- 1Department of Chemistry, College of Science, University of Hafr Al Batin, Hafr Al Batin, Saudi Arabia
- 2School of Chemistry, University of the Punjab, Lahore, Pakistan
Aflatoxins, a group of mycotoxins, represent a heterogeneous class of secondary metabolites that pose a significant risk to food safety and public health due to their potent toxicity. Aflatoxins are widely distributed in the environment, with high levels frequently observed in hot and humid conditions. There is an ongoing development of various methods for detecting aflatoxins in food and feed samples. Herein, a review of these methods is presented with special emphasis on molecularly imprinted polymers (MIPs) as selective materials for aflatoxins’ detection. The key findings of various methods for real-time analysis of food and feed samples are presented and analyzed, providing a comparative assessment of their performance. Furthermore, the challenges and limitations of these methods are discussed, considering their commercialization prospects and real-world requirements.
1. Introduction
Mycotoxins are a diverse group of extremely poisonous secondary metabolites produced by certain species of fungi (molds), such as Aspergillus, Fusarium, and Penicillium (Kumar et al., 2017; Mahato et al., 2019). Aspergillus molds produce the most potent carcinogens known as aflatoxins (Richard, 2007). This class of mycotoxins includes naturally occurring aflatoxins produced by Aspergillus molds such as AFB1, AFG1, AFB2, and AFG2, and their derivatives such as AFM1 and AFM2 (Tahir et al., 2018). Although there are ~20 different types of aflatoxins, the two main groups, i.e., B and G, are commonly found in contaminated food samples. The designation of B and G groups is based on their Bluish and Greenish fluorescence properties (Kardani et al., 2023). The M group aflatoxins are formed as a result of the hydroxylation of AFB1 and AFB2 correspondingly (Yadav et al., 2021). Aflatoxins infect a wide variety of food products including crops, dates, cereals and cereal-containing foods, dried fruits, coffee beans, cocoa, bakery items, and most importantly eggs, milk, and meat obtained from animals fed with contaminated feeds (Wogan, 1966; Jafari, 2018; Almaghrabi, 2022; Ozcelikay et al., 2022).
Aflatoxins are closely related compounds with slight differences in their chemical composition. They consist of chemical compounds that are chemically similar to one another yet have subtle differences. The bluish and green fluorescence properties of the B and G groups are a result of their corresponding cyclopentane and lactone rings, respectively (Liu et al., 2020). The G family of aflatoxins has 3 lactone rings compared to a cyclopentenone ring of the B family. Additionally, AFB1 and AFG1 include an 8,9-double bond in the form of a vinyl ether at the terminal furan ring, but AFB2 and AFG2 do not (Jaimez et al., 2000), as shown in Figure 1A. The order of their toxicity is AFB1 > AFG1 > AFB2 > AFG2. AFM1 and AFM2 have low toxicity (Tahir et al., 2018). AFB1 is considered the most toxic aflatoxin and it is assumed that the main mechanism involved in its carcinogenicity is epoxidation resulting in the formation of AFB1-8,9-epoxides that strongly interact with DNA (Shan, 2019).
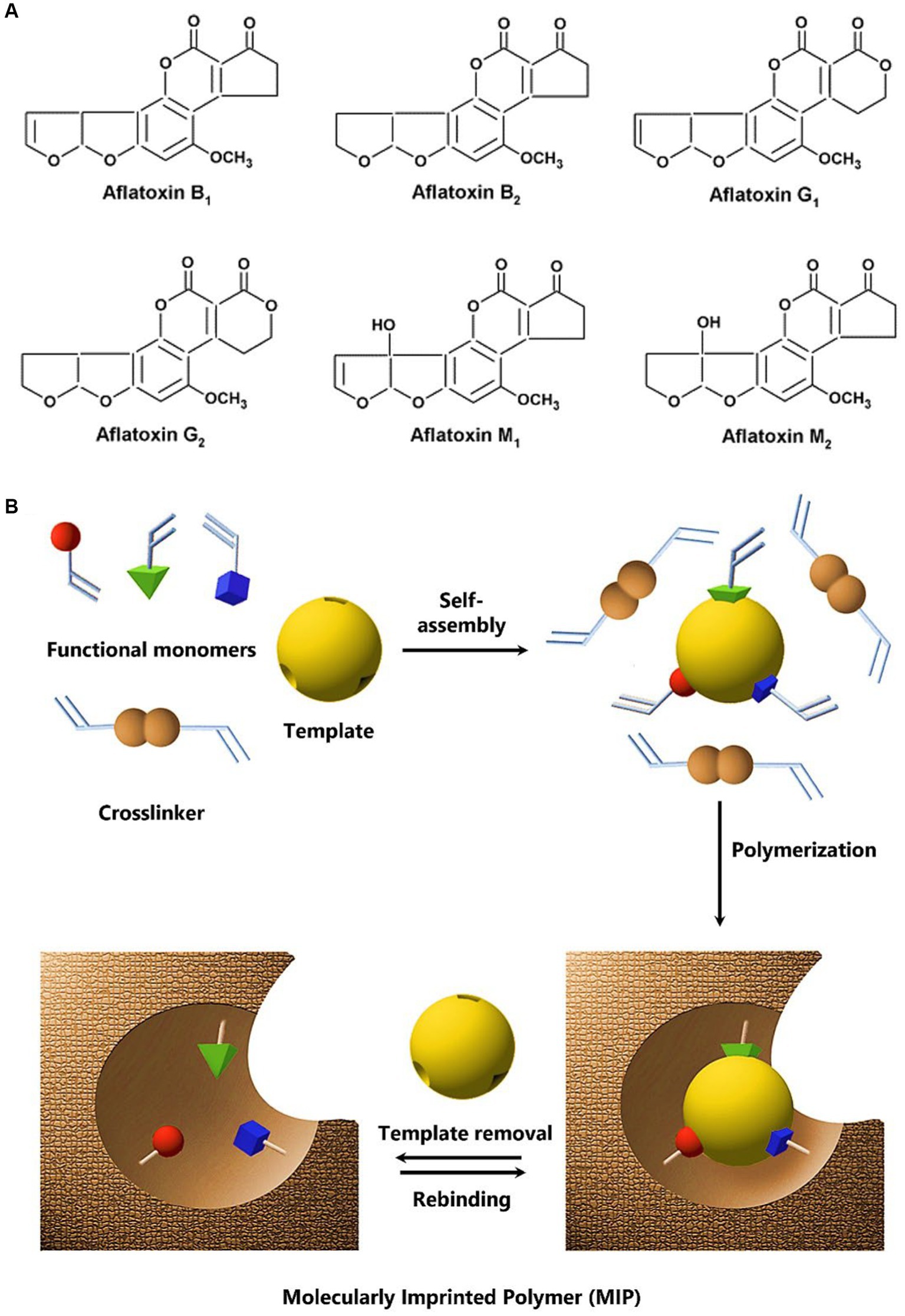
Figure 1. (A) Chemical structure of various aflatoxins. (B) The principle of non-covalent molecular imprinting: functional monomers self-assemble around the template molecule through non-covalent interactions, followed by polymerization in the existence of a crosslinker that results in the formation of a three-dimensional polymer network structure with embedded imprinted sites. Upon removal of the template molecule, the resulting binding cavities or imprints exhibit specific recognition and binding capabilities toward the target molecule. Adapted with permission from Haupt (2003); Copyright American Chemical Society.
International Agency for Research on Cancer (IARC) has declared AFB1 as a group I cancer-causing agent (Marchese et al., 2018). AFB1 is also a naturally occurring fatal liver carcinogen. It is toxic to humans, non-human primates, rodents, poultry, and fish. For instance, AFB1 is found to be the most common hepatotoxicant in fish feed originating from plants, resulting in the mortality of fish (Dirican, 2015). AFB1, in its epoxide form, binds to DNA, RNA, and proteins with carcinogenic effects (Bbosa et al., 2013; Hamid et al., 2013). De Vries et al. (1989) conducted a diagnostic investigation on 125 pregnant women in Kenya and reported that 54% of women’s blood was contaminated with aflatoxins resulting in stunted growth of newborn children, while 37% of aflatoxins were also present in umbilical cord blood. The aflatoxins’ influence on growth impairment in human children and animals is widely reported and reviewed (Khlangwiset et al., 2011; Khoshpey et al., 2011; Smith et al., 2017).
Aflatoxins, in a higher dose, are toxic to animal cells and can cause histological changes at low doses (Qian et al., 2014). On long-term exposure to aflatoxins, tumors may form (Wogan, 1966). Owing to their carcinogenicity and hepatotoxicity, many scientists are working to develop new and innovative methods for detecting these toxins in food and feed samples (Chauhan et al., 2016; Hatamabadi et al., 2020; Hua et al., 2022). Separation and purification of aflatoxins from food products are possible due to their fluorescent behavior in ultraviolet light (Zhang and Banerjee, 2020). They are lipophilic in nature and soluble in moderately polar solvents such as dimethyl sulfoxide, methanol, and chloroform. Previously, these compounds were easily separated by using methanol: water as a solvent (Urano et al., 1993).
Thin-layer chromatography (TLC) with LOD of 1.133 ng/band (Pradhan and Ananthanarayan, 2020; Salisu et al., 2021) and high-performance liquid chromatography (HPLC) with LOD of 0.012 ng/mL (Saini and Abdel-Rehim, 2020; Shuib and Saad, 2022) are commonly reported techniques for the separation of aflatoxins from other secondary metabolites and their subsequent analysis. On the other side, immunochemical methods exhibited LOD values down to 0.12 pg/mL and include radioimmunoassay (RIA), enzyme-linked immunosorbent assay (ELISA), and immunoaffinity column assay (ICA), which take advantage of the affinity of monoclonal or polyclonal antibodies for the identification and quantification of aflatoxins in agricultural products (Gross et al., 2019; Raysyan et al., 2020; Zhan et al., 2021). Solid phase extraction (SPE) with 0.0048 ng/g LOD (Yu et al., 2019; Chen et al., 2021) and optical methods (Kim et al., 2022) are also used for the analysis and quantification of aflatoxins in agricultural products. However, before analysis, the sample should be cleaned to avoid interference during the application of any analysis technique. These conventional methods reveal a low limit of detection (LOD), greater selectivity, and precision, but they are non-portable, expensive, time-consuming, and need strict supervision, which limit their practical applications.
Molecularly imprinted polymer (MIP) sensors with optical, electrochemical, and mass-sensitive signal transduction mechanisms are non-destructive, rapid, cost-effective, easy-to-operate devices that need minimal sample preparation, thereby appearing as excellent alternatives for aflatoxins detection in agricultural products (Hua et al., 2022). MIP sensors have a high degree of tolerance for harsh environmental influence. Alongside remarkable molecular recognition capabilities, MIPs resist high temperature and pressure, and mechanical strain, and are stable in various solvents, acids, and bases (Andersson et al., 1993; Mosbach and Haupt, 1998; Haupt and Mosbach, 2000; Zhang et al., 2006; Ye and Mosbach, 2008). Compared to natural or biological receptors that possess natural identification sites for aflatoxins, MIPs have a longer shelf-life and can be used again and again without being damaged or losing information and sensitivity (Kempe et al., 1993; Andersson, 2000; Chen et al., 2011, 2016). Herein, we present a succinct review of the recent progress in MIP sensors toward real-time quantification of aflatoxins in food and feed samples.
2. Molecular imprinting
Synthesis of a polymer in the presence of a non-reactive target molecule, i.e., the template, results in the formation of molecular recognition sites, a process known as molecular imprinting (Haupt et al., 2011; Haupt, 2012). Figure 1B presents a schematic of the principle of non-covalent molecular imprinting (Haupt, 2003). The polymerization process maintains the spatial configuration of functional monomers and the template molecule, which is further stabilized by crosslinking. So, the subsequent MIP can recognize the target analyte selectively at sites that were inferred from the template (Ahmad et al., 2019). MIPs are synthetic polymers that have been engineered to have a specific recognition function for a particular target such as aflatoxins (Wang et al., 2020). In principle, MIPs can be fabricated for any analyte of concern including small organic molecules to biological macromolecules such as proteins, and microorganisms (Altintas, 2016; Haupt et al., 2020).
Instead of direct imprinting of target molecules, MIPs can be designed using structural analogs or dummy templates. For instance, due to toxicity and high cost of aflatoxins, 5,7-dimethoxycoumarin (DMC) (Guo et al., 2019), quercetin (Liang et al., 2019), 7-acetoxy-4-methylcoumarin (Rui et al., 2019), and 6-phenyl-4-methylchroman-2-one (Song et al., 2019) have been used as dummy template molecules for the synthesis of MIPs. DMC is the most common dummy template for AFB1 (He et al., 2021). MIP synthesis requires a template molecule, functional monomers, and a crosslinker to create selective and responsive materials, as shown in Figure 1B. Sergeyeva et al. (2017) created a virtual library of 24 organofunctional monomers that interact with AFB1 and AFB2 via non-covalent bonding to test their efficacy. Acrylamide is one of the potent monomers that can interact well with AFB1 (Kamaruzaman et al., 2021). Thus, molecular imprinting provides plentiful opportunities in the design of an efficient MIP sensor to ensure the monitoring of aflatoxins in real samples.
MIPs can be produced using various methods such as bulk or surface imprinting, suspension, emulsion, and electropolymerization. For instance, Díaz-Bao et al. (2016) used bulk polymerization method to prepare an AF-MIP using 5,7-dimethoxycoumarin (DMC) as a template, methacrylic acid (MAA) as a monomer, ethylene glycol dimethacrylate (EGDMA) as crosslinker, 2,2′-azobis-(2-methylbutyronitrile) (AIBN) as initiator and toluene/methanol (90,10) as porogen. The developed MIP was applied to extract aflatoxins from spiked samples of baby formulas and cereal-based baby food. In another report, Song et al. (2019) prepared MIPs by suspension polymerization by applying 6-methyl-4-phenylchroman-2-one as the pseudo-template, methacrylic acid, and glycidyl methacrylate as the co-monomers, 2,2′-azobisisobutyronitrile as initiator and polyvinyl alcohol as stabilizing agent. The MIPs produced have a recovery rate of 96% and demonstrate effective performance in detecting AFB1 in soy sauce.
3. MIP sensors for aflatoxins
3.1. Electrochemical sensors
Electrochemical sensors measure the concentration of target molecules and translate chemical information into an electrical signal in the form of a change in current or potential (Suryanarayanan et al., 2010; Karimi-Maleh et al., 2020). Other than a transduction principle, electrochemistry or electrochemical devices serves as an important tool for the fabrication of MIPs. Hutchins and Bachas (1995) were the first to report an electrochemically synthesized MIP. They developed a potentiometric sensor for the nitrate ions and demonstrated the influence of electrochemical elements on nitrate identification but experienced much intervention from hydroxide and thiocyanate ions. Later, electropolymerization of several molecularly imprinted conductive polymers remained an attractive approach to bind MIP particles with the transducer surface (Moreira Gonçalves, 2021). Nevertheless, the electrochemical synthesis of MIPs is beyond the scope of current work, and this section mainly focuses on MIP-based electrochemical sensors for aflatoxins and their analytical performance outcomes.
For instance, Jiang et al. (2015) fabricated an electrochemical sensor for highly specific, precise, and sensitive detection of AFB1 via electropolymerization of p-aminothiophenol (PATP)-modified gold nanoparticles in the presence of AFB1 as the template. Linear sweep voltammetry (LSV) was employed for the detection of AFB1 in an electrolyte solution containing a redox probe, i.e., [Fe(CN)6]3−/4–. The electrochemical sensor revealed a linear response in the concentration range of 1 fg/mL–1 μg/mL along with a very low limit of quantification (LOQ) equivalent to 0.3 fg/mL. Compared to the non-imprinted electrochemical sensor, the MIP sensor exhibit 10-fold increased sensitivity towards AFB1 (Jiang et al., 2015).
El Hassani et al. (2020) developed AFB2 sensors either by electrochemically depositing ZnO nanoparticles on screen-printed gold electrodes and covering them with chitosan and AFB2 (CS-ZnO) or by depositing another layer of AFB2-imprinted polypyrrole (PPy/CS-ZnO). They reported extremely low LODs of 1.9 and 0.6 fM with CS-ZnO and PPy/CS-ZnO sensors, respectively. The sensors also demonstrated good recovery (86–99%) of aflatoxins in milk samples. Recently, Wood and Mugo (2022) fabricated a hypodermic needle-based electrochemical sensor with DMC-imprinted polyaniline at multiwalled carbon nanotubes and cellulose nanocrystals infused on the surface of a needle. The sensor was used to detect AFB1 in real milk samples spiked with a known concentration of aflatoxins and revealed exceptional sensitivity toward AFB1 with a 3 nM LOD.
Photoelectrochemical (PEC) sensor makes use of light to initiate an electrochemical reaction on the MIP surface that results in an electrical signal, e.g., a change in photocurrent, that is proportional to light intensity and can be used to determine the concentration of aflatoxins in a sample. PEC sensors have found applications in various fields, including environmental monitoring, biomedical sensing, and industrial process control (Shi et al., 2019; Qiu and Tang, 2020). The most recent reports suggest that MIP-PEC devices can detect AFB1 and other mycotoxins in food and feed samples with great accuracy and specificity (Mao et al., 2023; Wu et al., 2023). For instance, Mao et al. (2023) created water-stable CsPbBr3 perovskite quantum dots embedded on reduced graphene oxide (rGO) and rolled into CsPbBr3/rGO nanoscrolls by a solvent-assisted self-rolling process. Molecularly imprinted poly(methacrylic acid) thin film was then deposited on CsPbBr3/rGO/ITO electrodes to detect 0.001–1,000 ng/mL AFB1. The MIP-PEC sensor exhibited <1 pg/mL LOD and a 92.0%–109.4% recovery of AFB1 with a relative standard deviation (RSD = 1.3%–6.1%).
3.2. Optical sensors
Optical sensors involve a broad class of detectors that can analyze the optical characteristics of a substance, e.g., phosphorescence (Madurangika Jayasinghe et al., 2020), fluorescence (Chmangui et al., 2019; Guo et al., 2019), scattering (Fan et al., 2023), refractive index, etc., and transform them into an electronic signal. By monitoring the optical responses resulting from the formation of a MIP-analyte complex, MIP optical sensors offer a practical and diverse solution for monitoring small molecules (Saylan et al., 2019; Fang et al., 2021). Yarynka et al. (2021) and Sergeyeva et al. (2022) published several reports on the optical/fluorescent detection of aflatoxins. They developed a smartphone-based optical biomimetic sensor using MIPs from acrylamide and 2-acrylamido-2-methyl-1-propansulfonic acid monomers that could selectively detect AFB1 in wheat and maize flour samples (Sergeyeva et al., 2019). Recently, Chi and Liu (2023) utilized silanes (tetraethoxysilane, and aminopropyltriethoxy silane) as functional monomers and aptamer-modified CdTe/ZnS quantum dots as an optical signal probe to prepare a fluorometric sandwich biosensor. The sensor was used to monitor AFB1 levels in various edible oils, e.g., peanut, corn, and olive, and exhibited good reliability down to 4.0 pg/mL. A similar method was reported earlier but with lower sensitivity (Guo et al., 2019).
On the other hand, surface plasmon resonance (SPR) sensors translate surface-bound analyte interactions into a change in the refractive index (Wei et al., 2019; Akgönüllü et al., 2022). The phenomenon is observed when incident light reaches a metal film, i.e., usually Au or Ag, at the interface of media with different refractive indices. Akgönüllü et al. (2020, 2021) fabricated MIP-SPR sensors for the detection of AFB1 and AFM1 using hydroxyethyl methacrylate (HEMA) and N-methacryloyl-1-phenylalanine (MAPA) as functional monomers, respectively. They integrated Au nanoparticles with MIP-SPR sensors to demonstrate excellent LOD of 1.04 pg/mL for AFB1 in peanut and corn samples, and 0.4 pg/mL for AFM1 in milk samples. Hence, these MIP-SPR sensor systems provide an efficient point-of-care solution for food safety checks.
3.3. Mass sensors
A mass-sensitive transducer such as a quartz crystal microbalance (QCM) has been combined with MIPs to monitor aflatoxins (Susilo et al., 2021). The gadget measures surface-analyte interactions by monitoring the changes in resonance frequency (Mujahid et al., 2019), and has several advantages including high sensitivity and real-time output. In recent years, QCM-based sensors have emerged as a potentially useful technology for the investigation of molecular interactions on solid surfaces and the measurement of chemicals and biomolecules (Nasrullah et al., 2021, 2022). With the use of Au nanoparticles doped molecularly imprinted poly(2-aminothiophenol) layer and covalent organic frameworks (COF), Gu et al. (2019) developed a QCM sensor for the detection of AFB1. The crosslinked MIP/COF-Au layer contained recognition sites that allowed selective detection of AFB1, while COF provided a greater surface area and enhanced sensitivity. The sensor could detect AFB1 down to 2.8 pg./mL level. Also, the minimum recovery of AFB1 in real samples including peanuts, pistachio, rice, and wheat was in the range of 87–95.1%, which demonstrated the sensor’s potential for real-time monitoring of aflatoxins. Albeit, MIP-QCM sensors offer label-free detection of aflatoxins and can be miniaturized or fabricated in an array to simultaneously detect multiple analytes (Iqbal et al., 2010), they are not researched enough to monitor multiple aflatoxins in solution or food/feed samples.
4. Limitations and challenges
A performance comparison of different types of MIP sensors is provided in Table 1. Evidently, in the recent past, the designed MIP sensors have realized great results in the laboratory and with real samples. These include a broad linear detection range, e.g., from 10−15 to 10−6 mol/L (Jiang et al., 2015), very low LOD, i.e., <1 fg/mL (El Hassani et al., 2020), and identification and a minimum of >98% recovery of AFB1 from real samples (Mao et al., 2021; Wang et al., 2021; Chen et al., 2023). Hence, MIP sensors exhibit superior results compared to certain analytical and spectroscopic methods such as surface-enhanced Raman spectroscopy (Fan et al., 2023), phosphorescence (Madurangika Jayasinghe et al., 2020), and fluorescence spectroscopy (Chmangui et al., 2019; Guo et al., 2019). Particularly, the use of MIP in electrochemical sensors has demonstrated high sensitivity, selectivity, ease of fabrication, and utility, making them a promising option for field use. However, we are still far from the practical realization of these MIP-electrochemical sensors for in-field analysis of crops and/or monitoring food and feed quality.
The major challenges of MIP sensors include the following: (a) the steps and materials involved in the synthesis and fabrication of MIP-based selective materials that could increase cost and reduce the reproducibility of the devices; (b) the need for significant quantities of the target analyte during MIP synthesis that poses a health hazard because of the potent toxicity of aflatoxins; and (c) the difficulty in regenerating sensors, i.e., difficulty in removing the template that reduces the reusability of devices. On the other hand, some inherent limitations of bulk polymerization to synthesize MIPs include the uneven distribution of recognition sites and residual analyte traces after MIP creation, which may hinder the reproducibility of the devices and results, whereas the decay of recognition sites over time could affect the devices’ stability and shelf-life. Furthermore, the cost and toxicity of the nanostructured materials integrated with MIPs to enhance their sensing properties may also challenge their commercial prospects.
To overcome these limitations and challenges, the researchers must develop novel, non-toxic, and greener methods for the synthesis of MIPs and nanomaterials that are stable, easy to fabricate, and reusable for practical applications. While combining MIPs with various functional nanomaterials can enhance the sensitivity of various MIP sensors, developing new synthetic methodologies can expedite MIP synthesis without compromising their sensitivity and selectivity. Computational approaches may help identify more suitable and interactional monomers for different aflatoxins depending on the structure and affinity of the pendent functional groups (Sergeyeva et al., 2019, 2022). Various templating strategies, i.e., direct or dummy templates, and their competing benefits need to be understood to optimize sensor response and avoid selectivity issues.
On the other hand, developing disposable MIP sensors can solve several issues. For example, the need to regenerate sensors or imprinted cavities, and reusability problems would be prevented along with the reduced cost of the device, which again can be achieved using electrochemical principles. Overall, the development and implementation of MIP-based electrochemical sensors have the potential to improve their effectiveness and usefulness, especially if combined with novel nanomaterials and new synthesis methodologies. The ultimate goal should be to create MIP-modified disposable nanosensors that can be applied to the in-field analysis of food and feed samples and routine monitoring of food quality and that have a broader commercialization scope.
5. Outlook
Aflatoxins are highly toxic carcinogenic substances that can contaminate agricultural products, posing a significant threat to human health. The conventional methods used for detecting aflatoxins in agricultural products are limited by their lack of portability, high cost, meticulous surveillance, and destructive analysis. MIP sensors offer a high degree of selectivity towards targeted toxins and can tolerate harsh environmental influences during in-field analysis. Among MIP sensors, electrochemical sensors are the most efficient tools for detecting aflatoxins in agricultural products, and MIPs in combination with other nano-sized reinforcements can further boost their sensitivity. MIP sensors also offer several advantages, including quick performance, user-friendly operation, non-tedious sample preparation, and non-destructive analysis. In addition, MIP sensors utilize a wide range of signal-transducing mechanisms and tailor-made binding sites, which results in high recognition specificity and sensitivity with picomolar-femtomolar LODs. MIPs combined with optical, SERS, and QCM transducers also hold great potential for detecting aflatoxins and are becoming increasingly popular for detecting these toxins with high sensitivity and accuracy. However, MIP sensors for aflatoxins still require improvements for commercial development and real-world applications such as better reproducibility, recovery of recognition sites, reusability, and affordability. Despite the imperfections of complexity, degradation of recognition sites, and cost-optimization, MIP sensor development holds great promise for the detection and monitoring of aflatoxins in food and feed samples to reduce health risks.
Author contributions
AAl, AM, and AAf contributed to the conception and design of the study. AS, HI, AB, and UH organized the database, conducted the literature survey, and wrote the first draft of the manuscript. NI performed the analysis and compiled data. AAl, AM, NI, and AAf wrote sections of the manuscript. All authors contributed to the article and approved the submitted version.
Funding
This research was funded by the Ministry of Education and the University of Hafr Al Batin, Saudi Arabia, grant number IFP-A-2022-2-3-13.
Acknowledgments
The authors gratefully acknowledge the technical and financial support from the Ministry of Education and the University of Hafr Al Batin, Hafr Al Batin, Saudi Arabia.
Conflict of interest
The authors declare that the research was conducted in the absence of any commercial or financial relationships that could be construed as a potential conflict of interest.
Publisher’s note
All claims expressed in this article are solely those of the authors and do not necessarily represent those of their affiliated organizations, or those of the publisher, the editors and the reviewers. Any product that may be evaluated in this article, or claim that may be made by its manufacturer, is not guaranteed or endorsed by the publisher.
References
Ahmad, O. S., Bedwell, T. S., Esen, C., Garcia-Cruz, A., and Piletsky, S. A. (2019). Molecularly imprinted polymers in electrochemical and optical sensors. Trends Biotechnol. 37, 294–309. doi: 10.1016/j.tibtech.2018.08.009
Akgönüllü, S., Armutcu, C., and Denizli, A. (2022). Molecularly imprinted polymer film based plasmonic sensors for detection of ochratoxin a in dried fig. Polym. Bull. 79, 4049–4067. doi: 10.1007/s00289-021-03699-6
Akgönüllü, S., Yavuz, H., and Denizli, A. (2020). SPR nanosensor based on molecularly imprinted polymer film with gold nanoparticles for sensitive detection of aflatoxin B1. Talanta 219:121219. doi: 10.1016/j.talanta.2020.121219
Akgönüllü, S., Yavuz, H., and Denizli, A. (2021). Development of gold nanoparticles decorated molecularly imprinted–based Plasmonic sensor for the detection of aflatoxin M1 in Milk samples. Chemosensors 9:363. doi: 10.3390/chemosensors9120363
Almaghrabi, M. A. (2022). The occurrence of aflatoxins in date palm (Phoenix dactylifera L.) worldwide. J. Food Qual. 2022:e1326861. doi: 10.1155/2022/1326861
Altintas, Z. (2016). “Advanced imprinted materials for virus monitoring” in Advanced molecularly imprinting materials. eds. A. Tiwari and L. Uzun (Hoboken, NJ: John Wiley & Sons, Ltd), 389–411.
Andersson, L. I. (2000). Molecular imprinting: developments and applications in the analytical chemistry field. J. Chromatogr. B Biomed. Sci. Appl. 745, 3–13. doi: 10.1016/s0378-4347(00)00135-3
Andersson, L. I., Ekberg, B., and Mosbach, K. (1993). “Bioseparation and catalysis in molecularly imprinted polymers” in Molecular interactions in bioseparations. ed. T. T. Ngo (Boson, MA: Springer), 383–394.
Bbosa, G. S., Kitya, D., Lubega, A., Ogwal-Okeng, J., Anokbonggo, W. W., and Kyegombe, D. B. (2013). “Review of the biological and health effects of aflatoxins on body organs and body systems” in Aflatoxins. ed. M. Razzaghi-Abyaneh (London, UK: IntechOpen)
Chauhan, R., Singh, J., Sachdev, T., Basu, T., and Malhotra, B. D. (2016). Recent advances in mycotoxins detection. Biosens. Bioelectron. 81, 532–545. doi: 10.1016/j.bios.2016.03.004
Chen, J., Liu, F., Li, Z., Tan, L., Zhang, M., and Xu, D. (2021). Solid phase extraction based microfluidic chip coupled with mass spectrometry for rapid determination of aflatoxins in peanut oil. Microchem. J. 167:106298. doi: 10.1016/j.microc.2021.106298
Chen, L., Mamat, X., and Aisa, H. A. (2023). Determination of aflatoxin B1 by biomass derived porous carbon modified electrode with molecularly imprinted polymer. Electroanalysis 35:e202200371. doi: 10.1002/elan.202200371
Chen, L., Wang, X., Lu, W., Wu, X., and Li, J. (2016). Molecular imprinting: perspectives and applications. Chem. Soc. Rev. 45, 2137–2211. doi: 10.1039/C6CS00061D
Chen, L., Xu, S., and Li, J. (2011). Recent advances in molecular imprinting technology: current status, challenges and highlighted applications. Chem. Soc. Rev. 40, 2922–2942. doi: 10.1039/c0cs00084a
Chi, H., and Liu, G. (2023). A fluorometric sandwich biosensor based on molecular imprinted polymer and aptamer modified CdTe/ZnS for detection of aflatoxin B1 in edible oil. LWT 180:114726. doi: 10.1016/j.lwt.2023.114726
Chmangui, A., Driss, M. R., Touil, S., Bermejo-Barrera, P., Bouabdallah, S., and Moreda-Piñeiro, A. (2019). Aflatoxins screening in non-dairy beverages by Mn-doped ZnS quantum dots–molecularly imprinted polymer fluorescent probe. Talanta 199, 65–71. doi: 10.1016/j.talanta.2019.02.057
Díaz-Bao, M., Regal, P., Barreiro, R., Fente, C. A., and Cepeda, A. (2016). A facile method for the fabrication of magnetic molecularly imprinted stir-bars: a practical example with aflatoxins in baby foods. J. Chromatogr. A 1471, 51–59. doi: 10.1016/j.chroma.2016.10.022
El Hassani, N. E., Bouchikhi, B., and El Bari, N. (2020). Recent development of an electrochemical imprinted sensor for the detection of trace-level of unmetabolized aflatoxin B2 in dairy milk. J. Electroanal. Chem. 865:114123. doi: 10.1016/j.jelechem.2020.114123
Fan, L., Zhang, Q., Wang, F., and Yang, H. (2023). Dummy molecularly imprinted solid-phase extraction-SERS determination of AFB1 in peanut. Spectrochim. Acta A Mol. Biomol. Spectrosc. 288:122130. doi: 10.1016/j.saa.2022.122130
Fang, L., Jia, M., Zhao, H., Kang, L., Shi, L., Zhou, L., et al. (2021). Molecularly imprinted polymer-based optical sensors for pesticides in foods: recent advances and future trends. Trends Food Sci. Technol. 116, 387–404. doi: 10.1016/j.tifs.2021.07.039
Gross, M., Ploetz, C. P., and Gottschalk, C. (2019). Immunochemical detection of mycotoxins in donkey milk. Mycotoxin Res. 35, 83–87. doi: 10.1007/s12550-018-0333-2
Gu, Y., Wang, Y., Wu, X., Pan, M., Hu, N., Wang, J., et al. (2019). Quartz crystal microbalance sensor based on covalent organic framework composite and molecularly imprinted polymer of poly(o-aminothiophenol) with gold nanoparticles for the determination of aflatoxin B1. Sensors Actuators B Chem. 291, 293–297. doi: 10.1016/j.snb.2019.04.092
Guo, P., Yang, W., Hu, H., Wang, Y., and Li, P. (2019). Rapid detection of aflatoxin B1 by dummy template molecularly imprinted polymer capped CdTe quantum dots. Anal. Bioanal. Chem. 411, 2607–2617. doi: 10.1007/s00216-019-01708-2
Hamid, A. S., Tesfamariam, I. G., Zhang, Y., and Zhang, Z. G. (2013). Aflatoxin B1-induced hepatocellular carcinoma in developing countries: geographical distribution, mechanism of action and prevention (review). Oncol. Lett. 5, 1087–1092. doi: 10.3892/ol.2013.1169
Hatamabadi, D., Mostafiz, B., Beirami, A. D., Banan, K., Moghaddam, N. S. T., Afsharara, H., et al. (2020). Are molecularly imprinted polymers (MIPs) beneficial in detection and determination of mycotoxins in cereal samples? Iran. J. Pharm. Res. 19, 1–18. doi: 10.22037/ijpr.2020.112677.13887
Haupt, K. (2003). Molecularly imprinted polymers: the next generation. Anal. Chem. 75:376A–383A. doi: 10.1021/ac031385h
Haupt, K., Linares, A. V., Bompart, M., and Bui, B. T. S. (2011). “Molecularly imprinted polymers” in Molecular imprinting. ed. K. Haupt (Berlin: Springer), 1–28.
Haupt, K., Medina Rangel, P. X., and Bui, B. T. S. (2020). Molecularly imprinted polymers: antibody mimics for bioimaging and therapy. Chem. Rev. 120, 9554–9582. doi: 10.1021/acs.chemrev.0c00428
Haupt, K., and Mosbach, K. (2000). Molecularly imprinted polymers and their use in biomimetic sensors. Chem. Rev. 100, 2495–2504. doi: 10.1021/cr990099w
He, J.-X., Pan, H.-Y., Xu, L., and Tang, R.-Y. (2021). Application of molecularly imprinted polymers for the separation and detection of aflatoxin. J. Chem. Res. 45, 400–410. doi: 10.1177/1747519820980373
Hua, Y., Ahmadi, Y., Sonne, C., and Kim, K.-H. (2022). Progress and challenges in sensing of mycotoxins using molecularly imprinted polymers. Environ. Pollut. 305:119218. doi: 10.1016/j.envpol.2022.119218
Hutchins, R. S., and Bachas, L. G. (1995). Nitrate-selective electrode developed by electrochemically mediated imprinting/doping of polypyrrole. Anal. Chem. 67, 1654–1660. doi: 10.1021/ac00106a002
Iqbal, N., Mustafa, G., Rehman, A., Biedermann, A., Najafi, B., Lieberzeit, P. A., et al. (2010). QCM-arrays for sensing terpenes in fresh and dried herbs via bio-mimetic MIP layers. Sensors (Basel) 10, 6361–6376. doi: 10.3390/s100706361
Jafari, A. A. (2018). Mycotoxins, a major challenge in global food security. J. Nutr. Food Secur. 3, 1–3.
Jaimez, J., Fente, C. A., Vazquez, B. I., Franco, C. M., Cepeda, A., Mahuzier, G., et al. (2000). Application of the assay of aflatoxins by liquid chromatography with fluorescence detection in food analysis. J. Chromatogr. A 882, 1–10. doi: 10.1016/S0021-9673(00)00212-0
Jiang, M., Braiek, M., Florea, A., Chrouda, A., Farre, C., Bonhomme, A., et al. (2015). Aflatoxin B1 detection using a highly-sensitive molecularly-imprinted electrochemical sensor based on an electropolymerized metal organic framework. Toxins 7, 3540–3553. doi: 10.3390/toxins7093540
Kamaruzaman, S., Nasir, N. M., Mohd Faudzi, S. M., Yahaya, N., Mohamad Hanapi, N. S., and Wan Ibrahim, W. N. (2021). Solid-phase extraction of active compounds from natural products by molecularly imprinted polymers: synthesis and extraction parameters. Polymers 13:3780. doi: 10.3390/polym13213780
Karczmarczyk, A., Dubiak-Szepietowska, M., Vorobii, M., Rodriguez-Emmenegger, C., Dostálek, J., and Feller, K.-H. (2016). Sensitive and rapid detection of aflatoxin M1 in milk utilizing enhanced SPR and p(HEMA) brushes. Biosens. Bioelectron. 81, 159–165. doi: 10.1016/j.bios.2016.02.061
Kardani, F., Mirzajani, R., Tamsilian, Y., Kiasat, A., and Bakhshandeh Farajpour, F. (2023). A novel immunoaffinity column based metal–organic framework deep eutectic solvents @ molecularly imprinted polymers as a sorbent for the solid phase extraction of aflatoxins AFB1, AFB2, AFG1 and AFG2 from cereals samples. Microchem. J. 187:108366. doi: 10.1016/j.microc.2022.108366
Karimi-Maleh, H., Karimi, F., Alizadeh, M., and Sanati, A. L. (2020). Electrochemical sensors, a bright future in the fabrication of portable kits in analytical systems. Chem. Rec. 20, 682–692. doi: 10.1002/tcr.201900092
Kempe, M., Mosbach, K., and Fischer, L. (1993). Chiral separation using molecularly imprinted heteroaromatic polymers. J. Mol. Recognit. 6, 25–29. doi: 10.1002/jmr.300060103
Khlangwiset, P., Shephard, G. S., and Wu, F. (2011). Aflatoxins and growth impairment: a review. Crit. Rev. Toxicol. 41, 740–755. doi: 10.3109/10408444.2011.575766
Khoshpey, B., Farhud, D. D., and Zaini, F. (2011). Aflatoxins in Iran: nature, hazards and carcinogenicity. Iran. J. Public Health 40, 1–30.
Kim, Y.-K., Baek, I., Lee, K.-M., Qin, J., Kim, G., Shin, B. K., et al. (2022). Investigation of reflectance, fluorescence, and Raman hyperspectral imaging techniques for rapid detection of aflatoxins in ground maize. Food Control 132:108479. doi: 10.1016/j.foodcont.2021.108479
Kumar, P., Mahato, D. K., Kamle, M., Mohanta, T. K., and Kang, S. G. (2017). Aflatoxins: a global concern for food safety, human health and their management. Front. Microbiolo. 7:2170. doi: 10.3389/fmicb.2016.02170
Liang, Y., He, J., Huang, Z., Li, H., Zhang, Y., Wang, H., et al. (2019). An amino-functionalized zirconium-based metal-organic framework of type UiO-66-NH2 covered with a molecularly imprinted polymer as a sorbent for the extraction of aflatoxins AFB1, AFB2, AFG1 and AFG2 from grain. Microchim. Acta 187:32. doi: 10.1007/s00604-019-3959-7
Liu, D., Li, W., Zhu, C., Li, Y., Shen, X., Li, L., et al. (2020). Recent progress on electrochemical biosensing of aflatoxins: a review. TrAC Trends Anal. Chem. 133:115966. doi: 10.1016/j.trac.2020.115966
Madurangika Jayasinghe, G. D. T., Domínguez-González, R., Bermejo-Barrera, P., and Moreda-Piñeiro, A. (2020). Room temperature phosphorescent determination of aflatoxins in fish feed based on molecularly imprinted polymer – Mn-doped ZnS quantum dots. Anal. Chim. Acta 1103, 183–191. doi: 10.1016/j.aca.2019.12.060
Mahato, D. K., Lee, K. E., Kamle, M., Devi, S., Dewangan, K. N., Kumar, P., et al. (2019). Aflatoxins in food and feed: an overview on prevalence, detection and control strategies. Front. Microbiol. 10:2266. doi: 10.3389/fmicb.2019.02266
Mao, L., Xiao, Y., Liu, H., Zhang, X., Wang, S., Huang, W.-H., et al. (2023). Water-stable CsPbBr3/reduced graphene oxide nanoscrolls for high-performance photoelectrochemical sensing. Adv. Funct. Mater. 33:2213814. doi: 10.1002/adfm.202213814
Mao, L., Xue, X., Xu, X., Wen, W., Chen, M.-M., Zhang, X., et al. (2021). Heterostructured CuO-g-C3N4 nanocomposites as a highly efficient photocathode for photoelectrochemical aflatoxin B1 sensing. Sensors Actuators B Chem. 329:129146. doi: 10.1016/j.snb.2020.129146
Marchese, S., Polo, A., Ariano, A., Velotto, S., Costantini, S., and Severino, L. (2018). Aflatoxin B1 and M1: biological properties and their involvement in cancer development. Toxins (Basel) 10:214. doi: 10.3390/toxins10060214
Moreira Gonçalves, L. (2021). Electropolymerized molecularly imprinted polymers: perceptions based on recent literature for soon-to-be world-class scientists. Curr. Opin. Electrochem. 25:100640. doi: 10.1016/j.coelec.2020.09.007
Mosbach, K., and Haupt, K. (1998). Some new developments and challenges in non-covalent molecular imprinting technology. J. Mol. Recognit. 11, 62–68. doi: 10.1002/(SICI)1099-1352(199812)11:1/6<62::AID-JMR391>3.0.CO;2-5
Mujahid, A., Afzal, A., and Dickert, F. L. (2019). An overview of high frequency acoustic sensors—QCMs, SAWs and FBARs—chemical and biochemical applications. Sensors 19:4395. doi: 10.3390/s19204395
Nasrullah, A., Afzal, A., Mujahid, A., Lieberzeit, P. A., Bajwa, S. Z., Mustafa, G., et al. (2021). Imprinted polymer and Cu2O-graphene oxide nanocomposite for the detection of disease biomarkers. Meas. Sci. Technol. 32:105111. doi: 10.1088/1361-6501/ac0bdc
Nasrullah, A., Roshan, S., Latif, U., Mujahid, A., Mustafa, G., Bajwa, S. Z., et al. (2022). ZnO nanoparticles and β-cyclodextrin containing molecularly imprinted polymers for gravimetric sensing of very-low-density lipoprotein. Meas. Sci. Technol. 33:045106. doi: 10.1088/1361-6501/ac471c
Ozcelikay, G., Cetinkaya, A., Kaya, S. I., Yence, M., Canavar Eroğlu, P. E., Unal, M. A., et al. (2022). Novel sensor approaches of aflatoxins determination in food and beverage samples. Crit. Rev. Anal. Chem., 1–20. doi: 10.1080/10408347.2022.2105136
Pradhan, S., and Ananthanarayan, L. (2020). Standardization and validation of a high-performance thin-layer chromatography method for the quantification of aflatoxin B1 and its application in surveillance of contamination level in marketed food commodities from the Mumbai region. J. Planar Chromat. 33, 617–630. doi: 10.1007/s00764-020-00073-6
Qian, G., Tang, L., Guo, X., Wang, F., Massey, M. E., Su, J., et al. (2014). Aflatoxin B1 modulates the expression of phenotypic markers and cytokines by splenic lymphocytes of male F344 rats. J. Appl. Toxicol. 34, 241–249. doi: 10.1002/jat.2866
Qiu, Z., and Tang, D. (2020). Nanostructure-based photoelectrochemical sensing platforms for biomedical applications. J. Mater. Chem. B 8, 2541–2561. doi: 10.1039/C9TB02844G
Raysyan, A., Eremin, S. A., Beloglazova, N. V., De Saeger, S., and Gravel, I. V. (2020). Immunochemical approaches for detection of aflatoxin B1 in herbal medicines. Phytochem. Anal. 31, 662–669. doi: 10.1002/pca.2931
Richard, J. L. (2007). Some major mycotoxins and their mycotoxicoses—an overview. Int. J. Food Microbiol. 119, 3–10. doi: 10.1016/j.ijfoodmicro.2007.07.019
Roushani, M., Farokhi, S., and Rahmati, Z. (2022). Development of a dual-recognition strategy for the aflatoxin B1 detection based on a hybrid of aptamer-MIP using a Cu2O NCs/GCE. Microchem. J. 178:107328. doi: 10.1016/j.microc.2022.107328
Rui, C., He, J., Li, Y., Liang, Y., You, L., He, L., et al. (2019). Selective extraction and enrichment of aflatoxins from food samples by mesoporous silica FDU-12 supported aflatoxins imprinted polymers based on surface molecularly imprinting technique. Talanta 201, 342–349. doi: 10.1016/j.talanta.2019.04.019
Saini, S. S., and Abdel-Rehim, M. (2020). Integrated extraction approach for trace analysis of aflatoxin B1 in domestic water tanks using HPLC. Sep. Sci. Plus 3, 167–174. doi: 10.1002/sscp.202000013
Salisu, B., Anua, S. M., Ishak, W. R. W., and Mazlan, N. (2021). Development and validation of quantitative thin layer chromatographic technique for determination of total aflatoxins in poultry feed and food grains without sample clean-up. J. Adv. Vet. Anim. Res. 8, 656–670. doi: 10.5455/javar.2021.h558
Saylan, Y., Akgönüllü, S., Yavuz, H., Ünal, S., and Denizli, A. (2019). Molecularly imprinted polymer based sensors for medical applications. Sensors 19:1279. doi: 10.3390/s19061279
Sergeyeva, T., Yarynka, D., Lytvyn, V., Demydov, P., Lopatynskyi, A., Stepanenko, Y., et al. (2022). Highly-selective and sensitive plasmon-enhanced fluorescence sensor of aflatoxins. Analyst 147, 1135–1143. doi: 10.1039/D1AN02173G
Sergeyeva, T., Yarynka, D., Piletska, E., Linnik, R., Zaporozhets, O., Brovko, O., et al. (2019). Development of a smartphone-based biomimetic sensor for aflatoxin B1 detection using molecularly imprinted polymer membranes. Talanta 201, 204–210. doi: 10.1016/j.talanta.2019.04.016
Sergeyeva, T., Yarynka, D., Piletska, E., Lynnik, R., Zaporozhets, O., Brovko, O., et al. (2017). Fluorescent sensor systems based on nanostructured polymeric membranes for selective recognition of aflatoxin B1. Talanta 175, 101–107. doi: 10.1016/j.talanta.2017.07.030
Shan, Y. (2019). “The toxic effects of aflatoxin B1: an update” in Aflatoxin B1 occurrence, detection and toxicological effects. ed. X. D. Long (London, UK: IntechOpen)
Shi, L., Yin, Y., Zhang, L.-C., Wang, S., Sillanpää, M., and Sun, H. (2019). Design and engineering heterojunctions for the photoelectrochemical monitoring of environmental pollutants: a review. Appl. Catal. B Environ. 248, 405–422. doi: 10.1016/j.apcatb.2019.02.044
Shuib, N. S., and Saad, B. (2022). In-syringe dispersive micro-solid phase extraction method for the HPLC-fluorescence determination of aflatoxins in milk. Food Control 132:108510. doi: 10.1016/j.foodcont.2021.108510
Singh, A. K., Lakshmi, G. B. V. S., Fernandes, M., Sarkar, T., Gulati, P., Singh, R. P., et al. (2021). A simple detection platform based on molecularly imprinted polymer for AFB1 and FuB1 mycotoxins. Microchem. J. 171:106730. doi: 10.1016/j.microc.2021.106730
Smith, L. E., Prendergast, A. J., Turner, P. C., Humphrey, J. H., and Stoltzfus, R. J. (2017). Aflatoxin exposure during pregnancy, maternal Anemia, and adverse birth outcomes. Am. J. Trop. Med. Hyg. 96, 770–776. doi: 10.4269/ajtmh.16-0730
Song, L., He, J., Chen, N., and Huang, Z. (2019). Combined biocompatible medium with molecularly imprinted polymers for determination of aflatoxins B1 in real sample. J. Sep. Sci. 42, 3679–3687. doi: 10.1002/jssc.201900564
Suryanarayanan, V., Wu, C.-T., and Ho, K.-C. (2010). Molecularly imprinted electrochemical sensors. Electroanalysis 22, 1795–1811. doi: 10.1002/elan.200900616
Susilo, M. D. D., Jayadi, T., Kusumaatmaja, A., and Nugraheni, A. D. (2021). Development of molecular imprinting polymer nanofiber for aflatoxin B1 detection based on quartz crystal microbalance. Mater. Sci. Forum 1023, 103–109. doi: 10.4028/www.scientific.net/MSF.1023.103
Tahir, N. I., Hussain, S., Javed, M., Rehman, H., Shahzady, T. G., Parveen, B., et al. (2018). Nature of aflatoxins: their extraction, analysis, and control. J. Food Saf. 38:e12561. doi: 10.1111/jfs.12561
Urano, T., Trucksess, M. W., and Page, S. W. (1993). Automated affinity liquid chromatography system for on-line isolation, separation, and quantitation of aflatoxins in methanol-water extracts of corn or peanuts. J. Agric. Food Chem. 41, 1982–1985. doi: 10.1021/jf00035a031
Vries, H. R. D., Maxwell, S. M., and Hendrickse, R. G. (1989). Foetal and neonatal exposure to aflatoxins. Acta Paediatr. 78, 373–378. doi: 10.1111/j.1651-2227.1989.tb11095.x
Wang, Z., Li, J., Xu, L., Feng, Y., and Lu, X. (2014). Electrochemical sensor for determination of aflatoxin B1 based on multiwalled carbon nanotubes-supported au/Pt bimetallic nanoparticles. J. Solid State Electrochem. 18, 2487–2496. doi: 10.1007/s10008-014-2506-z
Wang, C., Wang, H., Zhang, M., Zeng, B., and Zhao, F. (2021). Molecularly imprinted photoelectrochemical sensor for aflatoxin B1 detection based on organic/inorganic hybrid nanorod arrays. Sensors Actuators B Chem. 339:129900. doi: 10.1016/j.snb.2021.129900
Wang, Q., Yang, Q., and Wu, W. (2020). Progress on structured biosensors for monitoring aflatoxin B1 from biofilms: a review. Front. Microbiol. 11:408. doi: 10.3389/fmicb.2020.00408
Wei, T., Ren, P., Huang, L., Ouyang, Z., Wang, Z., Kong, X., et al. (2019). Simultaneous detection of aflatoxin B1, ochratoxin a, zearalenone and deoxynivalenol in corn and wheat using surface plasmon resonance. Food Chem. 300:125176. doi: 10.1016/j.foodchem.2019.125176
Wogan, G. N. (1966). Chemical nature and biological effects of the aflatoxins. Bacteriol. Rev. 30, 460–470. doi: 10.1128/br.30.2.460-470.1966
Wood, M., and Mugo, S. M. (2022). A MIP-enabled stainless-steel hypodermic needle sensor for electrochemical detection of aflatoxin B1. Anal. Methods 14, 2063–2071. doi: 10.1039/D1AY02084F
Wu, J., Xie, Z., Li, M., Lin, Y., Tan, X., and Huang, K. (2023). Molecularly imprinted photoelectrochemical sensing supported by Bi2S3/Bi2O2CO3 direct Z-scheme heterojunction for aflatoxin B1 detection. Sensors Actuators B Chem. 378:133143. doi: 10.1016/j.snb.2022.133143
Yadav, N., Yadav, S. S., Chhillar, A. K., and Rana, J. S. (2021). An overview of nanomaterial based biosensors for detection of aflatoxin B1 toxicity in foods. Food Chem. Toxicol. 152:112201. doi: 10.1016/j.fct.2021.112201
Yarynka, D. V., Sergeyeva, T. A., Piletska, E. V., Linnik, R. P., Antonyuk, M. Z., Brovko, O. O., et al. (2021). Validation of aflatoxin B1 MIP membrane-based smartphone sensor system for real sample applications. Biopolym. Cell 37, 346–356. doi: 10.7124/bc.000A60
Ye, L., and Mosbach, K. (2008). Molecular imprinting: synthetic materials as substitutes for biological antibodies and receptors†. Chem. Mater. 20, 859–868. doi: 10.1021/cm703190w
Yu, X., Li, Z., Zhao, M., Lau, S. C. S., Ru Tan, H., Teh, W. J., et al. (2019). Quantification of aflatoxin B1 in vegetable oils using low temperature clean-up followed by immuno-magnetic solid phase extraction. Food Chem. 275, 390–396. doi: 10.1016/j.foodchem.2018.09.132
Zhan, S., Hu, J., Li, Y., Huang, X., and Xiong, Y. (2021). Direct competitive ELISA enhanced by dynamic light scattering for the ultrasensitive detection of aflatoxin B1 in corn samples. Food Chem. 342:128327. doi: 10.1016/j.foodchem.2020.128327
Zhang, K., and Banerjee, K. (2020). A review: sample preparation and chromatographic Technologies for Detection of aflatoxins in foods. Toxins 12:539. doi: 10.3390/toxins12090539
Keywords: aflatoxins, food quality, molecular imprinting, mycotoxins, sensors
Citation: Ali A, Sadiqa A, Ilyas H, Bibi A, Hussain U, Iqbal N, Mujahid A and Afzal A (2023) Ensuring food safety with molecularly imprinted polymers: innovative methods for the detection of aflatoxins in food and feed samples. Front. Sustain. Food Syst. 7:1210104. doi: 10.3389/fsufs.2023.1210104
Edited by:
John Franklin Leslie, Kansas State University, United StatesReviewed by:
Jinfang Nie, Guilin University of Technology, ChinaCopyright © 2023 Ali, Sadiqa, Ilyas, Bibi, Hussain, Iqbal, Mujahid and Afzal. This is an open-access article distributed under the terms of the Creative Commons Attribution License (CC BY). The use, distribution or reproduction in other forums is permitted, provided the original author(s) and the copyright owner(s) are credited and that the original publication in this journal is cited, in accordance with accepted academic practice. No use, distribution or reproduction is permitted which does not comply with these terms.
*Correspondence: Asghar Ali, asgharali@uhb.edu.sa; Adeel Afzal, adeel.chem@pu.edu.pk