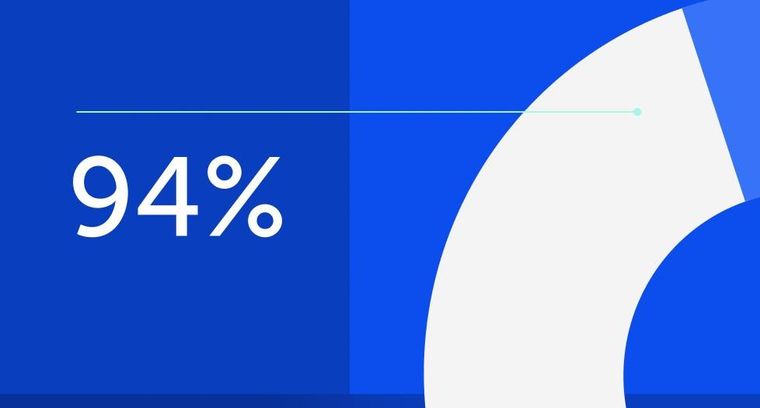
94% of researchers rate our articles as excellent or good
Learn more about the work of our research integrity team to safeguard the quality of each article we publish.
Find out more
REVIEW article
Front. Sustain. Food Syst., 13 September 2023
Sec. Climate-Smart Food Systems
Volume 7 - 2023 | https://doi.org/10.3389/fsufs.2023.1188467
The feed-food competition for environmental and economic resources raises increasing concerns about the production and supply of protein for the global livestock sector. Risks to food-security and approaching deadlines for global sustainable development, means exploring alternative protein feed ingredients is imperative. This Review discusses the potential for soilless, local and circular protein feed sources to provide solutions for key sustainability and food-security threats to the global livestock sector, through their partial incorporation in future livestock feeds and feeding systems. In doing so, it offers a holistic insight into the potential opportunities, but also risks associated with such alternatives. Through this analysis, a four-point strategic plan is synthesized to facilitate higher-level policy making that may enable implementation of these alternative ingredients at commercial scales, building toward a more sustainable and resilient livestock industry.
Animal-sourced foods, including livestock products are generally considered protein-rich and essential for human nutrition due to their enhanced bioavailability of many beneficial nutrients and superior protein quality (Leroy et al., 2022). Sustainable livestock systems are essential to human and planetary existence, helping secure full human growth especially in areas where fortification and/or supplementation is not feasible, as well as ensuring management of lands and conservation of agricultural biodiversity (Robinson et al., 2011).
However, significant concerns have been raised regarding the efficiency of resource use and environmental impacts of conventional livestock production systems, particularly considering the feed-food competition for land and energy resources (Ertl et al., 2016). For this reason, the future of livestock feeds is of major concern to all stakeholders of the agri-food system, including policy makers, industry, regulatory authorities, and consumers (Makkar, 2018; Gurgel et al., 2021). The conversations around the feed-food competition for resources have become particularly relevant in recent years, considering planet limited biophysical capacity and uncertainties associated with macroeconomic, geopolitical, and socioeconomic developments that threaten feed availability and food-security; such uncertainties include the on-going Ukraine-Russia conflict, Brexit, disparity in household incomes experienced globally, inflation, and energy shortages (van Hal et al., 2019). Emphasis has been given on soy production, which constitutes one of the major sources of protein for livestock. Governmental authorities, non-governmental organizations and policy makers globally acknowledge the potentially damaging effects of soy production on endangered ecosystems (e.g., the Cerrado) and local rural communities (Cabezas et al., 2019; Kusumaningtyas and van Gelder, 2019). Such impacts may become relevant for other conventional protein crops too that are widely used as livestock feed protein sources and take up to 15% of the animal diet compositions, such as oilseed crops (e.g., sunflower seed, rapeseed), particularly considering the global demand for sustainable intensification of livestock production (Ben Hassen and El Bilali, 2022). Furthermore, increases in adoption of plant-based diets by humans is expected to intensify land-related feed-food competition and unintended negative sustainability impacts associated with protein crops even in countries with established boundaries for arable land (e.g., USA) (de Visser et al., 2014; US Soybean Export Council, 2018).
In this Review, we call for a focused discussion regarding the diversification of protein sources in livestock feeds as imperative to reducing the production of unsustainable production of protein crops. We propose the exploration of a partial substitution of soy in feeds by soilless, local and circular alternatives, that could cover livestock nutritional needs while addressing key sustainability issues and securing food safety. To facilitate future discussions regarding their implementation and because it is not always possible to discuss in too much detail the potential differences between variations of alternatives (e.g., comparing between the effectiveness of different microalgae species), we classify the alternatives in a systematic way that aligns with literature and represents the four protein production systems that have been investigated in most depth:
Alternative plant-based sources and crop growing methods: representing genetically modified/edited crops (GM/GE), local/home-grown crops (e.g., legumes), hydroponics, and seaweed farming:
i. Cellular agriculture: representing protein extraction at a microscopic level from bacterial, fungal and micro-algal organisms.
ii. Circular agriculture: representing protein sourcing from processed food wastes (e.g., restaurants, hotels, retailers), former foods (e.g., bakery and confectionery) and by-products of other industries (e.g., brewing, biofuel).
iii. Animal by-products: representing the sourcing of Processed Animal Proteins (PAPs) from swine, poultry and ruminant by-products or from insect farming.
This Review provides a lens into the opportunities that such alternatives present to relieve pressures of the feed-food competition across the three sustainability pillars, as defined in the global sustainable development goals of the United Nations (UN) and the Food and Agriculture Organization (FAO) (Food and Agriculture Organization, 2014; United Nations Department of Economic and Social Affairs Sustainable Development, 2022). We address economic and social implications separately and not as socio-economic, while acknowledging the significance of their interrelations throughout. Furthermore, we address issues of animal health and welfare within the social pillar of sustainability, as proposed by recent research and policies regarding sustainability of livestock production systems (European Commission, 2017; Tallentire et al., 2019). Finally, we present emerging threats and risks associated with the implementation of alternative protein sources to synthesize pragmatic recommendations for a guided conversation and actions toward sustainable livestock diets. While this Review briefly discusses issues of scalability, it is our position that the potential sustainability implications and trade-offs of alternative protein feeds need to be resolved through future research that overcomes significant primary data limitations, prior to discussions regarding their implementation at large scales.
The majority of global livestock production systems rely heavily on unsustainable, plant-based sources to cover the needs for protein in livestock nutrition, the most common of which is soybean meals (Food and Agriculture Organization, 2018). The production of conventional protein crops and soy in particular is directly linked to negative environmental impacts including land degradation and deforestation, fossil fuel depletion, atmospheric pollution and global warming, acidification and eutrophication, and negative impacts to biodiversity (Semper-Pascual et al., 2019; Andretta et al., 2021; Song et al., 2021). Moreover, the production of protein crops at large scales requires large financial investments mainly associated with synthetic and chemical inputs (e.g., fertilizers, pesticides, herbicides), labor, fossil fuel, and land (e.g., rent) (Food and Agriculture Organization, 2016). Until now, these agri-environmental issues were associated primarily with the production of soy, however an uncontrolled intensification of other types of oilseed crop production to meet global livestock sector and human food requirements may raise similar concerns (Henchion et al., 2017). Therefore, the current and emerging sustainability related issues discussed in this Review should not be viewed as specific to soy production, but rather as potential implications related to conventional protein crop production more broadly.
From an economic perspective, importing and transporting protein feeds over long distances, for example importing soy from South-America to support European livestock production, incurs significant costs and risks especially considering the instabilities in global trading dynamics and volatility of fossil fuel prices (Taghizadeh-Hesary et al., 2019). Considering the social domain, production of conventional protein crops for livestock feed threatens availability of land for food and water resources suitable for human consumption, reduces quality of ecosystem services, and often threatens food safety through accumulation of hazardous chemical contaminants (Figure 1A).
Figure 1. Sustainability implications of protein crop production for livestock feeds under different protein sourcing scenarios. (A) Conventional crop protein production with associated emissions to atmosphere, soil and aquatic ecosystems and socioeconomic impacts. (B) Potential opportunities for sustainability enhancement associated with the incorporation of alternative protein ingredients in livestock feeds at commercial scales, such as protein from insects reared on waste substrates and from farmed seaweed. (C) Potential risks to sustainability, such as biological and chemical threats to feed and food safety and threats to biodiversity.
Shifting from conventional soy production to local, home-grown protein crops can help reduce land-related environmental pressures in high-risk regions of the global South (i.e., Latin America, Central Africa, Indonesia and Southeast Asia) by up to 11 times (Sasu-Boakye et al., 2014; Stévant et al., 2017). For example, fava beans, peas or lucerne (alfalfa) grown in Western Europe could substitute quantities of imported soy from South-America. Using well-established databases, we compared through life cycle analysis the production and transportation of 1 ton of soy imported in a French livestock system from Brazil, against the production of 1 ton of lucerne produced and used within France (Agribalyse, 2022). The results showed that lucerne feed was associated with up to 95% lower impact potential for global warming, 63% for land-use, 97% for freshwater eutrophication and 98% for acidification (for methodological details, see Supplementary material; Agribalyse, 2022). Climate change scenarios project that even major soy producers of the North may suffer yield reductions in the future (e.g., U.S soy 86–92% reduction by 2050), further highlighting a need to diversify protein sources with other local crops (Cordeiro et al., 2019; Yu et al., 2021). Local protein crop production can also help mitigate environmental impacts associated with packaging and transportation of feeds over long distances (e.g., cross-Atlantic), reducing approximately 10% of feed production’s total environmental footprint (Figure 1B; Taelman et al., 2015; Herrero et al., 2016).
In addition to shifting to alternative protein crops, incorporating GM/GE climate-resilient variants of soy or other oilseed crops may help improve resource efficiency in protein feed production (28% less fossil fuel and renewable energy) and reduce negative impacts, including global warming potential by up to 28% and freshwater toxicity and eutrophication by 72 and 51%, respectively (Alig and Ahearn, 2017; Eriksson et al., 2018; Paiva et al., 2020).
Protein production from alternative cultivation methods, such as seaweed (macroalgae) farming and hydroponics, may have close-to-zero requirements regarding land and water (Parsons et al., 2019; Koesling et al., 2021) and require 74–100% less fossil-fuel compared to conventional soy production (Stévant et al., 2017). Such alternatives also make minimal use of synthetic and chemical fertilizers, the production and use of which accounts for up to 95% of the environmental footprint of conventional crop production for impacts including aquatic acidification and eutrophication, and land transformation (Niero et al., 2015; Paul et al., 2018; Chen et al., 2020).
Cellular agriculture within the livestock feed production context, is defined by the use of cell-culturing biotechnologies to produce protein feeds from bacterial, fungal, or micro-algal cellular organisms. Widely used examples of cellular protein sources include yeast protein (Saccharomyces cerevisiae), protein from filamentous fungi (Fusarium graminearum), and protein from methane eating bacterial (Methylophilus methylotrophus). It requires facilities that occupy primarily urban land (e.g., laboratories, vertical farms) and therefore can help free-up large areas of arable land depending on the amounts of soy or other oilseed crops that cellular protein substitutes, while largely minimizing impacts associated with transportation of protein feeds, since they can be located close to feed manufacturers and transport hubs. Protein extraction from yeast, for example, is associated with 71% lower land-use related impacts, 34% lower global warming potential, and 67% lower eutrophication potential (Agribalyse, 2022). Furthermore, cellular-protein extraction facilities use electricity for their operations, which can be sourced more sustainably than fossil fuel used for conventional soy production. With advancements in the renewable energy sector, the environmental impacts of cellular agriculture can be minimized further, since currently the production of electricity accounts for 83–94% of global warming potential, freshwater eutrophication and terrestrial acidification associated with its implementation at large scales (Kobayashi et al., 2022).
Environmental benefits of circular agriculture approaches may hold even more potential than cellular protein. Generating protein feeds from food waste, former foods and industry by-products not only bypasses the need for arable land, but also reduces land requirements for waste disposal. For example, sourcing 1 ton of bread or biscuit meal from food waste does not involve land-use (zero impact). Moreover, depending on the treatment method of waste streams, these alternatives can generate up to 99% less global warming and acidification related emissions, and have up to 83% lower eutrophication potential compared to soy (Agribalyse, 2022). On a system level, incorporating food waste in feeds for European pork production could reduce its overall land footprint by approximately 21% and the greenhouse gases associated with relevant protein feed production by almost 12 times (Zu Ermgassen et al., 2016; Dou et al., 2018).
PAPs from insects present another environmentally sustainable alternative compared to conventional protein sources. Currently, the production and use of seven insect species (Musca domestica, Alphitobius diaperinus, Hermetia illucens, Gryllodes sigillatus, Gryllus assimilis, Tenebrio molitor, and Acheta domesticus) has been explored in-depth and legally enabled in the EU for commercial exploitation in farmed fish feeds and pet feeds (Madau et al., 2020). However, the commercial implementation of insect meals at much larger scales to support global livestock production requires further research to optimize mass rearing processes (Madau et al., 2020). Insect farming relies almost exclusively on energy that can be sourced renewably (i.e., electricity as opposed to diesel), therefore significantly reducing the energy footprint of livestock protein feeds (Asdrubali et al., 2015; Madau et al., 2020). Furthermore, it can reduce land-use related impacts by 98% and GHG emissions by 60% when compared to soy and other conventional protein meals (Figure 1B; van Zanten et al., 2015; van Huis and Oonincx, 2017; Smetana et al., 2021). Using waste streams (e.g., manure) to rear insects mitigates unintended impacts from waste disposal systems, further reducing eutrophication and acidification of ecosystems, and impacts on biodiversity (Zheng et al., 2019; Gao et al., 2021). Such strategies can reduce total nitrogen related emissions from livestock systems by up to 62% (Figure 1B; Elahi et al., 2022).
PAPs from swine and poultry by-products can achieve comparable environmental performance with insect meals (Parker, 2018). An important factor that may affect the environmental benefits of such alternatives is whether they are obtained from the slaughterhouse (as animal by-products) or retailers (e.g., market’s butcher, as food waste), due to packaging and transportation related emissions. For example, animal fat meals at the retailer are associated with up to 82% lower global warming potential compared to soy, whereas obtaining them at slaughterhouse can achieve up to 95% lower impact (Agribalyse, 2022).
Circular and local protein sourcing can significantly drive production and lower supply costs. Large reductions can be achieved immediately by minimizing the use of costly inputs used in conventional protein crops, such as fertilizers, irrigation water, pesticides/herbicides and fossil fuel (Kumar et al., 2020). Localizing and diversifying protein can help reduce transportation costs if more protein feeds are produced closer to the receiving markets or to transportation hubs (Lo et al., 2021). Because circular and soilless alternatives are less affected by the volatility of fossil fuel and synthetic input prices, they may offer relative stability for the global livestock feed market and help secure availability of feed worldwide (Lioutas and Charatsari, 2021).
Coupling local protein production with local energy sources may allow governmental authorities to gain better control over the input requirements for production and market needs of the livestock feed sector. Shifting from imported fossil fuel to local renewable energy for protein production may present a viable and more resilient future pathway for future feeds (Punzi, 2019). Furthermore, diversifying protein sources may also help avoid incidents where feed producers shift to more profitable alternatives for their land (e.g., energy crops for biofuel) in times of crises, therefore leading to a more robust livestock sector overall (United States Department of Agriculture, Foreign Agricultural Service, 2022). All these are important opportunities for economic viability of the industry, especially considering the uncertainties in on-going global trading dynamics (e.g., Brexit) and geopolitical developments (e.g., Ukraine-Russia conflict) (Taghizadeh-Hesary et al., 2019; Choi et al., 2021; Yao et al., 2021; Schiffling and Valantasis Kanellos, 2022).
While current literature identifies large gaps and uncertainties regarding the economic inputs and outputs associated with alternative protein feed production (e.g., economies of scale in mass insect rearing of different species, recycled food waste streams), some evidence points toward their economic viability and profitability (Shurson, 2020; Niyonsaba et al., 2021; Rzymski et al., 2021). A number of studies also suggests that future advancements in biotechnology may further increase cost-effectiveness through synergies between waste streams of industries that can be used as resources for protein production (e.g., food waste from restaurants, former foods from bakery, manure from livestock) (Ritala et al., 2017; Jones et al., 2020).
Furthermore, the Covid-19 pandemic has raised awareness about the investment in developing automation technologies and has driven advancements in treatment practices that eliminate the risks of pathogen and disease dispersal, which may drive growth, increasing acceptance and popularity of circular alternatives (Henry, 2020).
Social sustainability in the context of sustainable livestock production commonly refers to potential issues associated with impacts on human health and safety related to livestock farming activities, human rights, working conditions, and social development as reflected through customers and local communities’ perspectives. In this Review, we follow the suggestion of recent literature, and additionally consider the potential impacts of protein feed ingredients choices on animal health and welfare, on animal growth and performance, and in relation to the acceptability and digestibility of alternative feeds (Tallentire et al., 2019). These latter implications are discussed primarily through the scope of nutritional suitability because they are mainly associated with the presence of anti-nutritional factors and metabolites in alternative protein feeds.
From a nutritional perspective, the alternative protein sources presented here can provide animals with protein that is comparable to conventional soy (Table 1). Furthermore, they can introduce to their diets important bioactive compounds that can improve gut health, such as antimicrobial peptides, chitin, and lauric acid (Gasco et al., 2018). Algae in poultry diets can improve growth performance, laying rates and product quality (Coudert et al., 2020). GM/GE protein crops often improve feed nutrient profiles without compromising animal and human health (Buzoianu et al., 2013; Naegeli et al., 2020). Food waste can also be a good source of amino acids, minerals, fatty acids, and vitamins essential for animal growth, however there is no clear evidence that it can significantly improve animal performance. Literature suggests that food waste does not affect meat quality, while some studies propose it may even improve it (Dou et al., 2018).
Table 1. Examples of potential alternative proteins used in livestock feeds that could substitute conventional protein crop production as more sustainable protein sources.
Local protein solutions can also stimulate economic and social growth in local rural communities mitigating the negative impacts of urbanization. By diversifying protein production, local producers and smallholders may acquire a more central role in the agricultural sector (Swain and Teufel, 2017). The potential synergies to funnel waste streams from various industries as resources for livestock feeds can promote cross-sectoral knowledge sharing and collaborations, and opportunities for education as the demand for more specialized on-farm labor may increase (Marinoudi et al., 2019). On-farm work safety can be reduced significantly through production methods that minimize the use of hazardous agrochemicals (e.g., pesticides, herbicides, chemical fertilizers) and that rely on automated technologies as opposed to conventional crop production (Elahi et al., 2019). Furthermore, the potential to preserve ecosystem services and avoid negative environmental impacts contributes to improved human wellbeing and quality of life (Rukundo et al., 2018; Flach et al., 2021).
Damages or decay of grains and seeds due to poor conditions of transportation and storage can largely increase the potential risks for biological contaminations of humans through the food chain, such as outbreaks of mycotoxins or viral diseases that can cause severe human health impairments. Climate change (increased ambient temperatures and humidity in particular) and instabilities in global trading of feeds (e.g., significant delays in transportation of feeds due to Brexit or the Ukraine-Russia conflict) have further increased such risks, especially in the absence of state-of-the-art storage and transportation technologies.
Diversifying protein sources in livestock feeds through local, circular, and soilless alternatives could help reduce reliance on imported protein feeds (i.e., grains, seeds), thus reducing food and feed safety risks associated with international transportation and long duration storage, as the ones discussed above. For example, UK livestock production systems importing sunflower seeds from Ukraine, a major producer worldwide, have experienced significant disruptions in the past 2 years and have often received severely damaged and contaminated grains due to the deteriorated storage conditions in Ukraine and extremely long delays at the customs during transportation (unintended consequences of the conflict and relevant trading deals post-Brexit). Therefore, in such cases supplementing livestock diets with protein feeds sourced within the UK (e.g., fava beans, peas, protein through circular streams) could enhance livestock system resilience while largely mitigating risks to human health (Figure 1B; Becton et al., 2022).
Incorporating several of the alternative ingredients discussed here in livestock feeds, could also potentially increase human health risks through exposure to allergens and additives. For example, food waste from households, restaurants, retailers, and former foods from bakery and confectionery often contains small quantities of the majority of all 14 known allergens, such as traces of nuts, and various artificial sweeteners (e.g., sucralose) and artificial coloring agents (e.g., tartrazine). These substances can reach humans through bioaccumulation in livestock tissues, and can cause allergic reactions manifested through various symptoms ranging from skin rashes to gastrointestinal issues. However, there are also several advantages to using alternative feeds in this aspect. For example, GM/GE variants may suppress the expression of potential allergenic proteins in plant development, therefore enhancing their acceptability (Dubois et al., 2015).
Incorporating alternative protein sources at large scales may present opportunities for sustainable development; however, they may also give rise to potential unintended consequences (Table 2). In some cases, and while home-grown crops like fava beans and peas can relieve land-use related impacts in vulnerable ecosystems of the global South, their total land footprint can be higher by up to 7.6% and their marine eutrophication potential by up to 43% compared to conventional soy (Agribalyse, 2022). Furthermore, shifting protein crop production rapidly may cause a displacement of local crops and wildlife, and have potential knock-on effects on land-use (Eriksson et al., 2018). Therefore, local solutions should not be viewed as a panacea, and it is critical choosing specific home-grown crop feeds is done considering also regional vulnerabilities, for example the existence of Nitrogen Vulnerable Zones, as well as the capacity, potential, and needs of different regions for production and trading of livestock feeds and other agricultural produce that requires similar resources (e.g., crops for human food). Future research should try to provide answers to what the land-use implications in the global North would be, if the majority of livestock producers made the switch to home-grown protein, and what the production limit would be before this resulted to new, local land-related impacts.
Table 2. Summary of key opportunities and risks to sustainability and food safety associated with the implementation of alternative protein feed ingredients.
It is important to consider that circular and soilless alternatives, such as insect farming or food waste for feed, may also have unintended impacts on land-use change especially as the scale and size of production systems increase (Shah and Wu, 2019; Doi and Mulia, 2021). Soilless systems require a robust management plan to avoid the abandonment of crop land, which in combination with effects of climate change in the South, including increasing temperatures and frequencies of extreme droughts, can exert pressures on soil organic carbon and biodiversity (Pacheco et al., 2018; Olsson et al., 2019; Winkler et al., 2021).
In the aquatic ecosystems, seaweed farming can also create competition for light and nutrients between cultivated and wild species (e.g., planktonic communities), pollution from artificial material as farming infrastructure, noise disturbances to animals due to increased vessel activity in the area and may significantly alter the geomorphology of coastal ecosystems (Figure 1C; Campbell et al., 2019).
Finally, while there is a strong case for the GHG emission reductions that alternative protein feeds can achieve, there may be unintended increases in N and P concentrations in the manure and urea of livestock animals if they are fed alternatives that provide more imbalanced protein compared to soy and other conventional feeds (Trabue et al., 2021).
To date, hydroponics appear to be less energy efficient than other conventional crop production methods. For example, the production of 1 ton of hydroponic fodder requires approximately 60% more energy (mainly due to electricity consumption) than 1 ton of soy (Agribalyse, 2022). However, advancements in renewable energy sourcing open up far more opportunities for improvement of energy efficiency for hydroponics than conventional crop production, since the latter relies much more on fossil fuel.
Much of literature is conflicted about the economic viability of local, circular and soilless alternatives implemented at large scales. For example, seaweed farming, insect farming and cellular alternatives may be a good solution when implemented in less-developed countries especially as post-harvest processing technologies and biotechnologies become better and more affordable (Duarte et al., 2021), but not a cost-effective industry in the North due to the higher labor and energy related costs (van den Burg et al., 2016; Emblemsvåg et al., 2020). Such economic constraints may present challenges for their marketability as commercial feeds due to the low prices of competing conventional protein sources (Arru et al., 2019).
A critical condition for circular agriculture alternatives to be viable and cost-effective is the proper treatment of waste streams prior to their use as feed or feedstock, to minimize the risks of pathogen and disease outbreaks that may result to severe economic consequences through impaired animal performance (Dou et al., 2018). Ensuring high hygiene standards through timely collection of the waste, thermal treatment, appropriate transportation and handling practices incurs costs that need to be accounted for when evaluating the feasibility of such feeding strategies (Figure 1C; Pinotti et al., 2021; Rajeh et al., 2021).
While alternative protein sources may not necessarily jeopardize animal growth, there is a risk in achieving a comparable crude protein utilization with conventional protein sources that may affect optimal animal performance (e.g., carcass composition), especially compared to what the more balanced protein sources can offer (e.g., soy) (Gasco et al., 2019; Luciano et al., 2020). It can also be difficult to persuade livestock animals to consume the quantities of alternative protein sources required to achieve optimal growth (Mainardes and DeVries, 2016). Even when palatability is not a critical issue for animal welfare, the inclusion levels of alternative protein sources should be carefully considered due to anti-nutritional factors that can be toxic beyond certain concentrations. For example, leguminous feeds contain mimosine and its metabolites, which can be toxic for most livestock species at high concentrations (Lakshmi et al., 2020). While the development of sweet varieties of lupins have mitigated issues of toxicity caused by high levels of alkaloids, other anti-nutritional factors like non-starch polysaccharides may impair animal performance and are therefore a limiting factor for use at large scales (Olkowski, 2018). Food waste with plant components can contain high concentrations of enzyme inhibitors like tannins and alkaloids, which reduce feed intake and nutrient utilization (Georganas et al., 2020). Food wastes and former foods also may contain secondary metabolites and toxins that can be harmful to animals, such as chocolate residues that are high in theobromine (Makinde et al., 2019; Klein et al., 2021).
Consumer perception and acceptance has always been a big concern and a barrier to the adoption of alternative proteins for livestock feed (Figure 1C). Although meat consumers and livestock farmers seem to be positive about alternatives such as insects, algae, and lab-grown feeds used in livestock production there is still much to be explored regarding how to maximize marketability and acceptance of livestock fed with protein alternatives (Verbeke et al., 2015; Onwezen et al., 2019). Such opposing attitudes and cultural biases (e.g., disgust factor) are often being developed and maintained through misinformation by media (Altmann et al., 2022; Khaemba et al., 2022). Livestock producers may attempt to overcome such issues of marketability by withholding relevant information, which consists of feed and food fraud and mislabeling violations, and can be often noticed when such alternatives as GM/GE crops, animal by-products, and insect meals are used (Montgomery et al., 2020). Such incidents not only threaten customer trust and acceptance, but also food security since they may often exclude vital information about potential sources of fungal, bacterial, or chemical contamination.
A major food safety concern associated with alternatives that upgrade waste streams to protein is that of the bioaccumulation of biological and chemical contaminants (Figure 1C). The EU and US have very strict regulations that require food waste, former foods and wastewater to be thoroughly treated (e.g., hydrothermal treatment at 110°C for an hour) for viral and bacterial contaminants, and require the precise detection and treatment of chemical contaminants including toxic heavy metals, traces of pesticides and nanoplastics (Dou et al., 2018; Pinotti et al., 2019). This is a critical condition that currently limits the use of such alternatives as livestock feeds in wide scales in those regions (EU and US), or even as substrates for insect farming, seaweed farming and cellular protein production (Dou et al., 2018; Pinotti et al., 2019). Other regions, like countries of Southeast Asia (e.g., South Korea) also have started implementing precise protocols for the systematic regulation of food waste hygienic processing to enable their commercial incorporation in livestock feeds (Dou et al., 2018).
Sourcing protein from seaweed that is grown in coastal waters also has been associated with human food safety issues due to the heavy metal accumulation such as arsenic, cadmium and copper (Tirado et al., 2010). Such contaminants can travel from protein feeds to livestock animals and then reach humans where they can cause severe immediate effects like inflammatory responses, disruption on gut microbiota and effects on nutrient absorption, and chronic inflammation that increases the risks for cancer (Smith et al., 2018; Magnoli et al., 2019; Prata et al., 2020).
Another critical biosecurity concern is associated with the re-introduction of PAPs in the EU from swine and poultry. This should be done following strict protocols of cross-species feeding to avoid disease outbreaks similar to the BSE/TSE epidemic of the 1980s that occurred in the UK (Woodgate and Wilkinson, 2021). As a consequence of such past incidents ruminant PAPs are still prohibited in the EU, with the exception of collagen, gelatine, and milk, that should strictly be fed to non-ruminant species. In the US, only a very small number of pig production farms currently uses food waste that contains animal parts as feed, following thorough thermal processing at licensed facilities (Dou et al., 2018).
Insect meals can also be disease vectors or carry harmful heavy metals. The potential for such threats to be realized is largely increased when wastewater and/or waste substrates are used for their rearing, particularly in the absence of precise treatment methods as mentioned above.
Finally, we highlight the importance to consider the introduction of novel allergens in the human as a potential biosecurity threat. Several of the alternatives discussed here contain the majority of all known food allergens (e.g., food waste, home-grown protein, former foods particularly nuts, animal by-products). These allergens may be transferred down the food chain causing severe human allergies and even death (Advisory Committee on Animal Feedingstuffs, 2009; Testa et al., 2017; Bingemann et al., 2019).
Substituting conventional soy and other protein crops in livestock feeds with more sustainable alternatives should undoubtedly be a priority of the livestock and agri-food sector (Song et al., 2021; European Parliamentary Research Service, 2022). As we expect to experience several environmental and socioeconomic threats to feed and food security in the next 2–10 years, building a resilient protein supply is critical. The Ukraine-Russia conflict has not only blocked the supply of sunflower meals from one of the largest producers globally (Ukraine), but has also frozen large European investments that aimed to support local Ukrainian soy production aiming to replace unsustainable imports from South America. Other geo-political developments including Brexit and disease outbreaks (African Swine Fever in Southeast Asia) have exacerbated feelings of insecurity of agricultural stakeholders due to uncertainty around future trading partners, impaired production, disrupted supply of labor, import/duty policies and limited support through subsidies. Climate-related impacts and extreme weather events may lead to impaired productivity and poorer nutrient profiles of conventional protein feeds and increased outbreaks of biological contaminants (Alava et al., 2017).
Alternative protein sources have already been used to substitute protein crops in small scales, as in pet feeds or feeds for fish (aquaculture). Among the most popular examples are insect meals, swine and poultry PAPs used in the feeds of domestic canines and felines. While to some extent, this example may be used to illustrate the potential for these alternatives to be used safely for livestock, there are still several obstacles—mainly socioeconomic—to be overcome prior to their being sustainably produced and integrated into livestock feeds at scale. There appears to be no “silver bullet, free from trade-offs” livestock feed formulation that can guarantee global sustainability across all three pillars. Literature has identified stakeholder dialogue as imperative to evaluate the effectiveness of solutions for sustainable development through the understanding and assessment of potential trade-offs associated with their implementation (Hebinck et al., 2021). Through our analysis of the potential opportunities, risks, and trade-offs of solutions for improved livestock feed sustainability, we invite a dialogue between relevant stakeholders and the co-development of a set of livestock diet scenarios specific to the different livestock species. We propose that in the center of this approach needs to be a shift toward more sustainable local, circular and soilless protein sources. Key sustainability trade-offs can then be quantified for each scenario which will inform region-specific policies for sustainable livestock production. We synthesize four key strategic regional policy pathways to guide future livestock feed formulation in consideration of global sustainable development goals (Table 3; United Nations Department of Economic and Social Affairs Sustainable Development, 2022).
• Decoupling protein production from fossil fuel by shifting to alternatives that rely almost exclusively on renewable energy (e.g., solar, wind, geothermal) will reduce the overall environmental footprint of livestock feeds. This needs to be supported by a strict regulation monitoring renewable energy price to ensure the economic viability of alternatives and feed market stability, as well as by further research that may enable uninterrupted and abundant supply of energy from such sources.
• Developing economic strategies for alternative proteins at subnational level, as opposed to lateral measures and policies may facilitate adoption of these solutions at larger scales, especially considering the geographic variability in labor and direct input costs, taxes, and support through subsidies. We propose that future policies financially incentivize local protein production and consumption to help the shift from the less expensive, unsustainable imported alternatives.
• Understanding and addressing social biases against circular livestock feed solutions through efficient stakeholder engagement. This is critical to ensure marketability and overall economic viability of circular alternatives, and therefore facilitate their implementation at large scales. Further exploration of stakeholder concerns may help identify additional risks and unintended consequences of such alternatives, thus we propose that the development of future livestock feed scenarios considers all stakeholders perspectives.
• Further enhancing feed and food safety with improved protocols regarding the precise and early detection and monitoring of biological and chemical contaminants in alternative feeds. This is imperative for enabling the safe adoption of alternatives like cellular and insect protein reared on waste substrates, food waste and former foods as protein sources, and PAPs. Emerging feed and food security threats, like the impacts of climate change and storage/transportation conditions on biological contaminant blooms, should be considered throughout future livestock feed scenarios.
Table 3. Example of interactions between alternative protein sources for livestock feeds and global sustainable goals.
Immediate action is required to reshape the global livestock feed market and enhance its future resilience to environmental, macro-economic and geopolitical instabilities (e.g., climate change, Ukraine conflict, energy crisis). Future research should focus on the quantification of synergies and trade-offs between sustainable protein solutions and within and between sustainability pillars to enable accurate spatiotemporal comparisons of alternatives and facilitate regionalized decision making. Any discussion regarding sustainability trade-offs in livestock feed production must include a detailed analysis of who benefits when there are benefits, and who losses when there are losses. It is the position of this Review that such sustainability concerns should be fully addressed and resolved prior to efforts for implementation at larger scales. Research into alternative sources suggests that the converse, i.e., considering scalability as a priority, is likely to lead to problems of adoption (Marcellin et al., 2022). Anticipatory policies should be in place to compensate for losses through such trade-offs and to scope the future of the livestock sector beyond the time horizon suggested by the current sustainability agendas (e.g., 2030 as in UN SDGs). The relevant discussions should now also focus on how circularity and localization of protein feeds can fit and synergize with relevant sustainability policies about regenerative and transformative agricultural systems to form clear guidelines for a more sustainable agri-food sector.
GP contributed in the study design, performed the literature review and life cycle assessment, analyzed and interpreted all data and information, and was the primary contributor in writing, reviewing, and approving the manuscript also acting as the corresponding author. BD was responsible for funding acquisition, contributed in the study design, supervised the manuscript writing process, and reviewed and approved the final manuscript. IK was responsible for funding acquisition, a major contributor in the study design, supervised the manuscript writing process, and reviewed and approved the final manuscript. All authors contributed to the article and approved the submitted version.
The project was co-funded by the Food Standards Agency (FSA) Grant number FS900202, and the Economic and Social Research Council (ESRC) Impact Accelerator Accounts of Queen’s University Belfast and University of York. The authors confirm that there has been no significant financial support provided for this work that could have influenced this outcome.
The authors declare that the research was conducted in the absence of any commercial or financial relationships that could be construed as a potential conflict of interest.
All claims expressed in this article are solely those of the authors and do not necessarily represent those of their affiliated organizations, or those of the publisher, the editors and the reviewers. Any product that may be evaluated in this article, or claim that may be made by its manufacturer, is not guaranteed or endorsed by the publisher.
The Supplementary material for this article can be found online at: https://www.frontiersin.org/articles/10.3389/fsufs.2023.1188467/full#supplementary-material
Advisory Committee on Animal Feedingstuffs (2009). Potential for carry-over of allergens from animal feed into derived animal products. Available at: https://acaf.food.gov.uk/sites/default/files/mnt/drupal_data/sources/files/multimedia/pdfs/committee/acaf0904.pdf (Accessed March 20, 2022).
Agribalyse. (2022). Agribalyse version 3.1. Life cycle inventory database for the agriculture and food sector. ADEME. Available at: https://doc.agribalyse.fr/documentation-en/agribalyse-data/data-access (Accessed November 10, 2022).
Alava, J. J., Cheung, W. W., Ross, P. S., and Sumaila, U. R. (2017). Climate change–contaminant interactions in marine food webs: toward a conceptual framework. Glob. Chang. Biol. 23, 3984–4001. doi: 10.1111/gcb.13667
Alig, R. J., and Ahearn, M. C. (2017). “Effects of policy and technological change on land use” in Economics of rural land-use change. eds. K. P. Bell, K. J. Boyle, and J. Rubin (Oxfordshire, England, UK: Routledge), 43–56.
Altmann, B. A., Anders, S., Risius, A., and Mörlein, D. (2022). Information effects on consumer preferences for alternative animal feedstuffs. Food Policy 106:102192. doi: 10.1016/j.foodpol.2021.102192
Andretta, I., Hickmann, F. M., Remus, A., Franceschi, C. H., Mariani, A. B., Orso, C., et al. (2021). Environmental impacts of pig and poultry production: insights from a systematic review. Front. Vet. Sci. 8:750733. doi: 10.3389/fvets.2021.750733
Arru, B., Furesi, R., Gasco, L., Madau, F. A., and Pulina, P. (2019). The introduction of insect meal into fish diet: the first economic analysis on European sea bass farming. Sustainability 11:1697. doi: 10.3390/su11061697
Asdrubali, F., Baldinelli, G., D’Alessandro, F., and Scrucca, F. (2015). Life cycle assessment of electricity production from renewable energies: review and results harmonization. Renew. Sust. Energ. Rev. 42, 1113–1122. doi: 10.1016/j.rser.2014.10.082
Becton, L., Davis, P., Sundberg, P., and Wilkinson, L. (2022). Feed safety collaborations: experiences, progress and challenges. Transbound. Emerg. Dis. 69, 182–188. doi: 10.1111/tbed.14297
Ben Hassen, T., and El Bilali, H. (2022). Impacts of the Russia-Ukraine war on global food security: towards more sustainable and resilient food systems? Foods 11:2301. doi: 10.3390/foods11152301
Bingemann, T. A., Santos, C. B., Russell, A. F., and Anagnostou, A. (2019). Lupin: an emerging food allergen in the United States. Ann. Allergy Asthma Immunol. 122, 8–10. doi: 10.1016/j.anai.2018.09.467
Buzoianu, S. G., Walsh, M. C., Rea, M. C., Cassidy, J. P., Ryan, T. P., Ross, R. P., et al. (2013). Transgenerational effects of feeding genetically modified maize to nulliparous sows and offspring on offspring growth and health. J. Anim. Sci. 91, 318–330. doi: 10.2527/jas.2012-5360
Cabezas, S. C., Bellfield, H., Lafortune, G., Streck, C., and Hermann, B. (2019). Towards more sustainability in the soy supply chain: how can EU actors support zero-deforestation and SDG efforts. Climate focus. Deutsche Gesellschaft für Internationale Zusammenarbeit (GIZ), Federal Ministry for Economic Cooperation and Development. Available at: https://globalcanopy.org/wp-content/uploads/2020/12/20191209_ClimateFocus_GIZ_SoySupplyChain.pdf. (Accessed May 20, 2022).
Campbell, I., Macleod, A., Sahlmann, C., Neves, L., Funderud, J., Øverland, M., et al. (2019). The environmental risks associated with the development of seaweed farming in Europe-prioritizing key knowledge gaps. Front. Mar. Sci. 6:107. doi: 10.3389/fmars.2019.00107
Chen, P., Zhu, G., Kim, H. J., Brown, P. B., and Huang, J. Y. (2020). Comparative life cycle assessment of aquaponics and hydroponics in the Midwestern United States. J. Clean. Prod. 275:122888. doi: 10.1016/j.jclepro.2020.122888
Choi, H. S., Jansson, T., Matthews, A., and Mittenzwei, K. (2021). European agriculture after Brexit: does anyone benefit from the divorce? J. Agric. Econ. 72, 3–24. doi: 10.1111/1477-9552.12396
Cordeiro, M. R., Rotz, A., Kroebel, R., Beauchemin, K. A., Hunt, D., Bittman, S., et al. (2019). Prospects of forage production in northern regions under climate and land-use changes: a case-study of a dairy farm in Newfoundland, Canada. Agronomy 9:31. doi: 10.3390/agronomy9010031
Coudert, E., Baéza, E., and Berri, C. (2020). Use of algae in poultry production: a review. Worlds Poult. Sci. J. 76, 767–786. doi: 10.1080/00439339.2020.1830012
de Visser, C., Schreuder, R., and Stoddard, F. (2014). The EU’s dependence on soya bean import for the animal feed industry and potential for EU produced alternatives. Oilseeds Fats Crops Lipids 21:D407. doi: 10.1051/ocl/2014021
del Mar Contreras, M., Lama-Muñoz, A., Gutiérrez-Pérez, J. M., Espínola, F., Moya, M., and Castro, E. (2019). Protein extraction from Agri-food residues for integration in biorefinery: potential techniques and current status. Bioresour. Technol. 280, 459–477. doi: 10.1016/j.biortech.2019.02.040
DiGiacomo, K., and Leury, B. J. (2019). Insect meal: a future source of protein feed for pigs? Animal 13, 3022–3030. doi: 10.1017/S1751731119001873
Doi, H., and Mulia, R. N. (2021). Future land use for insect meat production among countries: a global classification. Front. Nutr. 8:661056. doi: 10.3389/fnut.2021.661056
Dou, Z., Toth, J. D., and Westendorf, M. L. (2018). Food waste for livestock feeding: feasibility, safety, and sustainability implications. Glob. Food Sec. 17, 154–161. doi: 10.1016/j.gfs.2017.12.003
Duarte, C. M., Bruhn, A., and Krause-Jensen, D. (2021). A seaweed aquaculture imperative to meet global sustainability targets. Nat. Sustain. 5, 185–193. doi: 10.1038/s41893-021-00773-9
Dubois, A. E., Pagliarani, G., Brouwer, R. M., Kollen, B. J., Dragsted, L. O., Eriksen, F. D., et al. (2015). First successful reduction of clinical allergenicity of food by genetic modification: mal d 1-silenced apples cause fewer allergy symptoms than the wild-type cultivar. Allergy 70, 1406–1412. doi: 10.1111/all.12684
Edwards, H. M. 3rd, Douglas, M. W., Parsons, C. M., and Baker, D. H. (2000). Protein and energy evaluation of soybean meals processed from genetically modified high-protein soybeans. Poult. Sci. 79, 525–527. doi: 10.1093/ps/79.4.525
Elahi, E., Weijun, C., Zhang, H., and Abid, M. (2019). Use of artificial neural networks to rescue agrochemical-based health hazards: a resource optimisation method for cleaner crop production. J. Clean. Prod. 238:117900. doi: 10.1016/j.jclepro.2019.117900
Elahi, U., Xu, C., Wang, J., Lin, J., Wu, S., Zhang, H., et al. (2022). Insect meal as a feed ingredient for poultry. Anim. Biosci. 35, 332–346. doi: 10.5713/ab.21.0435
Emblemsvåg, J., Kvadsheim, N. P., Halfdanarson, J., Koesling, M., Nystrand, B. T., Sunde, J., et al. (2020). Strategic considerations for establishing a large-scale seaweed industry based on fish feed application: a Norwegian case study. J. Appl. Phycol. 32, 4159–4169. doi: 10.1007/s10811-020-02234-w
Eriksson, M., Ghosh, R., Hansson, E., Basnet, S., and Lagerkvist, C. J. (2018). Environmental consequences of introducing genetically modified soy feed in Sweden. J. Clean. Prod. 176, 46–53. doi: 10.1016/j.jclepro.2017.12.113
Ertl, P., Knaus, W., and Zollitsch, W. (2016). An approach to including protein quality when assessing the net contribution of livestock to human food supply. Animal 10, 1883–1889. doi: 10.1017/S1751731116000902
European Commission. (2017). Study on the application of the broilers directive (DIR 2007/43/EC) and development of welfare indicators. Directorate General for Health and Food Safety, Luxembourg.
European Fat Processors and Renderers (2022). The facts about processed animal proteins. Available at: https://efpra.eu/publications/ (Accessed May 20, 2022).
European Parliamentary Research Service. (2022). Towards deforestation-free commodities and products in the EU. Available at: https://www.europarl.europa.eu/RegData/etudes/BRIE/2022/698925/EPRS_BRI(2022)698925_EN.pdf (Accessed February 15, 2022).
Flach, R., Abrahão, G., Bryant, B., Scarabello, M., Soterroni, A. C., Ramos, F. M., et al. (2021). Conserving the Cerrado and Amazon biomes of Brazil protects the soy economy from damaging warming. World Dev. 146:105582. doi: 10.1016/j.worlddev.2021.105582
Food and Agriculture Organization (2014). Sustainability assessment of food and agriculture systems – guidelines version 3.0. Available at: https://www.fao.org/3/i3957e/i3957e.pdf (Accessed February 10, 2022).
Food and Agriculture Organization. (2016). Handbook on agricultural cost of production statistics: guidelines for data collection, compilation and dissemination. FAO, Rome.
Food and Agriculture Organization (2018). World livestock: transforming the livestock sector through the sustainable development goals. Rome, Italy: Food and Agriculture Organisation (FAO).
Gao, G., Gao, L., Jiang, M., Jian, A., and He, L. (2021). The potential of seaweed cultivation to achieve carbon neutrality and mitigate deoxygenation and eutrophication. Environ. Res. Lett. 17:014018. doi: 10.1088/1748-9326/ac3fd9
Gasco, L., Biasato, I., Dabbou, S., Schiavone, A., and Gai, F. (2019). Animals fed insect-based diets: state-of-the-art on digestibility, performance and product quality. Animals 9:170. doi: 10.3390/ani9040170
Gasco, L., Finke, M., and Van Huis, A. (2018). Can diets containing insects promote animal health? J. Insects Food Feed 4, 1–4. doi: 10.3920/JIFF2018.x0001
Georganas, A., Giamouri, E., Pappas, A. C., Papadomichelakis, G., Galliou, F., Manios, T., et al. (2020). Bioactive compounds in food waste: a review on the transformation of food waste to animal feed. Foods 9:291. doi: 10.3390/foods9030291
Giraldo, P. A., Shinozuka, H., Spangenberg, G. C., Cogan, N. O., and Smith, K. F. (2019). Safety assessment of genetically modified feed: is there any difference from food? Front. Plant Sci. 10:1592. doi: 10.3389/fpls.2019.01592
Glencross, B. D., Huyben, D., and Schrama, J. W. (2020). The application of single-cell ingredients in aquaculture feeds—a review. Aust. Fish. 5:22. doi: 10.3390/fishes5030022
Gurgel, A. C., Reilly, J., and Blanc, E. (2021). Challenges in simulating economic effects of climate change on global agricultural markets. Clim. Chang. 166, 1–21. doi: 10.1007/s10584-021-03119-8
Hebinck, A., Zurek, M., Achterbosch, T., Forkman, B., Kuijsten, A., Kuiper, M., et al. (2021). A sustainability compass for policy navigation to sustainable food systems. Glob. Food Sec. 29:100546. doi: 10.1016/j.gfs.2021.100546
Henchion, M., Hayes, M., Mullen, A. M., Fenelon, M., and Tiwari, B. (2017). Future protein supply and demand: strategies and factors influencing a sustainable equilibrium. Foods 6:53. doi: 10.3390/foods6070053
Henry, R. (2020). Innovations in agriculture and food supply in response to the COVID-19 pandemic. Mol. Plant 13, 1095–1097. doi: 10.1016/j.molp.2020.07.011
Herrero, M., Henderson, B., Havlík, P., Thornton, P. K., Conant, R. T., Smith, P., et al. (2016). Greenhouse gas mitigation potentials in the livestock sector. Nat. Clim. Chang. 6, 452–461. doi: 10.1038/nclimate2925
Jones, S. W., Karpol, A., Friedman, S., Maru, B. T., and Tracy, B. P. (2020). Recent advances in single cell protein use as a feed ingredient in aquaculture. Curr. Opin. Biotechnol. 61, 189–197. doi: 10.1016/j.copbio.2019.12.026
Kamal, H., Le, C. F., Salter, A. M., and Ali, A. (2021). Extraction of protein from food waste: an overview of current status and opportunities. Compr. Rev. Food Sci. Food Saf. 20, 2455–2475. doi: 10.1111/1541-4337.12739
Khaemba, C. N., Kidoido, M. M., Owuor, G., and Tanga, C. M. (2022). Consumers’ perception towards eggs from laying hens fed commercial black soldier fly (Hermetia illucens) larvae meal-based feeds. Poult. Sci. 101:101645. doi: 10.1016/j.psj.2021.101645
Khoshnevisan, B., Tabatabaei, M., Tsapekos, P., Rafiee, S., Aghbashlo, M., Lindeneg, S., et al. (2020). Environmental life cycle assessment of different biorefinery platforms valorizing municipal solid waste to bioenergy, microbial protein, lactic and succinic acid. Renew. Sust. Energ. Rev. 117:109493. doi: 10.1016/j.rser.2019.109493
Klein, C., Feist, M., Knubben-Schweizer, G., and Dobenecker, B. (2021). Case of suspected theobromine poisoning in dairy cattle. J. Anim. Physiol. Anim. Nutr. 105, 997–1001. doi: 10.1111/jpn.13469
Kobayashi, Y., Kärkkäinen, E., Häkkinen, S. T., Nohynek, L., Ritala, A., Rischer, H., et al. (2022). Life cycle assessment of plant cell cultures. Sci. Total Environ. 808:151990. doi: 10.1016/j.scitotenv.2021.151990
Koesling, M., Kvadsheim, N. P., Halfdanarson, J., Emblemsvåg, J., and Rebours, C. (2021). Environmental impacts of protein-production from farmed seaweed: comparison of possible scenarios in Norway. J. Clean. Prod. 307:127301. doi: 10.1016/j.jclepro.2021.127301
Kumar, K., Gambhir, G., Dass, A., Tripathi, A. K., Singh, A., Jha, A. K., et al. (2020). Genetically modified crops: current status and future prospects. Planta 251:91. doi: 10.1007/s00425-020-03372-8
Kusumaningtyas, R., and van Gelder, J. W. (2019). Setting the bar for deforestation-free soy in Europe. A benchmark to assess the suitability of voluntary standard systems. Profundo Research and Advice, IUCN National Committee, Amsterdam.
Lakshmi, R., Kumari, K., Adegbeye, M., and Ravikanth, P. (2020). Anti-nutritional factors in Indian leguminous top feeds: a review on their feeding management. Int. J. Livest. Res. 10:8. doi: 10.5455/ijlr.20200326042936
Leroy, F., Beal, T., Gregorini, P., McAuliffe, G. A., and van Vliet, S. (2022). Nutritionism in a food policy context: the case of ‘animal protein’. Anim. Prod. Sci. 62, 712–720. doi: 10.1071/AN21237
Lioutas, E. D., and Charatsari, C. (2021). Enhancing the ability of agriculture to cope with major crises or disasters: what the experience of COVID-19 teaches us. Agric. Syst. 187:103023. doi: 10.1016/j.agsy.2020.103023
Lo, B., Kasapis, S., and Farahnaky, A. (2021). Lupin protein: isolation and techno-functional properties, a review. Food Hydrocoll. 112:106318. doi: 10.1016/j.foodhyd.2020.106318
Luciano, A., Tretola, M., Ottoboni, M., Baldi, A., Cattaneo, D., and Pinotti, L. (2020). Potentials and challenges of former food products (food leftover) as alternative feed ingredients. Animals 10:125. doi: 10.3390/ani10010125
Madau, F. A., Arru, B., Furesi, R., and Pulina, P. (2020). Insect farming for feed and food production from a circular business model perspective. Sustainability 12:5418. doi: 10.3390/su12135418
Magnoli, A. P., Poloni, V. L., and Cavaglieri, L. (2019). Impact of mycotoxin contamination in the animal feed industry. Curr. Opin. Food Sci. 29, 99–108. doi: 10.1016/j.cofs.2019.08.009
Mainardes, G. A., and DeVries, T. J. (2016). Effect of social feeding environment on the feeding behaviour of dairy cows and their willingness to consume a novel feed. Appl. Anim. Behav. Sci. 185, 23–29. doi: 10.1016/j.applanim.2016.10.002
Makinde, O. J., Okunade, S. A., Opoola, E., Sikiru, A. B., Ajide, S. O., and Elaigwu, S. (2019). “Exploration of cocoa (Theobroma cacao) by-products as valuable potential resources in livestock feeds and feeding systems” in Theobroma Cacao-deploying science for sustainability of global cocoa economy. ed. P. O. Aikpokpodion (London, England, UK: IntechOpen).
Makkar, H. P. S. (2018). Feed demand landscape and implications of food-not feed strategy for food security and climate change. Animal 12, 1744–1754. doi: 10.1017/S175173111700324X
Marcellin, E., Angenent, L. T., Nielsen, L. K., and Molitor, B. (2022). Recycling carbon for sustainable protein production using gas fermentation. Curr. Opin. Biotechnol. 76:102723. doi: 10.1016/j.copbio.2022.102723
Marinoudi, V., Sørensen, C. G., Pearson, S., and Bochtis, D. (2019). Robotics and labour in agriculture, A context consideration. Biosyst. Eng. 184, 111–121. doi: 10.1016/j.biosystemseng.2019.06.013
Montgomery, H., Haughey, S. A., and Elliott, C. T. (2020). Recent food safety and fraud issues within the dairy supply chain (2015–2019). Glob. Food Sec. 26:100447. doi: 10.1016/j.gfs.2020.100447
Morais, T., Inácio, A., Coutinho, T., Ministro, M., Cotas, J., Pereira, L., et al. (2020). Seaweed potential in the animal feed: a review. J. Mar. Sci. Eng. 8:559. doi: 10.3390/jmse8080559
Naegeli, H., Bresson, J. L., Dalmay, T., Dewhurst, I. C., Epstein, M. M., Firbank, L. G., et al. (2020). Assessment of genetically modified soybean SYHT 0H2 for food and feed uses, import and processing, under regulation (EC) no 1829/2003 (application EFSA-GMO-DE-2012-111). EFSA J. 18:e05946. doi: 10.2903/j.efsa.2020.5946
Niero, M., Ingvordsen, C. H., Jørgensen, R. B., and Hauschild, M. Z. (2015). How to manage uncertainty in future life cycle assessment (LCA) scenarios addressing the effect of climate change in crop production. J. Clean. Prod. 107, 693–706. doi: 10.1016/j.jclepro.2015.05.061
Niyonsaba, H. H., Höhler, J., Kooistra, J., Van der Fels-Klerx, H. J., and Meuwissen, M. P. M. (2021). Profitability of insect farms. J. Insects Food Feed 7, 923–934. doi: 10.3920/JIFF2020.0087
Olkowski, B. (2018). Feeding high lupine based diets for broiler chickens: effect of soybean meal substitution with yellow lupine meal at various time points of growth cycle. Livest. Sci. 218, 114–118. doi: 10.1016/j.livsci.2018.10.017
Olsson, L., Barbosa, H., Bhadwal, S., Cowie, A., Delusca, K., Flores-Renteria, D., et al. (2019). “Land degradation” in Climate change and land: an IPCC special report on climate change, desertification, land degradation, sustainable land management, food security, and greenhouse gas fluxes in terrestrial ecosystems. eds. P. R. Shukla, J. Skea, E. Calvo Buendia, V. Masson-Delmotte, and H. O. Pörtner, et al. Geneva, Switzerland: The Intergovernmental Panel on Climate Change (IPCC).
Onwezen, M. C., Van den Puttelaar, J., Verain, M. C. D., and Veldkamp, T. (2019). Consumer acceptance of insects as food and feed: the relevance of affective factors. Food Qual. Prefer. 77, 51–63. doi: 10.1016/j.foodqual.2019.04.011
Pacheco, F. A. L., Fernandes, L. F. S., Junior, R. F. V., Valera, C. A., and Pissarra, T. C. T. (2018). Land degradation: multiple environmental consequences and routes to neutrality. Curr. Opin. Environ. Sci. Health 5, 79–86. doi: 10.1016/j.coesh.2018.07.002
Paiva, P. F. P. R., De Lourdes Pinheiro Ruivo, M., Da Silva Júnior, O. M., De Nazaré Martins Maciel, M., Braga, T. G. M., De Andrade, M. M. N., et al. (2020). Deforestation in protect areas in the Amazon: a threat to biodiversity. Biodivers. Conserv. 29, 19–38. doi: 10.1007/s10531-019-01867-9
Parker, R. (2018). Implications of high animal by-product feed inputs in life cycle assessments of farmed Atlantic salmon. Int. J. Life Cycle Assess. 23, 982–994. doi: 10.1007/s11367-017-1340-9
Parsons, S., Allen, M. J., Abeln, F., McManus, M., and Chuck, C. J. (2019). Sustainability and life cycle assessment (LCA) of macroalgae-derived single cell oils. J. Clean. Prod. 232, 1272–1281. doi: 10.1016/j.jclepro.2019.05.315
Paul, M. J., Nuccio, M. L., and Basu, S. S. (2018). Are GM crops for yield and resilience possible? Trends Plant Sci. 23, 10–16. doi: 10.1016/j.tplants.2017.09.007
Pignolet, O., Jubeau, S., Vaca-Garcia, C., and Michaud, P. (2013). Highly valuable microalgae: biochemical and topological aspects. J. Ind. Microbiol. Biotechnol. 40, 781–796. doi: 10.1007/s10295-013-1281-7
Pilarska, A. A., Pilarski, K., Wolna-Maruwka, A., Boniecki, P., and Zaborowicz, M. (2018). Use of confectionery waste in biogas production by the anaerobic digestion process. Molecules 24:37. doi: 10.3390/molecules24010037
Pinotti, L., Giromini, C., Ottoboni, M., Tretola, M., and Marchis, D. (2019). Insects and former foodstuffs for upgrading food waste biomasses/streams to feed ingredients for farm animals. Animal 13, 1365–1375. doi: 10.1017/S1751731118003622
Pinotti, L., Luciano, A., Ottoboni, M., Manoni, M., Ferrari, L., Marchis, D., et al. (2021). Recycling food leftovers in feed as opportunity to increase the sustainability of livestock production. J. Clean. Prod. 294:126290. doi: 10.1016/j.jclepro.2021.126290
Prata, J. C., da Costa, J. P., Lopes, I., Duarte, A. C., and Rocha-Santos, T. (2020). Environmental exposure to microplastics: an overview on possible human health effects. Sci. Total Environ. 702:134455. doi: 10.1016/j.scitotenv.2019.134455
Punzi, M. T. (2019). The impact of energy price uncertainty on macroeconomic variables. Energy Policy 129, 1306–1319. doi: 10.1016/j.enpol.2019.03.015
Rajeh, C., Saoud, I. P., Kharroubi, S., Naalbandian, S., and Abiad, M. G. (2021). Food loss and food waste recovery as animal feed: a systematic review. J. Mater. Cycles Waste Manag 23, 1–17. doi: 10.1007/s10163-020-01102-6
Ritala, A., Häkkinen, S. T., Toivari, M., and Wiebe, M. G. (2017). Single cell protein—state-of-the-art, industrial landscape and patents 2001–2016. Front. Microbiol. 8:2009. doi: 10.3389/fmicb.2017.02009
Robinson, T. P., Thornton, P. K., Franceschini, G. N., Kruska, R. L., Chiozza, F., Notenbaert, A. M. O., et al. (2011). Global livestock production systems. Rome, Italy: FAO and ILRI.
Roques, S., Koopmans, S. J., Mens, A., van Harn, J., van Krimpen, M., and Kar, S. K. (2022). Effect of feeding 0.8% dried powdered Chlorella vulgaris biomass on growth performance, immune response, and intestinal morphology during grower phase in broiler chickens. Animals 12:1114. doi: 10.3390/ani12091114
Rukundo, E., Liu, S., Dong, Y., Rutebuka, E., Asamoah, E. F., Xu, J., et al. (2018). Spatio-temporal dynamics of critical ecosystem services in response to agricultural expansion in Rwanda, East Africa. Ecol. Indic. 89, 696–705. doi: 10.1016/j.ecolind.2018.02.032
Rzymski, P., Kulus, M., Jankowski, M., Dompe, C., Bryl, R., Petitte, J. N., et al. (2021). COVID-19 pandemic is a call to search for alternative protein sources as food and feed: a review of possibilities. Nutrients 13:150. doi: 10.3390/nu13010150
Sasu-Boakye, Y., Cederberg, C., and Wirsenius, S. (2014). Localising livestock protein feed production and the impact on land use and greenhouse gas emissions. Animal 8, 1339–1348. doi: 10.1017/S1751731114001293
Sauvant, D., Perez, J. M., and Tran, G. (Eds.) (2004). Tables of composition and nutritional value of feed materials: pigs, poultry, cattle, sheep, goats, rabbits, horses and fish. Paris, France: Wageningen Academic Publishers.
Schiffling, S., and Valantasis Kanellos, N. (2022). Five essential commodities that will be hit by war in Ukraine The Conversation Available at: https://researchonline.ljmu.ac.uk/id/eprint/16422.
Semper-Pascual, A., Decarre, J., Baumann, M., Busso, J. M., Camino, M., Gómez-Valencia, B., et al. (2019). Biodiversity loss in deforestation frontiers: linking occupancy modelling and physiological stress indicators to understand local extinctions. Biol. Conserv. 236, 281–288. doi: 10.1016/j.biocon.2019.05.050
Shah, F., and Wu, W. (2019). Soil and crop management strategies to ensure higher crop productivity within sustainable environments. Sustainability 11:1485. doi: 10.3390/su11051485
Shurson, G. C. (2020). “What a waste”—can we improve sustainability of food animal production systems by recycling food waste streams into animal feed in an era of health, climate, and economic crises? Sustainability 12:7071. doi: 10.3390/su12177071
Smetana, S., Spykman, R., and Heinz, V. (2021). Environmental aspects of insect mass production. J. Insects Food Feed 7, 553–571. doi: 10.3920/JIFF2020.0116
Smith, M., Love, D. C., Rochman, C. M., and Neff, R. A. (2018). Microplastics in seafood and the implications for human health. Curr. Environ. Health Rep. 5, 375–386. doi: 10.1007/s40572-018-0206-z
Song, X. P., Hansen, M. C., Potapov, P., Adusei, B., Pickering, J., Adami, M., et al. (2021). Massive soybean expansion in South America since 2000 and implications for conservation. Nat. Sustain. 4, 784–792. doi: 10.1038/s41893-021-00729-z
Sońta, M., Rekiel, A., and Batorska, M. (2019). Use of duckweed (Lemna L.) in sustainable livestock production and aquaculture–a review. Ann. Anim. Sci. 19, 257–271. doi: 10.2478/aoas-2018-0048
Sońta, M., Rekiel, A., Więcek, J., Batorska, M., and Puppel, K. (2021). Alternative protein sources vs. GM soybean meal as feedstuff for pigs—meat quality and health-promoting indicators. Animals 11:177. doi: 10.3390/ani11010177
Stévant, P., Rebours, C., and Chapman, A. (2017). Seaweed aquaculture in Norway: recent industrial developments and future perspectives. Aquac. Int. 25, 1373–1390. doi: 10.1007/s10499-017-0120-7
Swain, B. B., and Teufel, N. (2017). The impact of urbanisation on crop–livestock farming system: a comparative case study of India and Bangladesh. J. Soc. Econ. Dev. 19, 161–180. doi: 10.1007/s40847-017-0038-y
Taelman, S. E., De Meester, S., Van Dijk, W., Da Silva, V., and Dewulf, J. (2015). Environmental sustainability analysis of a protein-rich livestock feed ingredient in the Netherlands: microalgae production versus soybean import. Resour. Conserv. Recycl. 101, 61–72. doi: 10.1016/j.resconrec.2015.05.013
Taghizadeh-Hesary, F., Rasoulinezhad, E., and Yoshino, N. (2019). Energy and food security: linkages through price volatility. Energy Policy 128, 796–806. doi: 10.1016/j.enpol.2018.12.043
Tallentire, C. W., Edwards, S. A., Van Limbergen, T., and Kyriazakis, I. (2019). The challenge of incorporating animal welfare in a social life cycle assessment model of European chicken production. Int. J. Life Cycle Assess. 24, 1093–1104. doi: 10.1007/s11367-018-1565-2
Testa, M., Stillo, M., Maffei, G., Andriolo, V., Gardois, P., and Zotti, C. M. (2017). Ugly but tasty: a systematic review of possible human and animal health risks related to entomophagy. Crit. Rev. Food Sci. Nutr. 57, 3747–3759. doi: 10.1080/10408398.2016.1162766
Tirado, M. C., Clarke, R., Jaykus, L. A., McQuatters-Gollop, A., and Frank, J. M. (2010). Climate change and food safety: a review. Food Res. Int. 43, 1745–1765. doi: 10.1016/j.foodres.2010.07.003
Trabue, S. L., Kerr, B. J., Scoggin, K. D., Andersen, D., and Van Weelden, M. (2021). Swine diets impact manure characteristics and gas emissions: part II protein source. Sci. Total Environ. 763:144207. doi: 10.1016/j.scitotenv.2020.144207
United Nations Department of Economic and Social Affairs Sustainable Development. (2022). The 17 sustainable development goals. Available at: https://sdgs.un.org/goals (Accessed February 10, 2022).
United States Department of Agriculture, Foreign Agricultural Service. (2022). Grain and feed update. Available at: https://www.fas.usda.gov/ (Accessed March 1, 2022).
US Soybean Export Council. (2018). U.S. soy sustainability assurance protocol. Available at https://ussec.org/ (Accessed May 20, 2022).
van den Burg, S. W., van Duijn, A. P., Bartelings, H., van Krimpen, M. M., and Poelman, M. (2016). The economic feasibility of seaweed production in the North Sea. Aquacult. Econ. Manag. 20, 235–252. doi: 10.1080/13657305.2016.1177859
van Hal, O., Weijenberg, A. A. A., De Boer, I. J. M., and Van Zanten, H. H. E. (2019). Accounting for feed-food competition in environmental impact assessment: towards a resource efficient food-system. J. Clean. Prod. 240:118241. doi: 10.1016/j.jclepro.2019.118241
van Huis, A., and Oonincx, D. G. (2017). The environmental sustainability of insects as food and feed. A review. Agron. Sustain. Dev. 37, 1–14. doi: 10.1007/s13593-017-0452-8
van Zanten, H. H., Mollenhorst, H., Oonincx, D. G., Bikker, P., Meerburg, B. G., and de Boer, I. J. (2015). From environmental nuisance to environmental opportunity: housefly larvae convert waste to livestock feed. J. Clean. Prod. 102, 362–369. doi: 10.1016/j.jclepro.2015.04.106
Verbeke, W., Spranghers, T., De Clercq, P., De Smet, S., Sas, B., and Eeckhout, M. (2015). Insects in animal feed: acceptance and its determinants among farmers, agriculture sector stakeholders and citizens. Anim. Feed Sci. Technol. 204, 72–87. doi: 10.1016/j.anifeedsci.2015.04.001
Winkler, K., Fuchs, R., Rounsevell, M., and Herold, M. (2021). Global land use changes are four times greater than previously estimated. Nat. Commun. 12, 2501–2510. doi: 10.1038/s41467-021-22702-2
Woodgate, S. L., and Wilkinson, R. G. (2021). The role of rendering in relation to the bovine spongiform encephalopathy epidemic, the development of EU animal by-product legislation and the reintroduction of rendered products into animal feeds. Ann. Appl. Biol. 178, 430–441. doi: 10.1111/aab.12676
Yao, G., Zhang, X., Davidson, E. A., and Taheripour, F. (2021). The increasing global environmental consequences of a weakening US–China crop trade relationship. Nat. Food 2, 578–586. doi: 10.1038/s43016-021-00338-1
Yu, C., Miao, R., and Khanna, M. (2021). Maladaptation of US corn and soybeans to a changing climate. Sci. Rep. 11, 1–12. doi: 10.1038/s41598-021-91192-5
Zheng, Y., Jin, R., Zhang, X., Wang, Q., and Wu, J. (2019). The considerable environmental benefits of seaweed aquaculture in China. Stoch. Env. Res. Risk A. 33, 1203–1221. doi: 10.1007/s00477-019-01685-z
Keywords: alternative protein sources, cellular agriculture, circular agriculture, environmental impact, food policy, food safety, soya production, sustainable development
Citation: Pexas G, Doherty B and Kyriazakis I (2023) The future of protein sources in livestock feeds: implications for sustainability and food safety. Front. Sustain. Food Syst. 7:1188467. doi: 10.3389/fsufs.2023.1188467
Received: 17 March 2023; Accepted: 30 August 2023;
Published: 13 September 2023.
Edited by:
S. Mondal, National Institute of Animal Nutrition and Physiology (ICAR), IndiaReviewed by:
Doriana Eurosia Angela Tedesco, University of Milan, ItalyCopyright © 2023 Pexas, Doherty and Kyriazakis. This is an open-access article distributed under the terms of the Creative Commons Attribution License (CC BY). The use, distribution or reproduction in other forums is permitted, provided the original author(s) and the copyright owner(s) are credited and that the original publication in this journal is cited, in accordance with accepted academic practice. No use, distribution or reproduction is permitted which does not comply with these terms.
*Correspondence: Georgios Pexas, Zy5wZXhhc0ByZWFkaW5nLmFjLnVr
Disclaimer: All claims expressed in this article are solely those of the authors and do not necessarily represent those of their affiliated organizations, or those of the publisher, the editors and the reviewers. Any product that may be evaluated in this article or claim that may be made by its manufacturer is not guaranteed or endorsed by the publisher.
Research integrity at Frontiers
Learn more about the work of our research integrity team to safeguard the quality of each article we publish.