- 1Facultad de Ciencias Agrarias y Agroindustriales, Departamento de Agroindustria, Universidad del Cauca, Popayán, Colombia
- 2Departamento de Ciencias Agrarias y del Ambiente, Universidad Francisco de Paula Santander, Ocaña, Colombia
Background: Quinoa consumption has created a challenge for producers and food processors. They need to study new cultivars and the functional properties of quinoa flours.
Methods: The structural and rheological properties of six quinoa cultivars (Titicaca, Blanca real, Soracá, Pasankalla, Puno and Nariño) grown at different altitudes were studied using Fourier Transform Infrared spectroscopy (FTIR) and dynamic oscillatory tests. The FTIR spectra revealed differences in the protein and starch structures among the cultivars, which could be related to their adaptation to different environmental conditions. The rheological analysis showed that the quinoa gels exhibited viscoelastic behavior, with a predominance of the elastic component (G’) over the viscous component (G”). The linear viscoelasticity range was determined by applying a strain sweep test (0.001–100%) at a constant frequency of 5 Hz. The frequency sweep test (0.01–100 Hz) at a constant strain amplitude of 0.1% within the linear regime was used to obtain the storage modulus (G’), the loss modulus (G”) and the complex viscosity (η*).
Results: The Burgers model was fitted to the experimental data, and the four parameters (η1, η2, R1, and R2) were obtained for each cultivar. The results showed that the cultivar., the altitude and their interaction had significant effects on the rheological properties of the quinoa gels. The cultivars grown at higher altitudes tended to have higher G’, G”, and η* values than those grown at lower altitudes, indicating a stronger gel network. The cultivars also differed in their relaxation times, with Titicaca and Blanca real having the shortest and longest times, respectively. These differences could be attributed to the variations in the protein and starch structures of the quinoa flours, as well as the water absorption and gelation properties of the cultivars.
Conclusion: The viscoelastic behavior of gels is influenced by the structural conformation of their components, such as proteins and starch. These components provide stiffness and elasticity to the gels. The structural conformation can change depending on the environmental conditions and the phenotypic characteristics of the components.
1. Introduction
Pseudocereals are a group of plants that produce seeds that resemble cereals but are not botanically related to them. They have several advantages over traditional cereals, such as higher protein and mineral content, gluten-free, and lower glycemic index. One of the most remarkable features of pseudocereals is their ability to adapt to different ecological conditions, such as different gradients of altitude, luminosity, salinity, and soil pH. This facilitates the production of the crop to different climatic regions (Huang et al., 2021) to produce grains with an industrial potential in food processing (Rodríguez-Gómez et al., 2021). Over time, many countries have tried to develop varieties, sharing and exchanging germplasm to improve distribution in the production of these grains. In Colombia, quinoa is a versatile crop that is adapting to different climates and soils. The most common cultivars are Blanca Real, Soraca, Pasankalla and Puno, among others. These cultivars have different traits, such as seed size and color, type of inflorescence, duration of the production cycle and nutritional value. In addition, these cultivars have shown high tolerance to drought and salinity, which makes them suitable for use in food security strategies (García-Parra et al., 2022a).
Grains have different nutritional qualities depending on the biotic and abiotic factors that affect their growth. Protein content, mineral composition, and antioxidant activity are some of the grain nutritional characteristics that can be influenced by external conditions (Miranda et al., 2013; Lesjak and Calderini, 2017). Quinoa and amaranth are grains with an excellent amino acid profile and high antioxidant potential, which increases their nutraceutical value (García-Parra et al., 2021). They are promising ingredients for novel foods with health benefits. Their proteins and starches can be modified by physical milling methods, which makes their flours suitable for the preparation of functional foods (Roa-Acosta et al., 2017, 2020). At an industrial level, its flours have been successfully used in the formulation of snacks and pasta (Muñoz-Pabon et al., 2022; Roa-Acosta et al., 2022), as well as in the non-food sector (Contreras-Jiménez et al., 2019; Felix et al., 2021). However, there is still a need to explore the potential applications of these grains in other food matrices, such as bakery products, dairy products, beverages, and meat products, as well as to evaluate their sensory acceptability and consumer preferences.
The rheological properties of quinoa flour-based dispersions are influenced by several factors, such as the cultivar., the altitude, the water content, and the temperature. Recently, García-Parra et al. (2022a) studied the consistency and fluidity of different quinoa flour-based dispersions from Blanca real, Pasankalla, Puno, Soracá and Titicaca cultivars. The researchers found significant differences in these rheological parameters. These differences were associated with the altitudinal gradient where the seeds were produced, as well as the variability of the cultivars. Therefore, studying the rheological properties is of great interest to expand the limits of its applicability, since viscoelasticity, that is, the storage modulus (G’) and loss modulus (G”) correlate with the yield and quality of the flour. However, measuring these properties can be complex and time-consuming. Therefore, mathematical models must be applied that allow us to obtain summary information in the form of parameters or constants, which can then be correlated with texture attributes and quality of final products. In this sense, there are models such as Burgers, used to describe the creep-recovery response of viscoelastic materials (Sun et al., 2020) as for example doughs in the bakery industry. This model consists of four elements: a spring and a dashpot in series (Maxwell element), and another spring and dashpot in parallel (Kelvin-Voigt element). The Burgers model can capture both the elastic and viscous behavior of materials under constant stress or strain. On the other hand, there is the potency model, where (S) is the gel force and (n) the exponent that helps predict the behavior of the gel (García-Salcedo et al., 2018). These last two parameters are used to predict the stability of dispersions over time. The potency model assumes that the gel strength is proportional to the concentration of the dispersed phase raised to a power (n). The objective of the study was to determine the viscoelastic behavior of gels obtained from cultivars to expand their potential use in tech-functional foods. The gels were prepared from different types of flours and their rheological properties were measured using a rheometer. The results showed that the Burgers and potency models fitted well to the experimental data and that the gels exhibited different degrees of elasticity and viscosity depending on the flour type and concentration. The study also revealed that some gels had high gel force and low exponent values, indicating a strong and stable network structure suitable for tech-functional foods.
2. Materials and methods
2.1. Materials
Grains of five quinoa cultivars were obtained from municipalities of Oicatá, Ventaquemada, and Tuta in the Department of Boyacá-Colombia. The cultivars were Titicaca, Blanca real, Soracá, Pasankalla, and Puno. Pasankalla had a dark brown phenotype, while the others had a white phenotype. The plants were grown in high-density plots. A random sample of 30 kg of each cultivar was collected for analysis (García-Parra et al., 2022a,b,c).
2.2. Methods
2.2.1. Approximate chemical composition
The Kjeldahl method (AOAC 2005, 960.52) and conversion factor of 6.25 was used to measure the total raw protein from the seeds. Fat content was determined by the method (AOAC 2005, 922.06) using Soxhlet (Soxtec 2050). Finally, the total carbohydrates were calculated by the difference (i.e., protein + ash + fat + moisture −100) (García-Parra et al., 2022a).
2.2.2. IR spectroscopy
The IR spectrum in reflection mode was measured using IRAFFINITY-1S (Shimadzu Corp., Japan) in the range of 400 cm−1 to 4,000 cm−1. The number of scans was 32 at a resolution of 4 cm−1 and a temperature of 25°C. A blank background spectrum was taken for each cultivar prior to the measurements (Garcia-Parra et al., 2022b). The spectra were processed with OriginPro, version 7th, and normalized over a baseline from 0 to 1 to highlight the highest transmittance peaks. To investigate the changes in protein secondary structure, we used Fourier transform deconvolution to resolve the amide I band in the range of 1,600–17,00 cm−1. We also quantified the starch content by detecting its characteristic peak at 875–1175 cm−1 band, following the protocol of Yang et al. (2015).
2.2.3. Flour production
An analytical knife mill, IKA A11 basic, Brazil, was used to produce flours. The flours were then sieved using an A.S.T.M.E.11 sieve to achieve a particle size distribution between 180 and 125 μm. The flours were packed and stored under vacuum for subsequent characterization.
2.2.4. Gels production
Aqueous dispersions of each flour were prepared at a concentration of 12 g•100 mL−1. A sequential test involving a flow test and a pasting test (García-Parra et al., 2021; Ortiz-Gómez et al., 2022). An AR 1500 rheometer, TA Instruments, New Castle, United States, equipped with a pasting cell for starch analysis (SPC) were used.
2.2.5. Rheological measurements
The dynamic rheological properties of dough samples were analyzed using an DHR-2 Rheometer (TA instruments, New Castle, DE, United States) with a Peltier plate temperature-controlled system (T = 30°C) and a 25 mm diameter smooth parallel plate geometry. Approximately 3 g of dough was loaded onto the bottom plate and the top plate lowered to a gap of 5 mm. Excess dough was trimmed off and removed from the outer edge of the top plate geometry, and then the dough residue at the bottom plate of the rheometer was cleaned up. Aluminum ring was then added to the outer edge of the dough to prevent drying during measurement, and the dough was allowed to rest for a 2 min equilibration period. For each dough sample, a dynamic oscillatory strain sweep (0.001–100%) (Wang et al., 2023) was conducted at a constant frequency (5 Hz) to determine the linear viscoelasticity range a dynamic oscillatory frequency sweep (0.01–100 Hz) was conducted at a constant strain amplitude (γ = 0.1%) within the linear viscoelastic regime.
2.3. Rheological models and model fitting
The power-law gel model proposed by Sun et al. (2020) was used to fit the storage modulus G’ (Pa) of flour samples from five quinoa cultivars. The Eq. (1) parameters showed how the climatic conditions influenced the quinoa gel strength.
The storage modulus G’(ω) was modeled using the power-law gel model, which uses the constants S and n to represent the gel force and the exponent that characterizes the gel behavior, respectively. The gamma function for 1-n, denoted by Γ (1-n), was used in the model (Sun et al., 2022).
As a second modeling, the four-parameter Burgers model was used, since in previous research (Sun et al., 2020) it managed to satisfactorily fit the frequency-dependent data in the linear viscoelastic region using Eq. (2).
The parameter (n1/R1) represents the Maxwell spring’s immediate elastic response of the material. The parameter (n2/R2) represents the Kelvin-Voigt element’s delayed elastic response of the material. The parameter n1 (viscous) is a Maxwell model constant. The data from each measurement was fitted to the model using GraphPad prism version 6 software.
2.4. Statistical analysis
Analysis of variance (two-way-ANOVA) was determined by Graphpad version 6 software to determine differences in rheological model parameters for quinoa flour gels at 0 h and 24 h. The independent factors were altitude (temperate, cold and warm) and cultivars (Titicaca, Blanca real, Soracá, Pasankalla and Puno). The assumptions of normality and homogeneity were evaluated by the Shapiro–Wilk and Bartlett tests. The multiple analysis was compared with the Tukey HSD test with a level of significance p ≤ 0.05. Correlations were established between the quantitative variables using the Spearman’s correlation and were plotted using the corrplot library. A double-grouping analysis was performed by the Manhattan distance and cluster method by Ward with the gplots and RColorBrewer libraries. The data were analyzed in statistical software R version 3.6.1.
3. Results and discussion
3.1. Approximate chemical composition and IR spectroscopy
The viscoelastic properties of polymers depend on the functional groups that are present in their molecular structure (Yang et al., 2021). These functional groups affect the intermolecular interactions, the degree of crosslinking, and the chain mobility of the polymers (Kontogiorgos, 2017; Ashfaq et al., 2020). As shown in Table 1, the protein content of quinoa grains varied according to the altitude gradient and the cultivar disruption. The starch content, however, was not influenced by this interaction. The altitude gradient is one of the main factors that affects the secondary structures of proteins and the starchy structures. The altitude gradient changes the transmittance of different functional groups, such as C=O; N-H; C-O-C and C-O, which can increase or decrease with the altitude. These functional groups are important for the formation and stability of the gel, as they form hydrogen bonds between the protein structures and with the water molecules around them (Shrestha et al., 2023). In this paper, we will present a multivariate analysis that explores how different functional groups influence the viscoelastic behavior of various polymer samples under different conditions.
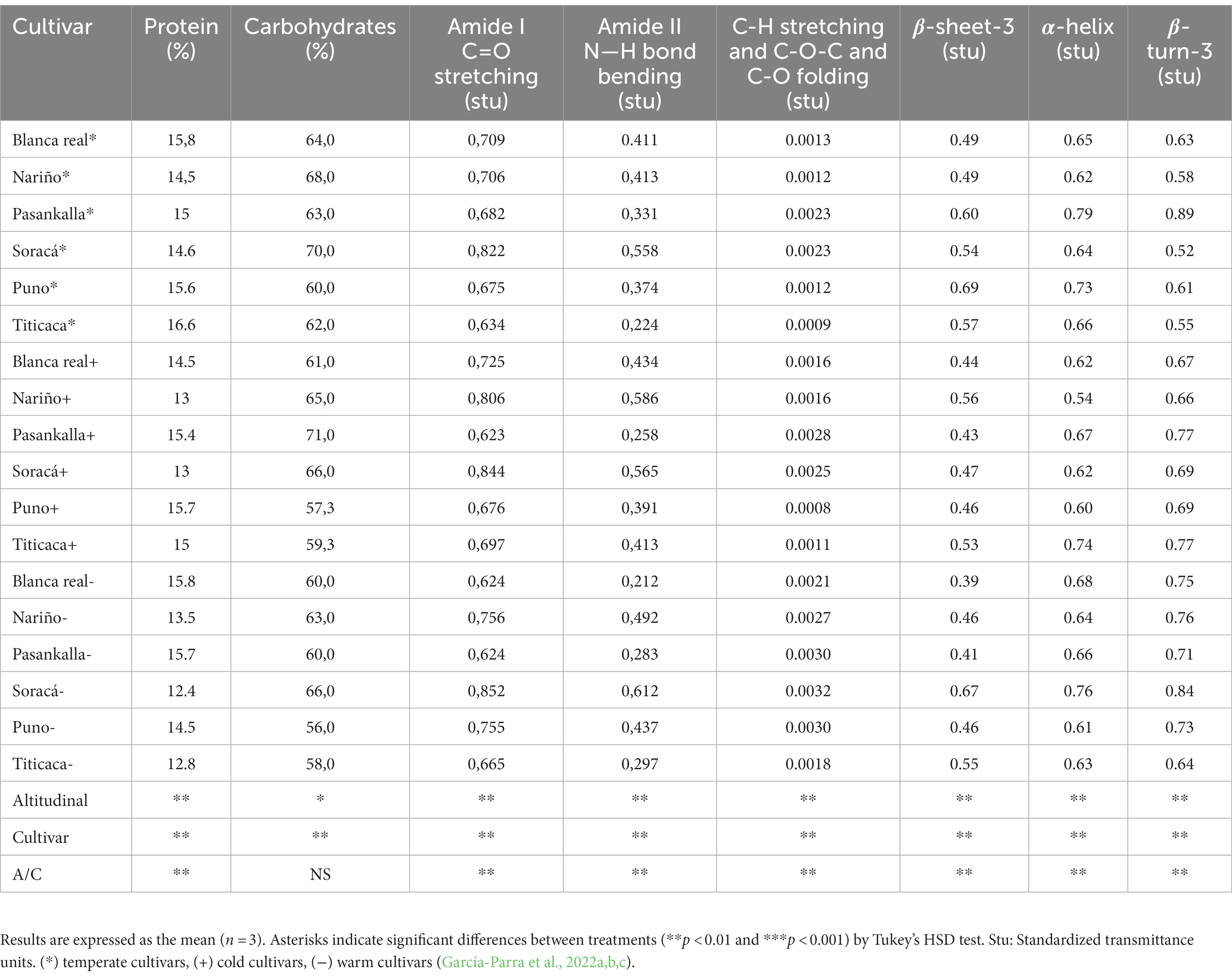
Table 1. Proximal and spectroscopic characteristics of quinoa grains established in different altitudinal gradients.
3.2. The rheological properties
The storage module (G’) of some gels after 0 h and 24 h of storage is plotted against oscillation frequency in Figure 1. The gels showed elastic behavior, with G’ being higher than the viscous module (G”). Warm-climate cultivars have higher elastic component of gels than cold and temperate ones. This means they are more shear-resistant. This may depend on how gel-forming substances react to temperature in different climates. The Titicaca cultivar gels have a low value of 100 Pa in this component, which means they are less deformation-resistant and more syneresis-prone (Yu et al., 2022). Contrarily, Puno gels at 0 and 24 h showed a high storage modulus (G ́), implying more resistance force (Shang et al., 2023), especially for those from warm climates. The gels of the Titicaca cultivar presented the same behavior (Figures 1E,F). The gels from the Puno and Titicaca cultivars showed high stability and elasticity, which are desirable properties for many industrial uses (Garcia-Valle et al., 2021). This information is relevant for understanding the quality and functionality of quinoa gels and their potential applications in food products.
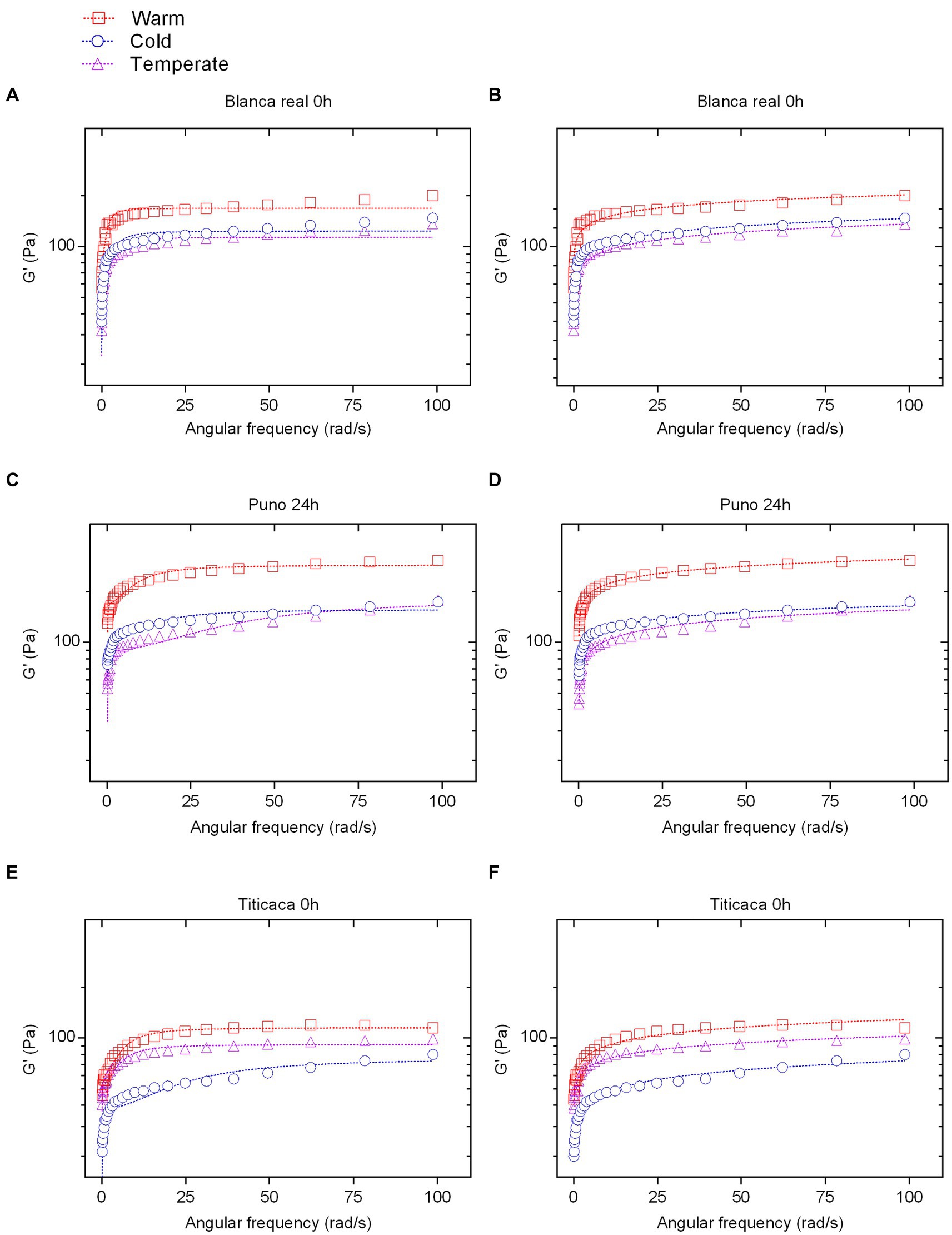
Figure 1. The storage moduli (G´, unfilled symbols) as a function of angular frequency (rad/s) for quinoa flour gel. The graphics (A,C,E) are showing the Burgers model fitting for the G´ of the gels. The graphics (B,D,F) are showing the Power-law model fitting for the G´ of the gels.
This study aimed to assess how gels from various growth and storage conditions behaved viscoelastically. To evaluate the elastic component as a function of frequency in the gels, dynamic oscillatory rheometry at small deformations was performed and the results were compared with rheological models that have been successfully used to describe the viscoelastic behavior of other kinds of flours (Sun et al., 2020, 2022; Kumar et al., 2023). The models used were the power model (Eq. 1) and the Burgers model (Eq. 2). Both models fitted well to the experimental data and showed significant differences among the gels in terms of their elasticity and viscosity.
3.3. Modeling the viscoelastic behavior of quinoa gels by power-law
Using the power model, we can compare the properties of doughs with different strengths and relaxation times. These properties depend on factors such as crop type, altitudes, gel stability time and composition. Figure 2 shows the gel index (n) and gel strength (s) from the power model (R2 > 0.95). These values reflect the quality of the gels and their strength, relaxation, and thermomechanical behavior (Selaković et al., 2021). The power model can also describe the linear viscoelastic behavior of flour doughs with various formulations (Sun et al., 2020, 2022). We analyzed how the five cultivars, and the three climates affected the stability of the gels at 0 and 24 h.
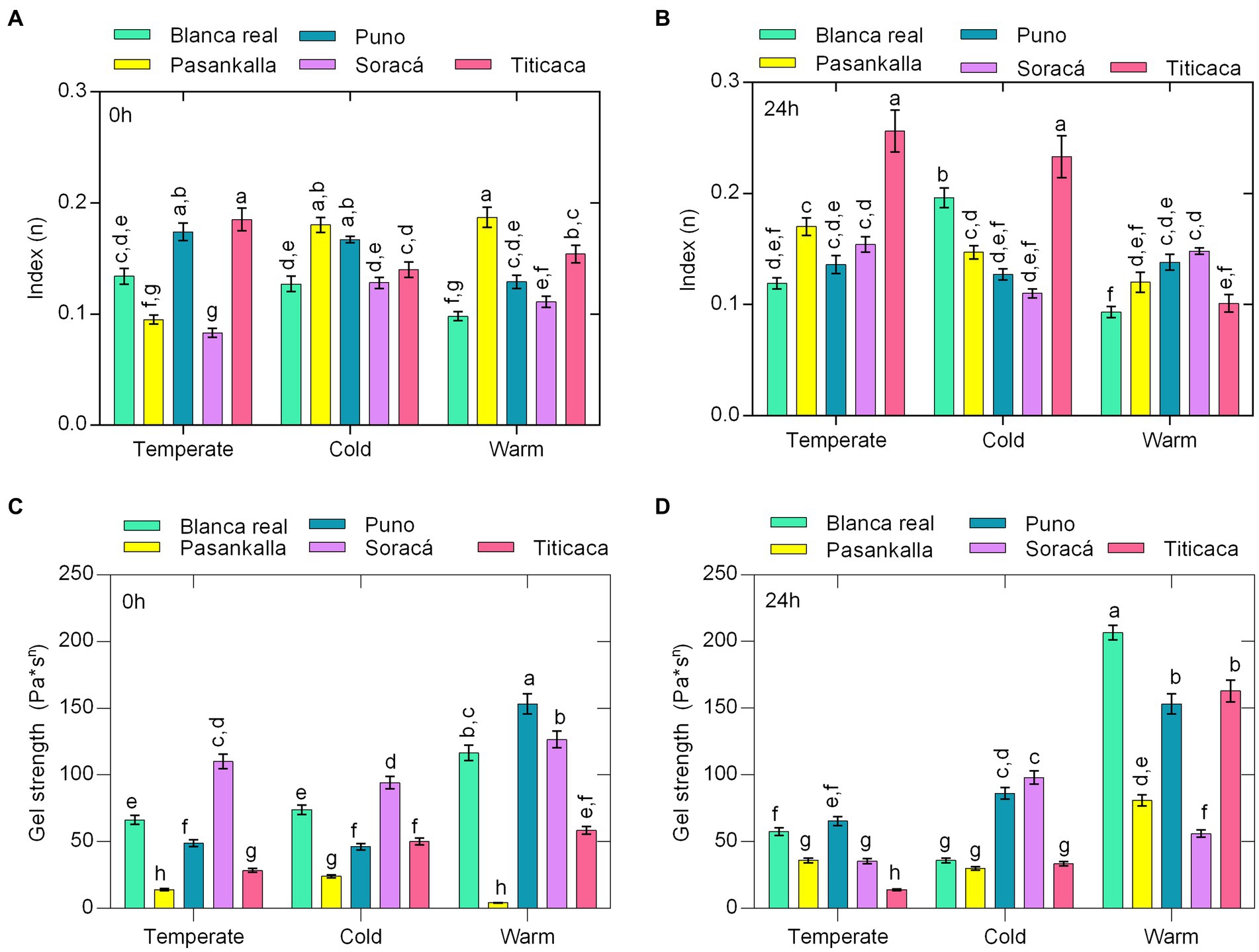
Figure 2. The gel index (n) at 0 h and 24 h (A,B) and the gel strength (s) at 0 h and 24 h (C,D) by Power-law model fitting.
Figures 2A,B illustrates the influence of cultivar and altitude on the n index at 0 (A) and 24 (B) hours of rest, but they are not significant sources of variation. Figure 2A shows the variation of the gel index (n) with respect to cultivar and altitude at the beginning of gel formation and at zero hours. The gel index (n) indicates the relaxation time; gels with shorter relaxation time have a higher index (Sun et al., 2022). Thus, it was determined that between 0 and 0.1 longer relaxation time, between 0.2 and 0.3 shorter relaxation time and the intermediate ones between 0.1 and 0.2. The figure shows that cultivar and altitude affect the gel index (N) of the samples significantly, as some cultivars relax faster than others at different altitudes. The rheological properties of quinoa-based gels depend on various factors, such as quinoa concentration, processing temperature, and cultivar type.
The climate affected the gels from different cultivars in various ways. For instance, Pasankalla gels relaxed faster in warm and cold climates, but slower in temperate ones. Soraca gels behaved similarly. Low index (n) gels were more elastic (G ́>> > G ́́́) and relaxed slower, while high index (n) gels were more viscous (G ́>G ́́́). These differences could relate to the composition and structure of quinoa proteins and starches, which varied by cultivar and environment. Kumar et al. (2023), found similar results for doughs with millet flours.
Figure 2C illustrates how the index (n) changes with cultivar and altitude. Blanca real and Titicaca cultivars show a significant interaction of these factors. They form harder gels in cooler climates and softer gels in warmer climates. This means that the temperature affects the structure and properties of the starch granules in these cultivars. Figures 2C,D displays the variation of the gel strength parameter with different seeding altitudes for the cultivar. For the Blanca Real cultivar., the results show that lower planting temperatures (cool climate) produce weaker gels, which could be due to insufficient hydrogen bonding between the macromolecule chains (Fonseca et al., 2021; Schafranski et al., 2021). This indicates that the seeding altitude influences the quality and stability of the gels made from this cultivar. The power model is a mathematical equation that connects gel strength to the concentration and molecular weight of the gel-forming agent. The gel strength parameter (S) is a measure of the strength of the network created by the particles present and the stored elastic energy, which differs by cultivar. The gel index of cultivars at different altitudes is important for making emulsions and foams because it affects the consistency and rheology of the emulsions and their applications (Fonseca et al., 2021; Sun et al., 2022; Kumar et al., 2023).
One possible explanation for the different behavior of the gels in different climates is the effect of temperature on the molecular structure. In temperate climates, the gelatin molecules may form a stable network that does not change much over time. In warm climates, however, the gelatin molecules may be more flexible and dynamic, allowing them to form stronger bonds with each other as time passes. This could result in a higher gel force and a more resilient gel texture.
The rheological parameters of the gels were analyzed statistically to assess the impact of time and cultivation on them. The viscoelasticity of the gels over time for various cultivation conditions and varieties is shown in Figure 3, which indicates that it was affected by all the factors and their interaction. A fixed factor model was used to obtain a final correlation between the viscoelasticity and the time and cultivation factors. Figures 3A,B show the n and S values for different temperate-climate cultivars. The gel index of Titicaca, Soracá and Pasankalla increased significantly after 24 h of gel resting. However, Pasankalla gel had more stability than Soracá gel, which decreased significantly in gel strength (Figure 3B). The results revealed that cold climate cultivars had lower gel strength and stability than warm climate cultivars after 24 h of rest. In Figures 3C,D (cold climates) the gels of Blanca Real and Titicaca cultivars lost strength after 24 h, while Puno cultivar gel was more stable at 24 h. A very significant effect is observed in the gels of warm climate cultivars especially Pasankalla, Titicaca, Blanca Real with an increase in gel strength and stability during the 24 h of rest. Starch retrogradation is a phenomenon that causes changes in the n and s parameters of gels. The presence of proteins and fibers in the gels can influence the retrogradation process (Roa-Acosta et al., 2020). The gel strength increased due to hydrogen-bonding interactions between starch and proteins-fibers. With less branching degree prevented starch from retrograding over time by blocking the reorganization of amylopectin and amylose double-helices during storage, through hydrogen-bonding interaction with starch (Yang et al., 2019; Fonseca et al., 2021; Yin et al., 2021; Yan et al., 2022).
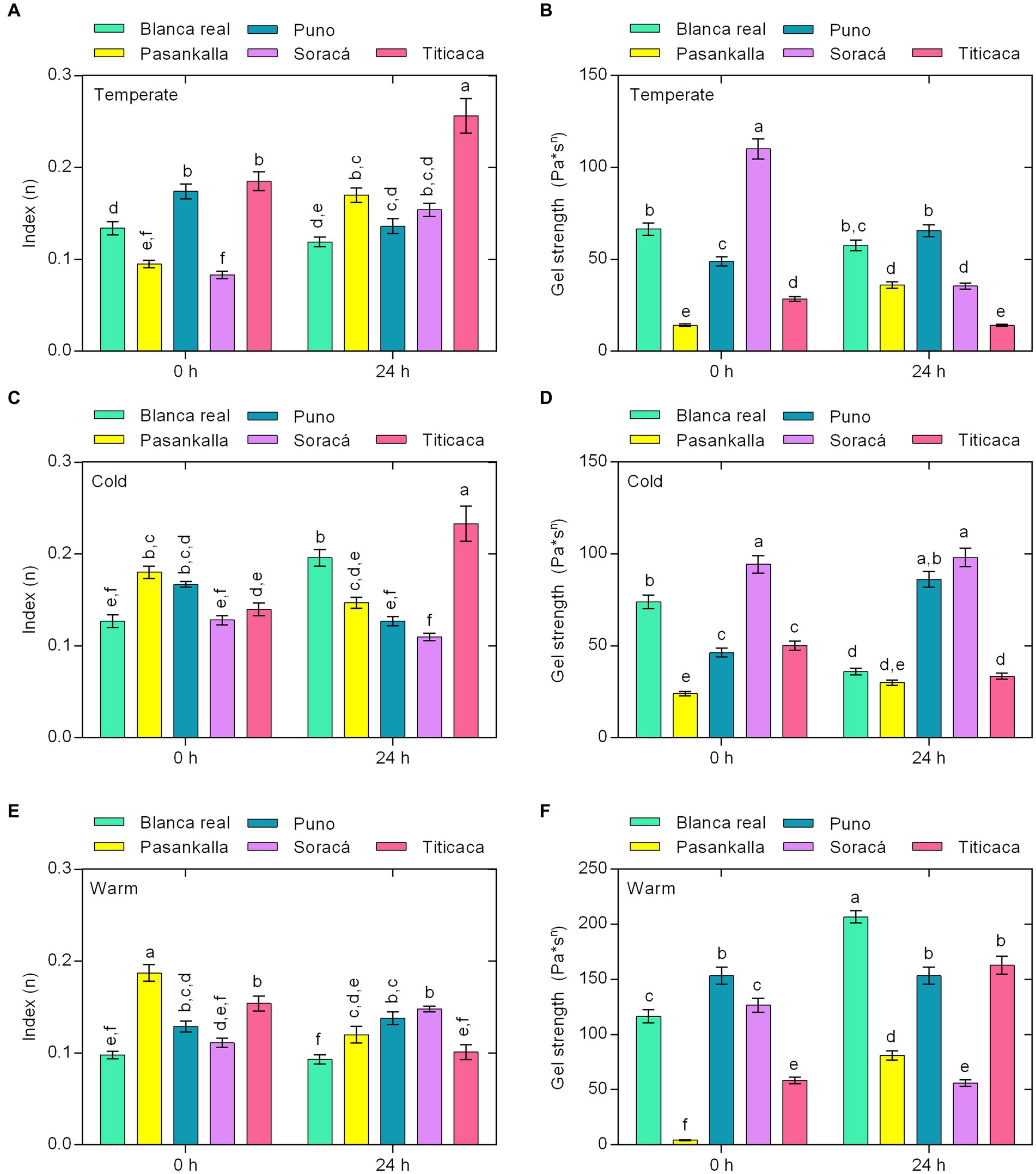
Figure 3. Effect of cultivars and time on rheological parameters: gel index (n) (A,C,E) and the gel strength (B,D,F) by Power-law model fitting.
3.4. Modeling the viscoelastic behavior of quinoa gels by burgers model
One of the important properties of polymeric materials is their relaxation time spectrum, which describes how they respond to small deformations over time. The relaxation time spectrum can be obtained from stress/strain data in the linear viscoelastic region, where the material behaves as a combination of elastic and viscous elements. Several theories have been developed to relate the relaxation time spectrum to different functions, such as the relaxation modulus, the creep compliance, or the storage and loss moduli (Ranalli et al., 2012; Sun et al., 2020). These functions can be used to model the viscoelastic behavior of polymeric materials under various loading conditions (Ranalli et al., 2012). The four-parameter Burgers model can also be fitted to the frequency-dependent data in the linear viscoelastic region using Eq. (2) (Sun et al., 2020).
The Burgers model was used to analyze the frequency-dependent data obtained from an oscillatory frequency test in the linear viscoelastic region. The parameters of the Burgers model are given in Table 1. The parameter (1/R1) indicates the immediate elastic response of the material due to the Maxwell spring. The parameter (n2/R2) indicates the delayed elastic response of the material due to the Kelvin-Voigt element. The parameter n1 (viscous) is a constant from the Maxwell model (Sanz et al., 2023).
The elastic behavior of quinoa gels, measured by the R2 component of Maxwell’s model, shows how well they withstand deformation under applied force. Table 2 shows that climate variation did not change the elasticity (R2) of Pasankalla gels significantly. These gels remained elastic under constant stress. The gels from Blanca Real and Soraca cultivars had higher elasticity and firmness than those from Pasankalla. This could be linked to the protein structure (mainly β-sheet) of these gels, which can form stable hydrogen bonds with themselves and with water molecules (Sun et al., 2022; Wu et al., 2022; Kumar et al., 2023). However, the gels from Puno and Titicaca cultivars lost these properties when grown at low temperatures (Figures 4A–C).
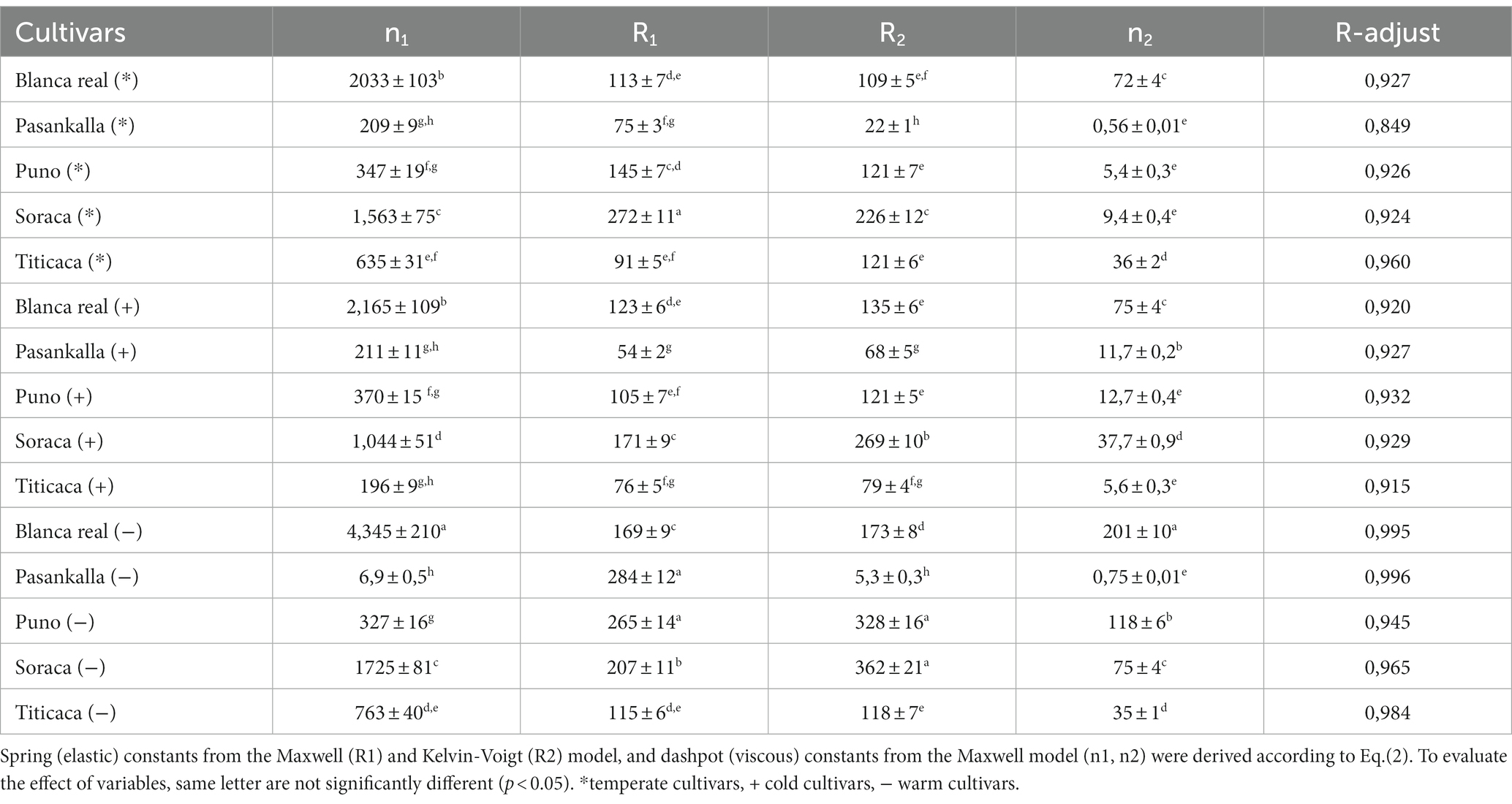
Table 2. Effect of cultivars and altitude on rheological parameters at 0 h by Burgers model fitting.
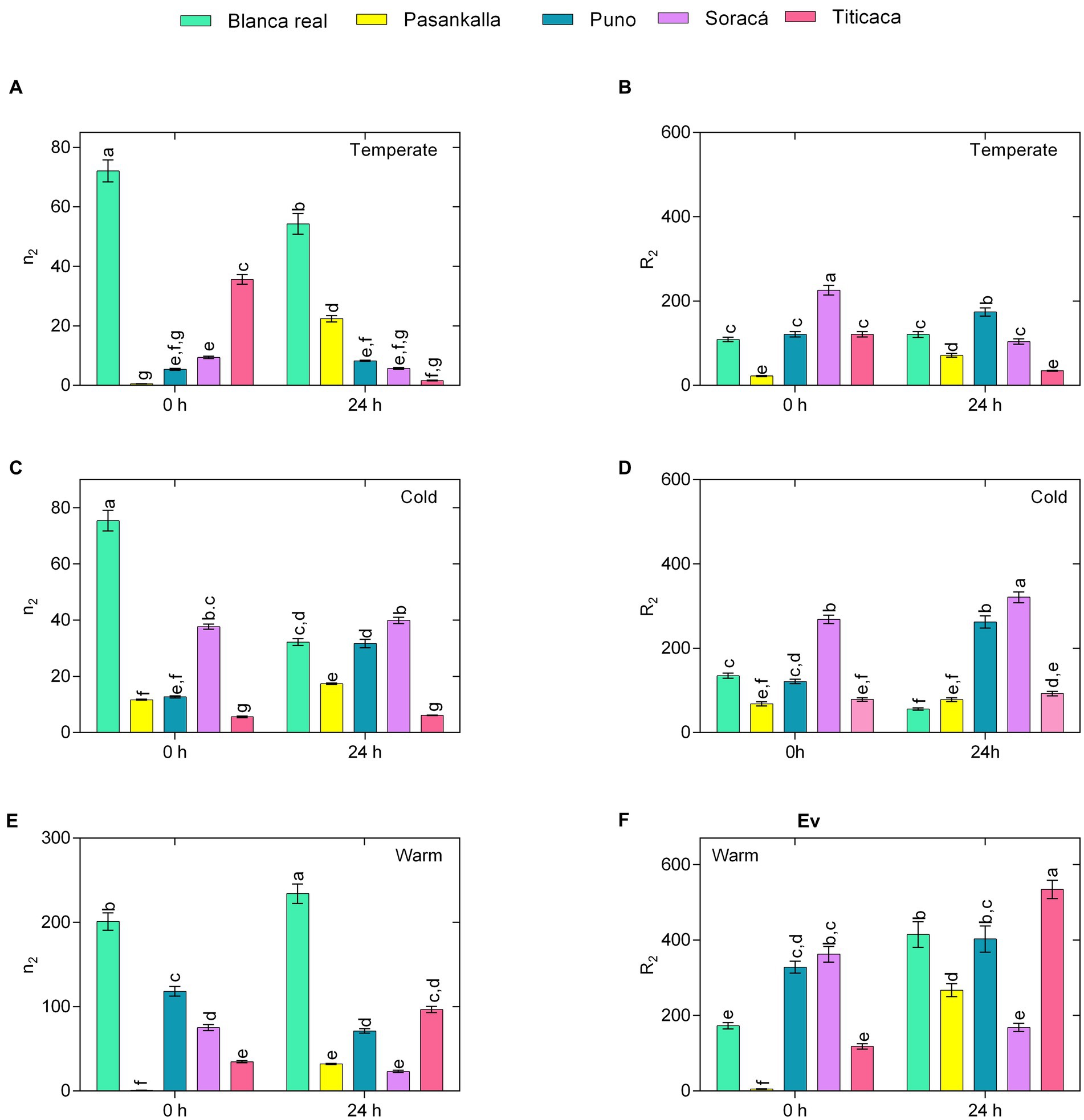
Figure 4. Effect of cultivars and time on rheological parameters: gel index (n) (A–C) and the gel strength (D–F) by Burgers model fitting.
The degree of cross-linking and rigidity of the starch network in the gels is related to the elasticity (Song and Zheng, 2007; Bockstaele et al., 2011). Most gels from warm climates had high elasticity values (Figures 4D–F). However, climates affected cultivars differently. For example, Soraca gels from warm, temperate climates had lower R2 values after 24 h, while cold climates increased R2 values. The opposite was true for Blanca Real, Pasankalla, and Titica gels. Puno gels were slightly affected. These results suggest that quinoa starch molecular structure and interactions depend on the climate of origin, which affects its gelation properties (Figures 5–7).
3.5. Multivariate analysis
Quinoa cultivars evaluated under cold weather conditions were grouped and compared according to their viscoelastic and structural characteristics. In this sense, the cultivars were divided into two groups according to the Heatmap. The first group is made up of the Soraca cultivar., while the second group are the Blanca Real, Titicaca, Pasankalla, and Puno cultivars. The variables were divided into two groups, the first consisting of S0, S1, ratio 1047/996, amide I and II. While the second group by the remaining variables.
The Soracá cultivar presented the largest values for these variables, which marked the difference with respect to the other cultivars, while the low values were obtained for the secondary protein structures such as ß-Turns-3 and α-helix. As well as, for gel indices in initial and final time, which measure the strength and stability of the gel network. These structures are related to the gel formation and texture of products. In addition, it was possible to identify that the variable of greatest variation between cultivars is the gel index at 0 h, being the cultivar Titicaca the most related. According to the correlation matrix, two characteristic behaviors were identified among the variables. There is a negative correlation between n and S parameters at 24 h. A negative correlation was identified between the S parameter at 0 h and the ß-Turns-3 and α-helix structures of the protein.
The results showed that under temperate climate conditions, the cultivars Puno and Blanca Real had similar viscoelastic and structural characteristics, while Soracá had the most distinctive features. The correlation analysis revealed two main groups of variables, one composed of the flow index at time and, ß-Turns-3, α-helix and ß-Sheet-3 structures. The two groups are composed of the other variables. The gel strength at 24 h was the variable that most differentiated the cultivars, with Soracá having the highest value and Titicaca having the lowest. The correlation matrix indicated that the flow index at 0 h was negatively correlated with the variables related to carbohydrates, while the gel strength at 0 h had a slightly negative correlation with some variables related to the protein secondary structure. This correlation became stronger after 24 h, suggesting that the hydration process affected the protein-carbohydrate interactions in the grains.
The results showed that the weather conditions had a significant effect on the properties of the quinoa grains. Thus, under warm weather conditions the cultivars Titicaca and Blanca Real showed greater similarity in relation to the attributes evaluated while the cultivar Soracá remained the one with the greatest difference in viscoelastic and structural terms. In this context, the variables were divided into two groups, the first consisting of gel strength, the flow index at 0 h, and the starches characteristics evaluated, while the other group was made up of the remaining variables. In this sense, the strength of the gel at 24 h remained the variables that determine the discrimination among the cultivars, so according to the correlation matrix, while a negative correlation with all the variables except for the 996 cm-1/1014 cm-1 ratio and the gel strength at time 0 h. However, the latter variable showed a significantly positive correlation with all variables unlike carbohydrate content and flow index at 0 h.
4. Conclusion
This study shows that quinoa grains can adapt their protein, carbohydrate and lipid content and structure to different environmental conditions, which affect their potential uses in the food industry. Choosing the right cultivars and growth conditions is important for the primary production cycle. The secondary structure of proteins and starch, as shown by FT-IR deconvolution, can help to distinguish the composition of quinoa grains, but they are also influenced by the environmental factors. The peaks related to β-sheet, α-helix and β-turns in proteins and the bands corresponding to crystalline and amorphous structures in starch changed significantly among the treatments. However, the 1,075 cm−1 band was stable across all treatments and could be used as a quality indicator for quinoa products. The viscoelastic behavior of gels depends on the structural conformation of their components, such as proteins and starch. These components give rigidity and elasticity to the gels. The structural conformation can vary depending on the environmental conditions and the phenotypic characteristics of the components. We performed oscillatory tests on different samples of gels and modeled them using the power gamma function and the Burger function. These functions represent the elastic and viscous modules of the gels, respectively. We also evaluated the relaxation properties of the gels by using oscillatory tests.
Data availability statement
The raw data supporting the conclusions of this article will be made available by the authors, without undue reservation.
Author contributions
MP-M, DR-A, and MG-P: conceptualization, methodology, software and investigation, and formal analysis. All authors have read and agreed to the published version of the manuscript and contributed to the article and approved the submitted version.
Funding
The authors express gratitude to the Universidad del Cauca, project ID 5637.
Conflict of interest
The authors declare that the research was conducted in the absence of any commercial or financial relationships that could be construed as a potential conflict of interest.
Publisher’s note
All claims expressed in this article are solely those of the authors and do not necessarily represent those of their affiliated organizations, or those of the publisher, the editors and the reviewers. Any product that may be evaluated in this article, or claim that may be made by its manufacturer, is not guaranteed or endorsed by the publisher.
References
Ashfaq, A., Clochard, M. C., Coqueret, C., Dispenza, C., Driscoll, M. S., Ulański, P., et al. (2020). Polymerization reactions and modifications of polymers by ionizing radiation. Polymers 12:2877. doi: 10.3390/polym12122877
Bockstaele, F., Leyn, I., Eeckhout, M., and Dewettinck, K. (2011). Non-linear creep-recovery measurements as a tool for evaluating the viscoelastic properties of wheat flour dough. J. Food Eng. 107, 50–59. doi: 10.1016/j.jfoodeng.2011.06.001
Contreras-Jiménez, B., Torres-Vargas, O. L., and Rodríguez-García, M. E. (2019). Physicochemical characterization of quinoa (Chenopodium quinoa) flour and isolated starch. Food Chem. 298:124982. doi: 10.1016/j.foodchem.2019.124982
Felix, M., Camacho-Ocaña, Z., López-Castejón, M. L., and Ruiz-Domínguez, M. (2021). Rheological properties of quinoa-based gels. An alternative for vegan diets. Food Hydrocoll. 120:106827. doi: 10.1016/j.foodhyd.2021.106827
Fonseca, L. M., Halal, S. L. M., Guerra-Días, A. R., and Rosa-Zavareze, E. (2021). Physical modification of starch by heat-moisture treatment and annealing and their applications: a review. Carbohydr. Polym. 274:118665. doi: 10.1016/j.carbpol.2021.118665
García-Parra, M. Á., Polo-Muñoz, M. P., Nieto-Calvache, J. E., Agudelo-Laverde, L. M., and Roa-Acosta, D. F. (2022a). Physicochemical, rheological and structural properties of flours from six quinoa cultivars grown in Colombia. Front. Sustain. Food Syst. 6. doi: 10.3389/fsufs.2022.936962
Garcia-Parra, M. Á., Roa-Acosta, D. F., Bravo-Gomez, J. E., Hernández-Criado, J. C., and Villada-Castillo, H. S. (2022b). Effects of altitudinal gradient on physicochemical and rheological potential of quinoa cultivars. Front. Sustain. 6:862238. doi: 10.3389/fsufs.2022.862238
García-Parra, M., Roa-Acosta, D., and Bravo-Gómez, J. E. (2022c). Effect of the altitude gradient on the physiological performance of quinoa in the central region of Colombia. Agronomy (Basel, Switzerland).12:2112. doi: 10.3390/agronomy12092112
García-Parra, M. Á., Roa-Acosta, D. F., García-Londoño, V., Moreno-Medina, B., and Bravo-Gómez, J. E. (2021). Structural characterization and antioxidant capacity of quinoa cultivars using techniques of FT-MIR and UHPLC/ESI-Orbitrap MS spectroscopy. Plan. Theory 10:1. doi: 10.3390/plants10102159
García-Salcedo, A. J., Torres-Vargas, O. L., Del Real, A., Contreras-Jiménez, B., and Rodríguez-García, M. E. (2018). Pasting, viscoelastic, and physicochemical properties of chia (Salvia hispanica L.) flour and mucilage. Food Struct. 16, 59–66. doi: 10.1016/j.foostr.2018.03.004
Garcia-Valle, D. E., Agama-Acevedo, E., Del Carmen Núñez, S. M., Alvarez-Ramirez, J., and Bello-Pérez, L. A. (2021). Extrusion pregelatinization improves texture, viscoelasticity and in vitro starch digestibility of mango and amaranth flours. J. Funct. Foods 80:104441. doi: 10.1016/j.jff.2021.104441
Huang, R., Huang, K., Guan, X., Li, S., Cao, H., Zhang, Y., et al. (2021). Effect of defatting and extruding treatment on the physicochemical and storage properties of quinoa (Chenopodium quinoa Willd) flour. LWT 147:111612. doi: 10.1016/j.lwt.2021.111612
Kontogiorgos, V. (2017). Linear viscoelasticity of gluten: decoupling of relaxation mechanisms. J. Cereal Sci. 75, 286–295. doi: 10.1016/j.jcs.2017.04.001
Kumar, P., Kaur, C., Rudra, S. G., and Arora, B. (2023). Extruded finger millet improves rheology and quality of composite maize flatbread. Int. J. Gastron. Food Sci. 31:100638. doi: 10.1016/j.ijgfs.2022.100638
Lesjak, J., and Calderini, D. F. (2017). Increased night temperature negatively affects grain yield, biomass and grain number in Chilean quinoa. Front. Plant Sci. 8:352. doi: 10.3389/fpls.2017.00352
Miranda, M., Vega-Gálvez, A., Martínez, E., López, J., Marín, R., Aranda, M., et al. (2013). Influence of contrasting environments on seed composition of two quinoa genotypes: nutritional and functional properties. Chil. J. Agric. Res. 73, 108–116. doi: 10.4067/S071858392013000200004
Muñoz-Pabon, K. S., Parra-Polanco, A. S., Roa-Acosta, D. F., Hoyos-Concha, J. L., and Bravo-Gómez, J. E. (2022). Physical and paste properties comparison of four snacks produced by high protein quinoa flour extrusion cooking. Front. Sustain. Food Syst. 6:2224. doi: 10.3389/fsufs.2022.852224
Ortiz-Gómez, V., Nieto-Calvache, J. E., Roa-Acosta, D. F., Solanilla-Duque, J. F., and Bravo-Gómez, J. E. (2022). Preliminary characterization of structural and rheological behavior of the quinoa Hyperprotein-defatted flour. Front. Sustain. Food Syst. 6:2332. doi: 10.3389/fsufs.2022.852332
Ranalli, N., Andrés, S., and Califano, A. N. (2012). Physicochemical and rheological characterization of “dulce de leche”. J. Texture Stud. 43, 115–123. doi: 10.1111/j.1745-4603.2011.00321.x
Roa-Acosta, D. F., Bravo-Gómez, J. E., Solanilla-Duque, J. F., Zuñiga-Galindez, J. Z., and Martínez-Cruz, J. A. (2022). Antioxidant potential of extruded snacks enriched with hyper-protein quinoa flour and vegetable extracts. Food Sci. Technol. 42:74621. doi: 10.1590/fst.74621
Roa-Acosta, D. F., Buera, M. P., Tolaba, M. P., and Santagapita, P. R. (2017). Encapsulation and stabilization of β-carotene in Amaranth matrices obtained by dry and wet assisted ball milling. Food Bioprocess Technol. 10, 512–521. doi: 10.1007/s11947-016-1830-y
Roa-Acosta, D. F., Solanilla-Duque, J. F., Agudelo-Laverde, L. M., Villada, H. S., and Tolaba, M. P. (2020). Structural and thermal properties of the amaranth starch granule obtained by high-impact wet milling. Int. J. Food Eng. 16. doi: 10.1515/ijfe-2020-0024
Rodríguez-Gómez, M. J., Prieto, J. M., Crúz-Sobrado, V., and Calvo-Magro, P. (2021). Nutritional characterization of six quinoa (Chenopodium quinoa Willd) varieties cultivated in southern Europe. J. Food Compos. Anal. 99:103876. doi: 10.1016/j.jfca.2021.103876
Sanz, T., Salvador, A., and Hernández, M. (2023). Creep–recovery and oscillatory rheology of flour-based systems. Adv. Food Rheol. Appl. 459-478. doi: 10.1016/b978-0-12-823983-4.00019-4
Schafranski, K., Ito, V. C., and Lacerda, L. G. (2021). Impacts and potential applications: a review of the modification of starches by heat-moisture treatment (HMT). Food Hydrocoll. 117:106690. doi: 10.1016/j.foodhyd.2021.106690
Selaković, A., Nikolić, I., Dokić, L., Šoronja-Simović, D., Šimurina, O., Záhorec, J., et al. (2021). Enhancing rheological performance of laminated dough with whole wheat flour by vital gluten addition. LWT 138:110604. doi: 10.1016/j.lwt.2020.110604
Shang, J., Zhao, B., Liu, C., Li, L., Hong, J., Liu, M., et al. (2023). Impact of wheat starch granule size on viscoelastic behaviors of noodle dough sheet and the underlying mechanism. Food Hydrocoll. 134:108111. doi: 10.1016/j.foodhyd.2022.108111
Shrestha, S., Hag, L., Haritos, V., and Dhital, S. (2023). Rheological and textural properties of heat-induced gels from pulse protein isolates: lentil, mungbean and yellow pea. Food Hydrocoll. 143:108904. doi: 10.1016/j.foodhyd.2023.108904
Song, Y., and Zheng, Q. (2007). Dynamic rheological properties of wheat flour dough and proteins. Trends Food Sci. Technol. 18, 132–138. doi: 10.1016/j.tifs.2006.11.003
Sun, X., Koksel, F., Nickerson, M. T., and Scanlon, M. J. (2020). Modeling the viscoelastic behavior of wheat flour dough prepared from a wide range of formulations. Food Hydrocoll. 98:105129. doi: 10.1016/j.foodhyd.2019.05.030
Sun, X., Pei, F., and Fang, Y. (2022). The effects of hydrocolloids on the thermomechanical, viscoelastic and microstructural properties of whole wheat flour dough. Food Chem. 370:130976. doi: 10.1016/j.foodchem.2021.130976
Wang, Y., Guo, J., Wang, C., Li, Y., Bai, Z., Luo, D., et al. (2023). Effects of konjac glucomannan and freezing on thermal properties, rheology, digestibility and microstructure of starch isolated from wheat dough. Food Sci. Technol. 177:114588. doi: 10.1016/j.lwt.2023.114588
Wu, Y., Ye, G., Li, X., Wang, L., Liu, Y., Tan, B., et al. (2022). Comparison of quality characteristics of six reconstituted whole wheat flour with different modified bran. LWT 153:112543. doi: 10.1016/j.lwt.2021.112543
Yan, W., Zhang, M., Zhang, M., Yadav, M. P., Jia, X., and Yin, L. (2022). Effect of wheat bran arabinoxylan on the gelatinization and long-term retrogradation behavior of wheat starch. Carbohydr. Polym. 291:119581. doi: 10.1016/j.carbpol.2022.119581
Yang, H., Yang, S., Kong, J., Dong, A., and Yu, S. (2015). Obtaining information about protein secondary structures in aqueous solution using Fourier transform IR spectroscopy. Nat. Protoc. 10, 382–396. doi: 10.1038/nprot.2015.024
Yang, L., Yang, L., and Lowe, R. L. (2021). A viscoelasticity model for polymers: time, temperature, and hydrostatic pressure dependent young's modulus and poisson's ratio across transition temperatures and pressures 157:103830. doi: 10.1016/j.mechmat.2021.103839,
Yang, C., Zhong, F., Douglas Goff, H., and Li, Y. (2019). Study on starch-protein interactions and their effects on physicochemical and digestible properties of the blends. Food Chem. 280, 51–58. doi: 10.1016/j.foodchem.2018.12.028
Yin, X., Zheng, Y., Kong, X., Cao, S., Chen, S., Liu, D., et al. (2021). RG- І pectin affects the physicochemical properties and digestibility of potato starch. Food Hydrocoll. 117:106687. doi: 10.1016/j.foodhyd.2021.106687
Keywords: power-law gel, Burgers model, quinoa, rheology, gels
Citation: Polo-Muñoz MP, Garcia-Parra M& and Roa-Acosta DF (2023) Viscoelastic behavior of gels obtained from five cultivars of quinoa at altitude gradient. Front. Sustain. Food Syst. 7:1222277. doi: 10.3389/fsufs.2023.1222277
Edited by:
Samuel Ayofemi Olalekan Adeyeye, Hindustan University, IndiaReviewed by:
Asad Mohammad Amini, University of Kurdistan, IranHulya Cakmak, Hittite University, Türkiye
Copyright © 2023 Polo-Muñoz, Garcia-Parra and Roa-Acosta. This is an open-access article distributed under the terms of the Creative Commons Attribution License (CC BY). The use, distribution or reproduction in other forums is permitted, provided the original author(s) and the copyright owner(s) are credited and that the original publication in this journal is cited, in accordance with accepted academic practice. No use, distribution or reproduction is permitted which does not comply with these terms.
*Correspondence: María Paula Polo-Muñoz, bWFyaWFwb2xvQHVuaWNhdWNhLmVkdS5jbw==