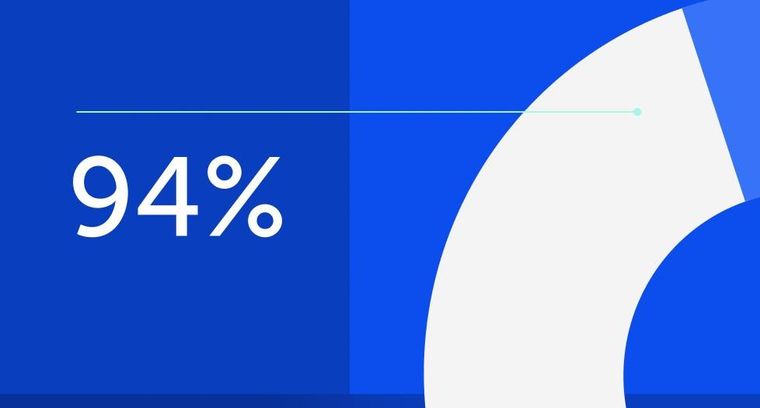
94% of researchers rate our articles as excellent or good
Learn more about the work of our research integrity team to safeguard the quality of each article we publish.
Find out more
ORIGINAL RESEARCH article
Front. Sustain. Food Syst., 23 August 2023
Sec. Crop Biology and Sustainability
Volume 7 - 2023 | https://doi.org/10.3389/fsufs.2023.1224530
This article is part of the Research TopicInterrelationships Between Food Security, Crop Field Management and TechnologyView all 9 articles
Inter-cropping between annual crops with tropical forages through integration crop-livestock systems (ICL) is considered a sustainable option to increase crop diversity and soybean productivity. In this study, we evaluated (1) the biomass production, desiccation efficiency, nutrient accumulation, and biomass decomposition of soil crop residues produced by Panicum maximum plants intercropped with maize in two different sowing methods during the second harvest and (2) investigated how soil crop residues impact the productivity of soybean. The experiment was conducted in a complete block design with three replicates. We compared conventional soybean cultivation with soybean cultivated over soil crop residues produced by a previous integration between maize and two Panicum maximum cultivars: Tamani and Zuri guinea grass, within and between rows of maize plants. Our results showed that Tamani guinea grass showed the highest desiccation efficiency. Zuri and Tamani guinea grass cultivated within and between maize plants resulted in higher biomass production and nutrient cycling potential, resulting in an increase of 28.4% in soybean productivity, compared to soybean grown without soil crop residues. We concluded that ICL system is an efficient method to increase the sustainability of soybean cultivation.
The intensification of land use for food production is a global, complex, and urgent challenge in a growing world population. Reaching future demands efficiently using natural resources is the central point for agricultural sustainability (Damian et al., 2023). Therefore, it is necessary to rethink the future of agricultural production to simultaneously ensure food security and alleviate environmental pressure (Yue et al., 2022). Integrated crop-livestock system (ICL) is considered an efficient, cheap, and sustainable strategy for food production, reducing costs, and risks and conserving natural resources (Silva et al., 2022). When well-managed, ICL systems provide multiple ecosystem services through increased carbon sequestration, water, and soil conservation (Maia et al., 2022), greater efficiency in nutrient use (Muniz et al., 2021), and production diversification (Meo-Filho et al., 2022).
ICL systems can be defined as the cultivation of annual crops and forage species intercropped and/or in rotation, promoting pastures recovery (Damian et al., 2023), greater stocks of soil organic matter and soil moisture (Laroca et al., 2018), improved production efficiency and increased soil fertility through nutrient cycling (Bansal et al., 2022). In addition, ICL systems can contribute to the reduction of greenhouse gas (GHG) emissions, mainly nitrous oxide, by reducing the use of nitrogenous fertilizers. These fertilizers when used as the only source of nitrogen can increase the soil emission of nitrous oxide (Carvalho et al., 2022). Additionally, the ICL system contributes to reducing fertilizer costs (Dias et al., 2020). Covering the soil with plant residues promotes less variation in soil temperature and preserves soil moisture (Calonego et al., 2017). Benefits to the biological properties of the soil are also observed, such as increased microbial activity and suppression of weeds through physical barriers, competition for light and nutrients, and allelopathic effects, reducing the use of pesticides (Vincent-Caboud et al., 2019).
One of the most crucial aspects of ICL systems that affect its success is the composition of forage species, which must present adaptability, versatility, and good performance. In the last years, Panicum maximum cultivars have shown potential to integrate ICL systems (Dias et al., 2020, 2021; Muniz et al., 2021). The correct choice of forage composition of the crop-livestock integration system must provide good soil cover (Andrade et al., 2017), satisfactory animal performance (Dias et al., 2021), adequate biomass production for no-tillage system, slow decomposition of plant residues, and gradual release of nutrients to meet the demand of the subsequent crop (Costa et al., 2020; Dias et al., 2020; Muniz et al., 2021). Therefore, knowledge of the decomposition of the remaining biomass, in the management of a no-tillage system, is fundamental for the adoption of practices to increase the efficiency of the system (Wenneck et al., 2021).
The success of the no-tillage system depends on the amount of biomass present on the soil surface, since it contributes to the accumulation of organic matter, providing improvements in the physical, chemical, and biological attributes of the soil over the years (Muniz et al., 2021). From the perspective of nutrient cycling in production systems, the amount and release rate of nutrients from plant residues left by a previously cultivated crop are of great importance for the nutritional management of the succeeding crop (Baptistella et al., 2020). Therefore, the periods of higher demand for plant nutrients and release of nutrients by plant residues must coincide (Muniz et al., 2021). In the las years, there’s has been a considerable increase in the cost of fertilizers. Therefore, the use of plant residues in agricultural systems should become more important since it provides a more efficient use of nutrients available in the soil (Dias et al., 2020), reducing the need of additional sources of nutrients (Soares et al., 2019). Moreover, ICL systems may increase carbon and nitrogen stocks in the soil (Torres et al., 2019), favoring the mineralization process if the C:N ratio is adequate.
In addition to the species composition, the forage sowing method is another crucial aspect of a successful ICL system. The sowing method is responsible for the success of the integration system due to its influence on biomass production for the no-tillage system and crop productivity due to the different levels of competition between plants (Guarnieri et al., 2019; Oliveira et al., 2020). In this context, integrated systems are technologies that ensure sustainability by improving soil quality (Sarto et al., 2020) and represent an important alternative to ensure high soybean yields in the Brazilian Savannah (Cerrado) (Pires et al., 2022).
Due to the lack of information regarding the performance of the new Panicum maximum cultivars and the adequate sowing methods in a ICL system, studies that investigate these topics are paramount. In this study, we evaluated (1) the biomass production, desiccation efficiency, nutrient accumulation, and biomass decomposition of soil crop residues produced by Panicum maximum plants intercropped with maize in two different sowing methods during the second harvest and (2) investigated how soil crop residues impact the productivity of soybean. We hypothesized that: (a) intercropping systems where the forage is cultivated between rows of maize is more beneficial than sowing in the same row due to the small competition between species, and (b) soybean plants grown over soil crop residues produced by previous integration systems will have better agronomic traits, mainly thousand grain weight and yield increase, when compared to soybean grown without soil cover (conventional soybean cultivation).
The experiment was conducted at Goiano Federal Institute in Rio Verde, Goiás, Brazil (17° 48′ 53′′ S e 50 o 54′ 02′′ W) between January 2021 to March 2022. The soil in the experimental site was classified as Latossolo Vermelho Acriférrico (Santos et al., 2018). A timeline showing the climate conditions registered during the experiment can be found in Figure 1.
Figure 1. Experimental timeline containing the main events and climate conditions registered during the experiment. Bars show the accumulated rainfall in each month, while symbols + lines show the monthly average temperature, maximum temperature, and minimum temperature.
In the first stage of the experiment, a consortium of maize plants (Zea mays hybrid P4285) with forage grasses of the Panicum maximum genus (cv. BRS Tamani and cv. BRS Zuri) was carried out within rows and between rows of maize plants, in addition to sowing maize in monoculture. When grown in both systems, monoculture and consortium, maize seeds were sown at 2 cm depth and 0.5 m distance between rows. Panicum seeds (Tamani and Zuri) were sown at 6 cm depth when intercropped within rows of maize plants to delay their germination compared to maize. When intercropped between rows of maize, Panicum seeds were sown at 0.25 m distance from the maize plants and at 2 cm depth. Each plot contained six rows 3 m long and 0.5 m apart from each other.
All measurements were conducted with plants located inside the four central lines, eliminating 0.5 m from borders. On 5 May 2021, maize and grasses were harvested for silage production. During the off-season (between June and August 2021), forages were successively clipped to simulate grazing. In August, we made the last clipping and forage plants were left in the field for regrowth, desiccation, and formation of biomass to cover the soil.
In the next stage, we prepared the soil for soybean planting. We collected soil samples at 0–20 cm deep and mixed them to form a composite sample. According to the chemical analysis, we observed the following soil characteristics: pH determined in CaCl2: 5.3; Ca: 2.30 cmolc dm−3; Mg: 1.35 cmolc dm−3; Al: 0.01; Al + H: 4.80 cmolc dm−3; K: 0.60 cmolc dm−3; cation exchange capacity of 9.06 cmolc dm−3; base saturation of 47.30%, P (Mehlich): 4.8 mg dm−3; Cu: 4.6 mg dm−3; Zn: 1.0 mg dm−3; Fe: 17.4 mg dm−3; and organic matter (MO) of 39.8 g kg−1. We applied limestone filler (1 ton ha−1) in the entire experimental field.
Soybean seeds (Bônus IPRO 8579 variety) were mechanized sowed in rows 50 cm apart from each other in October 2021. During soybean sowing, we applied phosphorus (120 kg ha−1 of P2O5) in the planting furrow. We did not apply potassium in treatments containing soil cover to utilize the nutrient cycling process provided by the integrated system. Indeed, we only applied potassium (85 kg ha−1 of K2O) in the treatment of Soybean without soil cover, to meet the needs of the crop, because in the absence of soil cover there would be no utilization of nutrient cycling. Fungicide (0.3 L ha−1 of Pyraclostrobin) was applied 40 days after sowing (DAS). We harvested soybean plants in March 2022.
In this study, the experiment was organized in three completely randomized blocks with three replications for each treatment (n = 3), totalizing 8 treatments where soybean was cultivated with or without plant cover residues as follows: Maize monoculture (Soybean cultivated with plant residues of maize without integration with forages), Tamani monoculture (Soybean cultivated with plant residues of Tamani guinea grass without integration with maize), Zuri guinea grass monoculture (Soybean cultivated with plant residues of Zuri guinea grass without integration with Maize), maize + Tamani within rows (Soybean cultivated with plant residues of Tamani guinea grass planted within rows of maize plants), maize + Tamani between rows (Soybean cultivated with plant residues of Tamani guinea grass planted between rows of maize plants), maize + Zuri guinea grass within rows (Soybean cultivated with plant residues of Zuri guinea grass planted within rows of maize plants), maize + Zuri guinea grass between rows (Soybean cultivated with plant residues of Zuri guinea grass planted between rows of Maize plants), and Soybean without soil cover (traditional Soybean monoculture without soil cover residues). A schematic showing the cropping systems can be found in Figure 2.
Figure 2. A Scheme showing the cropping systems: monoculture of maize (A); monoculture of Panicum forage plants (B); maize in intercropped with Panicum cultivated within rows of maize plants (C) and between rows of maize plants (D) and soybean without soil cover (E), during the entire experimental period.
The desiccation of forage plants was performed using Glyphosate (3 L ha−1) (480 g L−1 of active ingredient) in October 2021. Herbicide efficiency was evaluated based on Brazilian Society of Weed Sciences (SBCPD) guidelines (Gazziero, 1995). Desiccation efficiency was evaluated at 7, 14, and 21 days after herbicide application through a visual scale ranging from 0 to 100%, where 0% represents no injury promoted by herbicide and 100% representing the death of plants.
One day before soybean sowing, we collected all the biomass produced in a quadrat of 0.5 × 0.5 m (0.25 m2). Each quadrat was placed randomly in each plot. Plant material was clipped close the soil surface and dried under 55°C in an oven until reach constant dry weight. Then, biomass production (kg ha−1) was calculated.
The decomposition rate of forage biomass was evaluate using litter bags (2 mm mesh, 25 × 30 cm) (Thomas and Asakawa, 1993). Four litter bags per plot were filled with 300 g of dry biomass produced by the treatment and placed on the soil surface. Each litter bag was washed in running water to remove any soil residue and dried in a stoven at 55°C until constant dry weight to obtain the dry mass. Using the biomass production (kg ha−1) of each treatment, we calculated the percentage of biomass decomposition using the ratio between the biomass of litter bags (kg ha−1) and biomass production (Dias et al., 2020).
Biomass samples were grounded to measure the concentration of C, N, P, K, and S according to the method proposed by Malavolta et al. (1997). We calculated the C:N ratio. To evaluate the nutrient accumulation, the concentration of each macronutrient was multiplied by biomass production. The equivalent of fertilizers such as N, P2O5, and K2O released by soil cover produced by maize + forage plants was determined using the atomic mass of each element, according to conventions of analytical chemistry and the concentration of each nutrient (Santos et al., 2014).
Agronomic traits of soybean plants were measured in March 2022 (123 days after sowing). We evaluated the plant height (10 plants evaluated per plot), height of the first and last pod (10 plants evaluated per plot), the number of pods per plant (10 plants evaluated per plot), the number of grains per pod (10 plants per plot), thousand-grain weight, and productivity (kg ha−1).
The efficiency of desiccation was adjusted by regression equations. To describe biomass decomposition and nutrient accumulation, data were fitted with standard error to an exponential mathematical model (y = aekx) and linear to C:N ratio (y = a + bx), using Sigma Plot software. Comparisons between the estimated equations were performed according to Snedecor and Cochran (1989), which tests the homogeneity of the data (F) and the significance of the angular coefficients of the straight (0.4343 k) and linear (log a) of the linearized equations (logy = loga+0.4343kx). To calculate the half-life (t ½), that is, the time required to decompose 50% of the remaining biomass, we used the equation proposed by Paul and Clark (1989), in which, t ½ = 0.693/k, where t ½ is the dry biomass half-life and k is the dry biomass decomposition constant.
Nutrient concentration, fertilizer equivalent, soybean agronomic traits, and grain yield were submitted to analysis of variance using the R program version R-3.1.1 (2014) and ExpDes package (Ferreira et al., 2014). Means were compared using Tukey’s test, with a significance level of 5% probability. Principal component analysis (PCA) was performed using the computational packages “tidyverse,” “stats,” and “factoextra.”
We observed no significant differences in desiccation efficiency between monoculture and intercropped treatments. However, we observed differences in desiccation efficiency between forages (Figure 3). The desiccation efficiency of Tamani guinea grass was 28, 66, and 95% at 7, 14, and 21 days after herbicide application, respectively, while the desiccation efficiency of Zuri guinea grass was 17, 39, and 78% at 7, 14, and 21 days after herbicide application, respectively.
Figure 3. Desiccation efficiency of forage plants. Vertical bars represent the standard deviation of the mean.
The different cultivation systems and cultivars of Panicum maximum influenced soil cover biomass production (Table 1). The highest biomass production was observed for Zuri guinea grass monoculture followed by Zuri guinea grass cultivated between and within rows of maize. On average, Zuri guinea grass regardless sowing method produced 45.34% more biomass compared to maize. In addition, maize in monoculture showed the lowest biomass production when compared to all other treatments. Tamani guinea grass in monoculture showed the highest N concentration, followed by Tamani and Zuri guinea grass intercropped within rows and between rows of maize (Table 1). On average, Tamani guinea grass in monoculture, Tamani and Zuri guinea grass intercropped within rows and between rows of maize showed a 41.63% increase in N concentration when compared to maize biomass in monoculture. For P, K and S, only maize in monoculture had different and smaller concentration of nutrients when compared to concentrations in forages in monoculture and intercropped.
Table 1. Biomass production and initial concentration of nutrients in the biomass of different cultivation systems.
The remaining biomass at the end of soybean growing season (120 days) (Figure 4A) was 1,166; 1,753; 2,707; 1,417; 1,613; 2,032 and 2,323 kg ha−1 for maize monoculture, Tamani monoculture, Zuri monoculture, maize + Tamani within rows, maize + Tamani between rows, maize + Zuri within rows, and maize + Zuri between rows, respectively. These results indicate that Zuri guinea grass in monoculture or intercropped showed the highest remaining biomass. Regarding half-life, maize monoculture, and Zuri guinea grass in monoculture or in intercropped with maize showed the highest values (similar average values of 103 days). C:N ratio linearly decreased in all cultivation systems during biomass decomposition. In all evaluated seasons, maize showed the highest C:N ratio and Tamani guinea grass in monoculture the lowest ratio (Figure 4B).
Figure 4. Remaining biomass (A) and C:N ratio (B) of maize cultivation systems and Panicum maximum cultivars in monoculture and intercropping, during soybean growing season. Vertical bars represent the standard deviation of the mean.
The accumulation of nutrients in plant residues exponentially decreased during the growing season with influence of cultivation systems (Figure 5). We observed that after 0, 30, 60, and 90 days of biomass decomposition the accumulation of N, P, K, and S was higher for Zuri guinea grass in monoculture followed by Zuri guinea grass in intercropped between and within rows of maize. However, after 120 days of biomass decomposition, the accumulation of nutrients was similar between all cultivation systems. We observed that maize showed the lowest accumulation of all nutrients during the entire growing season.
Figure 5. Accumulation of N (A), P (B), K (C), and S (D) of maize cultivation systems and Panicum maximum cultivars in monoculture and intercropping, during soybean growing season. Vertical bars represent the standard deviation of the mean.
Compared with the initial accumulation of nutrients, we observed that after 120 days of biomass decomposition, the percentage of N released on the soil was approximately 74, 81, 80, 83, 79, 81, and 78% at maize monoculture, Tamani guinea grass monoculture, Zuri monoculture, maize + Tamani guinea grass within rows, maize + Tamani guinea grass between rows, maize + Zuri guinea grass within rows, and maize + Zuri between rows treatments, respectively. The percentage of P released on the soil was approximately 74, 78, 81, 79, 78, 79, and 76% at treatments maize monoculture, Tamani guinea grass monoculture, Zuri monoculture, maize + Tamani guinea grass within rows, maize + Tamani guinea grass between rows, maize + Zuri guinea grass within rows, and maize + Zuri between rows treatments, respectively. The percentage of K released on the soil was approximately 94, 94, 96, 9, 95, 96, and 95% at treatments maize monoculture, Tamani guinea grass monoculture, zuri monoculture, maize + Tamani guinea grass within rows, maize + Tamani guinea grass between rows, maize + Zuri guinea grass within rows, and maize + Zuri between rows treatments, respectively. The percentage of S released on the soil was approximately 72, 82, 83, 86, 85, 83, and 79% at treatments maize monoculture, Tamani guinea grass monoculture, zuri monoculture, maize + Tamani guinea grass within rows, maize + Tamani guinea grass between rows, maize + Zuri guinea grass within rows, and maize + Zuri between rows treatments, respectively.
Regarding nutrients half-life (t½), the lowest values were obtained for K: 40 days for maize in monoculture and 33 days for all forages in monoculture or in consortium, indicating a rapid and strong release rate of this nutrient. For all nutrients, maize in monoculture showed the lowest half-life (t½) values, releasing less nutrients to the soil, followed by Zuri guinea grass in monoculture and in intercropped.
We observed that Tamani and Zuri guinea grass in monoculture and Zuri guinea grass between rows promoted the best results in terms of N and P fertilizer equivalent, providing an increase of 67 and 63.27%, respectively, in the return of these nutrients to the soil, compared to maize biomass in monoculture (Table 2). For K, Zuri guinea grass in monoculture was the most efficient cultivar in releasing this nutrient back to the soil. In the contrary direction, maize showed the lowest values of fertilizer equivalent, releasing less nutrients in the soil.
Table 2. Equivalent of N, urea, P2O5, simple superphosphate (SSP), K2O and potassium chloride (KCl) of biomass in different cultivation systems.
Cultivation systems promoted changes in the agronomic traits of soybean plants and grain productivity (Table 3). Taller plants and higher values of first pod height, and number of pods were obtained in Tamani and Zuri guinea grass in monoculture and in consortium. All agronomic traits of soybean cultivated without soil cover were smaller when compared to soybean cultivated with soil cover produced by previous integration.
Table 3. Plant height, height of the first pod, number of pods per plant, number of grains per bean, thousand grain weight, and grain productivity of different cultivation systems.
We observed no statistical differences between treatments for the number of grains per pod (Table 3). However, the thousand-grain weight was approximately 16% smaller at soybean without soil cover treatment when compared to all other cultivation systems with soil cover. The highest productivity of grains was observed at Tamani and Zuri guinea grass, both in monoculture and in the consortium, followed by maize in monoculture, while the smaller productivity was observed in the conventional soybean cultivation (without soil cover).
According to the principal component analysis (PCA) we were able to understand the relationship between measured parameters and treatments discrimination. PCA is a technique used to comprehend the variance and covariance structure of data by employing linear combinations of all the variables. In this analysis, the covariance and/or correlation matrix are decomposed into eigenvalues and eigenvectors, with eigenvalues indicating the variance and eigenvectors representing the coefficients of each variable in the linear combinations. Each principal component is independent and estimated to retain the maximum amount of information regarding the total variation present in the data, in an ordered manner. The number of principal components is equal to the number of variables. In this study, 8 components were obtained, and it was observed that the first and second components explained 92.56% of the total variation in the data (Figure 6). Additionally, all the variables used displayed a strong correlation (r > 0.7) with the first component, suggesting that it effectively describes the structure of variance and covariance in the data. The criteria proposed by Zwick and Velicer (1982), which recommend that variances >70% are considered ideal, were satisfied in our analysis. Therefore, the PCA results were consistent with our initial findings. Figure 6 displays the scores for the treatments and variables represented through linear combinations, with the first component represented horizontally and the second component vertically.
Figure 6. Bidimensional dispersion of principal component analysis scores of the 8 parameters, observations, and average values of treatments regarding biomass production, nutrient accumulation, thousand grain weight, and soybean productivity. Treatments: Maize (Soybean cultivated with plant residues of maize without integration with forages), Tamani (Soybean cultivated with plant residues of Tamani guinea grass without integration with maize), Zuri (Soybean cultivated with plant residues of Zuri guinea grass without integration with maize), MTL: maize + Tamani within rows (Soybean cultivated with plant residues of Tamani guinea grass planted within rows of maize plants), MTE: maize + Tamani between rows (Soybean cultivated with plant residues of Tamani guinea grass planted between rows of maize plants), MZL: maize + Zuri within rows (Soybean cultivated with plant residues of Zuri guinea grass planted within rows of maize plants), MZE: maize + Zuri between rows (Soybean cultivated with plant residues of Zuri guinea grass planted between rows of maize plants), and Soybean (traditional Soybean monoculture without soil cover residues). Biomass = biomass production. Nitrogen, Phosphorous, Sulfur, weight: the thousand grain weight, productivity = soybean productivity.
In this study, we observed a greater desiccation efficiency of Tamani guinea grass. This result is presumably associated with the large proportion of leaves of this cultivar., making it more susceptible to glyphosate. On the other hand, Zuri guinea grass showed an efficiency of only 78% at 21 days after desiccation. This lower efficiency can be explained by the morphology of this forage, which has a greater number of tussocks (Rhodes et al., 2021). However, Zuri guinea grass in monoculture, followed by Zuri guinea grass in consortium, showed the highest biomass production and remaining biomass after a few weeks of decomposition. This result can be explained by the morphology of this cultivar., which has a tall size, high production of dry mass per hectare, caespitous growth, high regrowth vigor, and elevated production of support structures such as stems, demonstrating its high potential for biomass production (Almeida et al., 2022). In addition, Zuri guinea grass is recognized as a drought tolerant cultivar (Figure 1) and moderately adapted to conditions of excessive soil moisture (Bonfim-Silva et al., 2022).
It is important to highlight that at the end of the soybean cycle, the remaining biomass (Figure 3A) of Zuri guinea grass in monoculture and intercropped were higher by 25% compared to Tamani guinea grass in monoculture (1,753 kg ha−1) and 50% when compared to maize (1,166 kg ha−1), demonstrating that Zuri guinea grass provided the greatest soil cover during the soybean cycle. Moreover, it is important to note that the biomass produced by Zuri guinea grass in the different cultivation systems provided greater soil coverage until soybean harvest, demonstrating the potential to ensure production stability and soil protection in cases of dry spells. The results reported in this study are relevant in the decision making of the correct choice of forage, especially considering the Brazilian Savannah (Cerrado) located in the central region Brazil, which presents high temperatures throughout the year (Zagato et al., 2018).
Maize biomass remained in the soil between May and November 2021 (off-season) and had more time of decomposition when compared to forages. Although maize biomass has a large number of stalks, increasing the decomposition time on the soil surface, it has a small soil cover, corroborating the results reported by Dias et al. (2020) and Muniz et al. (2021). The biomass produced by maize + Zuri guinea grass in both sowing methods showed a longer half-life with an average of 103 days. This result can be explained by the fact that maize and Zuri guinea grass both have a high proportion of stems, a higher proportion of lignin and a high C:N ratio in the residues, promoting greater persistence of soil cover (Chen et al., 2021; Rhodes et al., 2021). However, as previously mentioned, maize biomass does not provide adequate soil cover.
The amount of time that biomass remains in the soil is determined by the rate of decomposition, which is directly influenced by the C:N ratio and lignin content of the residue (Muniz et al., 2021). According to Truong and Marschner (2018), C:N ratio values between 12 and 25 contribute to mineralization, while values above 50 contribute to the immobilization of nutrients in the soil. Therefore, an adequate range from 25 to 30 is ideal to balance mineralization and immobilization. Corroborating the findings of Mingotte et al. (2020), we found that in all evaluated decomposition times, the highest C:N ratio was observed for maize biomass, as shown in Figure 3B. According to Miguel et al. (2018), maize has a higher proportion of recalcitrant material (stem, cob, and straw), and the action and penetration of decomposing microorganisms is hampered due to the high concentration of fibers that confers resistance to the material. In the opposite direction, forage cultivation systems in monoculture or in intercropped showed lower values of C:N ratio in all decomposition periods. This result can be explained by the high ratio of leaves to stems that both cultivars have, leading to more intense biomass decomposition (Dias et al., 2020), especially for Tamani guinea grass, which presented the lowest C:N ratio. Different from what was observed for maize, biomass production produced an adequate amount of soil cover which remained in the soil until the final cycle of soybean development (Figure 3A).
According to our results, Tamani guinea grass produced the soil cover with the highest N concentration, presumably because this cultivar has a higher proportion of leaves, greatly contributing to the ecosystem N cycling (Muniz et al., 2021). N and K are the nutrients most extracted by forage plants (Costa et al., 2017) but at the same time, these nutrients are easily leached. Therefore, through its deep and aggressive root system, forage plants can absorb nutrients from deep layers and release them on the soil surface, benefiting the subsequent crop (Baptistella et al., 2020; Costa et al., 2021). We also observed a high N, P, K, and S accumulation in treatments containing Zuri guinea grass (Figure 4), which is presumable associated with the elevated production of biomass in these treatments (Table 1), where the accumulated nutrients were deposited in the soil and supplied the soybean demands, especially N, since in its initial phase, soybeans still do not present an efficient N fixation by biological activity (Muniz et al., 2021). According to Oliveira Junior et al. (2016), from all the N that soybean demands (190–372 kg ha−1), around 65–85% of N comes from biological fixation, while the rest is provided by the soil.
Corroborating the findings of Miguel et al. (2018), Baptistella et al. (2020), and Costa et al. (2021), K showed the shortest half-life (t½) of all nutrients. K is easily released from the plant tissue, since it is not part of any structure or organic molecule, being predominantly a free cation with high mobility in plants, being easily washed by rainwater after the disruption of plasma membranes (Taiz et al., 2017). In our study, K showed a release rate above 95% for all cropping systems with soil cover biomass. Since here we do not apply K fertilizer in treatments with soil cover, soil biomass contributed to the greater cycling rate of this nutrient, which is the most absorbed by soybean plants. During the soybean growing season, K sharply decreased its amount in the residues, corroborating the observations made by Dias et al. (2020) and Muniz et al. (2021). It is noteworthy that at the end of the soybean cycle (120 days), the remaining amount of K in the biomass was less than 5% of the total initial amount. In the opposite direction, the longest half-life (t½) for all nutrients was observed in maize monoculture treatment followed by Zuri guinea grass in monoculture and in consortium. The higher lignin content and C:N ratio of these crops, mainly maize, contributes to the immobilization of nutrients (Rhodes et al., 2021).
Soybean accumulates most of the macronutrients between 82 and 92 days of its development, and the highest absorption rate occurs between 39 and 58 days (Carmello and Oliveira, 2006). In the present study, the half-life of nutrients averaged 53, 66, 34, and 59 days for N, P, K, and S, respectively, with a release above 78% at 120 days of decomposition, demonstrating the potential of forages as supply of nutrients for soybean crop. The increased biomass production by Tamani and Zuri guinea grasses in monoculture and intercropped resulted in higher values of N and P fertilizer equivalent. According to our results, Zuri guinea grass in monoculture seems to be the most efficient cultivar in returning K to the soil, which can also be explained by the higher biomass production that this forage produced. The biomass produced by maize provided lower values of equivalent in fertilizers. This result shows the importance of forages in the nutritional balance of plants in integrated production systems, where part of the nutrients is returned to the soil by the mineralization process.
In the integrated systems is important to consider how much nutrients will be release from plant residues when calculating fertilizer recommendations, as a large proportion of these nutrients returns to the soil (Assmann et al., 2017). Our study shows the saving of mineral fertilizer (Table 2), with greater emphasis on the biomass produced by Zuri guinea grass, which showed savings of 185 kg of urea, 80 kg of simple superphosphate and 142 kg ha−1 of potassium chloride. This elevated amount of equivalent in nutrients obtained from plant residues can help producers to obtain a greater productivity reducing the cost of mineral fertilizers (Muniz et al., 2021).
The integrated crop-livestock system is a promising and sustainable technique for producing biomass for no-tillage systems, positively influencing the productivity of cultivated plants. In this study, we observed the clear benefits of integrated systems for soybean growth and grain productivity. In the cultivation systems with Tamani and Zuri guinea grasses intercropped or not, plant height, insertion of the first pod, and the number of pods per soybean plant were higher, presumably due to the higher amount of plant residues produced by each forage and the release of nutrients. Our results also demonstrate that the release of nutrients by plant residues was synchronized with the nutrient absorption by soybean plants during their development cycle, improving soybean productivity (Costa et al., 2021). These results have also been corroborated in other studies with different integration systems (Tanaka et al., 2019; Dias et al., 2020; Muniz et al., 2021; Pires et al., 2022).
The weight of 1,000 grains is a component of soybean yield, directly related to crop productivity. In this study, the highest values of the weight of 1,000 grains were obtained in cropping systems with soil cover biomass. Moreover, we observed higher grain yields, in integrated systems. All these previous results have been corroborated by other recent studies (Dias et al., 2020; Oliveira et al., 2020; Costa et al., 2021; Muniz et al., 2021). Higher soybean yields are related to improvements in soil biochemical and biological properties, which are positively influenced by integrated systems. In fallow areas during the off-season, the accumulation of biomass and nutrient absorption by soybean in the succession system is negatively affected, especially in years with unfavorable weather conditions, compromising crop productivity (Soratto et al., 2022). Therefore, the correct choice of forage and sowing method to compose the crop-livestock integration system optimizes land use, increases the diversity of production, and reduces the use of mineral fertilizers, bringing greater sustainability to agricultural systems. PCA analyses also reinforced our results by grouping Zuri and Tamani guinea grasses (Groups 3 and 4) due to the greater productivity, nutrient accumulation, and soybean productivity when compared to maize in monoculture and soybean without soil cover (Groups 1 and 2).
Tamani guinea grass showed the highest desiccation efficiency.
Zuri guinea grass in monoculture and intercropped in the two forms of sowing provided greater biomass production and nutrient cycling. However, both Zuri and Tamani guinea grass can be indicated as cover crops for positively influencing agronomic characteristics and soybean productivity.
Integrated systems with tropical forages for soil cover proved to be an efficient technique for biomass production and nutrient cycling since it makes better use of soil nutrients and contributes to the sustainability of agricultural systems in tropical regions.
The original contributions presented in the study are included in the article/Supplementary material, further inquiries can be directed to the corresponding author.
JS, KC, and LS wrote the manuscript. JS, LS, JC, and KO collected data in the field and processed the data. KC, AC, EH, and ES contributed with statistical analysis. JS, KC, ES, EH, LV, FS, CM, AS, and AC conceived and designed the experiments. All authors contributed to the revision of the manuscript.
This work was supported by National Council for Scientific and Technological Development (CNPq) and Coordination of Superior Level Staff Improvement (CAPES). CNPq provided a scholarship for João Victor Campos Pinho Costa (process 138753/2022–9). CAPES provided scholarships for João Antônio Gonçalves e Silva (process 88887.718294/2022–00) and Luciana Maria da Silva (process 88887.613180/2021–00).
The authors thank the Instituto Federal Goiano for supporting this study and the Coordination for the Improvement of Higher Education Personnel (CAPES) for granting the PhD scholarship.
The authors declare that the research was conducted in the absence of any commercial or financial relationships that could be construed as a potential conflict of interest.
All claims expressed in this article are solely those of the authors and do not necessarily represent those of their affiliated organizations, or those of the publisher, the editors and the reviewers. Any product that may be evaluated in this article, or claim that may be made by its manufacturer, is not guaranteed or endorsed by the publisher.
The Supplementary material for this article can be found online at: https://www.frontiersin.org/articles/10.3389/fsufs.2023.1224530/full#supplementary-material
Almeida, E. M. D., Montagner, D. B., Difante, G. D. S., Araújo, A. R. D., Santana, J. C. S., Gurgel, A. L. C., et al. (2022). Growth dynamics and nutrient uptake of panicum maximum under nitrogen fertilisation. New Zealand J. Agric Res. 66, 244–258. doi: 10.1080/00288233.2022.2057554
Andrade, C. A. O., Borghi, E., Bortolon, L., Bortolon, E. S. O., Camargo, F. P., Avanzi, J. C., et al. (2017). Straw production and agronomic performance of soybean intercropped with forage species in no-tillage system. Pesqui. Agropecu. Bras. 52, 861–868. doi: 10.1590/s0100-204x2017001000005
Assmann, J. M., Martins, A. P., Anghinoni, I., Denardin, L. G. O., Nichel, G. H., Costa, S. E. V. G. A., et al. (2017). Phosphorus and potassium cycling in a long-term no-till integrated soybean-beef cattle production system under different grazing intensities in subtropics. Nutr Cycl Agroecosyst, 108, 21–33. doi: 10.1007/s10705-016-9818-6
Bansal, S., Chakraborty, P., and Kumar, S. (2022). Crop–livestock integration enhanced soil aggregate-associated carbon and nitrogen, and phospholipid fatty acid. Sci. Rep. 12, 2781–2713. doi: 10.1038/s41598-022-06560-6
Baptistella, J. L. C., Andrade, S. A. L., Favarin, J. L., and Mazzafera, P. (2020). Urochloa in tropical agroecosystems. Front. Sustain. Food Syst. 4:119. doi: 10.3389/fsufs.2020.00119
Bonfim-Silva, E. M., Oliveira, N. P., Mazero, H. M., Alves, D. J., Oliveira, J. R., and Silva, T. J. A. (2022). Production and morphophysiological responses of Panicum maximum cv. BRS Zuri to water availability. Aust. J. Crop. Sci. 16, 1203–1211. doi: 10.21475/ajcs.22.16.11.p3703
Calonego, J. C., Raphael, J. P., Rigon, J. P., Oliveira Neto, L., and Rosolem, C. A. (2017). Soil compaction management and soybean yields with cover crops under no-till and occasional chiseling. Eur. J. Agron. 85, 31–37. doi: 10.1016/j.eja.2017.02.001
Carmello, Q. A. C., and Oliveira, F. A. (2006). Nutrição de lavouras de soja: situação atual e perspectivas. Visão Agrícola. 3, 8–11.
Carvalho, A. M., Santos, D. C. R., Ramos, M. L. G., Marchão, R. L., Vilela, L., Sousa, T. R., et al. (2022). Nitrous oxide emissions from a long-term integrated crop–livestock system with two levels of P and K fertilization. Land 11:1535. doi: 10.3390/land11091535
Chen, H., Liu, Y., Lü, L., Yuan, L., Jia, J., Chen, X., et al. (2021). Effects of no-tillage and Stover mulching on the transformation and utilization of chemical fertilizer N in Northeast China. Soil Tillage Res. 213:105131. doi: 10.1016/j.still.2021.105131
Costa, N. R., Andreotti, M., Crusciol, C. A. C., Pariz, C. M., Bossolani, J. W., Castilhos, A. M., et al. (2020). Can palisade and Guinea grass sowing time in intercropping systems affect soybean yield and soil chemical properties? Front. Sustain. Food Syst. 4:81. doi: 10.3389/fsufs.2020.00081
Costa, N. R., Andreotti, M., Crusciol, C. A. C., Pariz, C. M., Bossolani, J. W., Pascoaloto, I. M., et al. (2021). Soybean yield and nutrition after tropical forage grasses. Nutr. Cycl. Agroecosyst. 121, 31–49. doi: 10.1007/s10705-021-10157-2
Costa, R. R. G. F., Costa, K. A. P., Severiano, E. C., Santos, C. B., Rocha, A. F. S., Souza, W. F., et al. (2017). Nutrients cycling and accumulation in pearl millet and Paiaguas palisadegrass biomass in different forage systems and sowing periods. Sci. Agrár. 18, 166–178. doi: 10.5380/rsa.v18i4.51955
Damian, J. M., Matos, E. S., Pedreira, B. C., Carvalho, P. C. F., Premazzi, L. M., and Cerri, C. E. P. (2023). Intensification and diversification of pasturelands in Brazil: patterns and driving factors in the soil carbon stocks. Catena 220:106750. doi: 10.1016/j.catena.2022.106750
Dias, M. B. C., Costa, K. A. P., Severiano, E. C., Bilego, U., Furtini Neto, A. E., Almeida, D. P., et al. (2020). Brachiaria and Panicum maximum in an integrated crop-livestock system and a second-crop corn system in succession with soybean. J. Agric. Sci. 158, 206–217. doi: 10.1017/S0021859620000532
Dias, M. B. C., Costa, K. A. P., Severiano, E. C., Bilego, U. O., Vilela, L., Souza, W. F., et al. (2021). Cattle performance with Brachiaria and Panicum maximum forages in an integrated crop-livestock system. Afr. J. Range For. Sci. 39, 230–243. doi: 10.2989/10220119.2021.1901311
Ferreira, E. B., Cavalcanti, P. P., and Nogueira, D. A. (2014). Exp des: an R package for ANOVA and experimental designs. Appl. Math. 5, 2952–2958. doi: 10.4236/am.2014.519280
Gazziero, D. L. P. (1995). Procedimentos para instalação, avaliação e análise de experimentos com herbicidas. Londrina: Sociedade Brasileira da Ciência de Plantas Daninhas.
Guarnieri, A., Costa, K. A. P., Severiano, E. C., Silva, A. G., Oliveira, S. S., and Santos, C. B. (2019). Agronomic and productive characteristics of maize and Paiaguas palisadegrass in integrated production systems. Semin. Ciênc. Agrár. 40, 1185–1198. doi: 10.5433/1679-0359.2019v40n3p1185
Laroca, J. V. S., Souza, J. M. A., Pires, G. C., Pires, G. J. C., Pacheco, L. P., Silva, F. D., et al. (2018). Soil quality and soybean productivity in crop-livestock integrated system in no-tillage. Pesqui. Agropecu. Bras. 53, 1248–1258. doi: 10.1590/S0100-204X2018001100007
Maia, S. M. F., Medeiros, A. S., Santos, T. C., Lyra, G. B., Lal, R., Assad, E. D., et al. (2022). Potential of no-till agriculture as a nature-based solution for climate-change mitigation in Brazil. Soil Tillage Res. 220:105368. doi: 10.1016/j.still.2022.105368
Malavolta, E., Vitti, G.C., and Oliveira, S.A. (1997). Avaliação do estado nutricional de plantas: princípios e aplicações. 2 Piracicaba: Potafos. p. 319.
Meo-Filho, P., Berndt, A., Pezzopane, J. R. M., Pedroso, A. F., Bernardi, A. C. C., Rodrigues, P. H. M., et al. (2022). Can intensified pasture systems reduce enteric methane emissions from beef cattle in the Atlantic forest biome? Agronomy 12:2738. doi: 10.3390/agronomy12112738
Miguel, A. S. D. C. S., Pacheco, L. P., Carvalho, Í. C., Souza, E. D., Feitosa, P. B., and Petter, F. A. (2018). Phytomass and nutrient release in soybean cultivation systems under no-tillage. Pesqui. Agropecu. Bras. 53, 1119–1131. doi: 10.1590/s0100-204x2018001000005
Mingotte, F. L. C., Jardim, C. A., Yada, M. M., Amaral, C. B., Chiamolera, T. P. L. C., Coelho, A. P., et al. (2020). Impact of crop management and no-tillage system on grain and straw yield of maize crop. Cereal Res. Commun. 48, 399–407. doi: 10.1007/s42976-020-00051-y
Muniz, M. P., Costa, K. A. P., Severiano, E. C., Bilego, U. O., Almeida, D. P., Furtini Neto, A. E., et al. (2021). Soybean yield in integrated crop–livestock system in comparison to soybean–maize succession system. J. Agric. Sci. 159, 188–198. doi: 10.1017/S0021859621000393
Oliveira, I. P., Costa, K. A. P., Assis, R. L., Severiano, E. C., Dias, M. B. C., and Santos, C. B. (2020). Agronomic characteristics of soybean under the production and decomposition of sunflower and Paiaguas palisadegrass biomass in different integrated production systems. Aust. J. Crop. Sci. 14, 788–794. doi: 10.21475/ajcs.20.14.05.p2229
Oliveira Junior, A., Castro, C., Pereira, L. R., and Domingos, C. S. (2016). Estádios fenológicos e marcha de absorção de nutrientes da soja. Londrina: Embrapa Soja.
Paul, E.A., and Clark, F.E. (1989). Soil microbiology and biochemistry. San Diego, Academic Press, p.275.
Pires, G. C., Denardin, L. G. O., Silva, L. S., Freitas, C. M., Gonçalves, E. C., Camargo, T. A., et al. (2022). System fertilization increases soybean yield through soil quality improvements in integrated crop-livestock system in tropical soils. J. Soil Sci. Plant Nutr. 22, 4487–4495. doi: 10.1007/s42729-022-01050-0
Rhodes, A. C., Plowes, R. M., Goolsby, J. A., Gaskin, J. F., Musyoka, B., Calatayud, P. A., et al. (2021). The dilemma of Guinea grass (Megathyrsus maximus): a valued pasture grass and a highly invasive species. Biol. Invasions 23, 3653–3669. doi: 10.1007/s10530-021-02607-3
Santos, F. C., Albuquerque Filho, M. R., Vilela, L., Ferreira, G. B., Carvalho, M. C. S., and Viana, J. H. M. (2014). Decomposição e liberação de macronutrientes da palhada de milho e braquiária, sob integração lavoura-pecuária no cerrado baiano. Rev. Bras. Ciênc. Solo 38, 1855–1861. doi: 10.1590/S0100-06832014000600020
Santos, H. G., Jacomine, P. K. T., Anjos, L. H. C., Oliveira, V. A., Lumbreras, J. F., Coelho, M. R., et al. (2018). Sistema Brasileiro de Classificação de Solos. 5th Edn. Brasília: Embrapa CNPS, 356.
Sarto, M. V., Borges, W. L., Sarto, J. R. W., Pires, C. A. B., Rice, C. W., and Rosolem, C. A. (2020). Soil microbial community and activity in a tropical integrated crop-livestock system. Appl. Soil Ecol. 145:103350. doi: 10.1016/j.apsoil.2019.08.012
Silva, L. S., Laroca, J. V. S., Coelho, A. P., Gonçalves, E. C., Gomes, R. P., Pacheco, L. P., et al. (2022). Does grass-legume intercropping change soil quality and grain yield in integrated crop-livestock systems? Appl. Soil Ecol. 170:104257. doi: 10.1016/j.apsoil.2021.104257
Snedecor, G. W., and Cochran, W. G. (1989). Statistical methods. Octave Edn. Ames, Iowa: Iowa State University Press.
Soares, D. S., Ramos, M. L. G., Marchao, R. L., Maciel, G. A., de Oliveira, A. D., Malaquias, J. V., et al. (2019). How diversity of crop residues in long-term no-tillage systems affect chemical and microbiological soil properties. Soil Tillage Res, 194:104316. doi: 10.1016/j.still.2019.104316
Soratto, R. P., Guidorizzi, F. V., Sousa, W. S., Gilabel, A. P., Job, A. L., and Calonego, J. C. (2022). Effects of previous fall–winter crop on spring–summer soybean nutrition and seed yield under no-till system. Agronomy 12:2974. doi: 10.3390/agronomy12122974
Taiz, L., Zeiger, E., Møller, I.M., and Murphy, A. (2017). Fisiologia e desenvolvimento vegetal. 6. Porto Alegre: Artmed.
Tanaka, K. S., Crusciol, C. A., Soratto, R. P., Momesso, L., Costa, C. H., Franzluebbers, A. J., et al. (2019). Nutrients released by Urochloa cover crops prior to soybean. Nutr. Cycl. Agroecosyst. 113, 267–281. doi: 10.1007/s10705-019-09980-5
Thomas, R. J., and Asakawa, N. M. (1993). Decomposition of leaf litter tropical forage grasses and legumes. Soil Biol. Biochem. 25, 1351–1361. doi: 10.1016/0038-0717(93)90050-L
Torres, J. L. R., Júnior, J. M., Júnior, J. S., Vieira, D. D. S., Souza, Z. M., Assis, R. L., et al. (2019). Soil physical attributes and organic matter accumulation under no-tillage systems in the Cerrado. Soil Res. 57, 712–718. doi: 10.1071/SR19047
Truong, T. H. H., and Marschner, P. (2018). Respiration, available N and microbial biomass N in soil amended with mixes of organic materials differing in C/N ratio and decomposition stage. Geoderma 319, 167–174. doi: 10.1016/j.geoderma.2018.01.012
Vincent-Caboud, L., Casagrande, M., David, C., Ryan, M. R., Silva, E. M., and Peigne, J. (2019). Using mulch from cover crops to facilitate organic no-till soybean and maize production. A review. Agron. Sustain. Dev. 39, 1–15. doi: 10.1007/s13593-019-0590-2
Wenneck, G. S., Saath, R., Araujo, L. L., Pereira, G. L., Rezende, R., and Wenneck, G. S. (2021). Decomposition of the remaining straw during soybean growing in the midwestern Paraná. Brazil. Rev. Agricul. Neotrop. 8:6534. doi: 10.32404/rean.v8i4.6534
Yue, Q., Guo, P., Wu, H., Wang, Y., and Zhang, C. (2022). Towards sustainable circular agriculture: an integrated optimization framework for crop-livestock-biogas-crop recycling system management under uncertainty. Agric. Syst. 196:103347. doi: 10.1016/j.agsy.2021.103347
Zagato, L. Q. S. D., Araujo, L. C., Santos, S. N. A., Ludkiewicz, M. G. Z., Silveira, O., and Santos, A. C. (2018). Decomposition of straw resulting from different strategies of recovery of degraded pastures using an integrated crop-livestock system. Semin. Ciênc. Agrár. 39, 1397–1406. doi: 10.5433/1679-0359.2018v39n4p1397
Keywords: Glycine max L., Panicum maximum cv. BRS Tamani, Panicum maximum cv. BRS Zuri, sustainability, Zea mays
Citation: e Silva JAG, Costa KAdP, da Silva LM, Severiano EdC, Silva FG, Habermann E, Martinez CA, Vilela L, da Silva AG, Costa AC, Costa JVCP and de Oliveira KJ (2023) Integrated systems improve the sustainability of soybean cultivation in the tropical region. Front. Sustain. Food Syst. 7:1224530. doi: 10.3389/fsufs.2023.1224530
Received: 17 May 2023; Accepted: 03 August 2023;
Published: 23 August 2023.
Edited by:
Xiukang Wang, Yan'an University, ChinaReviewed by:
Rodolfo Lizcano Toledo, Tolima University, ColombiaCopyright © 2023 e Silva, Costa, da Silva, Severiano, Silva, Habermann, Martinez, Vilela, da Silva, Costa, Costa and de Oliveira. This is an open-access article distributed under the terms of the Creative Commons Attribution License (CC BY). The use, distribution or reproduction in other forums is permitted, provided the original author(s) and the copyright owner(s) are credited and that the original publication in this journal is cited, in accordance with accepted academic practice. No use, distribution or reproduction is permitted which does not comply with these terms.
*Correspondence: Kátia Aparecida de Pinho Costa, a2F0aWEuY29zdGFAaWZnb2lhbm8uZWR1LmJy
Disclaimer: All claims expressed in this article are solely those of the authors and do not necessarily represent those of their affiliated organizations, or those of the publisher, the editors and the reviewers. Any product that may be evaluated in this article or claim that may be made by its manufacturer is not guaranteed or endorsed by the publisher.
Research integrity at Frontiers
Learn more about the work of our research integrity team to safeguard the quality of each article we publish.