- 1Bidhan Chandra Krishi Viswavidyalaya, Mohanpur, India
- 2Institute of Agricultural Sciences, Siksha ‘O’ Anusandhan (Deemed to be University), Bhubaneswar, India
Changes in land use practices may affect the distribution of soil sulfur (S) fractions and their bioavailability. Therefore, this study was undertaken to assess the influence of different land use changes on the distribution of soil S fractions and their bioavailability for plant nutrition. Soil samples from farmers’ fields with different land use practices such as rice-mustard-jute (R-M-J), rice-lentil-jute (R-L-J), rice-lentil-sesame (R-L-S), rice-vegetable-jute (R-V-J), and rice-potato-green gram (R-P-G) were collected and analyzed for different fractions of S. The bioavailability of S was assessed by extracting the soil with six different extractants (acidic, neutral, and alkaline) with different extraction modes and chemistry. The results showed that changes in land use practices could influence the distribution of soil S fractions and their bioavailability. Organic S was the dominant fraction, accounting for 93.5% of total S across land use practices. The inorganic S fraction (water-soluble, sorbed, and occluded) varied significantly among the land use practices. Among the inorganic fractions, the water-soluble fraction was the dominant fraction across the land use practices. The bioavailability of S, as assessed by different chemical extractants, was in the following order: sodium hydrogen carbonate (NaHCO3) > mehlich-3 > ammonium bicarbonate-diethylenetriamine penta-acetic acid (AB-DTPA) > ammonium acetate-acetic acid (NH4Ac-HOAc) > calcium dihydrogen phosphate {Ca(H2PO4)2} > calcium chloride (CaCl2). By establishing relationships between extractable S and soil S fractions, it was observed that all the extractants could obtain S from the water-soluble, sorbed, and organic S fractions, with little extractability from the occluded fraction. Among the extractants tested, mehlich-3 extracted a similar amount of S corresponding to the inorganic fraction across the land use practices. It also maintained positive relationships with different fractions of S, and as a multi-nutrient extractant, its use in routine soil testing can be recommended.
1. Introduction
Sulfur (S) is a secondary nutrient element required for the growth and development of plants. As an important constituent of different biomolecules and enzyme systems, it plays an important role in crop production (Solomon et al., 2001). Although the requirement for S is crop-specific, oilseed crops require a higher amount of S for the synthesis of glucosinolates and oils (Padhan, 2016). Therefore, an inadequate supply of S during the crop growth period affects crop production and product quality. In recent years, soil S deficiency has been found in many parts of the world. The widespread S deficiency in soils may be due to the use of chemical fertilizers with high analysis but low sulfur content (Balik et al., 2009; Eriksen, 2009; Scherer, 2009), the restricted use of organic manures, which are a major source of S for plant nutrition (Reddy et al., 2002), and the introduction of high-yielding cultivars that remove higher amounts of S compared to traditional varieties (Kundu et al., 2020). It has been reported that approximately 58.6% of Indian soils are deficient in S (Shukla et al., 2021). Such S deficiency in soils causes significant yield reduction, and it is reported to be 50% in cereals (Zhao et al., 2001). Nearly 1.26 million tons of S are removed by different cropping systems, while only about 0.76 million tons are replenished by fertilizers (Tiwari and Gupta, 2006). Moreover, the use efficiency of the applied S is only 8–10% (Hegde and Murthy, 2005) in different crops, while it is 18% in cereals (Aula et al., 2019), which needs to be improved considering the escalating cost of S fertilizers and other environmental issues. The application of S to cereal-based cropping systems has been shown to improve yield and nutritional quality (Zhao et al., 2001). Cereal crops responded to different levels of S fertilization, and grain yield increased with increased S fertilization (Ying-Xin et al., 2017). However, contrary to this finding, Dhillon et al. (2019) observed a non-significant crop response to S fertilization, except for adequate S supply to the crop due to the mineralization of soil organic matter.
Sulfur fractions in soils are highly dynamic (Dutta et al., 2013). Soil S primarily occurs in organic and inorganic fractions, with the organic fraction being dominant and accounting for more than 90% of total S in agricultural soils (Wang et al., 2006; Sharma et al., 2014; Padhan et al., 2023). Plants uptake sulfate (SO42−)-S for their nutrition, which is supplied from the native soil reserves in addition to external fertilizer inputs. However, the availability of SO42−-S for plant uptake is governed by several soil properties. The presence of iron and aluminum oxides and/or soils with high clay content adsorb the SO42− ions through specific adsorption mechanisms that influence their availability to plants (Padhan, 2018; Das et al., 2020). Interestingly, this mechanism is more pronounced in soils with a pH below 6.5 (Williams and Steinbergs, 1962). A similar one operates when the soil is rich in CaCO3, which could bind the SO42− as occluded S (Hu et al., 2005). Murthy et al. (2002) reported that changes in land use practices could affect the distribution and availability of soil nutrients by altering soil properties and influencing biological transformations in the rooting zone. Soil S fractions and their bioavailability varied under different land uses, for example, in maize-wheat (Gourav et al., 2018), rice-wheat (Sharma et al., 2014; Meena et al., 2022), and finger millet-maize (Lavanya et al., 2019). The mineralization of organic S to an inorganic fraction or bioavailable form varies with changes in land use and types of crop species within the land use (Padhan et al., 2016). Suri et al. (2021) studied the relationship between the different fractions of soil S and their bioavailability under various land uses, viz., maize-wheat, paddy-wheat, vegetable-based cropping sequence, sugarcane-based cropping sequence, and orchard; the occurrence of S fractions followed the order of organic S > non-sulfate S > available S > exchangeable S > water-soluble S. Land use change has a strong influence on carbon storage by controlling the amount and quality of litter addition, decomposition rate, stabilization of soil organic carbon, etc. (Gelaw et al., 2014; Huang et al., 2018), which in turn influences the nutrient dynamics in soil. As the lion’s share of total soil S is associated with the organically bound form, bioavailability is influenced by land use management (Padhan et al., 2016). Information on the influence of land use changes on soil S fractions, extractable S, and their bioavailability for crop nutrition is scarce. We hypothesized that changes in land use could alter the distribution of different fractions of soil S by changing the proportion of inorganic and organic S fractions, further influencing its bioavailability. Thus, the present study was designed to ascertain the influence of land use changes on soil S fractions and their bioavailability for crop nutrition.
2. Materials and methods
Surface soil samples (0–15 cm) were collected from farmers’ fields in Nadia district of West Bengal with five different land uses, viz., rice-lentil-jute (R-L-J), rice-lentil-sesame (R-L-S), rice-mustard-jute (R-M-J), rice-vegetable-jute (R-V-J), and rice-potato-green gram (R-P-G), which were more than 10 years old. In total, 90 geo-referenced soil samples were collected after the rice crop was harvested using a simple random technique. The sampling site falls under the agroecological sub-region 15.1 of India and is characterized by a tropical, moist, sub-humid climate. It receives an annual rainfall of ~1,400 mm, and more than 70% of the rainfall occurs from June to September. In winter, the minimum temperature goes down to 8°C, while in summer, the maximum temperature goes up to 41°C. Different fertilizer schedules and doses are followed for all land uses. On average, R-L-J systems receive N:P:K at a rate of 94:60:80 kg/ha annually, while the R-L-S, R-M-J, R-V-J, and R-P-G systems receive 135:89:85, 175:120:125, 249:210:200 and 250:260:220 kg/ha/ year, respectively. For lentils and green grams, no fertilizers are used. Canal and/or groundwater sources are used for the irrigation of rice, jute, vegetables, and potatoes, while lentils and green grams are cultivated with residual soil moisture. The collected samples were air-dried, ground, sieved with a 2.0 mm sieve, and stored in moisture-proof bags for further analysis of soil properties. Different chemical properties and available nutrients in soils of all land uses were analyzed following standard protocols as outlined in Table 1.
2.1. Sequential fractionation of sulfur in soils
Fine soils (<2 mm) were used for the analysis of S fractions as per the method outlined by Morche (2008). The first step in the sequential fractionation was the extraction of the soil sample with demineralized water at 1:10 (w/v). After 30 min of shaking in a rotary shaker, the samples were centrifuged at 10,000 rpm for 10 min. In the second step, the samples were extracted with 0.032 M NaH2PO4 at a 1:10 (w/v) ratio by shaking for 30 min followed by centrifugation at 10,000 rpm for 10 min. The residual soil sample was extracted with 1 M HCl, maintaining the extraction ratio of 1:20 (w/v). After shaking for 60 min, the samples were centrifuged for 10 min at 10,000 rpm. The extracted S fractions were measured by following the method of Chesnin and Yien (1950). The sum of all three fractions was considered the total inorganic S fraction of the soil. The total S of soil samples was analyzed using the CHNS VarioEL cube analyzer (Elementar, Germany). Organic S was calculated as the difference between the total and inorganic S of soil samples. The extractable S from soils of all land uses was extracted with six different chemical extractants (two with a neutral solution pH, two with an acidic solution pH, and two with a slightly alkaline to alkaline solution pH) having different extraction modes and chemistry (Table 2).
Schematic representation of the sequential fractionation of sulfur in soil (Morche, 2008).
2.2. Statistical analysis
Statistical analysis was performed using the Windows-based SPSS program (ver. 22.0, SPSS Inc./IBM, Armonk, United States). Duncan’s Multiple Range Test (DMRT) was used to compare means. Pearson correlation was performed between S fractions and extractable S and between soil properties to establish their relationships. A multiple linear regression equation was also computed with extractable S as the dependent variable and the main soil properties as the independent variables.
3. Results
3.1. Influence of land use on soil properties and nutrient availability
Changes in land use significantly influenced soil properties and nutrient availability (Table 3). The soil pH, measured with a 0.01 M CaCl2 solution, ranged from 7.18 to 7.54. The highest soil pH was observed in the R-M-J system and the lowest in the R-P-G system. Soil organic carbon content (g kg−1) varied between 6.68 and 7.36 across different land use systems. Compared with the R-V-J system, the R-L-S, R-M-J, and R-L-J systems showed an increase in SOC content of 6.4, 8.2, and 10.2%, respectively. Calcium carbonate content showed an increasing trend with rising soil pH, while oxides of Fe, Al, and Mn decreased with increasing pH (Table 3; Supplementary Figure S1). The iron oxide content (g kg−1) ranged from 0.800 (R-M-J) to 1.063 (R-P-G). Similarly, Alo and Mno contents (g kg−1) ranged from 0.503 to 0.562 and from 0.342 to 0.408, respectively. Soils under all land uses had medium levels of available nitrogen content except for the R-M-J system, while they had high levels of available phosphorus and potassium. The lowest available N was found in the R-M-J system, while the highest available N was reported in the R-P-G system. However, the availability of cationic micronutrients (iron, manganese, copper, and zinc) was significantly higher in the R-L-J system compared to other land uses (Table 4).
3.2. Total and organic S fractions in soils under different land use practices
The data presented in Table 5 show that soil organic and total S contents varied significantly among the land uses. The highest organic and total S concentrations (249.1 and 266.4 mg kg−1, respectively) were observed in the R-P-G system, while the lowest (209.5 and 225.3 mg kg−1, respectively) were observed in the R-L-S system. Compared to the R-L-S system, the R-V-J and R-P-G systems showed an increase in total S content to the magnitude of 11.1 and 18.2%, respectively. The organic S followed a similar pattern of occurrence as the total S content in all land uses.
3.3. Inorganic soil S fractions under different land use practices
A significantly higher inorganic S fraction was observed in R-P-G land use, while the lowest amount was found in the R-M-J system. The water-soluble, sorbed, and occluded S fractions together constituted the inorganic fraction. Across all land uses, the inorganic S fraction constituted 6.5% of total soil S. The water-soluble S fraction was lowest in the R-M-J system (7.1 mg kg−1) and highest in the R-P-G system (11.2 mg kg−1). Similarly, the sorbed S fraction ranged from 1.0 to 2.0, being lowest in the R-M-J system and highest in the R-P-G system. On the other hand, R-P-G land use witnessed the lowest amount of occluded S fraction, while R-M-J land use showed the highest value. Across the land uses, inorganic S fractions were found in the order of water-soluble>occluded>sorbed S.
3.4. Extractable S in soil under different land use practices
Land use change significantly influenced the extractability of all six extractants (Table 6). The CaCl2 extractable S ranged from 8.4 mg kg−1 in the R-M-J system to 12.6 mg kg−1 in the R-P-G system. Irrespective of the extractants used, the lowest amount of extractable S was observed in the R-M-J system, while the highest amount was observed in the R-P-G system. Interestingly, the mehlich-3 and NaHCO3 extractants obtained on average 1.7 and 2.8 times more S than those extracted with CaCl2 solutions, respectively. Across all land uses, the order of extractability was: NaHCO3 > mehlich-3 > AB-DTPA > NH4Ac-HOAc > Ca(H2PO4)2 > CaCl2.
4. Discussion
4.1. Influence of land use changes on soil properties
Changes in land use significantly influence soil properties and nutrient availability. The low pH value in the R-P-G system could be due to the higher content of amorphous Feo and Alo. The presence of Feo and Alo generally lowers the soil pH due to their oxidation, which releases H+ ions into the soil system (Yang et al., 1997). Furthermore, the high quantity of chemical fertilizers applied in the R-P-G system compared to other land uses might be the reason for low soil pH. Higher SOC content in the R-M-J and R-L-J systems may be caused by the slow decomposition of organic matter due to the prevailing anaerobic environment during rice and jute cultivation. Manlay et al. (2002) also reported that anaerobic conditions could slow down the organic matter decomposition rate during rice cultivation. A high calcium carbonate content in the R-M-J system could be associated with the soil pH, which was supported by their positive correlations (S1). Similarly, the decrease of Feo, Alo, and Mno in all land uses with the increase in soil pH can be attributed to their strong negative relationships (S1). The highest amount of available nutrient content, especially N, P, and K, may be due to the high amount of fertilizer applied in the R-P-G system compared to other land uses. The increased availability of cationic micronutrients in R-L-J land use compared to the others may correspond to the increased organic carbon content, which was observed from their significant positive correlations (S1). A reduced soil environment in rice and jute cultivations could have altered the availability of cationic micronutrients.
4.2. Influence of land use on soil S fractions
The highest organic and total S were found in the R-P-G system, which might be due to the lower crop removal compared to oilseed-based land use. The sulfur requirement is high for oilseed crops compared to cereals and other crops, and the inclusion of crops with high S requirement in the land use could deplete the soil S. Meena et al. (2022) reported that the inclusion of pulses in the rice-wheat land use system could influence the availability of S. Shifting the virgin soils to cultivable lands with different agricultural management could change the equilibrium status of soil organic S and affect its distribution in surface layers (Solomon et al., 2003). Organic S accounted for an average of 93.5% of the total S, representing the dominant fraction in the soils. A similar magnitude of the organic S fraction under different land uses has been reported by other researchers (Padhan et al., 2016; Meena et al., 2022). The inorganic S fraction represented the pool of S available for plant uptake. The inorganic S present in soils is readily available (extracted with distilled water or any weak salt solution), adsorbed S (extracted with phosphate-containing solution), and sulfate co-precipitated with CaCO3 or BaCO3 (solubilized and extracted with HCl solution). Therefore, we employed the sequential fractionation procedure as described by Morche (2008), which includes demineralized water, 0.032 M NaH2PO4, and 1 M HCl solution. Among the three fractions, water-soluble S accounted for an average of 58% of the total inorganic S in the soils (Figure 1). This signifies that a higher proportion of inorganic S is readily available for all land uses. On average, the magnitude of water-soluble S was 1.9 times that of occluded and 6.4 times that of sorbed S. Increased amounts of water-soluble S in the R-P-G system compared to other land uses might be due to lower crop removal.
The sorbed S varied among land uses. Among all land uses, the R-P-G system had the highest amount of sorbed S, which suggests that this particular land use had a high amount of inorganic S bound to the colloidal matrix. The presence of high amounts of Feo and Alo in R-P-G could offer a specific adsorption site for SO4= (Singh, 1984; Das et al., 2020). This was confirmed by the positive relationships between the sorbed S and the oxides of Fe and Al (Supplementary Figure S2). A highly sorbed S could also suggest a high amount of bound SO4= in the soil system, as water extracts the readily available SO4= and leaves behind the adsorbed fraction. The low amount of sorbed S in the R-M-J system could be due to the organic anions released from the decomposition of soil organic matter, which could have displaced the adsorbed SO4= in the colloidal matrix of Feo and Alo into the soil solution. Previous research has also shown that SO4= and low-molecular-weight organic anions compete for the same adsorption site (Evans, 1986) and that removal of organic matter can increase the adsorption capacity of soils (Das et al., 2002). Moreover, the organic anions make chelation with oxides of Fe and Al (which preferentially adsorb SO4=) resulting in reduced sorption of SO4=. The magnitude of occluded S was higher than that of sorbed S for all land uses. A significant positive relationship between occluded S and CaCO3 content of soils confirmed that the presence of a high amount of carbonate could adsorb SO4=, rendering it unavailable for plant nutrition (Supplementary Figure S3). It was also observed that, on average, S co-precipitated with carbonate accounted for 32.8% of the total inorganic S. Such co-precipitated S could be released and made available to the plant, which is primarily governed by rhizosphere pH. In addition, nitrogenous fertilizers applied to the soil may have a favorable effect on the solubilization of CaCO3-occluded S, and this solubilized fraction may be partially transformed into a soluble organic form (Hu et al., 2005).
4.3. Extractable S
The CaCl2 solution extracted the lowest amount compared to other extractants across land uses, accounting for 4.2% of total soil S. Suri et al. (2021) reported similar observations in soils from different land use practices. On average, Ca(H2PO4)2, Mehlich-3, NH4Ac-HOAc, AB-DTPA, and NaHCO3 extracted 4.8, 7.3, 4.9, 12.0, and 6.2% of total soil S, respectively. The highest amount of NaHCO3−extractable S across land uses compared to other extractants could be due to the extraction of a portion of the organically-bound S (mostly ester sulfate) due to its high pH besides the readily available S (Kilmer and Nearpass, 1960). This was confirmed by the positive relationship between NaHCO3-extractable S and the organic S content of the soil (computed across different land uses). In addition, the presence of HCO3− in AB-DTPA and 0.5 M NaHCO3 displaced the sorbed S from the colloidal complex (Tabatabai, 1996), while also solubilizing partly the labile insoluble minerals in the soil (Soltanpour, 1985). Acidic extractants, particularly mehlich-3, showed higher extractability compared to neutral solvents {CaCl2 and Ca(H2PO4)2} and slightly alkaline solvents (AB-DTPA) across land uses. Low pH can solubilize the oxides of Fe and Al, thereby releasing the bound sulfate into the soil solution. Moreover, the acetate and nitrate anions in Mehlich-3 favored the extraction of S from the soil (Seth et al., 2018). The lowest amount of S extracted by a 0.15% CaCl2 solution in soils of all land uses could be due to its efficiency in extracting only the readily available or water-soluble S. Hu et al. (2005) reported that Cl−-based extractants can mobilize only the water-soluble SO4= and also have lower extraction power due to their tendency to form slowly soluble CaSO4 (Matula, 1999). Phosphate ions in Ca(H2PO4)2 solution could displace the sorbed S, thereby showing increased extractability compared to CaCl2 solution across land uses.
Among the five land uses, the R-P-G system always had the highest amount of extractable S compared to other land uses (Table 6). Moreover, the influence of the R-P-G system over the R-M-J system was more pronounced when S was extracted with 0.5 M NaHCO3(56.0), followed by CaCl2 (50.0), AB-DTPA (41.4), Ca(H2PO4)2 (40.8), Mehlich-3 (31.2), and NH4Ac-HOAc (30.3; Figure 2). High organic S content in the R-P-G system (Table 5), which increases the availability of S in soils (by mineralizing the labile S), was captured by the extractants used in the present study.
4.4. Extractable S and S fractions
By establishing the relationship between extractable S (neutral extractants) and S fractions across the land uses, it was observed that CaCl2 extractable S was positively correlated with water-soluble S (r = 0.968**), sorbed S (r = 0.950**), and organic S (0.696**), while negatively correlated with occluded S (r = −0.927**; Table 7; Figures 3A–D). A similar relationship was found between Ca(H2PO4)2-extractable S and S fractions. Both extractants are highly correlated with the water-soluble S fraction, which is considered the readily available S in the majority of agricultural soils. Acidic extractants mehlich-3 and NH4Ac-HOAc maintained significant positive correlations with water-soluble, sorbed, organic, and total S fractions while they were negatively correlated with occluded S (Table 7; Figures 4A–D). Interestingly, the NH4Ac-HOAc-extractable S showed a high degree of correlation with organic (r = 0.914**) and total S (0.928**) compared to mehlich-3 indicating that it could extract a higher proportion from the organic S fraction. A similar relationship existed between the S extracted by weakly alkaline to alkaline extractants (NaHCO3 and AB-DTPA) and the S fractions (Table 7; Figures 5A–D). Notably, the inorganic fraction closely corresponds to the amount of S extracted by Mehlich-3 and AB-DTPA. Occluded S was the only fraction of S that maintained a negative correlation with S obtained by the extractants across all land uses. From the relationships established between extractable S and S fractions, it can be inferred that all extractants primarily extracted the water-soluble, sorbed, and partially organic S fractions, with little extractability from the occluded S fraction.
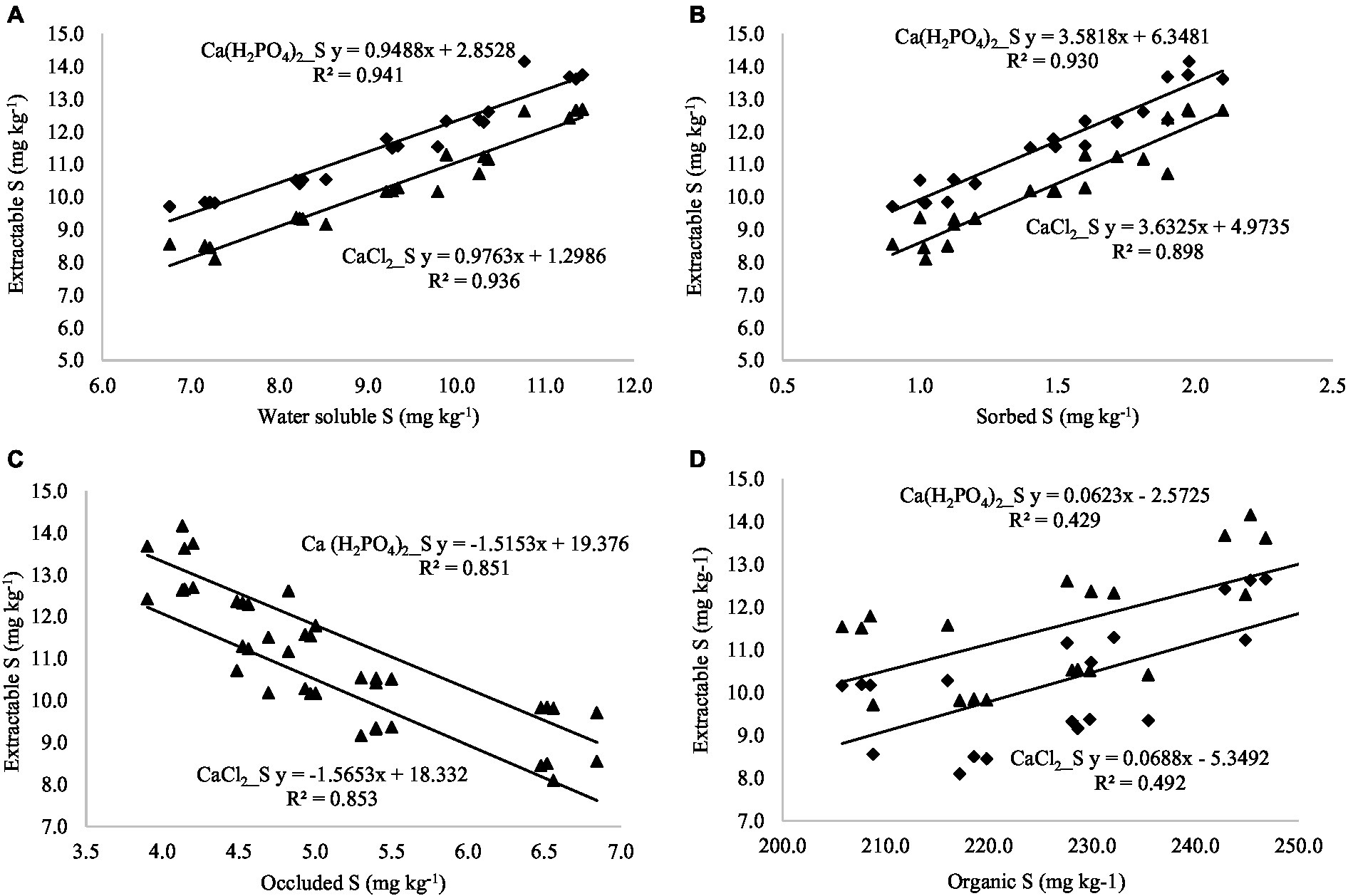
Figure 3. (A–D) Relationship between soil S fractions and extractable S (extracted with neutral pH extractants).
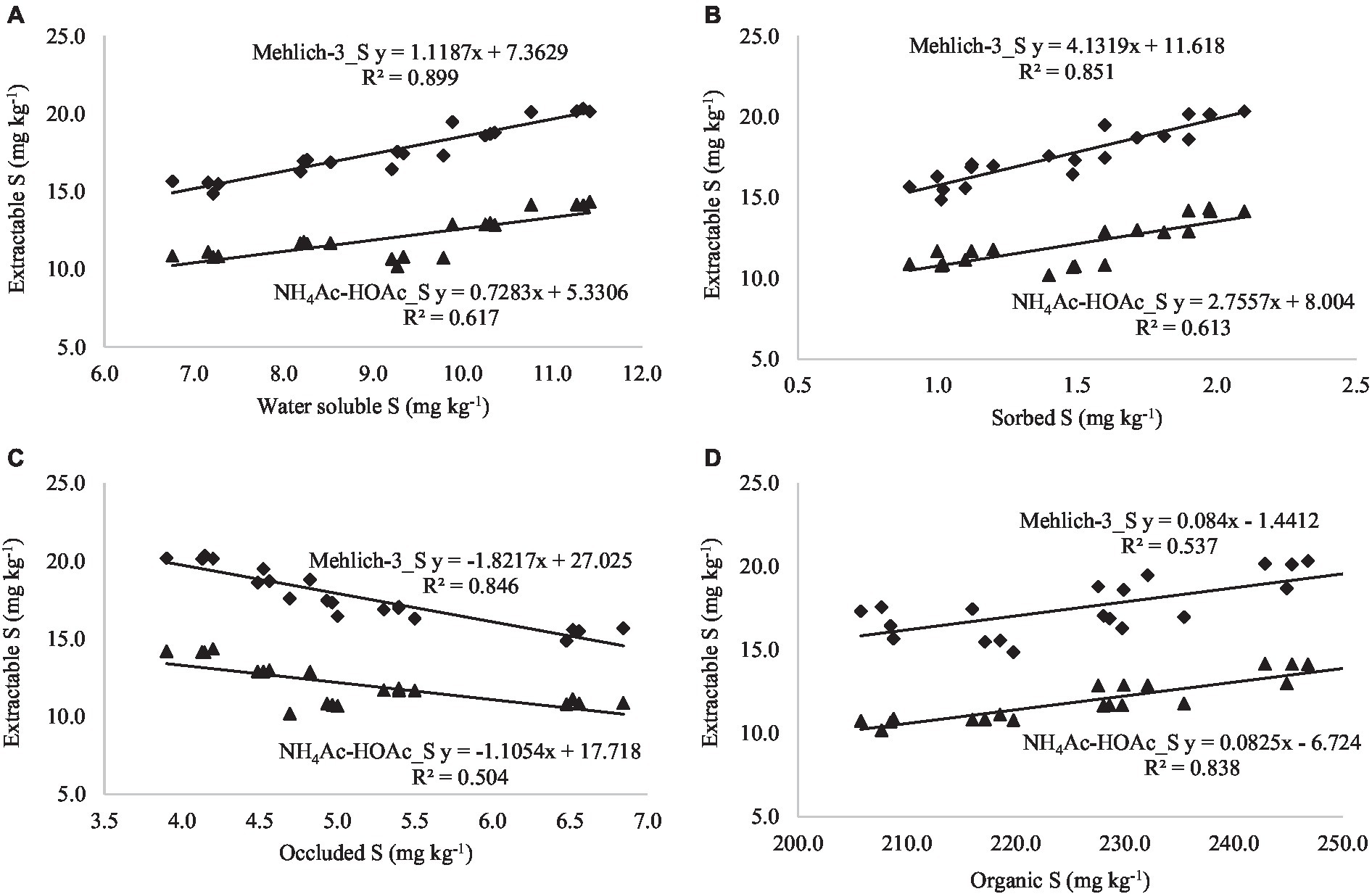
Figure 4. (A–D) Relationship between soil S fractions and extractable S (extracted with acidic pH extractants).
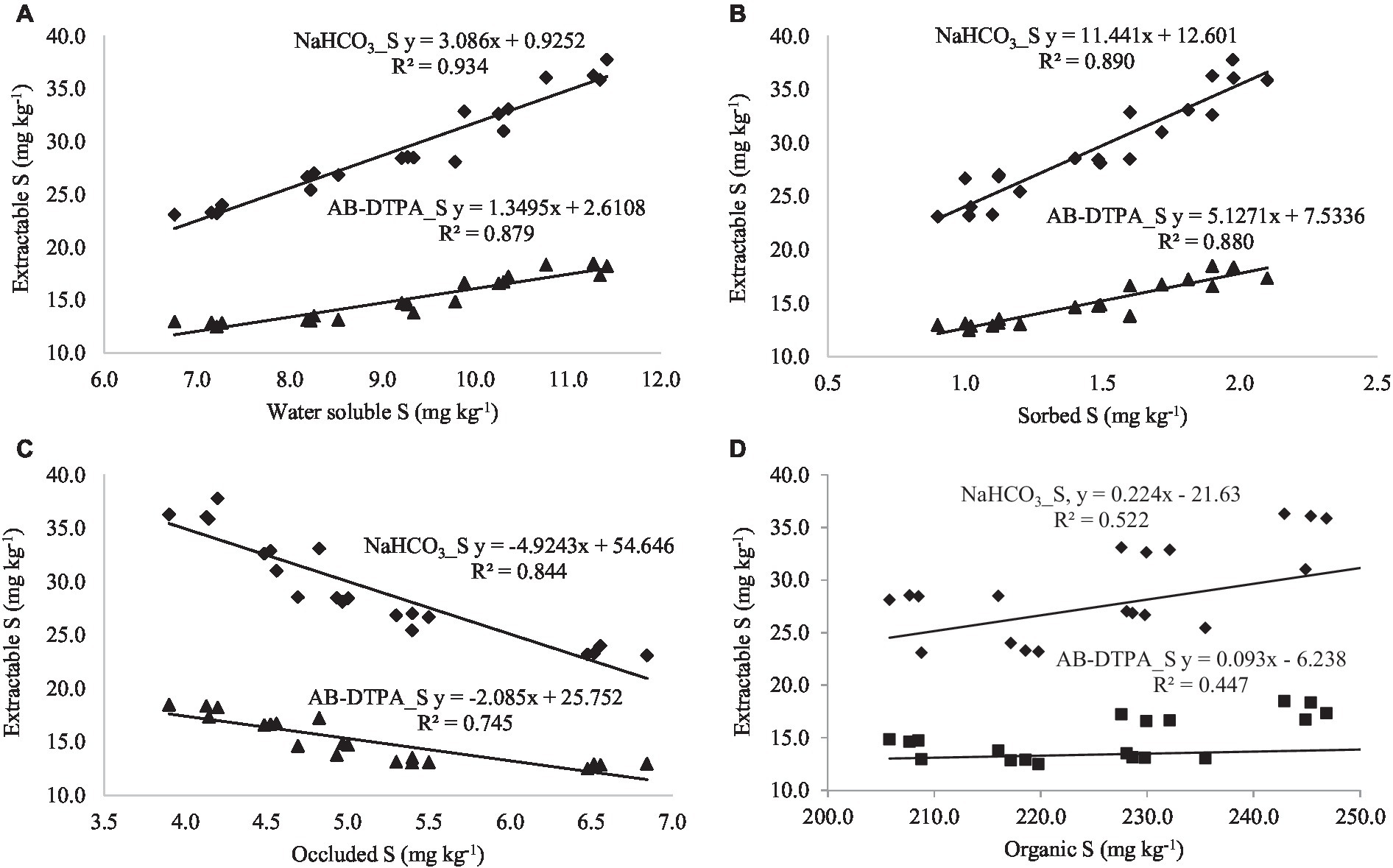
Figure 5. (A–D) Relationship between soil S fractions and extractable S (extracted with slightly alkaline to alkaline pH extractants).
4.5. Comparison of extractants
Acidic extractants, particularly mehlich-3, were able to extract 1.7 and 1.5 times more S than CaCl2 and Ca(H2PO4), respectively. Notably, the NH4Ac-HOAc extracted higher amounts of S than CaCl2 and Ca(H2PO4)2. Across all land uses, the order of extractability was: NaHCO3 > mehlich-3 > AB-DTPA > NH4Ac-HoAc > Ca(H2PO4)2 > CaCl2. A Pearson correlation was constructed among the extractable S to establish their relationships. The CaCl2-extractable S showed a strong positive correlation with Ca(H2PO4)2; r = 0.988**), mehlich-3 (r = 0.967**), NH4Ac-HoAc (r = 0.846**), NaHCO3 (r = 0.980**), and AB-DTPA (r = 0.956**; Table 8). Such dynamic relationships among the extractable S suggested that S could be obtained from similar pools, which was further supported by their relationship with different S fractions. Comparing the procedure involved during the extraction process, it can be noted that the shaking time for mehlich-3 and AB-DTPA was restricted to 5 min and 15 min respectively, and since they are primarily used as multi-nutrient extractants, they can be employed in routine laboratory analysis. However, the unavailability of NH4NO3 (banned in many countries), which is one of the primary chemicals in the mehlich-3 composition, calls into question the use of mehlich-3 in routine laboratory analysis in the future. The presence of Ca in Ca(H2PO4) and CaCl2 extractants flocculates the soil particles, and a clear extract can be obtained, which is highly essential for the turbidimetric determination of S. On the other hand, in the case of NaHCO3, the high pH of the extractant could partially solubilize the organic matrix of the soil, resulting in a turbid solution that needs to be clarified prior to S determination. To test the reproducibility of extractable S by all six extractants, the relative standard deviation (RSD) value was calculated. A high RSD value indicates poor reproducibility of the data, while a low RSD value shows greater reproducibility. Across all land uses, mehlich-3 had the lowest RSD value, while NaHCO3 showed a high RSD value signifying its poor reproducibility.
4.6. Influence of soil properties on the extractability of extractants
The results presented in Table 9 indicate that the extractability of different extractants is influenced by soil properties. In the case of CaCl2-extractable S, Fe oxide alone caused a variation of 93.4%, which was improved to 95.8% with the inclusion of CaCO3. Similarly, the variation in Ca(H2PO4)2-extractable S caused by Fe oxide alone was 91.8%, and it was improved to 94.4 and 95.8% with the inclusion of Fe oxide and Mn oxide and oxides of Fe, Mn, and Al, respectively. Both CaCO3 and Mn oxide could explain 82.8% of the variability in NH4Ac-HOAc-extractable S, and the exclusion of Mn oxide from the regression model decreased the predictability by 8.5%. The amount of S extracted by NaHCO3 was found to be influenced by the oxides of Fe and Al. In general, the oxides of Fe, Al, Mn, and CaCO3 are the main soil constituents that are highly responsible for predicting the variability of extractable S in soil under different land uses.
5. Conclusion
Land use practices significantly influence the distribution of S into different fractions and its bioavailability. Land use practices with high oxides of Fe and Al and CaCO3 content showed a high amount of sorbed and occluded S, respectively. Extractants used to capture the bioavailable S removed S from water-soluble, sorbed, and organic fractions, with little extractability from the occluded fraction. The dynamic relationships among the extractants and between extractable S and S fractions suggest that all extractants are efficient enough to extract the bioavailable S. However, the use of multi-nutrient extractants such as Mehlich-3 can save time and cost and, thus, can be recommended for routine laboratory analysis. Further research must focus on the replacement of NH4NO3 in Mehlich-3 with a suitable chemical compound.
Data availability statement
The original contributions presented in the study are included in the article/Supplementary material, further inquiries can be directed to the corresponding author.
Author contributions
DP: conceptualization, and manuscript preparation and analysis. PR: statistical analysis and manuscript editing. AS: writing review and editing. All authors contributed to the article and approved the submitted version.
Conflict of interest
The authors declare that the research was conducted in the absence of any commercial or financial relationships that could be construed as a potential conflict of interest.
Publisher’s note
All claims expressed in this article are solely those of the authors and do not necessarily represent those of their affiliated organizations, or those of the publisher, the editors and the reviewers. Any product that may be evaluated in this article, or claim that may be made by its manufacturer, is not guaranteed or endorsed by the publisher.
Supplementary material
The Supplementary material for this article can be found online at: https://www.frontiersin.org/articles/10.3389/fsufs.2023.1233223/full#supplementary-material
References
Aula, L., Dhillon, J. S., Omara, P., Wehmeyer, G. B., Freeman, K. W., and Raun, W. R. (2019). World Sulfur use efficiency for cereal crops. Agron. J. 111, 2485–2492. doi: 10.2134/agronj2019.02.0095
Balik, J., Kulhanek, M., Cerny, J., Szakova, J., Pavlikova, D., and Cermak, P. (2009). Differences in soil sulfur fractions due to limitation of atmospheric deposition. Plant soil environ. Plant Soil Environ. 55, 344–352. doi: 10.17221/101/2009-PSE
Bardsley, C. E., and Lancastor, J. D. (1960). Determination of reserve sulphate and soluble sulphate in soils. Proc. Soil Sci. Soc. Am. 24, 265–268. doi: 10.2136/sssaj1960.03615995002400040015x
Chesnin, L., and Yien, C. H. (1950). Turbidimetric determination of available sulfates. Proc. Soil Sci. Soc. Am. 15, 149–151. doi: 10.2136/sssaj1951.036159950015000C0032x
Das, S., Ghosh Bag, A., Ghosh, D., Chatterjee, N., Adhikary, S., Sahu, G., et al. (2020). Extractability of different extractants and availability of Sulphur in long-term rice growing alluvial soils of West Bengal. Int. J. Chem. Stud. 7, 1557–1563.
Das, P. K., Sahu, S. K., and Acharya, N. (2002). Effect of organic matter on sulphate adsorption in some Alfisols of Orissa. J. Indian Soc. Soil Sci. 50, 23–28.
Dhillon, J. S., Dhital, S., Lynch, T., Figueiredo, B., Omara, P., and Raun, W. R. (2019). In-season application of nitrogen and sulfur in winter wheat (Triticum aestivum L.). Agro. Geosci. Env. 2, 1–8. doi: 10.2134/age2018.10.00472(1)
Dutta, J., Sankhyan, N. K., Sharma, S. P., Sharma, G. D., and Sharma, S. K. (2013). Sulphur fractions in acid soil continuously fertilized with chemical fertilizers and amendments under maize-wheat system. J. Indian Soc. Soil Sci. 61, 195–201.
Eriksen, J. (2009). Soil sulfur cycling in temperate agricultural systems. Adv. Agron. Advan. Agronomy. 102, 55–89. doi: 10.1016/S0065-2113(09)01002-5
Evans, A. (1986). Effects of dissolved organic carbon and sulfate on aluminium mobilization in forest soil columns. Soil Sci. Soc. Am. J. 50, 1576–1578. doi: 10.2136/sssaj1986.03615995005000060038x
Fox, R. L., Olson, R. A., and Rhoades, H. F. (1964). Evaluating the sulfur status of soils by plant and soil tests. Proc. Soil Sci. Soc. Am. 28, 243–246. doi: 10.2136/sssaj1964.03615995002800020034x
Gelaw, A. M., Singh, B. R., and Lal, R. (2014). Soil organic carbon and total nitrogen stocks under different land uses in a semi-arid watershed in Tigray, northern Ethiopia. Agric. Ecosyst. Environ. 188, 256–263. doi: 10.1016/j.agee.2014.02.035
Gourav,, Sankhyan, N. K., Sharma, R. P., and Sharma, G. D. (2018). Vertical distribution of Sulfur fractions in a continuously fertilized acid Alfisol under maize–wheat cropping system. Commun. Soil Sci. Plant Anal. 49, 923–933. doi: 10.1080/00103624.2018.1448409
Hegde, D. M., and Murthy, I. Y. L. N. (2005). Management of secondary nutrients-achievements and challenges. Ind. J. Fert. 1, 93–100.
Hu, Z. Y., Zhao, F. J., and McGrath, S. P. (2005). Sulphur fractionation in calcareous soils and bioavailability to plants. Plant Soil 268, 103–109. doi: 10.1007/s11104-004-0229-0
Huang, X., Zhou, Y., and Zhang, Z. (2018). Carbon sequestration anticipation response to land use change in a mountainous karst basin in China. J. Environ. Manag. 228, 40–46. doi: 10.1016/j.jenvman.2018.09.017
Kilmer, V. J., and Nearpass, D. C. (1960). The determination of available Sulphur in soils. Proc. Soil Sci. Soc. Am. 24, 337–340. doi: 10.2136/sssaj1960.03615995002400050011x
Kundu, R., Adhikary, S., Padhan, D., Das, A., and Dutta, J. (2020). Extractable fractions of Sulphur in major soils of India. Chem. Sci. Rev. Lett. 9, 138–145. doi: 10.37273/chesci.CS2051017
Lavanya, K. R., Kadalli, G. G., Patil, S., Jayanthi, T., Naveen, D. V., and Channabasavegowda, R. (2019). Sulphur fractionation studies in soils of long term fertilizer experiment under finger millet – maize cropping sequence. Int. J. Curr. Microbiol. App. Sci. 8, 1334–1345. doi: 10.20546/ijcmas.2019.809.153
Lindsay, W. L., and Norvell, W. A. (1978). Development of a DTPA soil test for zinc, iron, manganese and copper. Soil Sci. Soc. Am. J. 42, 421–428. doi: 10.2136/sssaj1978.03615995004200030009x
Manlay, R. J., Chotte, J., Masse, D., Laurent, J., and Feller, C. (2002). Carbon, nitrogen and phosphorus allocation in agroecosystems of a west African savanna. III. Plant and soil components under continuous cultivation. Agric. Ecosyst. Environ. 88, 249–269. doi: 10.1016/S0167-8809(01)00220-1
Matula, J. (1999). Use of multinutrient soil tests for Sulphur determination. Commun. Soil Sci. Plant Anal. 30, 1733–1746. doi: 10.1080/00103629909370326
McKeague, I. A., and Day, J. H. (1966). Dithionite and oxalate extractable Fe and Al as aid in differentiating various classes of soils. Can. J. Soil Sci. 46, 13–22. doi: 10.4141/cjss66-003
Meena, S. K., Dwivedi, B. S., Meena, M. C., Datta, S. P., Singh, V. K., Mishra, R. P., et al. (2022). Long-term nutrient management in an intensive rice-wheat cropping system improves the quantities, qualities, and availability of soil sulfur. Front. Sustain. Food Syst. 6:997269. doi: 10.3389/fsufs.2022.997269
Mehlich, A. (1984). Mehlich-3 for soil test extractant: a modification of Mehlich-2 extractant. Commun. Soil Sci. Plant Anal. 15, 1409–1416. doi: 10.1080/00103628409367568
Morche, L. (2008). S-fluxes and spatial alterations of inorganic and organic Sulphur fractions in soil as well as their accumulation and depletion in the rhizosphere of agricultural crops by partial use of the radioisotope 35S. [Ph.D. Thesis.] Bonn, University of Bonn, 322. (In German).
Murthy, D., Kirschbaum, M. U. F., McMurtrie, R. E., and McGilvray, H. (2002). Does conversion of forest to agricultural land change soil carbon and nitrogen? A review of the literature. Glob. Chang. Biol. 8, 105–123. doi: 10.1046/j.1354-1013.2001.00459.x
Olsen, S.R., Cole, C.V., Watanabe, F.S., and Dean, A.L. (1954). Estimation of available phosphorus in soils by extraction with sodium bicarbonate, USDA circular 939, US Govt. Printing Office, Washington, DC.
Padhan, D. (2016). Response of oilseed crops to Sulphur fertilization-a relook. Progres. Res. Int. J. 11, 1376–1381.
Padhan, D. (2018). The locking and unlocking of nutrients in soils for plants under organic farming system. Ph. D thesis submitted to Bidhan Chandra Krishi Vuswavidyalaya, Mohanpur, India, pp. 1–323.
Padhan, D., Pradhan, A. K., Chakraborty, M., and Sen, A. (2016). Assessment of the effects of land use pattern on distribution of Sulphur fractions in soil. J. Appl. Nat. Sci. 8, 1685–1691. doi: 10.31018/jans.v8i3.1023
Padhan, D., Shivaraj, D., Doddagenigera Nagaraja, A., Rout, P. P., Babu, C. M., Aurade, R., et al. (2023). Changes in soil sulphur fractions as influenced by nutrient management practices in mulberry. Land. 12:1160. doi: 10.3390/land12061160
Page, A.L., Miller, R.H., and Keeney, D.R. (Eds) (1982). Methods of soil analysis, Part-2, 2, SSSAJ Madison, Wisconsin.
Reddy, K. S., Singh, M., Swarup, A., Rao, A. S., and Singh, K. N. (2002). Sulfur mineralization in two soils amended with organic manures, crop residues, and green manures. J. Plant Nutr. Soil Sci. 165, 167–171. doi: 10.1002/1522-2624(200204)165:2<167::AID-JPLN167>3.0.CO;2-1
Scherer, H. W. (2009). Sulfur in soils. J. Plant Nutr. Soil Sci. 172, 326–335. doi: 10.1002/jpln.200900037
Seth, A., Sarkar, D., Masto, R. E., Batabyal, K., Saha, S., Murmu, S., et al. (2018). Critical limits of Mehlich 3 extractable phosphorous, potassium, sulfur, boron and zinc in soils for nutrition of rice (Oryza sativa L.). J. Soil Sci. Plant Nutr. 18, 512–523. doi: 10.4067/S0718-95162018005001601
Sharma, U., Subehia, S. K., Rana, S. S., Sharma, S. K., and Negi, S. C. (2014). Soil Sulphur fractions and their relationship with soil properties and rice (Oryza sativa L.) yield under long-term integrated nutrient management in an acid Alfisol. Res. On Crops. 15, 738–745. doi: 10.5958/2348-7542.2014.01406.5
Shukla, A. K., Behera, S. K., Prakash, C., Tripathi, A., Patra, A. K., Dwivedi, B. S., et al. (2021). Deficiency of phyto-available Sulphur, zinc, boron, iron, copper and manganese in soils of India. Sci. Rep. 11:19760. doi: 10.1038/s41598-021-99040-2
Singh, B. R. (1984). Sulfate sorption by acid forest soils: 2. Sulfate adsorption isotherms with and without organic matter and oxides of aluminium and iron. Soil Sci. 138, 294–297. doi: 10.1097/00010694-198410000-00006
Solomon, D., Lehmann, J., and Martinez, C. E. (2003). Sulfur K-edge XANES spectroscopy as a tool for understanding sulfur dynamics in soil organic matter. Soil Sci. Soc. Am. J. Soil Sci. Society. America J. 67, 1721–1731. doi: 10.2136/sssaj2003.1721
Solomon, D., Lehmann, J., Tekalign, M., Fritzsche, F., and Zech, W. (2001). Sulfur fractions in particle-size separates of the subhumid Ethiopian highlands as influenced by land use changes. Geoderma 102, 41–59. doi: 10.1016/S0016-7061(00)00103-8
Soltanpour, P. N., and Schwab, A. P. (1977). A new soil test for simultaneous extraction of macro- and micro-nutrients in alkaline soils. Commun. Soil Sci. Plant Anal. 8, 195–207. doi: 10.1080/00103627709366714
Soltanpour, P. N. (1985). Use of ammonium bicarbonate DTPA soil test to evaluate elemental availability and toxicity. Commun. Soil Sci. Plant Anal. 16, 323–338.
Subbiah, B. V., and Asija, G. L. (1956). A rapid procedure for the determination of available nitrogen in soils. Curr. Sci. 25, 259–260.
Suri, D., Sharma, V. K., Kumar, P., Upadhayay, R. G., and Nazir, G. A. A. (2021). Sulphur dynamics under different land uses of outer Himalayan region of Himachal Pradesh. Environ. Conserv. J. 22, 265–270. doi: 10.36953/ECJ.2021.22331
Tiwari, K. N., and Gupta, B. R. (2006). Sulphur for sustainable high yield agriculture in Uttar Pradesh. Ind. J. Fert. 1, 37–52.
Walkey, A., and Black, I. A. (1934). An examination of the Degtjareff method for determining soil organic matter and a proposed modification of the chromic acid titration method. Soil Sci. 37, 29–38. doi: 10.1097/00010694-193401000-00003
Wang, J., Solomon, D., Lehmann, J., Zhang, X., and Amelung, W. (2006). Soil organic sulfur forms and dynamics in the Great Plains of North America as influenced by long-term cultivation and climate. Geoderma 133, 160–172. doi: 10.1016/j.geoderma.2005.07.003
Williams, C. H., and Steinbergs, H. (1959). Soil Sulphur fractions as chemical indices of available Sulphur in some Australian soils. Aust. J. Agric. Res. 10, 340–352. doi: 10.1071/AR9590340
Williams, C. H., and Steinbergs, A. (1962). The valuation of plant-available Sulphur in soils: I. the chemical nature of sulphate in some Australian soils. Plant Soil 17, 279–294. doi: 10.1007/BF01377668
Yang, X. E., Romheld, V., Marschner, H., Baligar, V. C., and Martens, D. C. (1997). Shoot photosynthesis and root growth of hybrid Riceand conventional Rice cultivar as affected by N and K levels in the rhizosphere. Pedosphere 7, 35–42.
Ying-xin, X., Hui, Z., Yun-ji, Z., Li, Z., Jia-heng, Y., Fei-na, C., et al. (2017). Grain yield and water use of winter wheat as affected by water and sulfur supply in the North China plain. J. Integr. Agric. 16, 614–625. doi: 10.1016/S2095-3119(16)61481-8
Keywords: sulfur fractions, organic S, land use practices, bioavailability of S, extractants, mehlich-3
Citation: Padhan D, Rout PP and Sen A (2023) Changes in land use practices influence soil sulfur fractions and their bioavailability. Front. Sustain. Food Syst. 7:1233223. doi: 10.3389/fsufs.2023.1233223
Edited by:
Antonio Santoro, University of Florence, ItalyReviewed by:
Abhijit Sarkar, Indian Institute of Soil Science (ICAR), IndiaVesna Radovanović, Educons University, Serbia
Copyright © 2023 Padhan, Rout and Sen. This is an open-access article distributed under the terms of the Creative Commons Attribution License (CC BY). The use, distribution or reproduction in other forums is permitted, provided the original author(s) and the copyright owner(s) are credited and that the original publication in this journal is cited, in accordance with accepted academic practice. No use, distribution or reproduction is permitted which does not comply with these terms.
*Correspondence: Dhaneshwar Padhan, ZGhhbmVzaHdhci5wYWRoYW5AcmVkaWZmbWFpbC5jb20=
†Present address: Dhaneshwar Padhan, Central Sericultural Research and Training Institute, Central Silk Board, Mysuru, India