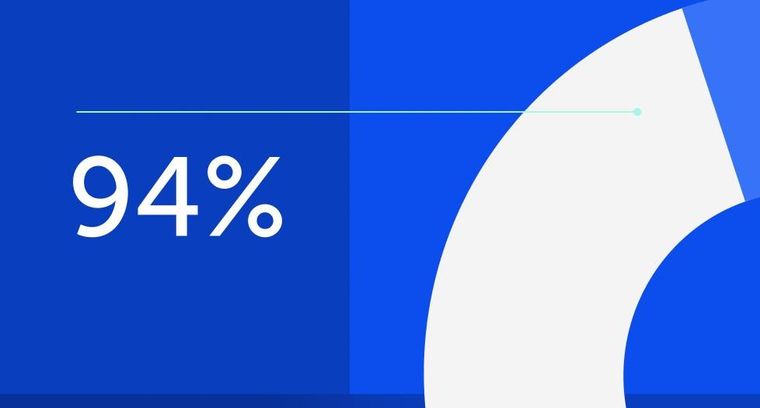
94% of researchers rate our articles as excellent or good
Learn more about the work of our research integrity team to safeguard the quality of each article we publish.
Find out more
ORIGINAL RESEARCH article
Front. Sustain. Food Syst., 20 July 2023
Sec. Sustainable Food Processing
Volume 7 - 2023 | https://doi.org/10.3389/fsufs.2023.1204307
Predicted famines due to population increase created an interest in the development of protein alternatives during the 1950s. Currently, a renewed interest in protein alternatives has developed as a potential strategy to decrease the environmental impact of protein production and meet the global demand for protein as the population increases. Fusarium venenatum A3/5/3, the organism used for mycoprotein production has been commercially available since the 1980s, however new fungal protein companies are currently interested in scaling up production. To aid guide efforts in this domain, we created an economic model with over 340 inputs that examines the continuous production of mycoprotein utilizing airlift bioreactors. Utilizing a sensitivity analysis, we identified critical processing inputs and then developed a user-friendly Excel model that allows for the exploration of customized production scenarios for interested stakeholders. Our findings indicate that mycoprotein can be cost competitive with beef on a price per protein basis. The findings also indicate that mycoprotein may not be an economically competitive alternative for other types of commodity meats (chicken) or for inexpensive meat-derived products (pet food) that utilize offal or meat byproducts not traditionally consumed in the modern western diet.
Modern interest in protein alternatives began in the late 1950’s when it was predicted that a worldwide shortage of high-protein foods would occur in the 1980s (Moore et al., 2021). In response, the Rank Hovis McDougall Research Centre began a project in 1964 to convert waste starch from cereal processing into a high-protein food (Finnigan, 2011). While the predicted protein shortage did not occur due to the Green Revolution, in 1969, the fungal strain Fusarium venenatum A3/5/3 was identified and selected as the organism which would convert glucose and ammonia into a protein-rich biomass called mycoprotein (Moore et al., 2021). After 10 years of safety testing (1970–1980) and a review of a 2 million word, 26-volume food safety report submitted to United Kingdom’s Ministry of Agriculture, Fisheries and Food, the first mycoprotein product was sold to the public in 1985. The product was branded as Quorn® and initially sold as a health food given its low fat (2.9%) and high fiber (5.1%) characteristics (Moore et al., 2021). Quorn® first become available in the United States in 2002 after approval by the United States’ Food and Drug Administration (Moore et al., 2021). Nearly 20 years after US regulatory approval of mycoprotein, a renewed interest in alternative protein sources has emerged, harkening back to the original concerns of the late 1950s and 1960s.
Looking forward, global demand for meat is expected to continually increase as incomes rise and the population increases [United Nations, 2017; Food and Agriculture Organization of the United Nations (FAO), 2019]. The projected increase in meat production has raised concerns about the anticipated environmental impacts, such as increased greenhouse gas (GHG) emissions and land, water, and energy resource consumption [Food and Agriculture Organization of the United Nations (FAO), 2018; Olivier and Peters, 2020]. These environmental concerns, as well as concerns related to animal welfare and human health, have driven interest in meat alternatives which are “food products that have the organoleptic qualities of meat, but whose origin is not from slaughtered animals” (Risner et al., 2020). The sum of these convergent trends has prompted a renewed interest in meat alternatives from scientists, non-profit groups, companies, governments, and investors. In addition to this expected demand for meat or meat-like products, analysts have predicted a substantial (60–70%) displacement of conventional ground beef with meat alternatives over the next ten years 10–20 years (Suhlmann et al., 2019; Tubb and Seba, 2019).
Meat alternatives can be broadly categorized into three groups: plant-based products, animal cell-based meat, and microbial-based products. The total commercial sector of meat alternatives has received $11.1 billion in capital investment since 2010 with 73% of the investment being raised since 2020 (Good Food Institute, 2022). The fermentation category of meat alternatives, including microbial cell proteins such as mycoprotein, received $1.7 billion in investment in 2021 (Good Food Institute, 2022). This level of investment suggests the need for a flexible technoeconomic model that examines the scaling of core production technologies and incorporates different biological factors and limitations, such as the specific growth rate of an organism. Other technoeconomic assessments (TEAs) have examined mycoprotein production from different perspectives including integration into a multi-product biorefinery and the use of agriculture waste streams as a fermentation substrate (Ritchie et al., 2017; Bulkan et al., 2020; Upcraft et al., 2021). Many TEAs utilize process simulation software that can require a significant economic investment and require additional training for effective use (AspenTech, 2022; Itelligen, Inc. 2022). To help overcome some of these challenges, we have developed an open-source excel model that allows the users to examine current industrial practices for mycoprotein production. Additionally, our model allows users to adjust model parameters to conduct scenario analyses that can highlight potential innovations in fungal meat alternatives research or production. The overall goal of the study is to provide a flexible model of industrial mycoprotein production which can be utilized for economic scenario analysis for interested parties.
To understand the economic potential of mycoprotein and a processed Quorn®-like product (PQP), we developed a TEA model utilizing process and chemical engineering methodology. Mycoprotein does not have a meat-like texture without additional processing and the need for this additional processing is product dependent (e.g. pet food vs. chicken nugget-like product). The modeled system is a continuous fermentation system operating at capacity that accounts for the time requirements for the initial growth phase as well as sanitation/cleaning periods. PQP then receives additional processing to achieve meat-like texture development. All variables and equations are available in Appendix A and the excel model has been supplied in the Supplementary material. The annual costs were divided into annualized capital costs and annual operating expenditures.
Mycoprotein is commercially produced utilizing aerobic airlift bioreactors operated in a continuous fashion (Finnigan, 2011; Moore et al., 2021). In addition to the primary bioreactor system, an RNA reduction system is utilized to reduce the RNA content of the mycoprotein, a centrifuge is used to dewater the mycoprotein, and a vacuum chiller is used to quickly reduce the temperature of mycoprotein to storage temperature (See Figure 1).
Commercial airlift bioreactors utilized for mycoprotein production have a reported working volume of 155 m3 and can produce approximately 2 metric tons of consumable mycoprotein per hour (Derbyshire and Ayoob, 2019). All calculations utilize working volume or other equipment specific working parameters in the presented scenarios. These reactors operate in a continuous fashion (for approximately 1,000 h) and a concentration of 10–15 g/L of biomass (wet basis) is maintained in the reactor while it is continuously harvested (Moore et al., 2021). To understand the required fermentation capacity of the system; a mass-balance of the mycoprotein production system was conducted (Equations 1–4, 12–16). This includes accounting for the heat induced RNA reduction (~68°C) that causes a ~ 30% loss of solids from the final mycoprotein product (Moore et al., 2021). This processing step is necessary because it reduces the RNA content of mycoprotein from ~8% (w/w) to ~1% (w/w) which is approximately the RNA content of mammalian liver and within the World Health Organization’s upper limit of 2% (w/w) RNA content for food products (Finnigan, 2011). The concern is in regard to the breakdown of nucleic acid in humans leading to excess uric acid in the bloodstream, which can cause gout and renal stones (Ragab et al., 2017; Moore et al., 2021). After the RNA reduction, mycoprotein water content is reduced to approximately 76–70% (w/w) (Finnigan, 2011; Moore et al., 2021).
Once the mass of harvestable mycoprotein per liter of growth medium was determined, the necessary fermentation capacity was calculated utilizing a user-defined hourly production goal and a reported specific growth rate of F. venenatum ATCC PTA-2684 (Equations 17, 18). The maximum dilution rate of the airlift bioreactor cannot exceed the maximum specific growth rate, otherwise the rate of biomass withdrawal exceeds the rate of biomass production and the cells will be washed out of the system. The necessary fermentation capacity and maximum bioreactor working volume was utilized to determine the number of airlift bioreactors needed to reach the hourly production goal during continuous operation (Equations 19–22). Equipment cost estimates were then applied to the system utilizing a method described in Food Plant Economics (Equations 23–26) (Maroulis and Saravacos, 2007a,c). The Food Plant Economics method for capital costs estimation was utilized throughout the model unless otherwise stated (Maroulis and Saravacos, 2007a, 2007c). Each airlift bioreactor system was outfitted with an individual RNA reduction vessel and a centrifuge. The quantity of vacuum chilling units is determined by model inputs.
The RNA reduction vessel heats the mycoprotein in suspension for 15–30 min (68°C). The RNA reduction vessels working volume was determined utilizing the airlift bioreactor’s working volume and an RNA reduction factor (Equation 28). The RNA reduction factor was estimated at ~10% of the airlift bioreactor working volume based upon the specific growth/withdrawal rate during the continuous phase of mycoprotein production and the hold time in the reactor. However, this factor is adjustable in the model to accommodate variable processing scenarios. The centrifuge processing rates were determined utilizing Equations 29, 30. Once the processing rate was determined, the capital expenditures were estimated utilizing methods described in Food Plant Economics (Maroulis and Saravacos, 2007a,c).
After centrifugation, the mycoprotein is chilled (~0°C) utilizing a vacuum cooling unit before being shipped to a facility that would further process it into consumer products. Vacuum chilling is considered to have high capital cost but is an economically viable cooling process given its ability to rapidly cool products and its low manpower requirements. Equations 31, 32 provide the capital cost estimation method for the vacuum cooling unit which utilizes a USD/kg-day costing unit and accounts for inflation, Lang factor, and material composition costs. The capital costs related to onsite storage of mycoprotein are not accounted for and the mycoprotein is transported to a PQP production facility at no cost in our limited model.
After being dewatered and cooled, mycoprotein can be further processed to develop a fibrous, meat-like texture. A series of processing steps are utilized for the development of the final PQP (Figure 2). The capital expenditures for each processing step were estimated utilizing the Food Plant Economics methodology and other literature sources as needed (Maroulis and Saravacos, 2007a,c).
The mycoprotein is considered to have an appearance and texture similar to bread dough but lacks its elasticity (Finnigan, 2011). Mixing tanks associated with breadmaking were utilized to estimate the capital expenditures for the mixing process. We utilized mixing tanks to estimate the unit cost (75,000 USD) and a base equipment sizing unit of 1,000 kg per hour (kg/h) was utilized.
After the mixing of mycoprotein and other PQP minor ingredients, the mass is discharged into common food processing equipment which utilizes pressure to shape the PQP into blocks (Finnigan, 2011). Bread forming equipment was utilized to estimate the unit costs (60,000 USD) at a base equipment sizing unit of 3,000 kg/h (Maroulis and Saravacos, 2007a).
Once formed into blocks, steam is utilized to raise the block’s internal temperature to 90°C. The steam cooking could be achieved using a variety of systems; however, a steam blanching/cooking system was utilized to estimate the unit costs (200,000 USD) at a base equipment sizing unit of 5,000 kg/h (Maroulis and Saravacos, 2007a).
The initial freezing occurs over 30 min and reduces the temperature to −10°C. Freezing can be achieved utilizing a variety of freezing technologies, however we utilized a belt freezer to estimate the unit costs (250,000 USD) at base equipment sizing unit of 2,000 kg/h (Maroulis and Saravacos, 2007a).
A cutter or grinder can be utilized depending upon the final desired PQP geometry. We utilized an estimate for a generic size reduction unit with an estimated unit costs ($10,000 USD) at a base equipment sizing unit of 1 kg/s (Maroulis and Saravacos, 2007a).
The freezer volume was determined utilizing a required storage time and the hourly production rate (Equations 34–36). Capital expenditures were then estimated utilizing estimates from the FAO, accounting for inflation (Equation 37) (Johnston et al., 1994).
Once the individual capital expenditures were determined, the total capital cost for each production process was calculated utilizing Equations 33, 38. Final reported PQP costs include mycoprotein capital costs. It should be noted that only items in the model are included in the capital expenses (see Supplementary material for adjustable model).
Manufacturing costs for mycoprotein and PQP can be broken into three categories: fixed manufacturing, variable capital costs, and indirect (overhead) costs. Fixed manufacturing costs are estimated as a percentage of the annual capital expenditure payment except loan and equity interest, which is accounted for separately. These fixed manufacturing cost include equipment maintenance, insurance, taxes, and royalty costs (Maroulis and Saravacos, 2007d). Indirect costs are unrelated to amount of product processed, such as sales expenses and local taxes, and are not accounted for in our model since these expenditures are outside of processing facility expenses and vary firm to firm. Our model estimates several variable capital expenditures for mycoprotein and PQP production, however, the model should be considered a limited model. Costs associated with general food production such as lighting, pumping, conveyor belts, packaging or transport are not included in the model. In this technoeconomic model, additional ingredients can be added to PQP production but in the current scenarios no additional ingredients have been considered. The estimated variable costs include growth medium components, other raw materials, some utilities (some energy, process water and wastewater processing) and labor costs.
Growth medium usage was accounted for during the growth phase and continuous production phase (Equations 39–42). The exact growth medium composition for commercial mycoprotein production was not available to the authors. To estimate the minimum glucose required, we utilize a reported protein content of mycoprotein and a reported glucose-to-protein conversion rate to determine annual cost for minimum required glucose (38 g/L) (Equations 43–47) (Moore et al., 2021). It should be noted that the initial goal of direct conversion of starch to biomass was found to be a rate limiting step during process development, so a highly refined glucose syrup is utilized as the carbon source (Whittaker et al., 2020). Annual expenses related to oxygen production were estimated utilizing reported industrial values and fungal oxygen consumption rates (Equations 48–50) (Humbird et al., 2017; Rossi et al., 2017). Ammonia usage was estimated via the protein content. Once the annual protein production was determined, a protein estimation factor taken from the Kjeldahl method (6.25) was used to convert the known protein mass to the estimated nitrogen mass (Mæhre et al., 2018). Once converted to nitrogen, another factor utilizing the molecular weights of nitrogen and ammonia was used to estimate the minimum mass of ammonia needed for annual production (Equations 51–53). These calculations were used to estimate the minimum cost of glucose and ammonia.
It has been reported that other minor growth medium components are utilized for mycoprotein production, however the composition of these minor ingredients has not been publicly reported (Harrison and Johnson, 2018). Vogel’s growth medium has been utilized in literature and was utilized to identify/quantify potential growth medium components (Wiebe et al., 1994; Hossenini et al., 2009; Fungal Genetic Stock Center, 2020). The prices for laboratory grade growth medium components were obtained from a scientific supply site (Merck KGaA, 2022). The total annual costs of other growth medium components were then determined utilizing Equation 54. One important note on the model is that growth medium components are supplied in excess during commercial operation to maintain a maximum specific growth rate and prevent mycotoxin formation (Moore et al., 2021). Additional ingredients can be added to the excel model for additional scenario development (see Supplementary material).
Energy costs were estimated utilizing a method which accounts for self-produced energy and energy sourced from a public supplier (Equations 55–57) (Maroulis and Saravacos, 2007b; Risner et al., 2020). Energy estimates for mycoprotein production include sterilization and cooling of the growth medium before the entering airlift bioreactor (Equations 58, 59), heating of the growth medium during RNA reduction (Equation 60), cooling of the mycoprotein via vacuum chilling (Equation 61), and compressed air energy estimates (Equation 62) (U.S. Department of energy, 2004). Equations 63, 64 were then utilized to estimate minimum energy costs in the mycoprotein production facility.
Minimum energy requirements for PQP production were estimated using several methods. The steam cooking and chilling of PQP was estimated using standard thermodynamic calculations (Equations 65, 66). The energy requirement for the size reduction equipment was estimated utilizing the semiempirical Bond law (Equations 67, 68) (Maroulis and Saravacos, 2007a) To obtain the proper meat-like texture, PQP is freezer-aged for approximately 2 weeks (Moore et al., 2021). To account for annual freezer energy, the freezer storage was estimated, and annual energy usage determined via Specific Electricity Consumption (SEC) number estimate (Equation 69) (Prakash and Singh, 2008). The PQP processing plant compressed air was estimated via a compressed air factor (Equation 70). The minimum energy usage and costs for a PQP were estimated utilizing Equations 71, 72.
The minimum process water utilized was the volume of growth medium utilized in a year. This does not include water used for sanitation or cleaning. The wastewater filtration and biological oxidation treatment volumes were determined utilizing volume/mass of media removed per kilogram of mycoprotein. The costs of the process water and wastewater treatment were obtained from literature (Maroulis and Saravacos, 2007b; Risner et al., 2020). Water usage for PQP is not accounted for and the required process and wastewater costs should be viewed as minimum costs.
Our model assumes that the production facility operates 24 h/day all year. We assume that the facilities are fully staffed, each shift is an 8-h shift and there is no overtime required. The facilities are assumed to be in a generic, standard income portion of the United States. The required manpower for each shift is estimated utilizing a standard method which assigns a manpower requirement by the amount and type of processing equipment in the facility (Equation 74) (Maroulis and Saravacos, 2007b). The labor costs for each facility were determined by using a mean hourly rate of 20 USD/h and a labor cost correction factor (Equations 74–76). This allowed for the estimation of total labor costs at each facility.
The minimum annual operating expenses for the mycoprotein and PQP production facilities were estimated using Equations 77–78. We utilize standard financing equations with a 20-year economic life to account for expenses related to equity recovery and debt (5% was utilized in our scenario analysis) for both the mycoprotein and PQP production facilities (Equations 79–88) (California Biomass Collaborative, 2016). These equations annualize the capital expenditures and allow for a total minimum annual cost to be determined for each production facility. After annualization of the capital expenditures, fixed annual operating costs were accounted for as a percentage (3%) of the annualized capital expenditures (Maroulis and Saravacos, 2007c).
We performed a sensitivity analysis of the mycoprotein and PQP production cost model using a standard one-factor-at-a-time (OAT) approach (Saltelli et al., 2008). We individually changed each input by ±25% and recorded its impact on the model’s output variables. We then converted the input back to the original value and repeated this for each input variable. This allowed for identification of impactful input variables which allowed for a streamlining of the model user interface and helped to inform our scenario design. Results for the sensitivity analysis can be found in Appendix B.
We identified >340 input variables which influence the capital and/or operating expenses for our limited model system. The capital costs in our base scenario (2000 kg/h) for mycoprotein was ~108 million USD. The main airlift bioreactor accounted for 66.7% of the capital costs. The reported maximum working volume (155 m3) of the airlift bioreactor was utilized in each scenario, however this volume is adjustable in the model (Moore et al., 2021). The capital costs of a single 155 m3 airlift bioreactor constructed with 304 stainless steel was estimated to be ~42 million USD and an additional bioreactor used to meet the production goal was estimated to be ~29 million USD. The estimated capital costs of the RNA reduction vessels, centrifuges and vacuum chillers is 18, 13, and 4 million USD, respectively (Figure 3).
Total capital expenses (does not include equipment utilized for mycoprotein production) for PQP were an order of magnitude lower than for mycoprotein production, estimated to be ~13.8 million USD. The PQP chiller was responsible for over 44% of the PQP capital costs. Figure 4 indicates estimated capital costs for the processing equipment utilized for PQP production. The size reduction equipment was by far the least impactful estimated capital costs. It should also be noted that the land purchase, working capital and start-up/validation are not accounted for in any capital expenditure, and this has the potential to increase total capital expenditures.
The annual operating expenses included debt/financing, growth medium, oxygen, energy, process water, wastewater treatment and labor expenses. An OAT sensitivity analysis was conducted to identify model inputs which were most impactful to the cost of mycoprotein production (Appendix B). The results of the sensitivity analysis were then utilized to develop a more limited interface with the inputs categorized into four broad input categories (general bioreactor operations, organism characteristics, continuous airlift bioreactor parameters, and growth medium characteristics). The sensitivity analysis informed the specification of three alternative scenarios relative to a baseline scenario. Scenario 1 is the baseline scenario and can be viewed in the excel model in the Supplementary material. All scenarios maintained the baseline settings except where noted. Scenario 2 doubled the capital costs of the mycoprotein production equipment before financing. Scenario 3 decreased the costs of biotin and zinc sulfate heptahydrate to mass produced, food-grade prices of $0.373/g and $0.0018/g, respectively. Scenario 4 doubled the required glucose amount due to glucose being maintained in excess to maximize the specific growth rate during commercial production (Finnigan, 2011; Moore et al., 2021). A reported production rate of 2,000 kg mycoprotein/h (moisture content of ~73%) was chosen for each scenario; however, this rate is user-defined within the model (Moore et al., 2021). Results of our baseline model estimate that mycoprotein can be produced for $3.55/kg and PQP can be produced for $4.03/kg. The protein content of mycoprotein has been reported as approximately 11–12% (w/w) which indicates protein production costs are approximately $29.56/kg (Derbyshire and Delange, 2021; Moore et al., 2021). Figure 5 provides a cost comparison across the scenarios. Protein production costs were highest in Scenario 4 where the glucose concentration was doubled. Decreasing the cost of biotin and zinc sulfate heptahydrate, key minor component cost drivers in scenario 3 reduced protein costs by 22%.
USDA reported costs of choice beef was $6.56/kg as net farm value in March 2022, whereas our base model for mycoprotein production is $3.55/kg and $4.03/kg for PQP (United States Department of Agriculture, 2022). These values initially seem economically favorable for mycoprotein but when examined on a protein basis the difference is less significant with mycoprotein being $29.56/kg of protein and beef being $29.95/kg of protein (Derbyshire and Delange, 2021; United States Department of Agriculture, 2022). It can also be noted that in March 2021, the cost of choice beef was reported to be $5.53/kg or $25.25/kg of protein. Broiler chickens were reported by the USDA to be $1.95/kg at wholesale (United States Department of Agriculture, 2022). Approximately 71% (excluding skin and bones) of a chicken carcass is usable meat (Orr et al., 1984) which would give an approximate cost of $2.74/kg. Chicken breast has been reported have 28.4 g of protein per 100 grams, leading to an estimated of protein sourced from chicken of approximately $9.64/kg (Derbyshire and Delange, 2021). Our model indicates that mycoprotein may be produced as an economic alternative to beef protein but will not be an economic alternative for inexpensive products such as chicken or offal utilized in pet food production.
Our technoeconomic model found that mycoprotein protein production utilizing a continuous production system was economically comparable to farm-raised beef protein production. However, if compared on a calorie basis, one kilogram of stewed beef mince has 2,090 kcal vs. one kilogram of mycoprotein which has 850 kcal. This difference in caloric density indicates that ~2.5 times more mycoprotein would need to be consumed to achieve the same caloric intake as stewed beef; and subsequently, stewed beef would also represent a significantly less expensive option in terms of available calories (Derbyshire and Delange, 2021). Chicken breast meat is reported to contain 1,600 kcal/kg and chicken appears to be the more economical choice as a protein source when compared to mycoprotein or beef. If only examined from a nutrient density viewpoint, an edible insect like the mopane caterpillar (Imbrasia belina) which is reported to contain 4,090 kcal/kg and 352 g of protein/kg may be of interest to food production stakeholders (Payne et al., 2016). An additional consideration would be protein digestibility which mycoprotein has been reported to have higher rating (99.6%) for human consumption when compared with beef or chicken (92 and 95.2%, respectively) (Edwards and Cummings, 2010; Hughes et al., 2011; Boye et al., 2012). While outside the scope of this TEA, techno-economic modeling of industrialized insect protein production would be necessary for a direct comparison of an insect protein source and mycoprotein.
The mycoprotein production system we modeled was a production system that operates continuously for ~1,000 h. The use of continuous airlift bioreactors allows for a five-fold increase in productivity when compared to a series of separate batch fermentations (Finnigan, 2011). It should be noted that batch fermentations are a norm in the commodity food/beverage fermentation industry (wine, beer, cheese), however cell biomass production is generally not the goal. Commercial yeast (Saccharomyces cerevisiae) biomass production mostly utilizes batch production; however, it is an important minor ingredient in baking, brewing, winemaking, etc. … not as a meat replacement. This difference in end use is illustrated by a difference in the global markets for meat and commercial yeast, ~USD 2.3 trillion and ~ USD 7 billion, respectively (Thomas, 2021; Wood, 2021). This indicates that a fungal protein meat replacement, such as mycoprotein needs to be produced in the most efficient manner possible. The results of our model indicate that mycoprotein protein production is approximately equivalent to beef protein in economic terms, but only when the 5-fold productivity benefit of continuous production is achieved.
Continuous airlift bioreactor technology is not a new technology. The development of the world’s largest aerobic fermenter (1,500 m3) occurred in the 1970’s. Operation began in 1979 and the bioreactor was decommissioned in 1987 due to economic and technical challenges (Humbird, 2020). This bioreactor was developed to produce an animal feed soy protein replacement, Pruteen from Methylophilus methylotrophus, a methane utilizing bacteria (Vasey and Powellf, 1984). Technical issues related to foaming and sterility required a systems control redesign that, when coupled with other economic issues, caused Pruteen to be sold at double the price of the soy protein it intended to replace in 1983 (Humbird, 2020). These factors led to the Pruteen plant decommissioning. However, the same technology was then utilized to scale up Quorn® production in the 1990s, albeit at an order of magnitude in reduced scale (155m3) as compared to the Pruteen production fermenter (Moore et al., 2021). While our technoeconomic model is adjustable for the scale-up of the continuous, airlift bioreactor fermentation system, it is likely that any order of magnitude increase in the scale of this system would likely require supplementation or innovation of the core technology.
Reduction in growth medium costs is an evident area where operating expenses can be reduced. It has been reported that near laboratory-grade minor ingredients are utilized for mycoprotein production (Harrison and Johnson, 2018). Scenario 3 was designed to examine the cost impacts of utilizing research grade minor ingredients (biotin and zinc sulfate heptahydrate) versus food-grade/lower purity ingredients. Biotin and zinc sulfate heptahydrate were adjusted to food-grade costs since they were most economically impactful minor ingredients in our model. The total cost reduction in scenario 3 was 22%, however, the feasibility of reducing the purity of the ingredients is not clear. A reduction in the purity could increase operating costs due to more stringent media sterilization protocols and/or require additional media optimization to maintain fungal growth rates (Blackwell, 2017). This indicates that it would be prudent to explore risks associated with utilizing lower purity ingredients relative to the potential cost savings. It should also be noted the original intent of the Rank Hovis McDougal Research Centre was to convert the waste starch product into a protein-rich food, however this determined to be not feasible due to technical/economic limitations related to starch uptake and conversion (Whittaker et al., 2020). Other recent mycoprotein have focused on a single feedstock from agriculture/food production co-products or waste streams which is highly valuable but provides less flexibility than our model (Ritchie et al., 2017; Bulkan et al., 2020; Upcraft et al., 2021). Our model TEA developed a flexible and open access model with the ability to change feedstock composition as innovation occurs in the alternative protein space.
Our techno-economic model indicated that there were several organism/product specific attributes that were impactful to capital and operating costs (Figures 6, 7). The amount of solids in a kilogram of mycoprotein influences the total required fermentation capacity, which in turn, is an input into multiple capital expense calculations, annual growth media usage, and oxygen use calculations. This influence derives from our detailed mass balance calculations (See Appendix A or the Supplementary material). Meanwhile, the protein content of the final mycoprotein product influences the determination of the minimum glucose/ammonia requirements as well as the thermodynamic calculations as the proportion of protein affects the specific heat of mycoprotein. Other expected organism related characteristics, such as specific growth rate and protein yield (g protein/g glucose), influenced the operating expenditures. These identified input variables can be utilized to guide research questions related to increasing protein production or identifying other viable organisms for use in a continuous airlift bioreactor system.
Our technoeconomic model indicates that mycoprotein and PQP can currently economically compete with beef when examined on a protein basis. However, this is for general choice cuts of beef and does not necessarily include cheaper ground products or green/red offal that is often used for pet food production or sold internationally. Our technoeconomic model highlights the importance of utilizing a continuous fermentation system (as opposed to a batch system) to achieve cost parity with beef protein. Potential reductions in cost can be achieved through advances in organism-specific parameters, such as protein content, achievable concentration (g/L), and specific growth rate. The customizable technoeconomic model we have provided can be utilized to explore multiple scenarios beyond those provided in this paper, including custom combinations of minor growth medium components, multiple combinations of materials used for bioreactor construction, different specific organism parameters, and many other scenarios given the full menu of >340 input variables (See Supplemental material).
The original contributions presented in the study are included in the article/Supplementary material, further inquiries can be directed to the corresponding author.
DR, CJ, and ES: conceptualization. DR: data curation, formal analysis, investigation, visualization, and writing-original draft. ES and DR: funding acquisition. DR and ES: methodology. ES and CJ: project administration, resources, and supervision. DR, KM, and ES: validation, and writing-review and editing. All authors contributed to the article and approved the submitted version.
The authors would like to thank the Mars Advanced Research Institute for funding the research.
The authors declare that the research was conducted in the absence of any commercial or financial relationships that could be construed as a potential conflict of interest.
The reviewer TB declared a past collaboration with the author KM to the handling editor.
All claims expressed in this article are solely those of the authors and do not necessarily represent those of their affiliated organizations, or those of the publisher, the editors and the reviewers. Any product that may be evaluated in this article, or claim that may be made by its manufacturer, is not guaranteed or endorsed by the publisher.
The Supplementary material for this article can be found online at: https://www.frontiersin.org/articles/10.3389/fsufs.2023.1204307/full#supplementary-material
AspenTech (2022). Aspen plus | leading process simulation software | AspenTech [WWW document]. Available at: (https://www.aspentech.com/en/products/engineering/aspen-plus).
Blackwell, J. (2017). Troubleshooting bacterial contamination in bioreactors [WWW document]. Bioprocess online. Available at: https://www.bioprocessonline.com/doc/troubleshooting-bacterial-contamination-in-bioreactors-0001 (accessed April 26, 2022).
Boye, J., Wijesinha-Bettoni, R., and Burlingame, B. (2012). Protein quality evaluation twenty years after the introduction of the protein digestibility corrected amino acid score method. Br. J. Nutr. 108, S183–S211. doi: 10.1017/S0007114512002309
Bulkan, G., Ferreira, J. A., Rajendran, K., and Taherzadeh, M. J. (2020). Techno-economic analysis of bioethanol plant by-product valorization: exploring market opportunities with protein-rich fungal biomass production. Fermentation 6:99. doi: 10.3390/fermentation6040099
California Biomass Collaborative (2016). Generic biomass power plant model [WWW document]. Energy cost calculator. Available at: https://biomass.ucdavis.edu/tools/energy-cost-calculator/ (accessed May 14, 2020).
Derbyshire, E., and Ayoob, K. T. (2019). Mycoprotein: nutritional and health properties. Nutr. Today 54, 7–15. doi: 10.1097/NT.0000000000000316
Derbyshire, E. J., and Delange, J. (2021). Fungal protein–what is it and what is the health evidence? A systematic review focusing on mycoprotein. Front. Sustain. Food Syst. :5:581682. doi: 10.3389/fsufs.2021.581682
Edwards, D. G., and Cummings, J. H. (2010). The protein quality of mycoprotein. Proc. Nutr. Soc. 69:E331. doi: 10.1017/S0029665110001400
Finnigan, T. J. A. (2011). “Mycoprotein: Origins, production and properties” in Handbook of Food Proteins. eds. G. O. Phillips and P. A. Williams (Chichester: John Wiley & Sons), 335–352.
Food and Agriculture Organization of the United Nations (FAO) , (2018). Global livestock environmental assessment model (GLEAM) 2.0. Rome. Woodhead, Sawston
Food and Agriculture Organization of the United Nations (FAO) , (2019). Animal Production and Health: Meat & Meat Products. Food and Agriculture Organization of the United Nations (FAO), Rome
Fungal Genetic Stock Center (2020). Vogels [WWW Document]. Available at: http://www.fgsc.net/methods/vogels.html (accessed July 1, 2021).
Good Food Institute (2022). Record $5 Billion Invested in ALT Proteins in 2021 [WWW document]. Available at: (https://gfi.org/press/record-5-billion-invested-in-alt-proteins-in-2021/).
Harrison, R., and Johnson, R. (2018). Mycoprotein production and food sustainability, London: Microbiology Today Magazine.
Hossenini, S., Khosravi, K., Mohammadifar, M., and Nikoopour, H. (2009). Production of mycoprotein by fusarium venenatum growth on modified Vogel medium. Asian J. Chem. 21, 4017–4022.
Hughes, G. J., Ryan, D. J., Mukherjea, R., and Schasteen, C. S. (2011). Protein digestibility-corrected amino acid scores (PDCAAS) for soy protein isolates and concentrate: criteria for evaluation. J. Agric. Food Chem. 59, 12707–12712. doi: 10.1021/jf203220v
Humbird, D. , (2020). Scale-Up Economics for Cultured Meat: Techno-Economic Analysis and Due Diligence. John Wiley & Sons. Hoboken, NJ.
Humbird, D., Davis, R., and McMillan, J. D. (2017). Aeration costs in stirred-tank and bubble column bioreactors. Biochem. Eng. J. 127, 161–166. doi: 10.1016/j.bej.2017.08.006
Itelligen, Inc. (2022). SuperPro Designer [WWW Document]. Available at: https://www.intelligen.com/ (accessed January, 28 2022).
Johnston, W. A., Nicholson, F. J., Roger, A., and Stroud, G. D. (1994). “Finance of freezing and cold storage” in Freezing and Refrigerated Storage in Fisheries. ed. W. A. Johnston (Rome: FAO)
Mæhre, H. K., Dalheim, L., Edvinsen, G. K., Elvevoll, E. O., and Jensen, I. J. (2018). Protein determination—method matters. Foods 7:5. doi: 10.3390/foods7010005
Maroulis, Z. B., and Saravacos, G. (2007a). “Capital costs of food plants” in Food Plant Economics (Boca Raton, FL: CRC Press), 83–133.
Maroulis, Z. B., and Saravacos, G. (2007b). “Operating cost of food plants” in Food plant economics (Boca Raton, FL: CRC Press), 135–174.
Maroulis, Z. B., and Saravacos, G. D. (2007d). “Process engineering economics” in Food Plant Economics (Boca Raton, FL: CRC Press), 65–100.
Merck KGaA (2022). MilliporeSigma | United States [WWW document]. Available at: https://www.sigmaaldrich.com/US/en (accessed April 12, 2022).
Moore, D., Robson, G., and Trinci, A. (2021). “The Quorn fermentation and evolution in fermenters” in 21st century guidebook to Fungi (Cambridge: Cambridge University Press).
Olivier, J. G. J., and Peters, J. A. H. W., (2020). Trends in Global CO2 and Total Greenhouse Gas Emissions. Netherland Environmental Assessment Agency. The Hague.
Orr, H. L., Hunt, E. C., and Randall, C. J. (1984). Yield of carcass, parts, meat, skin, and bone of eight strains of broilers. Pultry Sci. 63, 2197–2200. doi: 10.3382/ps.0632197
Payne, C. L. R., Scarborough, P., Rayner, M., and Nonaka, K. (2016). Are edible insects more or less ‘healthy’ than commonly consumed meats? A comparison using two nutrient profiling models developed to combat over- and undernutrition. Eur. J. Clin. Nutr. 70, 285–291. doi: 10.1038/ejcn.2015.149
Prakash, B., and Singh, R. P. (2008). Energy benchmarking of warehouses for frozen foods. Food Manuf. Effic. 1, 9–18. doi: 10.1616/1750-2683.0017
Ragab, G., Elshahaly, M., and Bardin, T. (2017). Gout: an old disease in new perspective – a review. J. Adv. Res. 8, 495–511. doi: 10.1016/j.jare.2017.04.008
Risner, D., Li, F., Fell, J. S., Pace, S. A., Siegel, J. B., Tagkopoulos, I., et al. (2020). Preliminary techno-economic assessment of animal cell-based meat. Foods 10:3. doi: 10.3390/foods10010003
Ritchie, H., Laird, J., and Ritchie, D. (2017). 3f bio: halving the cost of mycoprotein through integrated fermentation processes. Ind. Biotechnol. 13, 29–31. doi: 10.1089/ind.2017.29065.hri
Rossi, M. J., Nascimento, F. X., Giachini, A. J., Oliveira, V. L., and Furigo, A. (2017). Transfer and consumption of oxygen during the cultivation of the ectomycorrhizal fungus Rhizopogon nigrescens in an airlift bioreactor. Appl. Microbiol. Biotechnol. 101, 1013–1024. doi: 10.1007/s00253-016-7854-4
Saltelli, A., Ratto, M., Andres, T., Campolongo, F., Carboni, J., Gatelli, D., et al., (2008). Global Sensitivity Analysis. The Primer, 1st ed John Wiley & Sons, Chichester.
Suhlmann, G., Ziemben, F., Donnan, D., Gerhardt, C., and Warschun, M., (2019). When consumers go vegan, how much meat will be left on the table for agribusiness? Kearney, Kearney.
Thomas, S. , (2021). Global yeast market value is climbing by 6% CAGR from 2021-2026 to approach USD 11 billion [WWW document]. Fact and Factors. Available at: https://www.globenewswire.com/news-release/2021/12/27/2358090/0/en/Global-Yeast-Market-value-is-climbing-by-6-CAGR-from-2021-2026-to-approach-USD-11-Billion-With-COVID-19-Impact-Analysis-Facts-Factors.html (accessed April 26, 22).
Tubb, C., and Seba, T. (2019). Rethinking food and agriculture 2020-2030: the second domestication of plants and animals, the disruption of the cow, and the collapse of industrial livestock farming. Ind. Biotechnol. 17, 57–72. doi: 10.1089/ind.2021.29240.ctu
U.S. Department of energy , (2004). Energy tips-compressed air. U.S. Department of Energy, Washington DC.
United Nations , (2017). World population projected to reach 9.8 billion in 2050, and 11.2 billion in 2100 [WWW document]. The world population prospects: The 2017 revision. Available at: https://www.un.org/en/desa/world-population-projected-reach-98-billion-2050-and-112-billion-2100 (accessed April 27, 2022).
United States Department of Agriculture , (2022). USDA ERS-Meat Price spreads. Washington DC United States Department of Agriculture.
Upcraft, T., Tu, W. C., Johnson, R., Finnigan, T., Van Hung, N., Hallett, J., et al. (2021). Protein from renewable resources: mycoprotein production from agricultural residues. Green Chem. 23, 5150–5165. doi: 10.1039/D1GC01021B
Vasey, R. B., and Powellf, K. A. (1984). Single-cell protein. Biotechnol. Genet. Eng. Rev. 2, 285–311. doi: 10.1080/02648725.1984.10647802
Whittaker, J. A., Johnson, R. I., Finnigan, T. J. A., Avery, S. V., and Dyer, P. S. (2020). “The biotechnology of Quorn mycoprotein: past, present and future challenges” in Grand challenges in biology and biotechnology. ed. P. H. Rampelotto (Berlin: Springer Science and Business Media B.V.), 59–79.
Wiebe, M. G., Robson, G. D., Oliver, S. G., and Trinci, A. P. J. (1994). Use of a series of chemostat cultures to isolate “improved” variants of the Quorn® myco-protein fungus, fusarium graminearum A3/5. Microbiology (N Y) 140, 3015–3021. doi: 10.1099/13500872-140-11-3015
Wood, L. , (2021). Global meat markets, 2021–2025 by product (chicken, beef, mutton, pork and others), & type (raw and processed) [WWW document]. Businesswire. Available at: https://www.businesswire.com/news/home/20210920005477/en/Global-Meat-Markets-2021-2025-by-Product-Chicken-Beef-Mutton-Pork-and-Others-Type-Raw-and-Processed---ResearchAndMarkets.com (accessed April 26, 2022).
Keywords: mycoprotein, techno-economic, model, bioreactor, airlift, continuous, protein
Citation: Risner D, McDonald KA, Jones C and Spang ES (2023) A techno-economic model of mycoprotein production: achieving price parity with beef protein. Front. Sustain. Food Syst. 7:1204307. doi: 10.3389/fsufs.2023.1204307
Received: 14 April 2023; Accepted: 13 June 2023;
Published: 20 July 2023.
Edited by:
Samuel Ayofemi Olalekan Adeyeye, Hindustan University, IndiaReviewed by:
Tyler John Barzee, University of Kentucky, United StatesCopyright © 2023 Risner, McDonald, Jones and Spang. This is an open-access article distributed under the terms of the Creative Commons Attribution License (CC BY). The use, distribution or reproduction in other forums is permitted, provided the original author(s) and the copyright owner(s) are credited and that the original publication in this journal is cited, in accordance with accepted academic practice. No use, distribution or reproduction is permitted which does not comply with these terms.
*Correspondence: Edward S. Spang, ZXNzcGFuZ0B1Y2RhdmlzLmVkdQ==
Disclaimer: All claims expressed in this article are solely those of the authors and do not necessarily represent those of their affiliated organizations, or those of the publisher, the editors and the reviewers. Any product that may be evaluated in this article or claim that may be made by its manufacturer is not guaranteed or endorsed by the publisher.
Research integrity at Frontiers
Learn more about the work of our research integrity team to safeguard the quality of each article we publish.