- 1Department of Population Health and Reproduction, School of Veterinary Medicine, University of California, Davis, Davis, CA, United States
- 2Center for Animal Disease Modelling and Surveillance CADMS, Department of Medicine and Epidemiology, School of Veterinary Medicine, University of California, Davis, Davis, CA, United States
- 3Western Center for Food Safety, University of California, Davis, Davis, CA, United States
- 4Environmental Microbial & Food Safety Laboratory, U.S. Department of Agriculture, Agricultural Research Service, Beltsville, MD, United States
- 5Department of Soil Water and Climate, Southwest Research and Outreach Center, University of Minnesota, St. Paul, MN, United States
- 6University of Maine Cooperative Extension, Waldoboro, ME, United States
- 7Department of Agriculture, Food and Resource Sciences, University of Maryland Eastern Shore, Princess Anne, MD, United States
- 8Produce Safety Alliance, Department of Food Science, Cornell University, Geneva, NY, United States
- 9The Organic Center, Washington, DC, United States
Introduction: Biological soil amendments, including raw or untreated manure, are currently used to improve soil fertility, especially in organic operations that prohibit use of synthetic fertilizers. However, addition of untreated manure may pose a risk of contamination of fresh produce by pathogens of public health significance, including Listeria monocytogenes. Organic growers follow United States Department of Agriculture (USDA) National Organic Program regulations for raw manure use, which stipulate that harvest should commence no earlier than 90- or 120-days post-application, depending on direct contact between the edible portion of the produce and the soil. To inform the protection that such time-intervals provide, this study explored the farm-level risk factors associated with L. monocytogenes prevalence in USDA-certified organic farm soils amended with untreated manures.
Methods: A longitudinal, multi-regional study was conducted on 19 farms in four states (California, Minnesota, Maine, and Maryland) over two growing seasons (2017 and 2018). Untreated manure, soil, irrigation water, and produce samples were collected and cultured for L. monocytogenes. Mixed effect logistic regression was used to investigate risk factors associated with L. monocytogenes prevalence in soil.
Results and Discussion: Results showed that multiple factors influenced the odds of a soil-positive sample, including temporal [year (OR = 0.19), sampling day (OR = 0.09–0.48)] and weather-related [temperature range (OR = 0.48)] variables, manure characteristics [season of application (OR = 0.04, summer), presence of L. monocytogenes (OR = 2.89) and other pathogens in manure (OR = 5.24)], farm management factors [water source (OR = 2.73, mixed), number of year-round staff (OR = 0.02)], and soil characteristics [concentration of generic Escherichia coli (OR = 1.45), moisture (OR = 0.46), organic matter (OR = 7.30), nitrate (OR = 3.07), potassium (OR = 0.09) and calcium (OR = 2.48)]. This study highlights the complexity of L. monocytogenes prevalence in soil and contributes science-based metrics that may be used when determining risk-mitigation strategies for pathogen contamination.
1. Introduction
Globally, organic agriculture has experienced substantial growth in recent decades, with growth rates of 20% per year in some countries (Rana and Paul, 2017; Willer and Lernoud, 2017). The United States (US) ranks third in worldwide organic industry production, accounting for approximately 2.2 million hectares of organic growing land and profits of nearly $43.3 billion per year (Willer and Lernoud, 2017). The rapid increase in consumer demand and consumption of organic produce has been attributed to the perceived benefits, both nutritionally and environmentally, of organic farming compared to conventional farming (Lotter, 2003).
Organic farming is based on the principles of health, ecology, fairness, and care, and focuses on sustainable practices (Luttikholt, 2007). Therefore, regulations prohibit organic farms from using synthetic fertilizers and pesticides, and organic producers must find other ways to supplement their fields. Application of biological soil amendments of animal origin (BSAAOs) and other nutrient and crop management techniques, such as the use of cover crops, crop rotation, and intercropping with nitrogen-fixing crops are frequently utilized by organic farmers (Rosen and Bierman, 2005).
As defined by the US Food and Drug Administration (FDA), BSAAOs are soil amendments, partially or wholly composed of animal waste and other byproducts, including, but not limited to, raw or untreated manure, animal carcasses, swine slurry, and poultry litter (FDA, 2015b). Use of these natural alternatives has numerous benefits for the soil quality, including increasing organic matter and nutrients in soil, water retention, drainage, and aeration (Rosen and Allan, 2007). However, many of the animal sources of such amendments (e.g., cattle, sheep, and poultry) are known sources of foodborne pathogens that are often linked to fresh produce outbreaks, such as Escherichia (E.) coli O157:H7, non-O157 Shiga toxin-producing E. coli (STEC), Salmonella spp., and Listeria (L.) monocytogenes (Whipps et al., 2008; Olaimat and Holley, 2012). Therefore, while the use of BSAAOs offers multiple benefits, it also risks introduction of foodborne illness pathogens into the field, creating potential for contamination of produce (Nightingale et al., 2004). Currently, certified organic producers follow US Department of Agriculture (USDA)-National Organic Program (NOP) standards for incorporation of raw manure to mitigate the contamination risk, and these differ between crops whose edible portions do and do not come into direct contact with the soil amended with raw manure (FDA, 2015b; USDA NOP, 2018). For crops not directly in contact with soil, e.g., tomatoes and peppers, a 90-day minimum interval between raw or incompletely treated manure incorporation and the crop harvest is required. In contrast, a 120-day minimum interval is required for crops that directly contact the soil, e.g., leafy greens and root vegetables (USDA NOP, 2018). However, these intervals are based on the production cycle and environmental factors, and do not take into account other on-farm factors that may contribute to pathogen survival and potential crop contamination. A multitude of factors, including pathogen, animal manure, and soil types, as well as environmental conditions, are known to influence the persistence of pathogens in the soil, and their potential to contaminate fresh produce (Harris et al., 2003; Hutchison et al., 2005; Erickson et al., 2014; Sharma et al., 2019; Limoges et al., 2022). There is currently minimal research to support the NOP interval guidelines, and the Produce Safety Rule (PSR) of the Food Safety Modernization Act (FSMA) has not specified its own interval standards for raw manure; instead, the FDA is waiting until more data has been collected to make a final ruling (FDA, 2015a, 2018).
The wide natural distribution of L. monocytogenes across environments and hosts provides extensive opportunities for transmission to foods, including fresh produce specialty crops that are consumed without cooking. L. monocytogenes has been isolated in non-agricultural and agricultural environments (Linke et al., 2014; Harrand et al., 2020; Liao et al., 2021). For example, manure contaminated with L. monocytogenes used to fertilize a field can introduce the pathogen into the soil (Jiang et al., 2004). Additionally, studies have shown that L. monocytogenes may survive at detectable levels in soil for 128 days after manure application, allowing for potential contamination of produce by soil cultivation, splash events or contaminated water (Weller et al., 2016; Harrand et al., 2020). Foodborne illness outbreaks caused by L. monocytogenes, while relatively uncommon, can result in high morbidity and mortality, especially in pregnant, immunocompromised, and elderly individuals, with case fatality rates reaching approximately 20% (CDC, 2013; Pohl et al., 2019). While many listeriosis outbreaks are linked to contaminated animal products (e.g., deli meats, soft cheeses), several outbreaks have involved fresh produce as the vehicle of transmission in the US over the past decade, including cantaloupes in 2011, bean sprouts, caramel apples, nectarines, and peaches in 2014, fresh packaged salads in 2016 and 2021, and enoki mushrooms in 2020 and 2022 (CDC, 2013, 2023; Garner and Kathariou, 2016; Townsend et al., 2021).
Studies have shown that environmental factors have a positive association with L. monocytogenes detection and isolation (e.g., soil, water, vegetation) (Ivanek et al., 2009; Harrand et al., 2020). Additionally, adjacent land use for livestock pastures was associated with the prevalence of L. monocytogenes in agricultural fields (Harrand et al., 2020). Manure application, soil cultivation, and wildlife intrusion have also been shown to increase the risk of L. monocytogenes contamination of produce fields (Strawn et al., 2013b). However, many studies reporting on such risks were conducted on conventional farms and/or in controlled trials, often missing organic-specific variables, such as management practices and environmental conditions of organic production systems.
Longitudinal, multi-regional studies are necessary to establish the complex time-intervals between untreated manure application and harvest, and to identify specific risk factors associated with persistence of L. monocytogenes on organic farms using untreated manure-based soil amendments. In order to provide a more complete understanding and approach to risk mitigation and best practices for the use of untreated manure (i.e., manure that has not been processed to completion in a scientifically valid manner to reduce the presence of microorganisms of public health significance) in organic production, this study focused on a broad range of variables that may impact contamination risk in various regions, and was informed by multiple disciplines. Specifically, the objective of this study was to assess farm-level risk factors associated with L. monocytogenes prevalence in soils amended with untreated manure on USDA-NOP certified organic farms in four regions of the US.
2. Materials and methods
2.1. Study design and field sample collection
A longitudinal, multi-regional field study was conducted over 21 months (two growing seasons) from 2017 (March to December) to 2018 (February to December) on a total of 19 USDA-NOP-certified farms located in California (CA, 9), Minnesota (MN, 4), Maine (ME, 5), and Maryland (MD, 1). More details on study design and farm enrollment can be found in Ramos et al. (2021). Briefly, farms from the aforementioned four states were recruited through personal invitation, email, phone, or personal visit. Enrollment was based on the following criteria: (1) certified USDA-NOP fresh produce farm, (2) use of untreated manure in produce fields, (3) grow one or more of the following crops: leafy greens, root vegetables, and/or fruits, and (4) willingness to participate and provide biological samples and information about farm management practices and location. A total of 16 farms were enrolled in 2017, and 18 farms were enrolled in 2018, with 15 participating in both years.
2.2. Questionnaire design
A questionnaire was created to collect data from each farm about farm practices and environmental characteristics. The questionnaire included the following sub-categories: (1) farm demographics and information (e.g., farm size, number of staff, third-party certifications, marketing outlets, workers’ training); (2) crop production (e.g., crop types, previous plant/soil diseases), (3) soil characteristics and surrounding landscape use (e.g., soil type, soil testing, previous use of field, use for livestock/poultry, surrounding landscape use), (4) fertilizer use [e.g., manure, compost, source, storage, application method, rate and frequency, in-house versus commercial, other biological soil amendments, location of BSAAO relative to the crops (down/up-hill)]; (5) agricultural water (e.g., source, irrigation type, microbiological testing, irrigation frequency), and (6) management practices (e.g., tillage practices, crop rotation, livestock/poultry in property and proximity to livestock, crop-livestock integration, presence of domestic animals, presence of wildlife, evidence of animal feces in fields) (Supplementary material S1). Additionally, during each monthly visit, various factors were recorded, including domestic and wild animal intrusion, abnormal field conditions, irrigation frequency, and produce type and growth stage.
2.3. Sample collection
Samples were collected as previously described by Ramos et al. (2021). Participating farms were sampled monthly for a period of up to 180 days over two growing seasons. Before manure application, 5 untreated composite manure samples were collected. Each composite manure sample was collected from 5 separate spots and different depths in the manure pile (90–100 g total per composite sample), using a shovel and a sterile scoop (Fisher Scientific, Hampton, NH). Baseline soil samples were collected prior to manure application (d0A, baseline). For each manure and produce type, four composite soil samples (five subsamples each) were collected after manure incorporation and monthly (d0B, 30, 60, 90, 120, 150, 180 days post-application), for a total of seven collection times per crop cycle. Soil samples were collected using a stainless-steel soil core sampling probe (2.54 cm diameter) disinfected with 70% ethanol between samples and 15.2 cm deep from the soil surface (Ramos et al., 2021). Four fresh produce samples (approximately 100 g for leafy greens, and 150–200 g for fruits and root vegetables) were collected twice or three times depending on availability (d90, 120 and 150 days), using sterile scissors and aseptically transferred into sterile Whirl-Pak bags (Nasco, Modesto, CA). Agricultural water was collected once at each farm during each growing season as previously described (Ramos et al., 2021). Briefly, water samples were collected directly into a sterile Nalgene bottle (Thermo Scientific, Rochester, NY) by hand or with a sampling pole. A maximum of two irrigation water samples (1 L each) were collected from different sources (e.g., agricultural well, pond, reservoir, lagoon, or creek). All samples were transported or shipped to the laboratory on ice in insulated containers and were processed within 48 h of collection.
Over the two growing seasons, a total of 3,260 samples, including 2,461 soil, 233 manure (i.e., untreated manure from cattle, horse, poultry, or small ruminants), 527 fresh produce (leafy greens, root vegetables, or fruits), and 39 irrigation water samples were collected from the farms.
2.4. Sample preparation
Samples were prepared as previously described by Ramos et al. (2021). In this manuscript, we will describe only the laboratory analyses for isolation of L. monocytogenes and generic E. coli. Enrichment, isolation, and identification of non-O157 STEC, E. coli O157:H7, and Salmonella spp., are detailed in Ramos et al. (2021). To isolate and identify L. monocytogenes from soil and manure, samples were diluted 1:10 and 25 g of each sample was weighed and transferred to a 24-oz Whirl-Pak bag (Nasco, Modesto, CA) of 225 mL of Listeria Enrichment Broth (LEB). The enrichments were homogenized by manually massaging the bags for 1 min before incubation. Prepared LEB enrichments were incubated at 30°C for 18 h with 100 rpm shaking in a Multitron programmable shaking incubator and then kept at 6°C without shaking until further use for L. monocytogenes isolation.
To isolate and identify generic E. coli from the aforementioned soil, samples were diluted 1:10 and 30 g of each sample was weighed and transferred to a 24-oz Whirl-Pak bag (Nasco, Modesto, CA) of 270 mL of Tryptic Soy Broth (TSB). The enrichments were homogenized by manually massaging the bags for 1 min before incubation. Prepared TSB enrichments were incubated at 25°C for 2 h, and then at 42°C for 8 h with 100 rpm shaking in a Multitron programmable shaking incubator and then kept at 6°C without shaking until further use for generic E. coli isolation.
2.5. Bacterial detection and isolation of Listeria monocytogenes in soil and manure samples
Qualitative confirmation of L. monocytogenes was performed following the procedure outlined by Ramos et al. (2021). In summary, automated immunomagnetic separation was performed on the aforementioned LEB enrichment as previously described by Cooley et al. (2014) to concentrate any L. monocytogenes cells present. The resulting washed beads were transferred to Brilliance Listeria Agar (BLA; Oxoid, Hants, United Kingdom) plates supplemented with Brilliance Listeria Differential Supplement (Oxoid, Hants, United Kingdom) and Brilliance Listeria Selective Supplement (Oxoid, Harts, United Kingdom), and to 5 mL of Fraser broth (BD, Sparks, MD, United States) in 30 μL and 100 μL aliquots, respectively. The BLA plates and Fraser broth tubes were incubated at 37°C for 48 h. A maximum of 4 presumptive Listeria colonies on the BLA plate were re-streaked to a secondary, and eventually a tertiary BLA plate. If the Fraser broth turned black, a full 10 μL loop was transferred to a BLA plate where it would then follow the isolation steps for the BLA plates outlined above. All isolated presumptive L. monocytogenes colonies were streaked onto Tryptic Soy Agar plates that were incubated for 24 h at 37°C. The colonies were then PCR-confirmed by screening for the hylA gene (LM1: 5’-CGGAGGTTCCGCAAAAGATG-3′, LM2: 5’-CCTTCCAGAGTGATCGATGTT-3′) (Kawasaki et al., 2005). Confirmed positive colonies were then banked in a 15% glycerol-85% Tryptic Soy Broth solution and stored at −80°C.
2.6. Generic Escherichia coli enrichment, enumeration, and isolation
As previously described in Atwill et al. (2015) and Patterson et al. (2018), soil samples were tested for the presence and concentration of generic E. coli (Most Probable Number, MPN/g). In summary, each TSB bag (prior to enrichment and incubation) was used as source for the 48-well TSB reservoirs (E&K Scientific, Santa Clara, CA, United States) and then serially diluted. Briefly, the first column of a 48-well reservoir was filled with 5 mL of the sample, followed by five columns filled with 4.5 mL TSB. Serial dilutions were carried out up to 10−6 in four replications per sample (Atwill et al., 2015; Patterson et al., 2018). Samples were incubated at 25°C for 2 h, and then at 42°C for 8 h with 100 rpm shaking in a Multitron programmable shaking incubator and then kept at 6°C without shaking until further use. Four μL of each incubated sample dilution were then streaked onto CHROMagar ECC (CHROMagar, Paris, France), then incubated at 37°C for 24 h (Atwill et al., 2015). Using an existing MPN calculator (Curiale, 2004), the MNP series cell densities were calculated based on dilution to extinction. Up to four presumptive positives were selected to be re-streaked onto secondary and subsequently tertiary CHROMagar ECC plates. Following incubation, pure isolates were confirmed as generic E. coli using a PCR assay targeting the universal stress protein (uspA) gene (884 bp, 5’CCGATACGCTGCCAATCAGT3’, 5’ ACGCAGACCGTAGGCCAGAT 3′), as described previously (Chen and Griffiths, 1998). Up to four isolates from each positive sample were selected and preserved in an 85% TSB 15% glycerol solution and stored at −80°C.
2.7. Data management and sources of explanatory variables for statistical analyses
In this study, a binary absence or presence of L. monocytogenes in the soil after manure application was used as the main outcome of interest. Five data sources were used for investigated variables: sample data, survey data, Honest Observer by Onset (HOBO®, Bourne, MA) data, soil composition data, and weather data. The first four were obtained through this study and the weather data was sourced from the National Oceanic and Atmospheric Administration using the R package rnoaa (Chamberlain et al., 2021).
A total of 68 sample and survey variables were considered for analysis, of which 22 corresponded to data collected at the sample level, and the remaining 46 were variables created from 32 survey questions at the farm level (Table 1 and Supplementary Table S1). Data management and details for these variables are described in Pires et al. (2023). Briefly, survey data was collected yearly and was, therefore, matched to the farm and year of the soil samples. Survey questions relating to quantifiable values (such as application rate or duration of manure aging) were asked in the survey as either multiple choice or open-ended questions. Due to the variety of formats in which individuals answered open questions, these had to be categorized for analysis. HOBO data (consisting of soil temperature and moisture collected by underground sensors) was collected every 15 min, aggregated at the day level and matched to the specific sampling date and farm. Lag times of 1 day and 7 days were used for the HOBO data. Soil composition data was collected twice a year and had to be aggregated at the year and farm level to be spatio-temporally matched with microbiological samples. This was due to the fact that the number of soil composition samples collected per farm in a year varied from 2 to 60, and these were not always matched to the specific plot or collection date of the microbiological samples (Supplementary Table S2). Thus, it was not possible to use a finer spatio-temporal resolution for this data. Weather data was obtained from all weather stations within a 40 km radius of a farm and was aggregated to the day level before being matched with the sampling date and farm using an inverse squared distance weighted average. In this case lag times of 1 day, 3 days, and 7 days were used. Depending on the farm, between 1 and 231 weather stations were within the radius (median = 39). The closest station for each farm varied between 0.3 and 37.1 km (mean = 8.2 km) (Supplementary Table S3).
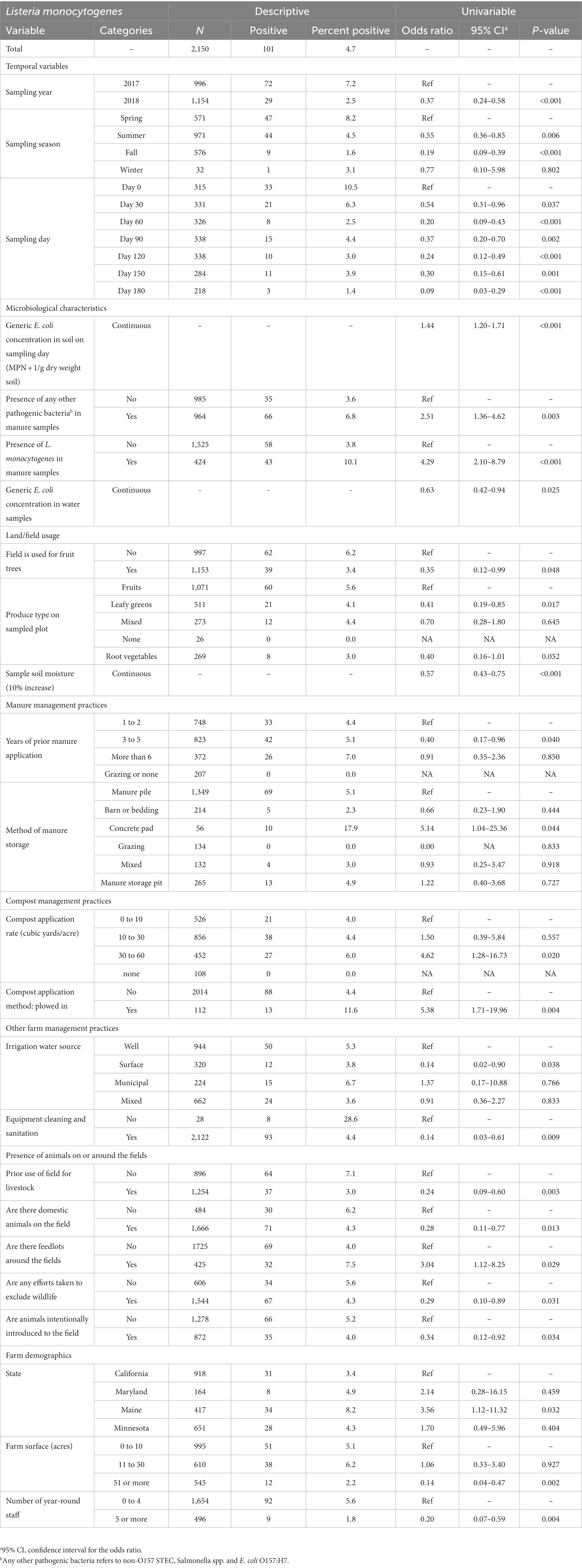
Table 1. Univariable mixed-effect logistic regression results for soil sample and survey variables that were significantly associated with the presence of Listeria monocytogenes in soil samples amended with untreated manure in certified organic farms.
2.8. Statistical analyses
The statistical analyses are similar to those used in our companion manuscript on non-O157 STEC Pires et al. (2023). Briefly, we used mixed-effect logistic regression in R with the lme4 package to identify relationships between potential risk factors and the presence of L. monocytogenes in soil (Bates et al., 2015). HOBO and weather data were standardized around the mean to allow for easier comparison of the effect sizes for variables with different units and value ranges. Soil moisture, which was a proportion of 0 to 1, was analyzed using a scale of 10% (from 0 to 10) for easier interpretation of the model coefficient. Farm and plot ID were used as nested random effects. All other variables described in section 2.6 and presented in Table 1 were analyzed as fixed effects. This includes the state, which was considered a fixed effect due to having only four categories and the fact that farms were unevenly distributed between states, with California having 9 farms and Maryland just 1. First, all variables were assessed using univariable models. Then, multivariable model selection was conducted through forward and backward stepwise model selection starting from an empty model. The Akaike Information Criterion (AIC) for model fit was used as the main criterion for variable selection. To assess the risk of multicollinearity, the modified generalized variance inflation factor [GVIF1/(2xDf)] was used on each multivariate model, following the convention that a value above 4 is a sign of collinearity, and a value above 10 is a sign of strong multicollinearity (Fox and Monette, 2023). The highest GVIF value observed was 3.16 in model 2. Due to a large number of missing values, analysis had to be divided into two sets, with one focusing on soil sample and survey data (model 1), and the other on weather and soil composition data (model 2). Year, sampling day, soil moisture at the sample-level, and generic E. coli concentration in the soil on the day of sampling were also included in model 2 as they were key variables significantly associated with the presence of L. monocytogenes in model 1. HOBO data, wind variables and compost application rate were not considered in multivariable analysis due to missing data (i.e., >205). Model fit and predictive performance were assessed using the receiver operating characteristics (ROC) curve method with the R package pROC (Robin et al., 2011). All data management and statistical analyses were conducted in R 4.0.3 (R Core Team, 2022). The significance level was set at value of p<0.05.
3. Results
Of 2,150 soil samples, 101 (4.7%) were positive for L. monocytogenes. None of the fresh produce or irrigation water samples were positive for L. monocytogenes.
3.1. Univariable analysis
3.1.1. Soil sample and survey variables
Results from the univariable analysis for all soil sample and survey variables significantly associated with the presence of L. monocytogenes in the soil are presented in Table 1 and are summarized below. Details for the other variables investigated can be found in Supplementary Table S1.
Project year was significantly associated with the presence of L. monocytogenes in the soil samples, with 2018 having lower odds than 2017 [odds ratio (OR) = 0.37, 95% confidence interval (CI) = 0.24–0.58]. Sampling day was also significantly associated with L. monocytogenes positivity of soil samples, with all later sampling times (i.e., d30 onwards) having lower odds when compared to d0B, as was sampling season, with summer (OR = 0.55, 95% CI = 0.36–0.85) and fall (OR = 0.19, 95% CI = 0.09–0.39) having lower odds than spring.
Regarding the microbiological characteristics of the soil, the concentration of generic E. coli in the soil on the day of sampling (OR = 1.44, 95% CI = 1.20–1.71) and in irrigation water samples (OR = 0.63, 95% CI = 0.42–0.94) had a significant positive and negative association with the presence of L. monocytogenes in the soil, respectively. Presence of L. monocytogenes in the soil was significantly associated with the presence of L. monocytogenes in manure samples (OR = 4.29, 95% CI = 2.10–8.79), as well as with the presence of other pathogens (i.e., E. coli O157:H7, non-O157 STEC and Salmonella spp.; OR = 2.51, 95% CI = 1.36–4.62) in manure.
An increase by 10% of soil moisture at the sample-level was significantly associated with lower odds of L. monocytogenes presence in the soil samples (OR = 0.57, 95% CI = 0.43–0.75). Produce type on the plot was also significantly associated with L. monocytogenes positivity, with leafy greens (e.g., lettuce, spinach) having lower odds than fruits (e.g., tomatoes, cucumbers) (OR = 0.41, 95% CI = 0.19–0.85).
Prior use of the field for livestock (OR = 0.24, 95% CI = 0.09–0.60), presence of domestic animals on the field (OR = 0.28, 95% CI = 0.11–0.77), and efforts taken to reduce wildlife (OR = 0.29, 95% CI = 0.10–0.89) were associated with significantly lower odds of L. monocytogenes positive soil samples, whereas the presence of feedlots around the fields was associated with significantly higher odds (OR = 3.04, 95% CI = 1.12–8.25).
As for farm management practices and demographics, irrigation water source was significantly associated with the presence of L. monocytogenes in the soil samples; specifically, the use of surface water had lower odds when compared to the use of well water (OR = 0.14, 95% CI = 0.02–0.90). Equipment cleaning and sanitation was associated with significantly lower odds of L. monocytogenes positivity (OR = 0.14, 95% CI = 0.03–0.61). The number of year-round staff was also significantly associated with L. monocytogenes presence in the soil samples, with farms employing five or more staff having lower odds compared to farms employing four or fewer staff (OR = 0.20, 95% CI = 0.07–0.59). Among manure management practices, only years of prior manure application and method of manure storage were significantly associated with L. monocytogenes presence in the soil samples; having 3 to 5 years of prior manure application on the field was associated with lower odds compared to 1 to 2 years (OR = 0.40, 95% CI = 0.17–0.96), and concrete pads storage was associated with higher odds compared to manure pile (OR = 5.14, 95% CI = 1.04–25.36). Compost application at a rate of 30 to 60 cubic yards/acre was associated with significantly higher odds of L. monocytogenes presence in the soil samples compared to a rate of 0 to 10 cubic yards/acre (OR = 4.62, 95% CI = 1.28–16.73). Compost application-incorporation method (plowing) was also significantly associated with higher odds of L. monocytogenes positivity (OR = 5.38, 95% CI = 1.71–19.96), but no other compost application methods were significantly associated with the presence of L. monocytogenes.
3.1.2. Weather and HOBO variables
Results from the univariable analysis for weather and HOBO variables are presented in Table 2. Precipitation levels on the day of sampling (OR = 0.29, 95% CI = 0.11–0.77) and the 3-day prior average (OR = 0.74, 95% CI = 0.55–0.98) were negatively associated with the presence of L. monocytogenes in the soil samples. Similarly, four of six HOBO soil moisture variables were significantly associated with the presence of L. monocytogenes in the soil samples, with increases in soil moisture leading to a reduction in odds of L. monocytogenes positivity. None of the weather temperature variables were associated with L. monocytogenes positivity, whereas nearly all HOBO soil temperature variables had a significant association with the presence of L. monocytogenes, except for minimum temperature on the day of sampling, with increasing soil temperatures leading to decreasing odds of L. monocytogenes positivity. The 7-day average for both average wind speed (OR = 1.51, 95% CI = 1.10–2.06) and wind gust speed (OR = 1.43, 95% CI = 1.06–1.93) were significantly positively associated with the presence of L. monocytogenes in the soil samples.
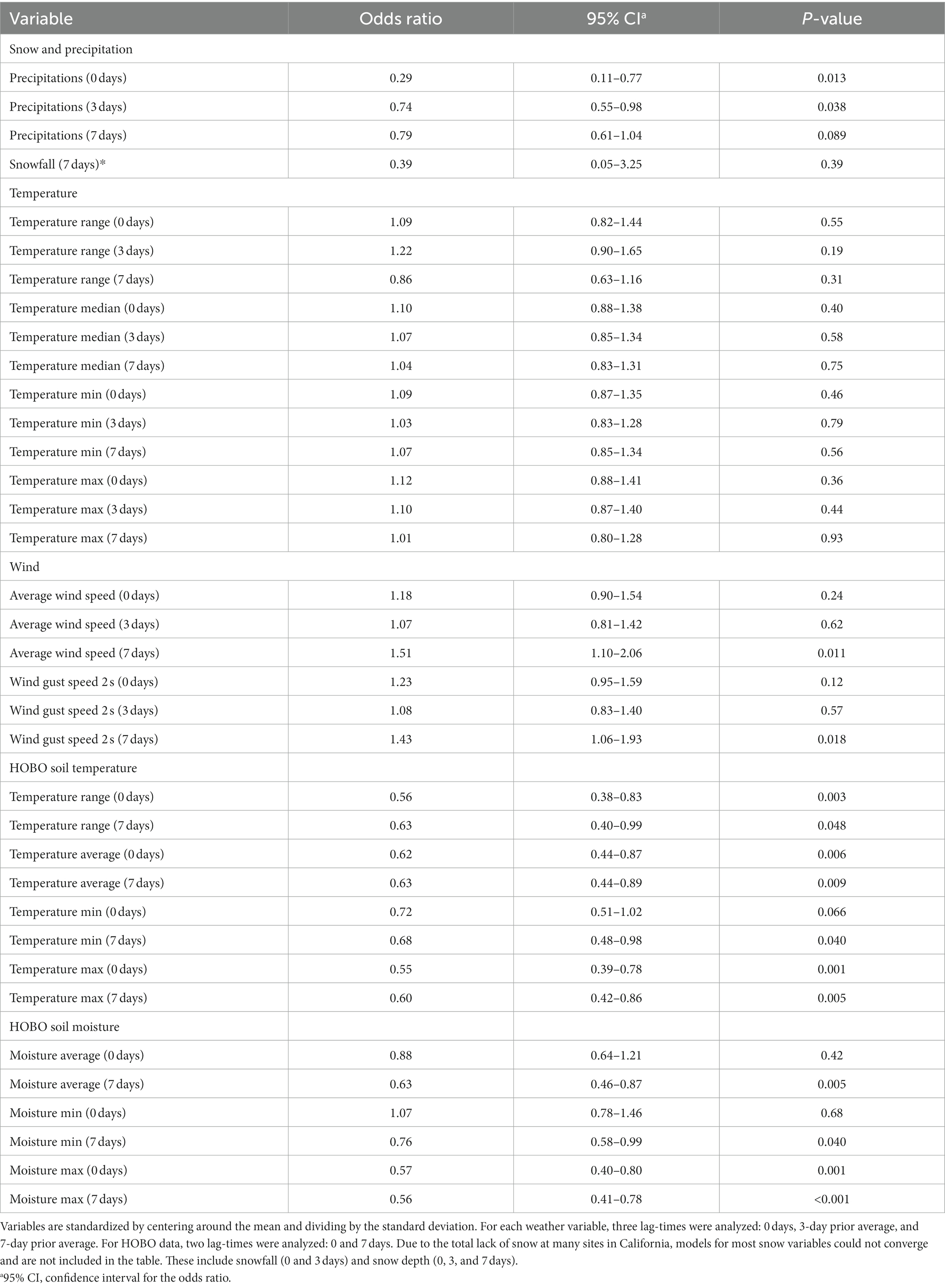
Table 2. Univariable mixed-effect logistic regression results showing the effect of weather and HOBO-related factors on the likelihood of L. monocytogenes presence in soil samples amended with untreated manure in certified organic farms.
3.1.3. Soil composition variables
Results from the univariable analysis for the soil composition variables are presented in Table 3. Of the 18 variables measured and analyzed, only three were significantly associated with the presence of L. monocytogenes in the soil samples: pH had a negative association (OR = 0.56, 95% CI = 0.39–0.80), whereas calcium (OR = 1.46, 95% CI = 1.02–2.10) and zinc (OR = 1.76, 95% CI = 1.07–2.90) levels had a positive association.
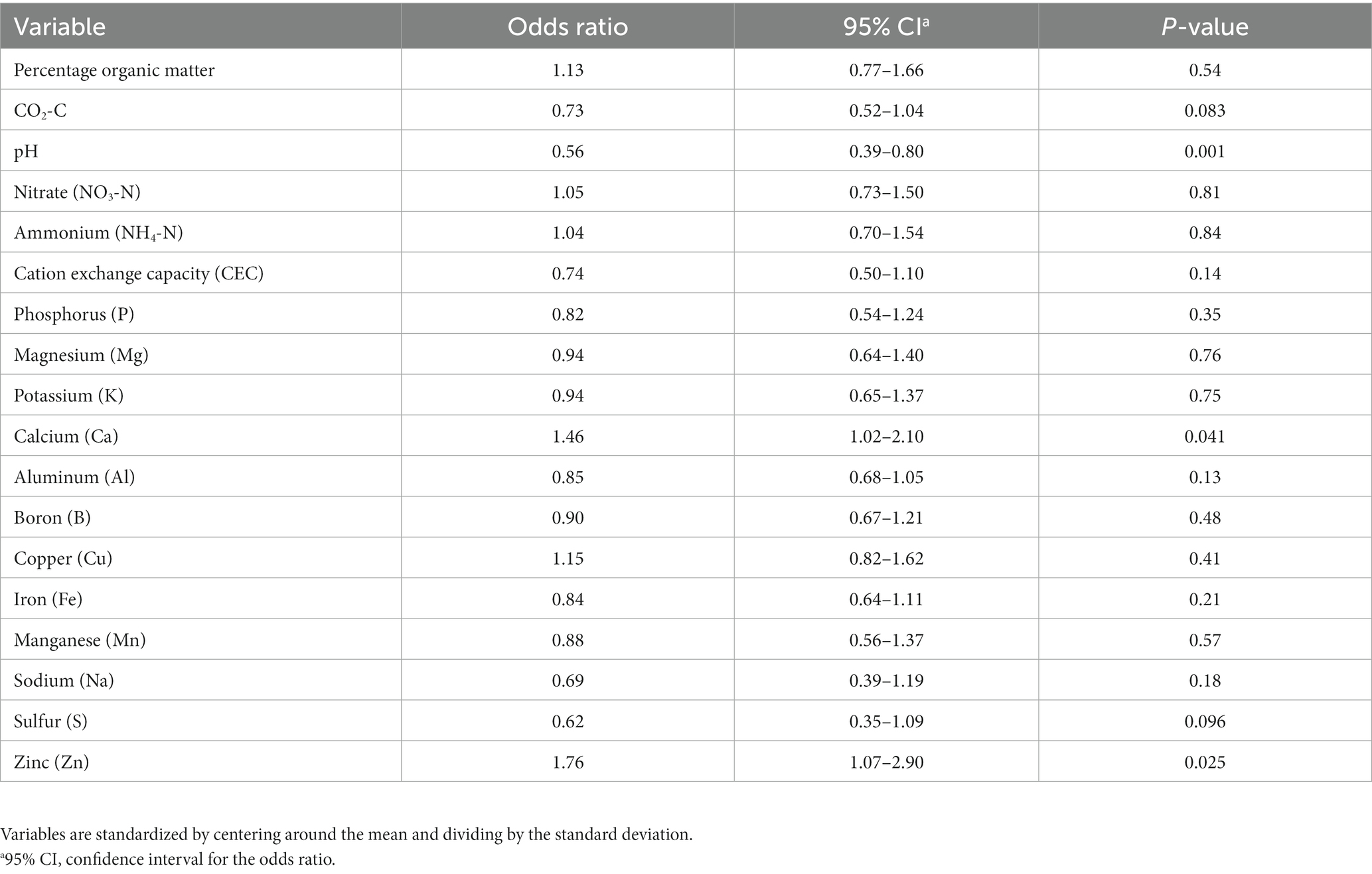
Table 3. Univariable mixed-effect logistic regression results showing the effect of soil composition variables on the likelihood of L. monocytogenes presence in soil samples amended with untreated manure in certified organic farms.
3.2. Multivariable analysis
3.2.1. Model 1: soil sample and survey variables
When subsetting the data by the variables used in model 1 selection, 73% (1,574) of observations were complete and therefore available for multivariate analysis. Eleven variables were included in the final multivariable model 1: year of sampling, sampling day, soil moisture at the sample-level, concentration of generic E. coli in the soil on the day of sampling, presence of any other pathogenic bacteria (i.e., E. coli O157:H7, non-O157 STEC, and Salmonella spp.) as a single binary variable, presence of L. monocytogenes in manure samples used on the same plot, previous use of the field for livestock, presence of domestic animals on the field, manure application season, irrigation water source, and number of year-round staff. Detailed results are presented in Table 4.
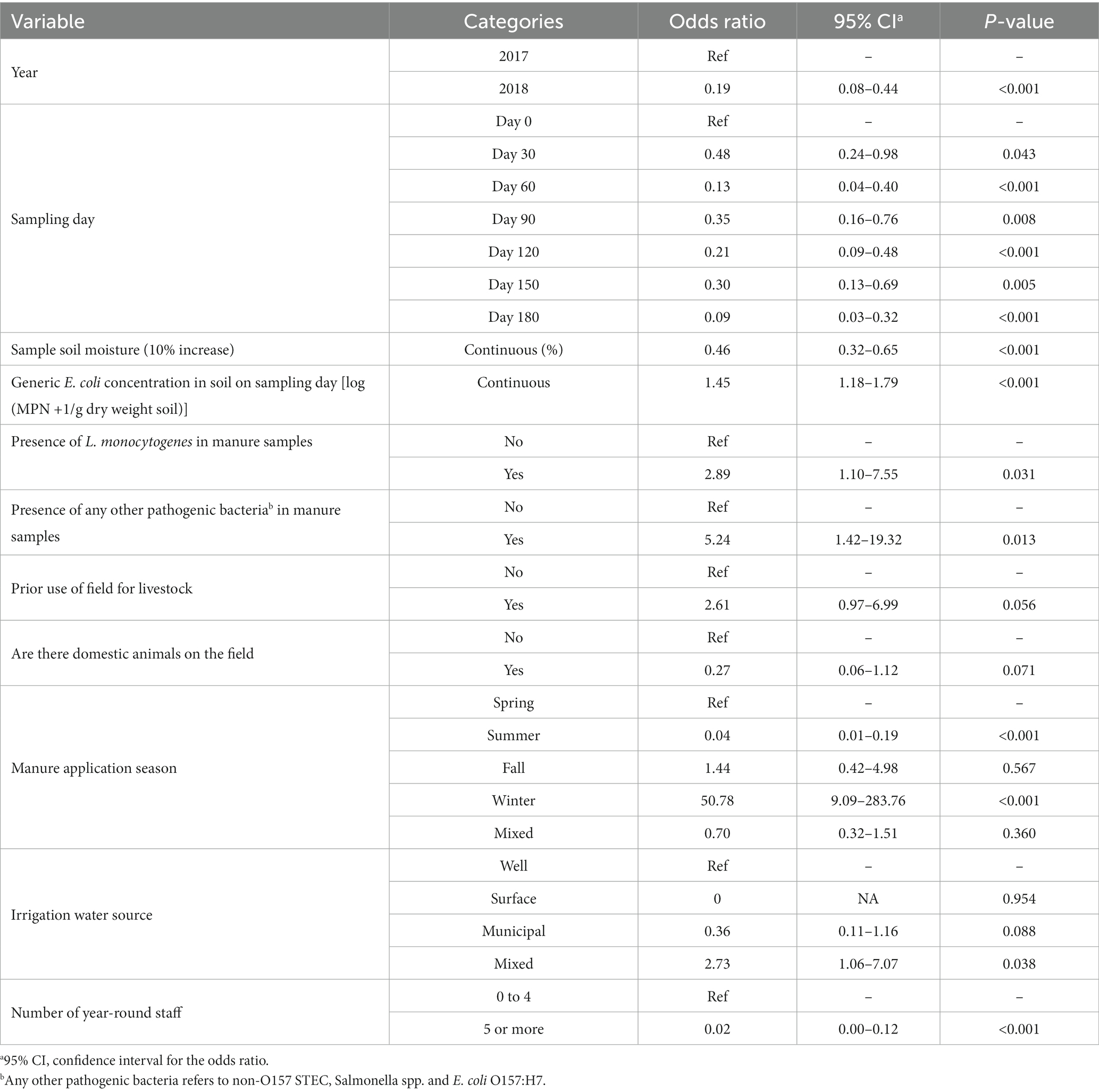
Table 4. Multivariable mixed-effect logistic regression results for the soil sample and survey variables (model 1) that were significantly associated with the presence of L. monocytogenes in soil samples amended with untreated manure in certified organic farms.
Year was significantly associated with the presence of L. monocytogenes in the soil samples, with 2018 having lower odds compared to 2017 [odds ratio (OR) = 0.19, 95% CI = 0.08–0.44]. Sampling day was significantly associated with L. monocytogenes positivity, with every sampling day from d30 onwards having lower odds compared to d0B post-manure application. An increase by 10% in soil moisture at the sample-level led to a decrease in odds of L. monocytogenes positivity by a factor of 0.46 (95% CI = 0.32–0.65). An increase of one unit of log (MPN + 1/dry weight of soil) of generic E. coli in the soil on the day of sampling was associated with increased odds of L. monocytogenes presence in the soil samples (OR = 1.45, 95% CI = 95% CI = 1.18–1.79). The presence of L. monocytogenes and other pathogenic bacteria in manure samples were associated with significantly higher odds of L. monocytogenes positivity (OR = 2.89 and 5.24, respectively, 95% CI = 1.10–7.55 and 1.42–19.32). Applying manure in winter was associated with significantly higher odds of L. monocytogenes positivity (OR = 50.78, 95% CI = 9.09–283.76), and summer with significantly lower odds (OR = 0.04, 95% CI = 0.01–0.19), when compared to spring. This differed from the univariable results, where no significant association was observed. The number of year-round staff was also significantly associated with L. monocytogenes presence in the soil samples, with farms employing five or more staff having lower odds compared to farms employing four or fewer staff (OR = 0.02, 95% CI = 0–0.12). Finally, regarding irrigation water source, use of mixed water sources was associated with significantly higher odds of L. monocytogenes presence in the soil samples compared to well water use (OR = 2.73, 95% CI = 1.06–7.07). This differed from the univariable results, where the use of surface water was associated with significantly lower odds of L. monocytogenes positivity, and none of the other water sources significantly differed from well water use. However, with the reduced sample size used for multivariable analysis, there were no positive L. monocytogenes samples in the surface water category, making it impossible to compute an odds ratio. The area under the curve (AUC) was 0.87, the sensitivity 0.80, and the specificity 0.82. Positive and negative predictive values were 0.21 and 0.99, respectively.
3.2.2. Model 2: weather and soil composition variables
After removing HOBO and wind variables, and subsetting the data by the variables used in model 2 selection, 92% (1,978) of observations were complete and available for multivariable analysis. Results for year, sampling day, concentration of generic E. coli in the soil on the day of sampling, and soil moisture were similar to those of model 1 and will not be described, but details can be found in Table 5. The final multivariable model 2 also included two weather variables and five soil composition variables: precipitation on the day of sampling, average temperature range in the seven days prior to sampling, percentage of organic matter in the soil, cation exchange capacity (CEC), and nitrate (NO3-N), potassium, and calcium levels in soil.
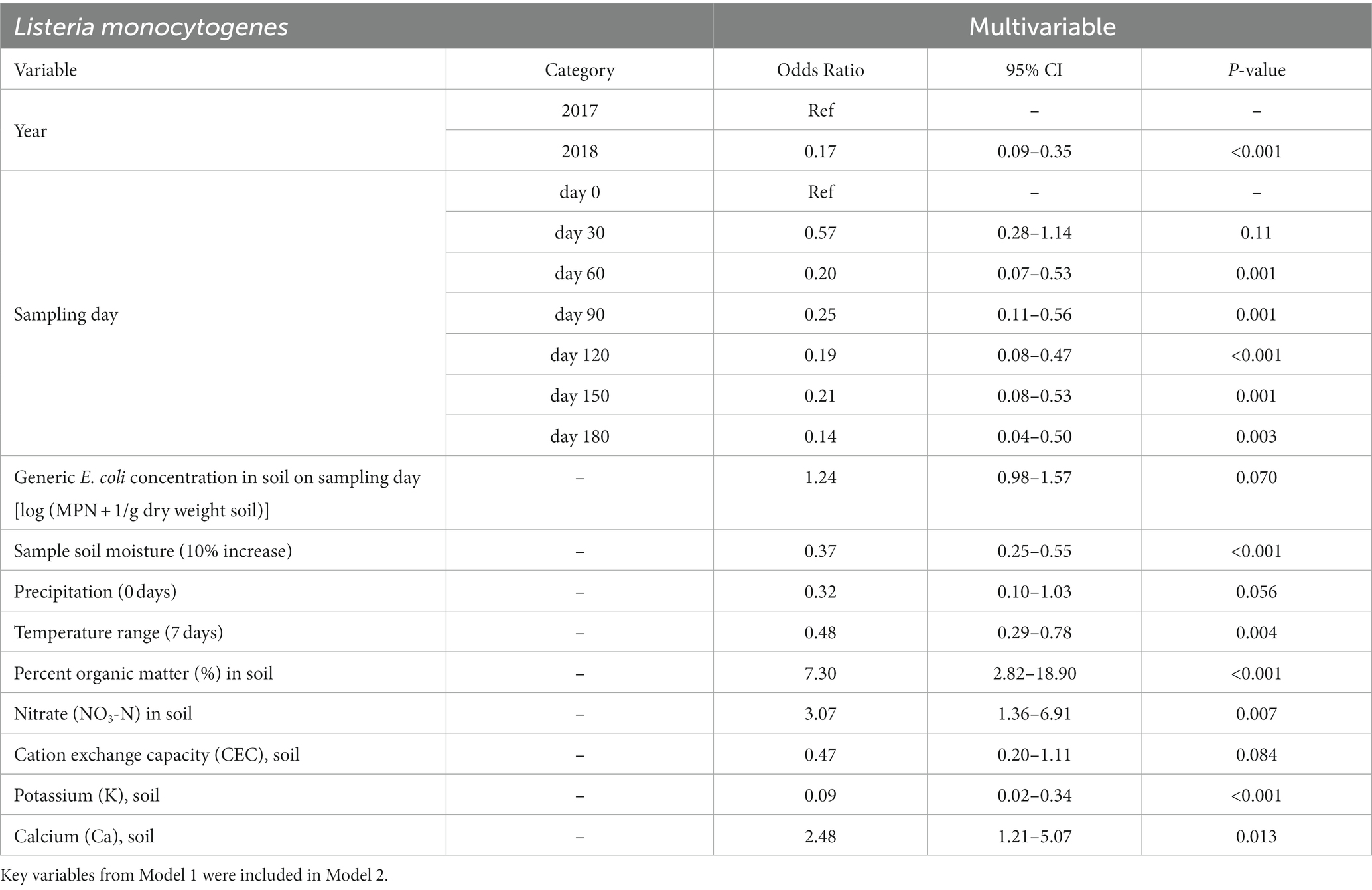
Table 5. Multivariable mixed-effect logistic regression results for weather factors and soil composition variables (model 2) that are significantly associated with the presence of L. monocytogenes in soil samples amended with untreated manure in certified organic farms.
Temperature range (7 days) was negatively associated with L. monocytogenes presence in the soil samples (OR = 0.48, 95% CI = 0.29–0.78). The result for temperature differed from the univariable analysis, where none of the temperature variables had a significant association with L. monocytogenes positivity. For the soil composition variables, percentage of organic matter (OR = 7.30, 95% CI = 2.82–18.90) and levels of NO3-N (OR = 3.07, 95% CI = 1.36–6.91) and calcium (OR = 2.48, 95% CI = 1.21–5.07) in the soil were positively associated with L. monocytogenes presence in the soil samples, whereas potassium levels were negatively associated with L. monocytogenes positivity (OR = 0.09, 95% CI = 0.02–0.34). Most of these results differed from the univariable results as none of these variables, except for calcium levels, were significantly associated with L. monocytogenes presence in the soil samples.
Model 2 also had moderately high performance metrics as a whole with the ROC curve analysis. The AUC was 0.89, the sensitivity 0.83, and the specificity 0.84. Positive and negative predictive values were 0.20 and 0.99, respectively.
4. Discussion
This longitudinal study is one of the first to simultaneously assess multiple risk factors, including farm management practices and demographics, environmental factors (i.e., soil and microbiological characteristics), and weather factors, associated with the presence of L. monocytogenes in soil amended with untreated manure on certified organic farms in four different regions of the US. Previous studies have found that farm management, landscape, meteorological, and spatio-temporal factors significantly impact the odds of L. monocytogenes contamination of produce fields (Strawn et al., 2013a,b; Weller et al., 2015). However, these studies were restricted to a single state/region of the US, used a limited number of samples collected in the same field/plot, and did not specifically focus on certified-organic farms. Additionally, this study provides scientific insight into the complex nature of L. monocytogenes persistence in the soil, and subsequent contamination of the farm environment, demonstrating the need for food safety guidelines to be data-driven and to account for more than a single metric of time interval between manure application and harvesting of fresh produce.
The odds of L. monocytogenes presence in the soil were significantly lower in 2018 than in 2017, which is similar to the patterns reported for non-O157 STEC on the studied farms (Pires et al., 2023). The majority of farm management practices remained the same during the 2 years, thus, the difference may be attributed to changes that could not be controlled, such as weather conditions, soil characteristics, and/or other environmental factors. Notably, 2017 was the second wettest year on record in CA, where the majority of the study farms were located, with some areas receiving 300% of the average precipitation, ending the state’s 5-year drought (California Department of Water Resources, 2017), whereas another statewide drought was recorded in 2018 (California Department of Water Resources, 2018). Runoff, especially from livestock farms, has previously been shown to increase the risk of L. monocytogenes contamination, and the historic amount of runoff in CA in 2017 may, therefore, have contributed to the increased presence of L. monocytogenes in the soil (Strawn et al., 2013a). Moreover, other studies have demonstrated a positive relationship between adjacent land use, specifically for livestock farming, and L. monocytogenes contamination (Sauders et al., 2006; Lyautey et al., 2007). In contrast, the other states involved in the project had normal or increased precipitation in 2018 when compared to 2017. These differences show the critical need for multi-year studies given the weather conditions may drastically change across the years.
In both models 1 and 2, sampling day was significantly associated with the presence of L. monocytogenes in the soil. While all sampling days had decreased odds of L. monocytogenes contamination when compared to d0B, d180 had the lowest. Previous studies have investigated the prevalence of L. monocytogenes in the soil over time (Hutchison et al., 2004; Locatelli et al., 2013). While Hutchison et al. (2004) found the maximum isolation time for L. monocytogenes was 120 days, other studies did not detect the pathogen beyond 2 weeks (Hutchison et al., 2004; Locatelli et al., 2013). Differences between these isolation times were attributed to environmental factors (e.g., climate, temperature, spatial coordinates) and soil characteristics (e.g., pH, soil chemistry, soil texture) (Locatelli et al., 2013); likewise we identified weather and soil factors associated with the presence of this foodborne pathogen. Certified organic farms follow the 90–120 days USDA-NOP standards time-interval between the application of raw manure and crop harvest, depending if the edible part is in contact or not with the soil (USDA NOP, 2018). The time-interval as a preventive measure to avoid contamination of the soil and produce is based on the assumption that an appropriate amount of time has passed to foodborne pathogen die-off (Phan-Thien et al., 2020). However, our findings suggest that the NOP 90- and 120-day wait times may be sufficient, and there was a significant decrease compared to 30 days post manure application; this time interval may be an impairment to the use of untreated manure in regions with multiple growing seasons, such as California. Moreover, this study shows that a range of on-farm and environmental factors are associated with the presence of foodborne pathogens in soil, indicating that those risk factors should be taken into account in pre-harvest food safety plans.
Regarding the microbiological characteristics of the soil, previous studies have shown that generic E. coli can serve as an indicator for foodborne pathogens in soil, including L. monocytogenes (Falardeau et al., 2017). Similarly, we observed increased concentrations of generic E. coli in the soil were associated with increased odds of L. monocytogenes presence. By contrast, no positive association was observed with generic E. coli concentrations in water, which confirms that it may not be a suitable predictor for L. monocytogenes in water, given the complex interactions between weather, and water microbial and physicochemical characteristics (Weller et al., 2020). The presence of other pathogens (i.e., non-O157 STEC, E. coli O157:H7, and Salmonella spp.) and of L. monocytogenes in manure applied to crop fields was associated with increased odds of L. monocytogenes in the soil. While some studies have found that L. monocytogenes survival is increased in the absence of normal competitors, they did not include other foodborne pathogens (Botzler et al., 1960; Dowe et al., 1997; McLaughlin et al., 2011). It is possible that characteristics of the manure, such as its percentage of organic matter, rather than microbial activity, influenced the presence of L. monocytogenes in the soil. Previous studies have noted potential L. monocytogenes transmission pathways from infected livestock feces/manure to soil (Nightingale et al., 2004). However, further analyses are needed to determine whether the strains of L. monocytogenes isolated from the soil in this study were the same as those present in the untreated manure applied to the field. Other studies have detailed the survival of Listeria spp. in the soil and established how the bacteria may be transferred to produce through contact with soil or splashing events caused by rain or irrigation, thereby highlighting the food safety risks associated with contaminated soil (Girardin et al., 2005; Oliveira et al., 2011). Additionally, L. monocytogenes is known to interact with the roots of leafy produce, and may internalize into mature plants as well as seedlings, but relatively low concentrations of L. monocytogenes have been isolated from contaminated fresh produce (Standing et al., 2013; Smith et al., 2018). Further studies are needed to determine which levels of L. monocytogenes in the raw manure may lead to the contamination of soil and subsequently to that of produce in fields amended with raw manure. Aging or composting manure before application to produce fields is a promising way to significantly decrease pathogen activity in manure, and thus decrease the risk of soil, and subsequent produce contamination (Gurtler et al., 2018).
Surprisingly, none of the variables related to the presence of domestic animals or wildlife were significantly associated with the presence L. monocytogenes in the soil, when they are known sources of foodborne pathogen contamination in produce fields (Hoelzer et al., 2011; Strawn et al., 2013a). However, it is important to note that domestic animal and wildlife presence were self-reported by farmers, and therefore may not have been accurately evaluated. The presence of fecal matter on the field may provide a more valid assessment of the presence of animals. However, while it was positively associated with the presence of non-O157 STEC in the soil, it was not associated with L. monocytogenes positivity (Pires et al., 2023).
Of the numerous farm management and demographic variables assessed in this study, only season of manure application, number of year-round staff, and water source, were significantly associated with L. monocytogenes presence in the multivariable models. Manure application in winter and summer was associated with higher and lower odds of L. monocytogenes positivity, respectively, when compared to spring. Unlike many other pathogens, L. monocytogenes has been found to survive, and even be at its highest prevalence, in winter months and conditions (Botzler et al., 1960; Guerini et al., 2007; Strawn et al., 2013a; Cooley et al., 2014). Additionally, previous studies reported an increase in L. monocytogenes survival in soil with decreased competition with native soil microbiota (Botzler et al., 1960; Dowe et al., 1997; McLaughlin et al., 2011). In winter months, other pathogens, such as Salmonella spp., persist at much lower rates than during warmer months, thus decreasing microbial competition for L. monocytogenes (Cooley et al., 2014). The increased survival of L. monocytogenes in winter months may contribute to the increased risk of L. monocytogenes transmission from untreated manure to the soil of the fields to which it is applied. Due to state and local regulations, or to follow the NOP 90- or 120-day time interval, the majority of farms apply manure to the fields in the fall, during which the odds of L. monocytogenes presence was lower (i.e., not significantly different from spring) (Pires et al., 2018).
We found that farms with five or more year-round staff have lower odds of L. monocytogenes presence in soil than smaller farms with four or fewer staff. A previous study in the southwest US found that farm hygiene was a protective factor of generic E. coli contamination in produce; however, this study did not explore soil contamination, or the effect the size that the farming operation had on contamination (Park et al., 2014). Larger farms with increased numbers of employees are more likely to participate in third party food safety certification programs or audits, such as GLOBALG.A.P (Schmit et al., 2020). Thus, larger farming operations are more likely to have a strong food safety culture and training programs that allow better implementation of measures that decrease the risk of microbial contamination of the fields and produce. Additionally, Ramos et al. (2019) reported that many small farmers find on-farm food safety plans and training intimidating without the assistance of third parties. This lack of employee training on smaller farms may result in inadequate application of Good Agricultural Practices, thus increasing the risk of L. monocytogenes contamination in produce fields. Additional outreach and educational support for these smaller farms may help decrease the risk of pathogen contamination of soil and fresh produce.
Water is a known reservoir of, and can serve as a transmission pathway for foodborne pathogens to soil and produce (Beuchat, 2006). In univariable analysis, only surface water significantly differed from well water, and it had a surprising negative association with L. monocytogenes presence in the soil. However, no positive surface water samples could be included in multivariable analysis due to the decrease in sample size. In multivariable analysis, we found that the use of mixed sources of irrigation water was positively associated with L. monocytogenes presence in the soil, whereas the use of municipal sources had a protective effect, although it was borderline significant. This could be due to differences in environmental contamination among water sources, as engineered water sources, such as municipal or well water, are generally more protected than surface and mixed irrigation water sources. Strawn et al. (2013a) indeed found that surface water was significantly more likely to be contaminated with L. monocytogenes than water from engineered sources. In order to reduce the contamination risks and food safety hazards from agricultural water, FSMA-PSR recently established requirements for microbial standards; however, the pre-harvest agricultural water rule is still under review (FDA, 2015a).
Interestingly, we found that the presence of L. monocytogenes in the soil was negatively associated with the 7-day temperature range, but none of the associations with other weather variables were significant in the multivariable models. This contrasts with previous studies, which found that higher precipitation levels increased the survival of L. monocytogenes (Park et al., 2014; Pang et al., 2017). Further investigation into the short- and long-term effects of precipitation on soil contamination by L. monocytogenes may offer better insights into the risk posed by this weather event. L. monocytogenes is known to survive the large temperature fluctuations associated with food production and processing (Liu, 2008; Smith et al., 2018), which may explain the negative association we observed with the 7-day temperature range. However, the absence of association with other temperature variables conflicts with previous findings, as L. monocytogenes survival is generally increased at cooler temperatures (McLaughlin et al., 2011; Strawn et al., 2013a).
Soil moisture increases with decreased temperature and increased precipitation, which favors L. monocytogenes survival (Botzler et al., 1960; Weis and Seeliger, 1975; Strawn et al., 2013a). Similarly, four of the six HOBO soil moisture variables were significantly negatively correlated with L. monocytogenes presence in the soil, with long-term (i.e., 7 days) variables showing slightly stronger effects than short-term (i.e., 0 days) variables. However, this contrasts with this study’s findings for soil moisture at the sample-level, which showed a significant negative association with L. monocytogenes presence.
Regarding soil composition, we found that an increase in the percentage of organic matter in the soil was associated with an increased odds of L. monocytogenes presence, which is similar to previous studies (Jamieson et al., 2002; Vogel et al., 2010; Strawn et al., 2013a). This increase in pathogen survival has been attributed to the effects of organic matter on soil moisture retention (Jamieson et al., 2002; Williams et al., 2015). However, we observed a negative association between soil moisture at the sample-level and L. monocytogenes presence. It is important to note that the addition of manure to the field may have a confounding effect as it increases the organic matter content of the soil, and may also introduce L. monocytogenes into the soil. Calcium concentration in the soil was positively associated with the presence of L. monocytogenes. This observation is similar to previous findings that identified calcium as one of the main soil characteristics that impacted L. monocytogenes survival in soil (Locatelli et al., 2013). Nitrate and potassium concentrations in the soil were positively and negatively associated with the presence of L. monocytogenes, respectively. A recent study reported that an increase in macronutrients in the soil, namely nitrogen, potassium, and phosphorus, was associated with a decrease in L. monocytogenes survival (Devarajan et al., 2021).
Of all the soil characteristics included in the final multivariable model, only calcium had a significant effect in the univariable analysis, indicating the presence of confounding factors among the variables. The complex nature of soil chemistry, and the intricate relationships among nutrients and soil characteristics (e.g., pH, organic matter) render it difficult to identify which variables are interacting and how they affect L. monocytogenes survival. Furthermore, research into the effects of soil chemical characteristics on L. monocytogenes presence in the soil is scant, with many studies identifying numerous interactions among the soil characteristics investigated, and others focusing only on soil textures (Dowe et al., 1997; Nicholson et al., 2005; Locatelli et al., 2013). Further studies are needed to identify the individual and combined effects of soil characteristics as well as the role the microbiome plays on the survival of pathogens in the soil.
On the one hand, one of the advantages of on-farm observational studies with repeated sampling (i.e., longitudinal studies), is that they allow to investigate the dynamics of foodborne pathogens in natural settings (as opposed to inoculation studies), with contamination loads in environment matrixes (e.g., manure and soil) as close as possible to those on commercial settings, and to account for time-variable factors. On the other hand, there are limitations inherent to this type of study design. First, because there are no interventions across the farms (besides manure application), some of the key factors affecting the survival of pathogens may vary (e.g., soil type, weather, irrigation); however, those were controlled for in statistical analysis. Second, the loss due to follow-up may contribute to an unbalanced sampling across the 2 years. In addition, other limitations are inconsistent sample collection for soil characteristic testing (due to scheduling limits, weather prohibiting sampling, unpredictable farming schedules, etc.), as well as missing weather data, which restricted some of the statistical analyses. Inconsistent sampling and missing data also contributed to an unbalanced data set for some variables. Moreover, data groups were collected with different temporal resolutions (sampling day, several times a year) and spatial resolutions (farm versus plot); this was partially accounted for by using random effects. These are limitations of observational studies conducted on commercial farms as opposed to controlled experimental field trials. In addition, we could not assess the genetic relatedness of the L. monocytogenes isolates detected in manure and respective amended soils. However, as an observational study, the goal was to assess the multitude of risk factors associated with the presence and persistence of this foodborne pathogen in USDA-NOP certified organic farms using untreated manure as a soil amendment. Additionally, detection of L. monocytogenes was limited by the sensitivity of the testing protocols used in this study. It is finally important to note that this study allowed us to report only observed associations and not to infer causal relationships.
5. Conclusion
This is the first study to examine L. monocytogenes contamination risk factors associated with untreated-manure–amended soil on organic farms in four regions of the US. The findings of this study highlight that factors beyond time intervals are associated with the presence of L. monocytogenes in soil. While sampling time was significantly associated with L. monocytogenes contamination, multiple environmental (weather, soil characteristics), management (manure application season, number of employees), and microbiological (generic E. coli, other foodborne pathogens) factors were significantly associated with the presence of L. monocytogenes in the soil. This emphasizes the need to further examine risk factors beyond time intervals, and incorporate those variables into regulations for the use of untreated manure on fresh produce fields.
Data availability statement
The raw data supporting the conclusions of this article will be made available by the authors, without undue reservation.
Ethics statement
Ethical review and approval was not required for the study on human participants in accordance with the local legislation and institutional requirements. Written informed consent from the participants was not required to participate in this study in accordance with the national legislation and the institutional requirements.
Author contributions
AFAP, MJ-R, PM, PP, MH, JS, and BM-L conceived the project, designed the study, and wrote the grant. AFAP, PM, FH, PP, and MH coordinated the efforts between the regional teams. AFAP oversaw the day-to-day aspects of the project. AFAP, MJ-R, and PM oversaw the laboratory work. TR, AK, CC-K, VH, and PA carried out the field work and sampling collection. TR, VH, and PA carried out the laboratory work. AFAP, JB, and BM-L developed the data analysis plan, which JB implemented. CC-K, JF, JB, and VH wrote the first draft of the manuscript. All authors contributed to manuscript revision, read, and approved the final manuscript.
Funding
This work was funded by the Organic Agriculture Research and Extension Initiative (OREI), National Institute of Food and Agriculture, U.S. Department of Agriculture, under award number 2016-51300-25724.
Acknowledgments
We acknowledge James Stover, Amanda Taylor, Zoe Scholar, Deonnie Huang, Rachel Hawkins, Amy Patamakomol, Ka Man Woo, Jason Lilley, and Nicholas Rowly for assistance in the laboratory and field work, as well as Tracy Misiewicz from the Organic Center for assistance with farmer recruitment. We would like to thank all the participating farms.
Conflict of interest
The authors declare that the research was conducted in the absence of any commercial or financial relationships that could be construed as a potential conflict of interest.
Publisher’s note
All claims expressed in this article are solely those of the authors and do not necessarily represent those of their affiliated organizations, or those of the publisher, the editors and the reviewers. Any product that may be evaluated in this article, or claim that may be made by its manufacturer, is not guaranteed or endorsed by the publisher.
Supplementary material
The Supplementary material for this article can be found online at: https://www.frontiersin.org/articles/10.3389/fsufs.2023.1222192/full#supplementary-material
References
Atwill, E. R., Chase, J. A., Oryang, D., Bond, R. F., Koike, S. T., Cahn, M. D., et al. (2015). Transfer of Escherichia coli O157:H7 from simulated wildlife scat onto romaine lettuce during foliar irrigation. J. Food Prot. 78, 240–247. doi: 10.4315/0362-028X.JFP-14-277
Bates, D., Mächler, M., Bolker, B., and Walker, S. (2015). Fitting linear mixed-effects models using lme4. J. Stat. Softw. 67. doi: 10.18637/jss.v067.i01
Beuchat, L. R. (2006). Vectors and conditions for preharvest contamination of fruits and vegetables with pathogens capable of causing enteric diseases. Br. Food J. 108, 38–53. doi: 10.1108/00070700610637625
Botzler, R. G., Cowan, A. B., and Wetzler, T. F. (1960). Survival of Listeria monocytogenes in soil. J. Bacteriol. 80, 316–320. doi: 10.1128/jb.80.3.316-320.1960
California Department of Water Resources (2017). Water year 2017: what a difference a year makes. California Department of Water Resources, California Natural Resources Agency, State of California.
California Department of Water Resources (2018). Water year 2018: hot and dry conditions return. California Department of Water Resources, California Natural Resources Agency, State of California.
CDC (2013). Vital signs: Listeria illnesses, deaths, and outbreaks United States, 2009–2011. Morb. Mortal. Wkly. Rep. 62, 448–452. [Online]. Available at: https://www.cdc.gov/mmwr/preview/mmwrhtml/mm6222a4.htm
CDC (2023). Listeria (Listeriosis) Listeria Outbreaks. Available at: https://www.cdc.gov/listeria/outbreaks/index.html (Accessed June 23, 2023).
Chamberlain, S., Hocking, D., Anderson, B., Salmon, M., Erickson, A., Potter, N., et al. (2021). Package ‘rnoaa’: “NOAA” weather data from R. Available at https://cran.r-project.org/web/packages/rnoaa/rnoaa.pdf (Accessed July 25, 2021).
Chen, J., and Griffiths, M. W. (1998). PCR differentiation of Escherichia coli from other Gram-negative bacteria using primers derived from the nucleotide sequences flanking the gene encoding the universal stress protein. Lett. Appl. Microbiol. 27, 369–371. doi: 10.1046/j.1472-765x.1998.00445.x
Cooley, M. B., Quiñones, B., Oryang, D., Mandrell, R. E., and Gorski, L. (2014). Prevalence of Shiga toxin producing Escherichia coli, Salmonella enterica, and Listeria monocytogenes at public access watershed sites in a California central coast agricultural region. Front. Cell. Infect. Microbiol. 4. doi: 10.3389/fcimb.2014.00030
Curiale, M. (2004). MPN Calculator. Available at: http://www.i2workout.com/mcuriale/mpn/index.html (Accessed July 25, 2019).
Devarajan, N., McGarvey, J. A., Scow, K., Jones, M. S., Lee, S., Samaddar, S., et al. (2021). Cascading effects of composts and cover crops on soil chemistry, bacterial communities and the survival of foodborne pathogens. J. Appl. Microbiol. 131, 1564–1577. doi: 10.1111/jam.15054
Dowe, M. J., Jackson, E. D., Mori, J. G., and Bell, C. R. (1997). Listeria monocytogenes survival in soil and incidence in agricultural soils. J. Food Prot. 60, 1201–1207. doi: 10.4315/0362-028X-60.10.1201
Erickson, M. C., Liao, J., Jiang, X., and Doyle, M. P. (2014). Inactivation of pathogens during aerobic composting of fresh and aged dairy manure and different carbon amendments. J. Food Prot. 77, 1911–1918. doi: 10.4315/0362-028X.JFP-14-194
Falardeau, J., Johnson, R. P., Pagotto, F., and Wang, S. (2017). Occurrence, characterization, and potential predictors of verotoxigenic Escherichia coli, Listeria monocytogenes, and Salmonella in surface water used for produce irrigation in the lower mainland of British Columbia, Canada. Plos One 12:e0185437. doi: 10.1371/journal.pone.0185437
FDA (2015a). Code for Federal Regulations Title 21 part 112: standards for the growing, harvesting, packing, and holding of produce for human consumption.
FDA (2015b). Produce safety rule (21 CFR 112): biological soil amendments of animal origin. Available at: https://www.fda.gov/files/food/published/Fact-Sheet--Biological-Soil-Amendments-of-Animal-Origin_Download.pdf (Accessed November 2, 2022).
FDA (2018). Standards for the growing, harvesting, packing, and holding of produce for human consumption: guidance for industry. Draft guidance. In: Federal Register Notice (Ed.), 54888–54891.
Fox, J., and Monette, G. (2023). Generalized collinearity diagnostics. J. Am. Stat. Assoc. 87, 178–183. doi: 10.1080/01621459.1992.10475190
Garner, D., and Kathariou, S. (2016). Fresh produce–associated listeriosis outbreaks, sources of concern, teachable moments, and insights. J. Food Prot. 79, 337–344. doi: 10.4315/0362-028X.JFP-15-387
Girardin, H., Morris, C. E., Albagnac, C., Dreux, N., Glaux, C., and Nguyen-The, C. (2005). Behaviour of the pathogen surrogates Listeria innocua and Clostridium sporogenes during production of parsley in fields fertilized with contaminated amendments. FEMS Microbiol. Ecol. 54, 287–295. doi: 10.1016/j.femsec.2005.04.003
Guerini, M. N., Brichta-Harhay, D. M., Shackelford, S. D., Arthur, T. M., Bosilevac, J. M., Kalchayanand, N., et al. (2007). Listeria prevalence and Listeria monocytogenes serovar diversity at cull cow and bull processing plants in the United States. J. Food Prot. 70, 2578–2582. doi: 10.4315/0362-028X-70.11.2578
Gurtler, J. B., Doyle, M. P., Erickson, M. C., Jiang, X., Millner, P., and Sharma, M. (2018). Composting to inactivate foodborne pathogens for crop soil application: a review. J. Food Prot. 81, 1821–1837. doi: 10.4315/0362-028X.JFP-18-217
Harrand, A. S., Strawn, L. K., Illas-Ortiz, P. M., Wiedmann, M., and Weller, D. L. (2020). Listeria monocytogenes prevalence varies more within fields than between fields or over time on conventionally farmed New York produce fields. J. Food Prot. 83, 1958–1966. doi: 10.4315/JFP-20-120
Harris, L. J., Farber, J. N., Beuchat, L. R., Parish, M. E., Suslow, T. V., Garrett, E. H., et al. (2003). Outbreaks associated with fresh produce: incidence, growth, and survival of pathogens in fresh and fresh-cut produce. Compr. Rev. Food Sci. Food Saf. 2, 78–141. doi: 10.1111/j.1541-4337.2003.tb00031.x
Hoelzer, K., Moreno Switt, A., and Wiedmann, M. (2011). Animal contact as a source of human non-typhoidal salmonellosis. Vet. Res. 42:34. doi: 10.1186/1297-9716-42-34
Hutchison, M. L., Walters, L. D., Moore, A., Crookes, K. M., and Avery, S. M. (2004). Effect of length of time before incorporation on survival of pathogenic bacteria present in livestock wastes applied to agricultural soil. Appl. Environ. Microbiol. 70, 5111–5118. doi: 10.1128/AEM.70.9.5111-5118.2004
Hutchison, M. L., Walters, L. D., Moore, T., Thomas, D. J. I., and Avery, S. M. (2005). Fate of pathogens present in livestock wastes spread onto fescue plots. Appl. Environ. Microbiol. 71, 691–696. doi: 10.1128/AEM.71.2.691-696.2005
Ivanek, R., Gröhn, Y. T., Wells, M. T., Lembo, A. J., Sauders, B. D., and Wiedmann, M. (2009). Modeling of spatially referenced environmental and meteorological factors influencing the probability of Listeria species isolation from natural environments. Appl. Environ. Microbiol. 75, 5893–5909. doi: 10.1128/AEM.02757-08
Jamieson, R. C., Gordon, R. J., Sharples, K. E., Stratton, G. W., and Madani, A. (2002). Movement and persistence of fecal bacteria in agricultural soils and subsurface drainage water: a review. Canadian biosystems engineering, 44, 1–9.
Jiang, X., Islam, M., Morgan, J., and Doyle, M. P. (2004). Fate of Listeria monocytogenes in bovine manure–amended soil. J. Food Prot. 67, 1676–1681. doi: 10.4315/0362-028X-67.8.1676
Kawasaki, S., Horikoshi, N., Okada, Y., Takeshita, K., Sameshima, T., and Kawamoto, S. (2005). Multiplex PCR for simultaneous detection of Salmonella spp., Listeria monocytogenes, and Escherichia coli O157:H7 in meat samples. J. Food Prot. 68, 551–556. doi: 10.4315/0362-028X-68.3.551
Liao, J., Guo, X., Weller, D. L., Pollak, S., Buckley, D. H., Wiedmann, M., et al. (2021). Nationwide genomic atlas of soil-dwelling Listeria reveals effects of selection and population ecology on pangenome evolution. Nat. Microbiol. 6, 1021–1030. doi: 10.1038/s41564-021-00935-7
Limoges, M. A., Neher, D. A., Weicht, T. R., Millner, P. D., Sharma, M., and Donnelly, C. (2022). Differential survival of Escherichia coli and Listeria spp. in northeastern U.S. soils amended with dairy manure compost, poultry litter compost, and heat-treated poultry pellets and fate in raw edible radish crops. J. Food Prot. 85, 1708–1715. doi: 10.4315/JFP-21-261
Linke, K., Rückerl, I., Brugger, K., Karpiskova, R., Walland, J., Muri-Klinger, S., et al. (2014). Reservoirs of Listeria species in three environmental ecosystems. Appl. Environ. Microbiol. 80, 5583–5592. doi: 10.1128/AEM.01018-14
Liu, D.. (2008). Handbook of Listeria monocytogenes. Boca Raton, Florida, USA: CRC Press Taylor & Francis Group.
Locatelli, A., Spor, A., Jolivet, C., Piveteau, P., and Hartmann, A. (2013). Biotic and abiotic soil properties influence survival of Listeria monocytogenes in soil. PLoS One 8:e75969. doi: 10.1371/journal.pone.0075969
Lotter, D. W. (2003). Organic agriculture. J. Sustain. Agric. 21, 59–128. doi: 10.1300/J064v21n04_06
Luttikholt, L. W. M. (2007). Principles of organic agriculture as formulated by the International Federation of Organic Agriculture Movements. NJAS Wagening. J. Life Sci. 54, 347–360. doi: 10.1016/S1573-5214(07)80008-X
Lyautey, E., Lapen, D. R., Wilkes, G., McCleary, K., Pagotto, F., Tyler, K., et al. (2007). Distribution and characteristics of Listeria monocytogenes isolates from surface waters of the south Nation River watershed, Ontario. Canada. Appl. Environ. Microbiol. 73, 5401–5410. doi: 10.1128/AEM.00354-07
McLaughlin, H. P., Casey, P. G., Cotter, J., Gahan, C. G. M., and Hill, C. (2011). Factors affecting survival of Listeria monocytogenes and Listeria innocua in soil samples. Arch. Microbiol. 193, 775–785. doi: 10.1007/s00203-011-0716-7
Nicholson, F. A., Groves, S. J., and Chambers, B. J. (2005). Pathogen survival during livestock manure storage and following land application. Bioresour. Technol. 96, 135–143. doi: 10.1016/j.biortech.2004.02.030
Nightingale, K. K., Schukken, Y. H., Nightingale, C. R., Fortes, E. D., Ho, A. J., Her, Z., et al. (2004). Ecology and transmission of Listeria monocytogenes infecting ruminants and in the farm environment. Appl. Environ. Microbiol. 70, 4458–4467. doi: 10.1128/AEM.70.8.4458-4467.2004
Olaimat, A. N., and Holley, R. A. (2012). Factors influencing the microbial safety of fresh produce: a review. Food Microbiol. 32, 1–19. doi: 10.1016/j.fm.2012.04.016
Oliveira, M., Usall, J., Viñas, I., Solsona, C., and Abadias, M. (2011). Transfer of Listeria innocua from contaminated compost and irrigation water to lettuce leaves. Food Microbiol. 28, 590–596. doi: 10.1016/j.fm.2010.11.004
Pang, H., McEgan, R., Mishra, A., Micallef, S. A., and Pradhan, A. K. (2017). Identifying and modeling meteorological risk factors associated with pre-harvest contamination of Listeria species in a mixed produce and dairy farm. Food Res. Int. 102, 355–363. doi: 10.1016/j.foodres.2017.09.029
Park, S., Navratil, S., Gregory, A., Bauer, A., Srinath, I., Szonyi, B., et al. (2014). Farm management, environment, and weather factors jointly affect the probability of spinach contamination by generic Escherichia coli at the preharvest stage. Appl. Environ. Microbiol. 80, 2504–2515. doi: 10.1128/AEM.03643-13
Patterson, L., Navarro-Gonzalez, N., Jay-Russell, M. T., Aminabadi, P., Antaki-Zukoski, E., and Pires, A. F. A. (2018). Persistence of Escherichia coli in the soil of an organic mixed crop-livestock farm that integrates sheep grazing within vegetable fields. Zoonoses Public Health 65, 887–896. doi: 10.1111/zph.12503
Phan-Thien, K., Metaferia, M. H., Bell, T. L., Bradbury, M. I., Sassi, H. P., Ogtrop, F. F., et al. (2020). Effect of soil type and temperature on survival of Salmonella enterica in poultry manure-amended soils. Lett. Appl. Microbiol. 71, 210–217. doi: 10.1111/lam.13302
Pires, A. F. A., Millner, P. D., Baron, J., and Jay-Russell, M. T. (2018). Assessment of current practices of organic farmers regarding biological soil amendments of animal origin in a multi-regional US study. Food Prot. Trends 35, 347–362.
Pires, A. F. A., Ramos, T. D. M., Baron, J. N., Millner, P. D., Pagliari, P. H., Hutchinson, M., et al. (2023). Risk factors associated with the prevalence of Shiga-toxin-producing Escherichia coli in manured soils on certified organic farms in four regions of the USA. Front. Sustain. Food Syst. 7:1125996. doi: 10.3389/fsufs.2023.1125996
Pohl, A. M., Pouillot, R., Bazaco, M. C., Wolpert, B. J., Healy, J. M., Bruce, B. B., et al. (2019). Differences among incidence rates of invasive listeriosis in the U.S. food net population by age, sex, race/ethnicity, and pregnancy status, 2008–2016. Foodborne Pathog. Dis. 16, 290–297. doi: 10.1089/fpd.2018.2548
Ramos, T. D. M., Jay-Russell, M. T., Millner, P. D., Baron, J. N., Stover, J., Pagliari, P., et al. (2021). Survival and persistence of foodborne pathogens in manure-amended soils and prevalence on fresh produce in certified organic farms: a multi-regional baseline analysis. Front. Sustain. Food Syst. 5:674767. doi: 10.3389/fsufs.2021.674767
Ramos, T. M., Jay-Russell, M. T., Millner, P. D., Shade, J., Misiewicz, T., Sorge, U. S., et al. (2019). Assessment of biological soil amendments of animal origin use, research needs, and extension opportunities in organic production. Front. Sustain. Food Syst. 3:73. doi: 10.3389/fsufs.2019.00073
Rana, J., and Paul, J. (2017). Consumer behavior and purchase intention for organic food: a review and research agenda. J. Retail. Consum. Serv. 38, 157–165. doi: 10.1016/j.jretconser.2017.06.004
R Core Team (2022). R: A language and environment for statistical computing. Available at: https://www.R-project.org/.
Robin, X., Turck, N., Hainard, A., Tiberti, N., Lisacek, F., Sanchez, J.-C., et al. (2011). pROC: an open-source package for R and S+ to analyze and compare ROC curves. BMC Bioinform 12:77. doi: 10.1186/1471-2105-12-77
Rosen, C. J., and Allan, D. L. (2007). Exploring the benefits of organic nutrient sources for crop production and soil quality. Hort Technol 17, 422–430. doi: 10.21273/HORTTECH.17.4.422
Rosen, C. J., and Bierman, P. M. (2005). Nutrient management for commercial fruit and vegetable crops in Minnesota. Available at: https://hdl.handle.net/11299/197955.
Sauders, B. D., Durak, M. Z., Fortes, E., Windham, K., Schukken, Y., Lembo, A. J., et al. (2006). Molecular characterization of Listeria monocytogenes from natural and urban environments. J. Food Prot. 69, 93–105. doi: 10.4315/0362-028X-69.1.93
Schmit, T. M., Wall, G. L., Newbold, E. J., and Bihn, E. A. (2020). Assessing the costs and returns of on-farm food safety improvements: a survey of good agricultural practices (GAPs) training participants. PLoS One 15:e0235507. doi: 10.1371/journal.pone.0235507
Sharma, M., Millner, P. D., Hashem, F., Vinyard, B. T., East, C. L., Handy, E. T., et al. (2019). Survival of Escherichia coli in manure-amended soils is affected by spatiotemporal, agricultural, and weather factors in the mid-Atlantic United States. Appl. Environ. Microbiol. 85, e02392–e02318. doi: 10.1128/AEM.02392-18
Smith, A., Moorhouse, E., Monaghan, J., Taylor, C., and Singleton, I. (2018). Sources and survival of Listeria monocytogenes on fresh, leafy produce. J. Appl. Microbiol. 125, 930–942. doi: 10.1111/jam.14025
Standing, T.-A., du Plessis, E., Duvenage, S., and Korsten, L. (2013). Internalisation potential of Escherichia coli O157:H7, Listeria monocytogenes, Salmonella enterica subsp. enterica serovar typhimurium and Staphylococcus aureus in lettuce seedlings and mature plants. J. Water Health 11, 210–223. doi: 10.2166/wh.2013.164
Strawn, L. K., Fortes, E. D., Bihn, E. A., Nightingale, K. K., Gröhn, Y. T., Worobo, R. W., et al. (2013a). Landscape and meteorological factors affecting prevalence of three food-borne pathogens in fruit and vegetable farms. Appl. Environ. Microbiol. 79, 588–600. doi: 10.1128/AEM.02491-12
Strawn, L. K., Gröhn, Y. T., Warchocki, S., Worobo, R. W., Bihn, E. A., and Wiedmann, M. (2013b). Risk factors associated with Salmonella and Listeria monocytogenes contamination of produce fields. Appl. Environ. Microbiol. 79, 7618–7627. doi: 10.1128/AEM.02831-13
Townsend, A., Strawn, L. K., Chapman, B. J., and Dunn, L. L. (2021). A systematic review of Listeria species and Listeria monocytogenes prevalence, persistence, and diversity throughout the fresh produce supply chain. Foods 10:1427. doi: 10.3390/foods10061427
USDA NOP (2018). Guidance processed animal manures in organic crop production. Available at: https://www.ams.usda.gov/sites/default/files/media/5006.pdf.
Vogel, B. F., Hansen, L. T., Mordhorst, H., and Gram, L. (2010). The survival of Listeria monocytogenes during long term desiccation is facilitated by sodium chloride and organic material. Int. J. Food Microbiol. 140, 192–200. doi: 10.1016/j.ijfoodmicro.2010.03.035
Weis, J., and Seeliger, H. P. R. (1975). Incidence of Listeria monocytogenes in nature. Appl. Microbiol. 30, 29–32. doi: 10.1128/am.30.1.29-32.1975
Weller, D., Brassill, N., Rock, C., Ivanek, R., Mudrak, E., Roof, S., et al. (2020). Complex interactions between weather, and microbial and physicochemical water quality impact the likelihood of detecting foodborne pathogens in agricultural water. Front. Microbiol. 11:134. doi: 10.3389/fmicb.2020.00134
Weller, D., Shiwakoti, S., Bergholz, P., Grohn, Y., Wiedmann, M., and Strawn, L. K. (2016). Validation of a previously developed geospatial model that predicts the prevalence of Listeria monocytogenes in New York state produce fields. Appl. Environ. Microbiol. 82, 797–807. doi: 10.1128/AEM.03088-15
Weller, D., Wiedmann, M., and Strawn, L. K. (2015). Spatial and temporal factors associated with an increased prevalence of Listeria monocytogenes in spinach fields in New York state. Appl. Environ. Microbiol. 81, 6059–6069. doi: 10.1128/AEM.01286-15
Whipps, J. M., Hand, P., Pink, D. A. C., and Bending, G. D. (2008). “Chapter 7 human pathogens and the phyllosphere” in Advances in applied microbiology. 64, 183–221. doi: 10.1016/S0065-2164(08)00407-3
Willer, H., and Lernoud, J. (2017). The world of organic agriculture. Statistics and emerging trends 2017. Version 1.3. Frick, Switzerland and Bonn, Germany: Research Institute of Organic Agriculture (FiBL), Frick, and IFOAM–organics international.
Keywords: biological soil amendments, foodborne pathogens, soil, raw manure, organic production, fresh produce, Listeria monocytogenes
Citation: Chandler-Khayd C, Di Francesco J, Baron JN, Ramos TDM, Aminabadi P, Jay-Russell MT, Haghani V, Millner PD, Pagliari PH, Hutchinson M, Kenney A, Hashem F, Martínez-López B, Bihn EA, Clements DP, Shade JB, Sciligo AR and Pires AFA (2023) Risk factors associated with the prevalence of Listeria monocytogenes in manured soils on certified organic farms in four regions of the United States. Front. Sustain. Food Syst. 7:1222192. doi: 10.3389/fsufs.2023.1222192
Edited by:
Arun K. Bhunia, Purdue University, United StatesReviewed by:
Daniel Lowell Weller, University of Rochester Medical Center, United StatesLi Maria Ma, Oklahoma State University, United States
Copyright © 2023 Chandler-Khayd, Di Francesco, Baron, Ramos, Aminabadi, Jay-Russell, Haghani, Millner, Pagliari, Hutchinson, Kenney, Hashem, Martínez-López, Bihn, Clements, Shade, Sciligo and Pires. This is an open-access article distributed under the terms of the Creative Commons Attribution License (CC BY). The use, distribution or reproduction in other forums is permitted, provided the original author(s) and the copyright owner(s) are credited and that the original publication in this journal is cited, in accordance with accepted academic practice. No use, distribution or reproduction is permitted which does not comply with these terms.
*Correspondence: Alda F. A. Pires, YXBpcmVzQHVjZGF2aXMuZWR1