al., 2021; Mellidou and Karamanoli, 2022); however, studies regarding the efficacy of microbial-derived materials such as signal molecules or cell-free supernatants are limited. Signal compounds are secreted into the producer strains growing medium, which, after being filtered, will still maintain the metabolites that possess plant growth stimulatory effects. Here, our experiments regarding the application of a bacterially produced compound, Th17, under stressful conditions highlights that compound concentration, stress level, and application method play pivotal roles in the effectiveness of Th17 as a plant growth biostimulant. The responses of plants to a single stress can be completely different from the conditions in which several stresses coincide. In this regard, the interaction of Th17 application with growing conditions was significant for several variables, while Th17 treatments caused different reactions at different stress levels. Plant morphological, physiological, and biochemical features were considerably affected by the induction of stresses where signal molecule treatments could partially assist in mitigating stress-associated damage. Above-ground morphological traits, including leaf area and fresh and dry biomass, were enhanced by the application of Th17 under stressed conditions; however, no distinguishable impacts were observed under optimal growth conditions. Specifically, the bacterial compound produced better results under moderate and severe drought stresses alone, but when the two stressors were combined, their effectiveness was reduced, particularly under the combination of severe drought and heat, which could be due to reasons such as high intensity of PEG induced-drought level or prolongation of stresses. This implies that increasing the PEG level with heat decreased the efficacy of Th17 compared to its effectiveness under less stressful conditions. Interestingly, plants respond to the higher concentration of the compound, Th1, for induvial stresses, either drought or heat; however, a lesser concentration (Th2) is required to alleviate the effect of stress combinations. A similar conclusion, distinct responses to different concentrations of bacterial compounds, was reached by other studies (Schwinghamer et al., 2015, 2016; Naamala et al., 2022). A clear example of this was observed in our previous study when three different concentrations of Th17, 10−7, 10−9, and 10−11 M, were applied, and nanomolar of Th17 was the most effective in enhancing seed germination, seedling growth, and vegetative growth under stressfully high temperatures (Nazari et al., 2022). Our findings are consistent with the work of Schwinghamer et al. (2016) regarding the application of different levels of two microbial compounds, LCO and Th17, which indicated concentration-dependent behavioral responses under various growth conditions and development stages. Similarly, previous work showed that specific concentrations of Th17 can enhance soybean [Glycine max (L.) Merr.] and corn (Zea mays L.) growth (Lee et al., 2009).
Drought stress broadly inhibits above-ground growth and increases dry matter allocation to the root portion of the plant, which results in a lowering of the shoot-to-root ratio. Additionally, PEG-induced osmotic stress mediates premature differentiation of the root apical meristem outgrowth of lateral roots. Through a highly complex process comprising an integrated network of antioxidants, metabolisms, redox, and hormonal regulation, plants can increase the water absorption area by encouraging the development of long, extensive root hairs and lateral roots under drought stresses (Ober and Sharp, 2003; Duan et al., 2010; Ji et al., 2014; Zia et al., 2021). Consistent findings are seen in our experiments; root variables, including total root length, total surface area, and volume, were increased with increasing PEG levels, whereas declines in root development were observed in the presence of heat and drought simultaneously. The interaction of seed treatment plus root drenching with Th17 and drought levels was significant and resulted in a more developed root system than that of controls, particularly at moderate and severe drought levels as well as under heat stress alone.
In contrast, no significant effects of the seed treatment plus root drenching were seen when drought and heat were combined, which could be due to the extreme stress levels leading to morphological, biochemical, and physiological damage, and consequently lower carbohydrate assimilation into roots. Additionally, for foliar experiments, across all conditions, no meaningful changes were observed due to the application of the compound. Our previous studies have indicated positive effects of treating canola seeds and root drenching with 10−9 and 10−11 M Th17, which caused germination increases, germination time reduction, and longer radicals under moderately high temperature and optimal conditions (Nazari et al., 2022). Similar root development effects on soybean have been reported for this plant growth regulator under salt stress and this was associated with changes in the level of ABA (Prudent et al., 2015). This might be one of the possible underlying mechanisms of root stimulation by Th17. As such, ABA is produced in the roots and transported to above-ground parts through xylem; it assists in stomatal closure, suppression of shoot growth, and maintenance of root elongation by crosstalk communication with phytohormones (Turan et al., 2021; Parwez et al., 2022). In another work, levels of auxin, which actively functions in cell division and lateral root development, and salicylic acid were increased in Arabldopsis thaliana rosettes in response to 10−9 M Th17 (Subramanian, 2014). All these findings are suggestive of a shift in the balance of plant hormones related to roots due to seed treatment and root drenching, which could be a possible explanation of the root system modification by Th17 application.
Plants are equipped with a compelling array of defenses, comprised of enzymatic and nonenzymatic systems, to reduce the accumulation of reactive oxygen species (ROS), which cause oxidative damage to lipids, proteins, and DNA, under stressful growing conditions. Responses of enzymatic activities to various stressors, either alone or in combination, differ. In this regard, PGPMs and their active compounds have been shown to aid plants in scavenging excessive ROS species (Zhou et al., 2016, 2019; Gowtham et al., 2022; Neshat et al., 2022; Sati et al., 2022). Likewise, we found that exposure to stressful conditions increased levels of antioxidant enzymes compared to optimal growth conditions. Rather than the single effect of stress stimuli, very interestingly, foliar spray of the microbial compound demonstrated significant positive interactions with drought stress levels; the greatest numbers of SOD, POD, and CAT were recorded in Th1 sprayed plants under severe drought stress whereas spraying a lesser concentration of the compound (Th2) produced better results when drought and heat were combined. Nevertheless, treating seeds plus root drenching with the supplement could not meaningfully assist plants in the production of more antioxidant enzymes compared to untreated plants. In another study, the effect of two types of PGPR-produced bacteriocins, BF4 and Th17, on the plant defense system illustrated that antioxidant enzyme levels considerably increased in treated soybean leaves compared to untreated ones (Jung et al., 2011). Similarly, results of omics studies highlighted the role of two microbial signal molecules, LCO and Th17, in stress tolerance of A. thaliana, in which energy and antioxidant pathway proteins were increased in treated plants, causing salt stress mitigation as opposed to controls (Subramanian, 2014). At this stage of understanding, we propose that boosting the antioxidant enzyme system could be one of the possible underlying mechanisms of stress alleviation, however, it seems certain that there are still many things to learn about this compound.
Under unfavorable conditions, considerable changes in plant physiological mechanisms occur, beginning with perception of stress signals, which trigger a series of molecular events, resulting in various levels of responses. We found that cooccurrence of heat and drought stresses led to a higher reduction in photosynthetic rate than either drought or heat stress alone, and drought stress caused declines to a larger extent than heat stress. Additionally, the negative effects of drought stress on carbon assimilation may contribute to stomatal closure, which resulted in decreased intercellular CO2; however, heat stress increased the conductivity of stomata and CO2 diffusion into leaves. They have implied the existence of different underlying mechanisms in photosynthesis reduction by drought and heat stress. Our observations suggest that the stomatal factors under drought stress and non-stomatal factors, including, but not limited to, damage to the photosynthetic apparatus, inactivation of some enzymes under heat stress, and the combination of stressors, caused declines in photosynthesis (Wang et al., 2010, 2018; Carmo-Silva et al., 2012; Distéfano et al., 2017; Fatma et al., 2021; Lal et al., 2021). Heat stress also increases transpiration, to cool leaves, reducing leaf water potential and exacerbating plant conditions under heat and drought combinations. Likewise, lower leaf temperatures were observed in lipo-chitooligosaccharides (LCO) treated plants, related to enhanced transpiration and to stomatal conductance or membrane permeability (Schwinghamer et al., 2016). Our findings link well with previous studies wherein similar physiological behaviors in response to stressors were depicted (Elferjani and Soolanayakanahally, 2018; Wang et al., 2018; dos Santos et al., 2022). However, treating plants with Th17 could compensate for photosynthesis reduction compared to controls. We found that spraying Th1 onto leaves could enhance carbon assimilation across all drought levels and heat stress alone in such a way that the greatest effect of the compound was detected, at a statistically significant level, 1 day after treatment. Under the combination of heat and drought, the positive impact of the lesser concentration, Th2, was noticeable one day after spraying across all stressors and combinations. In contrast, plants grown from Th17 treated seed plus root drenching showed inconsistent results. No significant effects were observed from these treatments, except for the notable impact of Th1 under heat stress alone 1 day after stress induction. Accordingly, plants with higher carbon assimilation rates showed slightly higher stomatal conductance and transpiration, but their effects were not significantly different from the controls. From this point, it could be suggested that non-stomatal factors more actively contributed to resulting higher efficiency of the photosynthesis than stomatal effects. In this regard, higher levels of antioxidant enzymes, as described earlier for those plants, could be one of the possible explanations for reduced levels of ROS, and consequently, cause less damage to photosystems and enzymes. Others have shown that leaf spray or root drenching of Th17 increased photosynthetic leaf rates and leaf greenness of soybean (Lee et al., 2009). In line with previous studies, Th17-treated plants had greater photosynthetic rates due to having more developed root systems and improved functions related to carbon and nitrogen acquisition (Prudent et al., 2015). The efficacy of Th17 in stimulating the photosynthetic rate was consistent with what has been found in our previous studies; the carbon assimilation enhanced in response to Th17, both Th1 and Th2, at moderately high temperatures (Nazari et al., 2022). Regardless of all positive responses, further agricultural field studies would be our next step to see if statistically different results are biologically different or not.
We found clear responses to Th17 concentrations related to growth conditions where plants reacted differently to the simultaneous prevalence of heat and drought as compared with individual stresses. Precisely, regardless of application method, 10−11 M treated plants could better tolerate the combination of heat and drought stress than the other concentrations and control; conversely, more promising results were caused by 10−9 M under individual stresses. Our results demonstrated that both application methods could assist plants in combating stressful conditions by varying mechanisms; however, no significant effects were seen for optimal growth conditions. Stimulation of the root system to uptake more water and nutrients might be one of the possible modes of action for seed treatment plus root drenching. Additionally, we speculate that spraying the compound increased the most important antioxidant enzymes contents, which scavenged excessive ROS and reduced associated damage to cells, consequently redcuing cell damage and increasing the efficiency of the photosystems. Collectively, Th17 has the potential to be a plant growth regulator, plus these results should be translated into real agricultural field setting. Further studies are aslo required to invistigate the signal's mode of action at molecular levels.
The raw data supporting the conclusions of this article will be made available by the authors, without undue reservation.
MN designed the experiment, collected data, and wrote the manuscript. DS advised on the scientific approach and provided editorial input and intellectual context as well as funding. All authors approved the submitted version.
This study was funded through a grant from Eastern Canadian Oilseeds Alliance (ECODA).
The authors declare that the research was conducted in the absence of any commercial or financial relationships that could be construed as a potential conflict of interest.
All claims expressed in this article are solely those of the authors and do not necessarily represent those of their affiliated organizations, or those of the publisher, the editors and the reviewers. Any product that may be evaluated in this article, or claim that may be made by its manufacturer, is not guaranteed or endorsed by the publisher.
Aioub, A. A., Elesawy, A. E., and Ammar, E. E. (2022). Plant growth promoting rhizobacteria (PGPR) and their role in plant-parasitic nematodes control: a fresh look at an old issue. J. Plant Dis. Prot. 129, 1305–1321. doi: 10.1007/s41348-022-00642-3
Antar, M., Gopal, P., Msimbira, L. A., Naamala, J., Nazari, M., Overbeek, W., et al. (2021). “Inter-organismal signaling in the rhizosphere,” in Rhizosphere Biology: Interactions Between Microbes and Plants, eds V. V. S. R. Gupta, and A. K. Sharma (Singapore: Springer), 255–293. doi: 10.1007/978-981-15-6125-2_13
Britton, C., and Mehley, A. (1955). Assay of catalase and peroxidase. Meth. Enzymol. 2, 764–775. doi: 10.1016/S0076-6879(55)02300-8
Carmo-Silva, A. E., Gore, M. A., Andrade-Sanchez, P., French, A. N., Hunsaker, D. J., Salvucci, M. E., et al. (2012). Decreased CO2 availability and inactivation of Rubisco limit photosynthesis in cotton plants under heat and drought stress in the field. Environ. Exp. Bot. 83, 1–11. doi: 10.1016/j.envexpbot.2012.04.001
Cassán, F., Vanderleyden, J., and Spaepen, S. (2014). Physiological and agronomical aspects of phytohormone production by model plant-growth-promoting rhizobacteria (PGPR) belonging to the genus Azospirillum. J. Plant Growth Regul. 33, 440–459. doi: 10.1007/s00344-013-9362-4
Chatterjee, A., and Solankey, S. (2015). “Functional physiology in drought tolerance of vegetable crops: an approach to mitigate climate change impact” in Climate Dynamics in Horticultural Science, eds M. L. Choudhary, V. B. Patel, and M. W. Siddiqui 1st ed. (Palm Bay, FL: Apple Academic Press), 149–171.
Chiaiese, P., Corrado, G., Colla, G., Kyriacou, M. C., and Rouphael, Y. (2018). Renewable sources of plant biostimulation: microalgae as a sustainable means to improve crop performance. Front. Plant Sci. 9, 1782. doi: 10.3389/fpls.2018.01782
Cohen, I., Zandalinas, S. I., Huck, C., Fritschi, F. B., and Mittler, R. (2021). Meta-analysis of drought and heat stress combination impact on crop yield and yield components. Physiol. Plant. 171, 66–76. doi: 10.1111/ppl.13203
Deutsch, C. A., Tewksbury, J. J., Tigchelaar, M., Battisti, D. S., Merrill, S. C., Huey, R. B., et al. (2018). Increase in crop losses to insect pests in a warming climate. Sci. Total Environ. 361, 916–919. doi: 10.1126/science.aat3466
Distéfano, A. M., Martin, M. V., Córdoba, J. P., Bellido, A. M., D'ippólito, S., Colman, S. L., et al. (2017). Heat stress induces ferroptosis-like cell death in plants. J. Cell Biol. 216, 463–476. doi: 10.1083/jcb.201605110
dos Santos, T. B., Ribas, A. F., de Souza, S. G. H., Budzinski, I. G. F., and Domingues, D. S. (2022). Physiological responses to drought, salinity, and heat stress in plants: a review. Stresses 2, 113135. doi: 10.3390/stresses2010009
Duan, Y., Zhang, W., Li, B., Wang, Y., Li, K., Han, C., et al. (2010). An endoplasmic reticulum response pathway mediates programmed cell death of root tip induced by water stress in Arabidopsis. New Phytol. 186, 681–695. doi: 10.1111/j.1469-8137.2010.03207.x
Elferjani, R., and Soolanayakanahally, R. (2018). Canola responses to drought, heat, and combined stress: shared and specific effects on carbon assimilation, seed yield, and oil composition. Front. Plant Sci. 9, 1224. doi: 10.3389/fpls.2018.01224
Fatma, M., Iqbal, N., Sehar, Z., Alyemeni, M. N., Kaushik, P., Khan, N. A., et al. (2021). Methyl jasmonate protects the PS II system by maintaining the stability of chloroplast D1 protein and accelerating enzymatic antioxidants in heat-stressed wheat plants. Antioxidants 10, 1216. doi: 10.3390/antiox10081216
Giannopolitis, C. N., and Ries, S. K. (1977). Superoxide dismutases: I. Occurrence in higher plants. Plant Physiol. 59, 309–314. doi: 10.1104/pp.59.2.309
Giordano, M., Petropoulos, S. A., and Rouphael, Y. (2021). Response and defence mechanisms of vegetable crops against drought, heat and salinity stress. Agriculture 11, 463. doi: 10.3390/agriculture11050463
Gowtham, H. G., Singh, S. B., Shilpa, N., Aiyaz, M., Nataraj, K., Udayashankar, A. C., et al. (2022). Insight into recent progress and perspectives in improvement of antioxidant machinery upon PGPR augmentation in plants under drought stress: a review. Antioxidants 11, 1763. doi: 10.3390/antiox11091763
Gray, E. J. (2005). Identification of a Novel Bacteriocin, Thuricin 17, Produced by Bacillus thuringiensis NEB17 Public Deposited [Master of Science]. Montréal, QC: McGill University. doi: 10.1111/j.1574-6968.2005.00054.x
Gray, E. J., Di Falco, M., Souleimanov, A., and Smith, D. L. (2006b). Proteomic analysis of the bacteriocin thuricin 17 produced by Bacillus thuringiensis NEB17. FEMS Microbiol. Lett. 255, 27–32.
Gray, E. J., Lee, K., Souleimanov, A., Di Falco, M., Zhou, X., Ly, D., et al. (2006a). A novel bacteriocin, thuricin 17, produced by plant growth promoting rhizobacteria strain Bacillus thuringiensis NEB17: isolation and classification. J. Appl. Microbiol. 100, 545–554. doi: 10.1111/j.1365-2672.2006.02822.x
Hugo, A., and Lester, P. (1984). Catalase in vitro. Meth. Enzymol. 105, 121–126. doi: 10.1016/S0076-6879(84)05016-3
IPCC, Pörtner, H.-O., Roberts, D. C., Tignor, M., Poloczanska, E. S., Mintenbeck, K., et al. (2022). Climate Change 2022: Impacts, Adaptation and Vulnerability. Geneva: IPCC.
Janni, M., Gullì, M., Maestri, E., Marmiroli, M., Valliyodan, B., Nguyen, H. T., et al. (2020). Molecular and genetic bases of heat stress responses in crop plants and breeding for increased resilience and productivity. J. Exp. Bot. 71, 3780–3802. doi: 10.1093/jxb/eraa034
Ji, H., Liu, L., Li, K., Xie, Q., Wang, Z., Zhao, X., et al. (2014). Peg-mediated osmotic stress induces premature differentiation of the root apical meristem and outgrowth of lateral roots in wheat. J. Exp. Bot. 65, 4863–4872. doi: 10.1093/jxb/eru255
Jung, W.-J., Mabood, F., Souleimanov, A., and Smith, D. L. (2011). Induction of defense-related enzymes in soybean leaves by class IId bacteriocins (thuricin 17 and bacthuricin F4) purified from Bacillus strains. Microbiol. Res. 167, 14–19. doi: 10.1016/j.micres.2011.02.004
Khalilzadeh, R., Seyed Sharifi, R., and Jalilian, J. (2018). Growth, physiological status, and yield of salt-stressed wheat (Triticum aestivum L.) plants affected by biofertilizer and cycocel applications. Arid Land Res. Manag. 32, 71–90. doi: 10.1080/15324982.2017.1378282
Khan, N., Bano, A., Rahman, M. A., Guo, J., Kang, Z., and Babar, M. (2019). Comparative physiological and metabolic analysis reveals a complex mechanism involved in drought tolerance in chickpea (Cicer arietinum L.) induced by PGPR and PGRS. Sci. Rep. 9, 119. doi: 10.1038/s41598-019-38702-8
Lal, M. K., Tiwari, R. K., Gahlaut, V., Mangal, V., Kumar, A., Singh, M. P., et al. (2021). Physiological and molecular insights on wheat responses to heat stress. Plant Cell Rep. 41, 501–518. doi: 10.1007/s00299-021-02784-4
Lamaoui, M., Jemo, M., Datla, R., and Bekkaoui, F. (2018). Heat and drought stresses in crops and approaches for their mitigation. Front. Chem. 6, 26. doi: 10.3389/fchem.2018.00026
Lee, K. D., Gray, E. J., Mabood, F., Jung, W.-J., Charles, T., Clark, S. R., et al. (2009). The class IId bacteriocin thuricin-17 increases plant growth. J. Planta 229, 747–755. doi: 10.1007/s00425-008-0870-6
Lin, Y., Watts, D. B., Kloepper, J. W., Feng, Y., and Torbert, H. A. (2020). Influence of plant growth-promoting rhizobacteria on corn growth under drought stress. Commun. Soil Sci. Plant Anal. 51, 250–264. doi: 10.1080/00103624.2019.1705329
Matse, D. T., Huang, C.-H., Huang, Y.-M., and Yen, M.-Y. (2020). Effects of coinoculation of Rhizobium with plant growth promoting rhizobacteria on the nitrogen fixation and nutrient uptake of Trifolium repens in low phosphorus soil. J. Plant Nutr. 43, 739–752. doi: 10.1080/01904167.2019.1702205
Mellidou, I., and Karamanoli, K. (2022). Unlocking PGPR-mediated abiotic stress tolerance: what lies beneath. Front. Sustain. Food Syst. 6, 832896. doi: 10.3389/fsufs.2022.832896
Michel, B. E. (1983). Evaluation of the water potentials of solutions of polyethylene glycol 8000 both in the absence and presence of other solutes. Plant physiology, 72, 66–70. doi: 10.1104/pp.72.1.66
Moon, Y. S., and Ali, S. (2022). Possible mechanisms for the equilibrium of ACC and role of ACC deaminase-producing bacteria. Appl. Microbiol. Biotechnol. 106, 877–87. doi: 10.1007/s00253-022-11772-x
Naamala, J., Msimbira, L. A., Subramanian, S., and Smith, D. L. (2022). Lactobacillus helveticus EL2006H cell-free supernatant enhances growth variables in Zea mays (maize), Glycine max L. Merill (soybean) and Solanum tuberosum (potato) exposed to NaCl stress. Front. Microbiol. 13, 1075633. doi: 10.3389/fmicb.2022.1075633
Nadeem, M., Li, J., Wang, M., Shah, L., Lu, S., Wang, X., et al. (2018). Unraveling field crops sensitivity to heat stress: mechanisms, approaches, and future prospects. J. Agronomy 8, 128. doi: 10.3390/agronomy8070128
Nazari, M., and Smith, D. L. (2020). A PGPR-produced bacteriocin for sustainable agriculture: a review of thuricin 17 characteristics and applications. Front. Plant Sci. 11, 916. doi: 10.3389/fpls.2020.00916
Nazari, M., Yaghoubian, I., and Smith, D. L. (2022). The stimulatory effect of Thuricin 17, a PGPR-produced bacteriocin, on canola (Brassica, napus L.) germination and vegetative growth under stressful temperatures. Front. Plant Sci. 13, 1079180. doi: 10.3389/fpls.2022.1079180
Neshat, M., Abbasi, A., Hosseinzadeh, A., Sarikhani, M. R., Dadashi Chavan, D., Rasoulnia, A., et al. (2022). Plant growth promoting bacteria (PGPR) induce antioxidant tolerance against salinity stress through biochemical and physiological mechanisms. Physiol. Mol. Biol. Plants 28, 347–361. doi: 10.1007/s12298-022-01128-0
Ober, E. S., and Sharp, R. E. (2003). Electrophysiological responses of maize roots to low water potentials: relationship to growth and aba accumulation. J. Exp. Bot. 54, 813–824. doi: 10.1093/jxb/erg060
Parwez, R., Aftab, T., Gill, S. S., and Naeem, M. (2022). Abscisic acid signaling and crosstalk with phytohormones in regulation of environmental stress responses. Environ. Exp. Bot. 199, 104885. doi: 10.1016/j.envexpbot.2022.104885
Prudent, M., Salon, C., Souleimanov, A., Emery, R., and Smith, D. L. (2015). Soybean is less impacted by water stress using Bradyrhizobium japonicum and thuricin-17 from Bacillus thuringiensis. Agron. Sustain. Dev. 35, 749–757. doi: 10.1007/s13593-014-0256-z
Sarkar, J., Chakraborty, B., and Chakraborty, U. (2018). Plant growth promoting rhizobacteria protect wheat plants against temperature stress through antioxidant signalling and reducing chloroplast and membrane injury. J. Plant Growth Regul. 37, 1396–1412. doi: 10.1007/s00344-018-9789-8
Sati, D., Pande, V., Pandey, S. C., and Samant, M. (2022). Recent advances in PGPR and molecular mechanisms involved in drought stress resistance. J. Soil Sci. Plant Nutr. 23, 106–124. doi: 10.1007/s42729-021-00724-5
Schwinghamer, T., Souleimanov, A., Dutilleul, P., and Smith, D. (2015). The plant growth regulator lipo-chitooligosaccharide (lco) enhances the germination of canola (Brassica napus [L.]). J. Plant Growth Regul. 34, 183–195. doi: 10.1007/s00344-014-9456-7
Schwinghamer, T., Souleimanov, A., Dutilleul, P., and Smith, D. L. (2016). The response of canola cultivars to lipo-chitooligosaccharide (Nod Bj V [C18, 1. MeFuc]) and thuricin 17. Plant Growth Regul. 78, 421–434. doi: 10.1007/s10725-015-0104-4
Shah, A., Nazari, M., Antar, M., Msimbira, L. A., Naamala, J., Lyu, D., et al. (2021). PGPR in agriculture: a sustainable approach to increasing climate change resilience. Front. Sustai. Food Syst. 211, 667546. doi: 10.3389/fsufs.2021.667546
Subramanian, S. (2014). Mass Spectometry Based Proteome Profiling to Understand the Effects of Lipo-chito-oligasaccharide and Thuricin 17 in “Arabldopsis thaliana” and “Glycine max” Under Salt Stress [PhD, McGill]. Mnotreal, QC.
Subramanian, S., Ricci, E., Souleimanov, A., and Smith, D. L. (2016). A proteomic approach to lipo-chitooligosaccharide and thuricin 17 effects on soybean germinationunstressed and salt stress. PLoS ONE 11, e0160660. doi: 10.1371/journal.pone.0160660
Suryadi, Y., Susilowati, D. N., and Fauziah, F. (2019). “Management of plant diseases by PGPR-mediated induced resistance with special reference to tea and rice crops,” in Plant Growth Promoting Rhizobacteria for Sustainable Stress Management, ed R. Z. Sayyed (New York, NY: Springer), 65–110 doi: 10.1007/978-981-13-6986-5_4
Tang, A., Haruna, A. O., Majid, N. M. A., and Jalloh, M. B. (2020). Potential PGPR properties of cellulolytic, nitrogen-fixing, phosphate-solubilizing bacteria in rehabilitated tropical forest soil. J. Microorganisms 8, 442. doi: 10.3390/microorganisms8030442
Turan, M., Arjumend, T., Argın, S., Yıldırım, E., Katırcıoğlu, H., Gürkan, B., et al. (2021). Plant root enhancement by plant growth promoting rhizobacteria. Plant Roots. doi: 10.5772/intechopen.99890
Wang, G. -P., Hui, Z., Li, F., Zhao, M. -R., Zhang, J., and Wang, W. (2010). Improvement of heat and drought photosynthetic tolerance in wheat by overaccumulation of glycinebetaine. Plant Biotechnol. Rep. 4, 213222. doi: 10.1007/s11816-010-0139-y
Wang, Q.-L., Chen, J.-H., He, N.-Y., and Guo, F.-Q. (2018). Metabolic reprogramming in chloroplasts under heat stress in plants. Int. J. Mol. Sci. 19, 849. doi: 10.3390/ijms19030849
Xia, Y., Farooq, M. A., Javed, M. T., Kamran, M. A., Mukhtar, T., Ali, J., et al. (2020). Multi-stress tolerant PGPR Bacillus xiamenensis PM14 activating sugarcane (Saccharum officinarum L.) red rot disease resistance. Plant Physiol. Biochem. 151, 640–649. doi: 10.1016/j.plaphy.2020.04.016
Yakhin, O. I., Lubyanov, A. A., Yakhin, I. A., and Brown, P. H. (2017). Biostimulants in plant science: a global perspective. Front. Plant Sci. 7, 2049. doi: 10.3389/fpls.2016.02049
Zhanassova, K., Kurmanbayeva, A., Gadilgereyeva, B., Yermukhambetova, R., Iksat, N., Amanbayeva, U., et al. (2021). ROS status and antioxidant enzyme activities in response to combined temperature and drought stresses in barley. Acta Physiol. Plant 43, 1–12. doi: 10.1007/s11738-021-03281-7
Zhou, C., Ma, Z., Zhu, L., Xiao, X., Xie, Y., Zhu, J., et al. (2016). Rhizobacterial strain Bacillus megaterium BOFC15 induces cellular polyamine changes that improve plant growth and drought resistance. Int. J. Mol. Sci. 17, 976. doi: 10.3390/ijms17060976
Zhou, R., Kong, L., Yu, X., Ottosen, C.-O., Zhao, T., Jiang, F., et al. (2019). Oxidative damage and antioxidant mechanism in tomatoes responding to drought and heat stress. Acta Physiol. Plant 41, 1–11. doi: 10.1007/s11738-019-2805-1
Keywords: microbial compounds, bacteriocins, canola, stressful conditions, biostimulants
Citation: Nazari M and Smith DL (2023) Mitigation of drought or a combination of heat and drought stress effects on canola by Thuricin 17, a PGPR-produced compound. Front. Sustain. Food Syst. 7:1237206. doi: 10.3389/fsufs.2023.1237206
Received: 09 June 2023; Accepted: 28 August 2023;
Published: 02 October 2023.
Edited by:
Marouane Baslam, Niigata University, JapanReviewed by:
Mohamed Anli, Centre Universitaire de Patsy, ComorosCopyright © 2023 Nazari and Smith. This is an open-access article distributed under the terms of the Creative Commons Attribution License (CC BY). The use, distribution or reproduction in other forums is permitted, provided the original author(s) and the copyright owner(s) are credited and that the original publication in this journal is cited, in accordance with accepted academic practice. No use, distribution or reproduction is permitted which does not comply with these terms.
*Correspondence: Donald L. Smith, ZG9uYWxkLnNtaXRoQG1jZ2lsbC5jYQ==
Disclaimer: All claims expressed in this article are solely those of the authors and do not necessarily represent those of their affiliated organizations, or those of the publisher, the editors and the reviewers. Any product that may be evaluated in this article or claim that may be made by its manufacturer is not guaranteed or endorsed by the publisher.
Research integrity at Frontiers
Learn more about the work of our research integrity team to safeguard the quality of each article we publish.