- 1Natural Resources Assessment Section, Washington State Department of Agriculture, Olympia, WA, United States
- 2Natural Resources Defense Council, Washington DC, United States
- 3Center for Sustaining Agriculture and Natural Resources, Washington State University, Wenatchee, WA, United States
The advent of “natural climate solutions” and “climate smart agriculture” has increased interest in managing agricultural lands to sequester soil carbon and mitigate climate change. This has led to enormous opportunities for soil scientists and growers alike, as new soil carbon initiatives are created by public, private, and philanthropic entities. It has also led to confusion over what is possible or practical to achieve through agricultural management, as soil carbon formation and storage is complex, and its response to management is context-dependent. This can pose challenges to decision makers tasked with creating defensible, science-informed policies and programs for building and protecting soil carbon. Here we summarize the science concerning the potential for agricultural soils to serve as a natural climate solution, in order to frame a discussion of current approaches in United States (US) policy and practice. We examine existing strategies such as soil health initiatives and direct incentive payments, as well as emerging schemes such as carbon markets and crop insurance reform. We suggest future directions for each strategy, and make recommendations for synthesizing approaches into a cohesive US policy portfolio. Guiding principles for this discussion include the notions that (i) climate change adaptation must be prioritized alongside climate change mitigation; (ii) soil carbon sequestration must be paired with greenhouse gas emission reductions; (iii) structural issues and barriers to adoption must be addressed as part of all policies and programs; (iv) practice- and place-specific programs must be administered in lieu of one-size-fits-all prescriptions; and (v) soil carbon science is not yet sufficiently advanced for the accounting and contractual frameworks proposed in cap-and-trade or regulatory approaches.
1. Introduction
Soil carbon was historically the subject of niche curiosity, with soil scientists and agronomists alone studying its accumulation and persistence, while progressive growers experimented in their fields (Feller and Bernoux, 2008). The urgency for society to mitigate climate change, however, has sparked intensive interest in managing agricultural land to maximize soil carbon sequestration. With the recent popularization of “regenerative farming” and “climate-smart agriculture,” gone are the days in which soil carbon belongs exclusively in the sphere of scientists and farmers (Amundson, 2022). Popular interest in soil carbon is evidenced in star-studded documentaries such as Kiss the Ground, or public outreach campaigns such as Chefs for Healthy Soil. Political and entrepreneurial interest is also clear in the enormous investments that governments, businesses, and nonprofits are making (Kreibich and Hermwille, 2021; Marston, 2022). For decision makers who have not spent their careers studying the minutiae of soil carbon, recent activity may beg the questions: What is soil carbon? Why is it so important? How can I design defensible, science-informed soil carbon policies and programs?
Here we seek to answer these questions for United States (US) practitioners by synthesizing the science, examining current US policy approaches, and outlining future directions. Drawing on the extensive scientific and policy literature on soil carbon, soil health, and natural climate solutions, 10 contemporary US strategies are analyzed in terms of their strengths and limitations. We address limitations by presenting actionable opportunities and highlighting successful programs throughout the US. We conclude by recommending guiding principles intended to build soil carbon and protect soils equitably, responsibly, and in perpetuity. These efforts are used to underscore that soils provide numerous benefits, which are essential for both climate change mitigation and climate change adaptation. The overall aim is to provide actionable direction for increasing soil carbon storage, while simultaneously encouraging a more comprehensive and holistic approach to soils in policy and practice.
2. Review methodology
Google Scholar was searched using keywords soil carbon OR natural climate solutions AND policy OR soil health, practice, economics, incentives, behavior, crop insurance, modeling, technical assistance, and regulation. Care was taken to include a representative sample of relevant works, with an emphasis on review papers, recent publications, and studies which present divergent perspectives on current controversies. The list of studies included is not exhaustive. The purpose of this narrative review is not to provide a quantitative or systematic assessment, but rather to survey recent and critical literature on this timely topic, and to broaden the contemporary discussion of soils beyond carbon. As such, a broad selection of publications was included which contribute to the overall objectives of encouraging a comprehensive approach to soil conservation in US policy and practice, and to highlight future opportunities. Likewise, the US programs and projects we describe are not exhaustive, but instead selectively presented to provide concrete and replicable examples.
3. A synthesis of the science
3.1. What is soil carbon?
Collectively, the world’s soils hold over three times more carbon than the atmosphere, and nearly double the carbon than in all terrestrial vegetation combined (Oelkers and Cole, 2008; Scharlemann et al., 2014). While soil carbon exists in myriad and diverse configurations, it can be broadly grouped into organic and inorganic forms. Soil organic matter (SOM) is the 1–5% of most soils not made up of minerals, air, and water, but is instead composed of animal and plant tissue in various stages of decomposition. SOM is roughly 58% soil organic carbon (SOC). The remaining portion includes other essential plant nutrients such as nitrogen, phosphorous, and sulfur. Soil inorganic carbon– primarily found in arid environments– also represents an important component of soil carbon (Monger et al., 2015). However, it is generally considered more difficult to increase via management and is a smaller soil carbon fraction than SOC. While some strategies can increase inorganic soil carbon (Kantola et al., 2017; Goll et al., 2021), most discussions of management focus on the carbon in SOM.
3.2. How is soil carbon accumulated and stored?
Figure 1 illustrates how atmospheric carbon dioxide (CO2) is converted into SOM through processes driven by plants and microbes (Dynarski et al., 2020; Angst et al., 2021). Plants use CO2 for photosynthesis, converting gaseous carbon to sugars that are stored in plant vegetative bodies, or exuded through plant roots into the soil. Soil microbes use the vegetative carbon in dead plants, leaves, or root exudates—along with dead micro or macro fauna, manure, compost, and other organic materials—as a substrate for metabolism and population growth. Most of the carbon in those organic inputs is converted back into CO2 and released into the atmosphere, while only 3-33% is retained in SOM (Cotrufo and Lavallee, 2022) or microbial bodies (Buckeridge et al., 2022). Over time microbes grow, multiply, and die, leaving behind microbially-processed carbon that can adhere to soil minerals and be protected for variable lengths of time. The biophysical process by which gaseous carbon is drawn down through plants, processed by microbes, and added to soils is called soil carbon sequestration. The amount of carbon sequestered, minus the amount lost, is called soil carbon storage (Jansson et al., 2021).
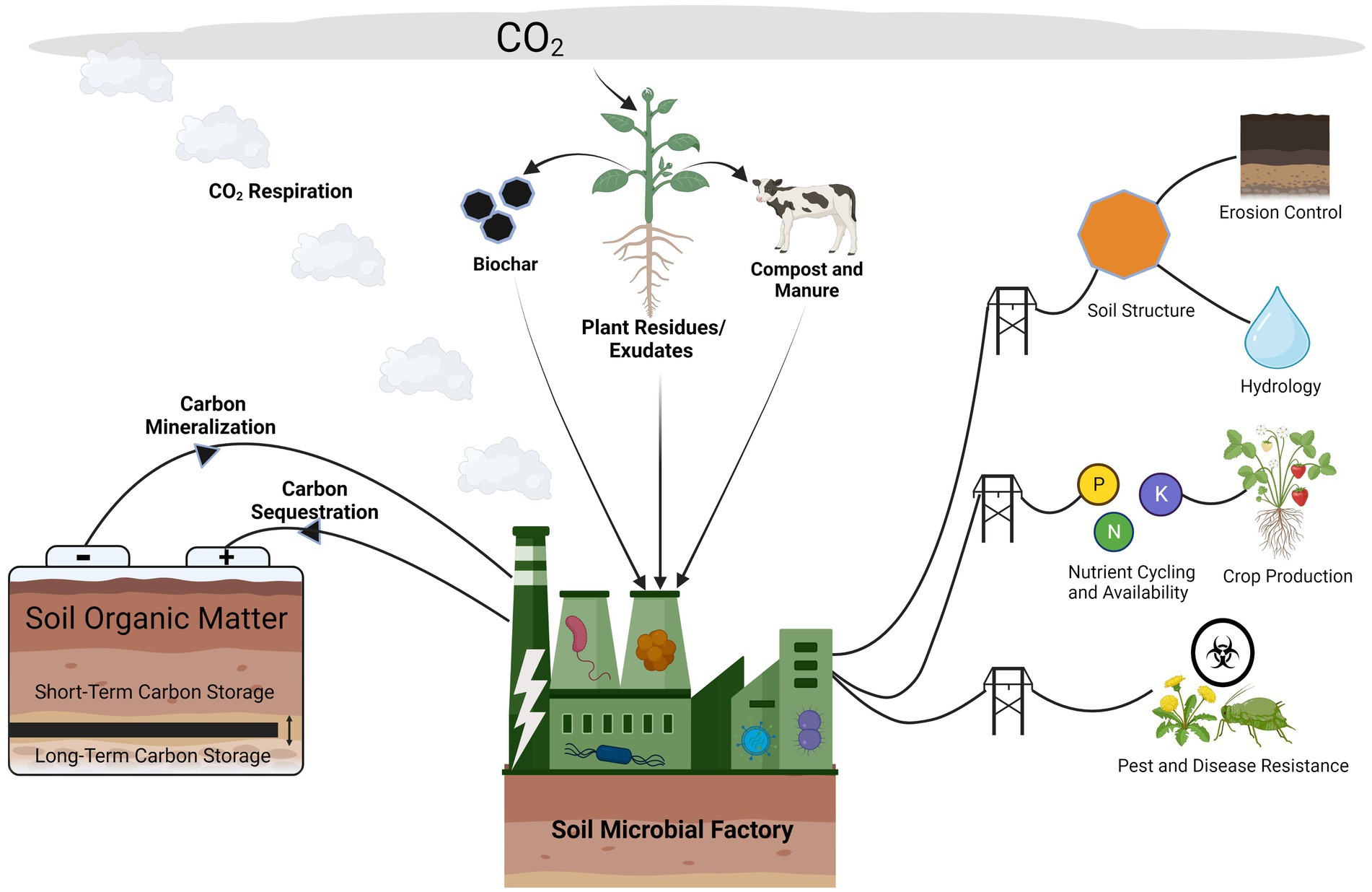
Figure 1. Conceptual diagram detailing how soil organic carbon is both accumulated and utilized by soil microbes to power a broad suite of ecosystem services.
The uniquely complex processes of soil carbon accumulation and storage have been well described in the scientific literature for decades. For more technical summaries, see Lal (2004), Janzen (2006), Miltner et al. (2012), Crowther et al. (2016), Lavallee et al. (2020), Angst et al. (2021), Feeney et al. (2022), Patoine et al. (2022), and Derrien et al. (2023).
3.3. Why is soil carbon so important for climate change mitigation and adaptation?
Increases in soil carbon are associated with numerous potential benefits, one of which is climate change mitigation (Bradford et al., 2019; Vermeulen et al., 2019; Amundson et al., 2022). Immediately halting the loss of existing soil carbon also has a climate impact, by stopping the continued release of CO2 into the atmosphere. Moreover, building and preserving soil carbon can promote the myriad benefits that SOM provides (Figure 1), and is key to helping growers and society adapt to climate change and reduce land use conversion (Kopittke et al., 2022).
Soil organic matter benefits are context-specific, but can include increased fertility and nutrient use efficiency (Tiessen et al., 1994), with the potential to decrease dependence on greenhouse gas (GHG) intensive fertilizers (Crippa et al., 2021) or increase crop yield per unit of land (frequently called sustainable intensification; Pretty and Bharucha, 2014). SOM can also improve soil structure (Oades, 1984), and therefore increase resistance to wind and water erosion (Barthès and Roose, 2002) and improve air and water quality (Fageria, 2012); improve soil water dynamics including infiltration, filtration, and water holding capacity (Emerson, 1995; Blanco-Canqui et al., 2013); and support soil biodiversity which can drive several vital functions such as residue decomposition, carbon and nitrogen cycling, and disease resistance (Schlatter et al., 2017; Delgado-Baquerizo et al., 2020). Independent of soil carbon or SOM, broadly protecting soils preserves wildlife habitat, recreation sites, cultural heritage, archeological records, and rural livelihoods.
3.4. How does agricultural management impact soil carbon?
Because agriculture is often referred to as a “natural climate solution,” a pervasive notion has emerged that climate change can be reversed by changing “bad” farming practices to “good” farming practices (e.g., conventional tillage to no-till). This notion overlooks the fact that carbon has substantially decreased in the vast majority of soils converted from unmanaged to agricultural land (Guo and Gifford, 2002; Scharlemann et al., 2014; Sanderman et al., 2017). Soils are indeed an important soil carbon store, and naturally low-carbon soils may sustain increases as the result of agricultural management (Sanderman et al., 2017). However, agriculture necessarily and inherently exploits soil carbon for crop production. Reversing climate change purely via soil carbon sequestration is not a feasible goal. Instead, it is feasible to regenerate soil carbon in many conditions, and to immediately halt the further loss of this invaluable carbon store.
Agricultural activities can diminish soil carbon stocks by reducing carbon inputs, and by increasing pathways for loss. Modern agriculture exports more carbon than it sequesters, through crop genetics (e.g., varieties that promote the growth of harvested grain or fruits rather than roots and root exudates; Jansson et al., 2010, 2021), and through management (e.g., removing crop residues rather than returning them to the field; Stella et al., 2019). Microbial processing, or mineralization, of SOM is necessary to supply valuable nutrients to crops. However, this process converts soil carbon back into CO2. The very same microbes responsible for building soil carbon must also deplete it to survive and to support plant growth, in an ongoing cycle of microbial and soil carbon turnover (Figure 1; Dynarski et al., 2020). SOM mineralization in the face of reduced carbon inputs diminishes soil carbon stocks, which can be further compounded by management: Mechanical tillage exposes once protected carbon to oxidation, mineralization, and erosion (Huggins and Reganold, 2008; Chowaniak et al., 2020; Yu et al., 2020); the burning of crop residues can destroy SOM (Collins et al., 1992); irrigation can result in soluble carbon leaching through the soil (Moore, 1997; McTiernan et al., 2001; Ruark et al., 2009; Shang et al., 2018; Sagar and Singh, 2020); and soil not held in place by ground cover or living roots, such as in fallowed fields, can be lost through runoff or erosion, carrying carbon with it (Sharratt et al., 2018).
Agricultural management can also increase soil carbon, or be tailored to protect existing carbon stocks. Terms like “regenerative farming” and “climate-smart agriculture” are frequently used to define a suite of practices aimed at optimizing crop production while protecting and building SOM. Practices include reduced tillage, reduced fallowing, cover cropping, livestock integration, adding carbon-based amendments (e.g., manure, compost, crop residues, or biochar), breeding crop varieties that produce more roots and root exudates, conservation crop rotation, agroforestry, and retiring marginal lands from production.
While these practices can deliver many on-farm benefits, they are not one-size-fits-all solutions for increasing soil carbon. For example, conservation tillage has been observed to increase (Bai et al., 2019; Ogle et al., 2019), decrease (Ogle et al., 2012), and have no effect on (Luo et al., 2010) soil carbon. In fact, one meta-analysis determined that conservation tillage reduced crop yield by an average of 5.1% across all crops and conditions evaluated (Pittelkow et al., 2015). Reduced crop yields may require more land be converted to agricultural production, which results in a net soil carbon loss overall (Guo and Gifford, 2002; Sanderman et al., 2017). The impact of cover cropping on soil carbon has also been observed to be condition-specific, with greater effects in fine-textured soils and when a legume is present in the cover crop species mix (Jian et al., 2020).
Further complicating carbon sequestration potential is that other essential nutrients (e.g., nitrogen, phosphorous, and sulfur) are required for the conversion of carbon inputs into SOM. This elemental balance, or stoichiometry, may even dictate whether carbon is respired as CO2 or transformed into microbial cells. Therefore, practices that literally add carbon to the soil do not necessarily build SOM or achieve carbon sequestration, if other necessary inputs are not also present (Schlesinger, 2022). Additionally, potential increases in soil carbon are not infinite, with many soils having a natural equilibrium or saturation point, after which gains as the result of management can plateau (Stewart et al., 2008).
While the potential for management to increase soil carbon is limited by environmental factors such as soil texture, nutrient content, and climate, it is also limited by social factors such as technical assistance availability, crop prices, and farmer culture. Management decisions, which are themselves the product of complex cultural and socioeconomic factors, play a significant role in balancing the tradeoffs between crop production and ecosystem services (Carlisle, 2016; Teixeira et al., 2018; Wade et al., 2021). These facts underscore the need for place-based research that considers not only the soil and climate context, but what unique barriers a grower may face in a particular region or cropping system. They also underscore the need to measure multiple outcomes including water and nutrient cycling and filtration, biodiversity support, crop production, farmer innovation and attitudes, climate change mitigation, and negative externalities.
3.5. Why is it so difficult to account for soil carbon?
There are many challenges in measuring soil carbon, estimating how long it will last, and quantifying increases that result from altered management (Chenu et al., 2019; Stanley et al., 2023). This is due to soil heterogeneity, the costs of comprehensive sampling, and the uncertainty associated with laboratory analysis methods and the use of models.
Soils accumulate and store carbon differently based on texture, depth, mineralogy, and climate, even within a single field (Wiesmeier et al., 2019; Basile-Doelsch et al., 2020). Taking sufficient samples to account for variability can be prohibitively labor- and cost-intensive. For example, it is common to sample only from the soil surface, despite the sizeable carbon stocks that may exist deeper in the soil profile (Gross and Harrison, 2019). This can lead to erroneous conclusions that carbon is being lost or gained as the result of management, when it has actually been vertically redistributed (Baker et al., 2007; Gál et al., 2007). In addition, soil carbon can be seasonally variable, with measurements differing by when in the year a sample is taken (Wuest, 2014).
There is also heterogeneity in analytical methods, which can lead to inconsistent results and interpretations among laboratories and statisticians (Wade et al., 2020; Crookston et al., 2021; Slessarev et al., 2023). Some methods can describe how much carbon is in the soil while providing little insight on how long it will be last. This is because soil carbon is stored in many forms, some of which are more protected from degradation than others (Lavallee et al., 2020). A single measurement may suggest a high carbon content, even if the carbon is mostly in plant residues and will soon be respired as CO2. This also raises the issue of non-permanence, in which gains in soil carbon can be measured, but then quickly reversed through management changes like an increase in tillage or fallowing (Smith, 2005; Dynarski et al., 2020). There are an increasing number of laboratory tests aimed at determining the quantity and stability of carbon stored in multiple forms (Stott, 2019). However, these tests can be resource-intensive to measure, and their correct interpretation is still in question.
Fortunately there have been a number of scientific and technological advances in soil carbon measurement and estimation, which is necessary for effective management and policy formulation (Paustian et al., 2019; Smith et al., 2020). These include the application of pedometrics– the branch of soil science which relies on statistical, mathematical, and big data applications– in soil carbon estimation (Finke, 2012); more accurate models due to the inclusion of machine learning (Keskin et al., 2019; Nguyen, 2021) or multimodel ensembles (Wallach et al., 2018); new high throughput and cost-effective measurement techniques such as spectroscopy (Ball et al., 2020; Barthès and Chotte, 2021) or remote sensing (Thaler et al., 2019); and increased efforts among practitioners to standardize soil databases across projects and regions (Norris et al., 2020).
3.6. Should our policies focus on soil carbon alone?
An exclusive policy focus on soil carbon for climate change mitigation devalues SOM co-benefits as incidental byproducts (Figure 2). In reality, these benefits are essential for adaptation to and resilience through current and future climate conditions. The sole focus on carbon also overlooks nitrogen’s contribution to climate change, with nitrous oxide having nearly 300 times the impact on global warming as CO2 (Forster et al., 2021). Reducing nitrous oxide emissions from the use of fertilizer and manure via precision agriculture or variable rate technology can play a major role in climate change mitigation, alongside sequestering soil carbon through cover cropping or reduced tillage (Skiba and Rees, 2014; Winiwarter and Mohankumar, 2015; Schulte-Uebbing et al., 2022). Policies and programs that protect soil and improve soil management– independent of the direct impact on soil carbon– are more likely to promote ecosystem services while simultaneously reducing GHGs.
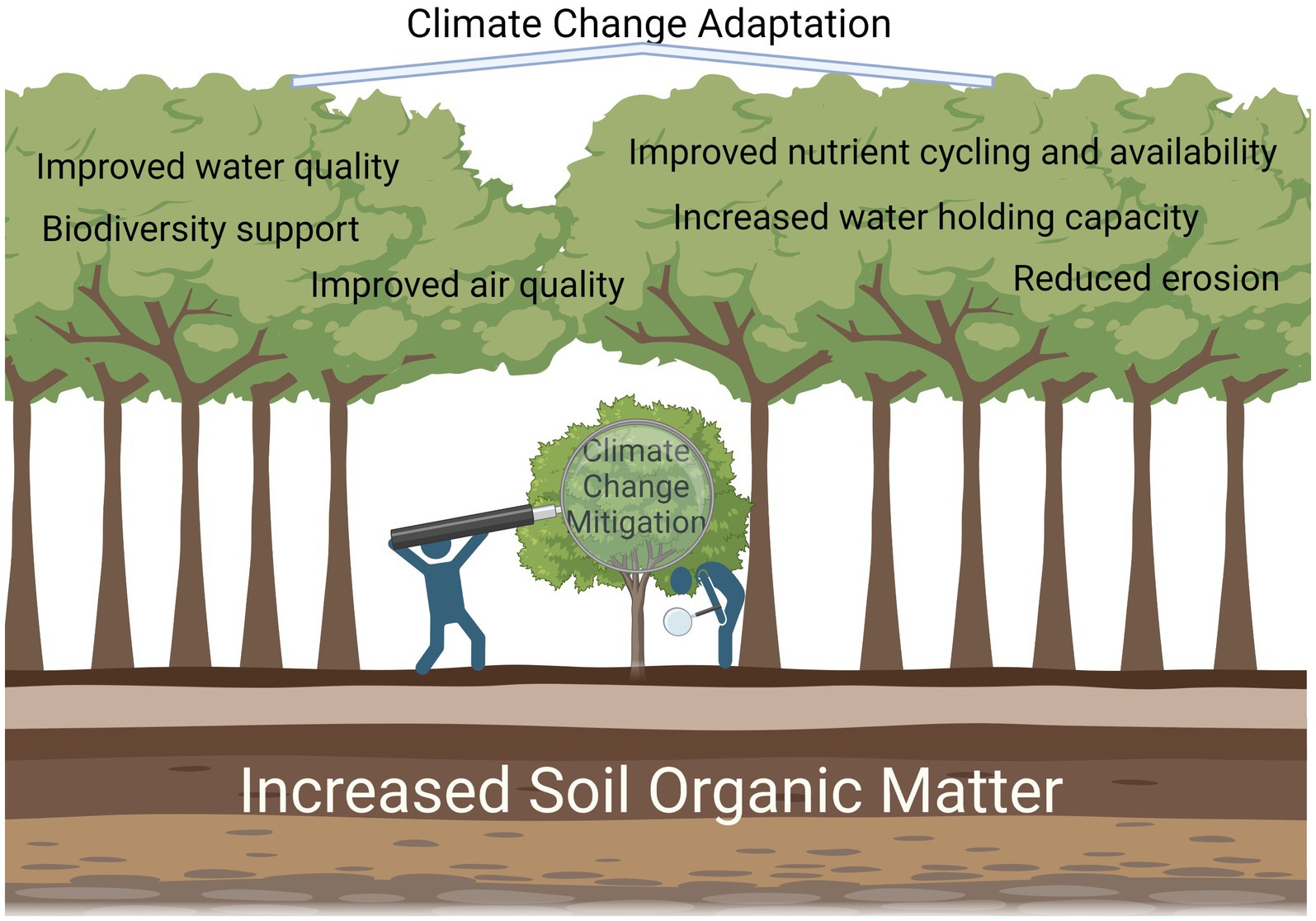
Figure 2. Missing the forest for the trees. This conceptual diagram illustrates how policies with a sole focus on increasing soil carbon for climate change mitigation may miss opportunities to promote other ecosystem services. Improving agricultural management can improve the capacity for growers and society to adapt to climate change, even in cases where it does not mitigate climate change.
4. Current approaches in policy and practice
While soil carbon is a relatively new policy domain, broadly protecting soils has long had its place in the US (United States Department of Agriculture, n.d.). Laws include the National Industrial Recovery Act of 1933, which created the Soil Erosion Service; the Soil Conservation Act of 1935, which created the Soil Conservation Service [known as the Natural Resources Conservation Service (NRCS) since 1994]; the Soil and Water Resources Conservation Act of 1977; and the Food Security Act of 1985. Additional soil-specific programs have been created through US Farm Bills, including the Conservation Reserve Program in 1985 and the Wetland Reserve Program in 1990. There is evidence that many of these laws resulted in increased soil carbon (Gebhart et al., 1994; Barker et al., 1996), though this was incidental to their primary goals of erosion prevention and resource conservation.
In contemporary lawmaking, increasing soil carbon is more explicitly at the center of policies and programs. Here we present current US approaches in terms of their strengths, limitations, and future opportunities. This analysis is informed by the state of knowledge of and limitations in soil carbon science and practice adoption sociology discussed in Section 3. It is presented within an overarching framework in which science should inform policy, which should support practice, which in turn should improve science. The objective is to aid decision makers in developing a science-informed policy portfolio that incorporates multiple complimentary approaches, and can be successfully integrated into this framework.
4.1. Soil health initiatives
Perhaps the most high-profile US strategy for protecting and increasing soil carbon is the creation of state-specific soil health initiatives. By 2021, twenty states formalized soil health initiatives through resolutions and laws, with an additional twenty signaling interest through related policy activity (State Healthy Soil Policy Map, 2021). Initiatives vary greatly in their level of funding, focus on stakeholder engagement, and projects in their portfolios.
4.1.1. Strengths
Because soil health is focused broadly on the soil’s capacity to provide multiple functions (Janzen et al., 2021), soil health initiatives can provide a flexible policy approach to reach beyond carbon sequestration. This is especially meaningful as the potential for agronomic co-benefits is more likely to motivate farmer adoption of conservation practices than the promise of payments for increased soil carbon (Buck and Palumbo-Compton, 2022). The flexibility of soil health initiatives allows programs to be tailored to the needs of specific communities (Warner and Watnick, 2021). Soil health initiatives provide a unifying entity for many other strategies for increasing soil carbon, which can be added to over time with increased funding and engagement.
4.1.2. Limitations
The presence of a soil health initiative can signal that action is being taken, even when sufficient levels of funding and engagement are not present. It can therefore have a “greenwashing” effect that reduces the pressure for more immediate action, such as GHG emissions reduction across all sectors (Seddon et al., 2021). There are also challenges in defining and quantifying substantive outcomes of “soil health” (Lehmann et al., 2020; Baveye, 2021a,b; Janzen et al., 2021; Powlson, 2021).
4.1.3. Opportunities
The creation of a federal soil health initiative coalition could address capacity differences across the US by facilitating knowledge exchange and the development of region-specific toolkits, best management practices, datasets, and soil carbon models. Cohesive materials and templates could be created for customizable soil health economic studies, survey approaches, data management strategies, and project monitoring and evaluation, among other topics. Furthermore, verified and peer reviewed toolkits for soil health science (e.g., Git repositories containing code for GIS, web, or extension products, and statistical models for project evaluation or climate modeling) could be aggregated and made public. The impact and widespread reliance on USDA tools such as COMET, SSURGO, and conservation technical guides illustrates the potential for central coordination to effectively advance the quality of soil health initiatives (Amundson, 2020). Such efforts are currently underway by groups such as the National Healthy Soils Policy Network, Carbon180, and American Farmland Trust.
4.2. Direct incentive payments
Many programs provide growers with grants, financial incentives, and cost share to alter agricultural management. The NRCS was first authorized to provide funding through the Environmental Quality Incentives Program (EQIP) in 1996, and has since distributed over $15 billion to help growers implement conservation practices (United States Department of Agriculture, 2022). Incentive payments can also come from local sources such as the Iowa or Illinois Soil and Water Conservation District Cost Share Programs, or state departments of agriculture as in the California, Maryland, or New Mexico Healthy Soils Programs. Corporations seeking to improve their sustainability portfolio or achieve a net-zero supply chain can also offer direct payments to growers (Marston, 2022).
4.2.1. Strengths
Offering financial assistance lowers the barriers to entry for growers to implement conservation practices (Piñeiro et al., 2020). It reduces the risk a grower may experience in experimenting with new practices, and has the potential to mitigate financial losses during transition periods. Financial incentives redistribute the cost of conservation from the grower to the public, who will also reap the benefits of improved air and water quality, carbon sequestration, and food security.
4.2.2. Limitations
One-time or short-term financial incentives do not address structural issues such as knowledge gaps, access to equipment, regional climate challenges, or cultural barriers. As such, there is the potential for growers to revert to “business as usual” practices once the grant period is complete (Wallander et al., 2021). Furthermore, resource limitations mean that not all who apply for funding will receive it. For example, only 30% of applicants for NRCS EQIP receive funding, amidst widespread inequities in how funds are distributed across regions, farm size, and demographic groups (Happ, 2021). Incentive programs frequently exclude early adopters, as funding is typically awarded to growers to implement a new practice rather than to sustain one. Additionally, while practices like cover cropping and compost amendment are eligible for funding in many programs, other emerging or experimental practices are not. This can hinder innovation and the development of new knowledge. Finally, contemporary US incentive programs largely reward the implementation of practices rather than the delivery of outcomes. Due to the heterogenous impact of agricultural management on soil carbon, incentive payments cannot uniformly lead to increased soil carbon storage.
4.2.3. Opportunities
Increased funding from bills such as the US Inflation Reduction Act can alleviate resource limitations, though may not address structural limitations. Investment in the underlying social and technical infrastructure is also required. Simultaneous investments should be made in research, technical assistance, and market development, as a multi-pronged approach can address structural challenges and extend conservation efforts beyond short-term funding cycles (Bell et al., 2023). Furthermore, funding should be directed towards incentives for emerging and experimental practices. This could reward innovative growers and improve the current state of knowledge. Programs like the USDA AgARDA provide a model that could be adapted for soil carbon research and practice implementation. Despite the drawbacks of practice-based rather than outcomes-based rewards (Weinberg and Claassen, 2006; Bartkowski, 2021), this may be the most feasible policy option pending further scientific advances (Jeffery and Verheijen, 2020). Ideally, practice-based incentive programs would incorporate research partners to advance site-specific soil carbon science, and to ensure that conservation practices are having the desired effect.
4.3. Carbon markets
The search for market-based incentives has led to the incorporation of soils in carbon markets, wherein participants can “offset” or “trade” GHG emissions in one sector or geography by increasing soil carbon elsewhere (Croft et al., 2021; Oldfield et al., 2021). Examples include companies that pay a grower to increase soil carbon via cover cropping, in exchange for maintaining or increasing GHG emissions at their factory. The inclusion of soil carbon offsets in carbon markets is controversial, with both supporters and detractors (Vermeulen et al., 2019; Bossio et al., 2020; Kreibich and Hermwille, 2021; National Sustainable Agriculture Coalition, 2021; Zelikova et al., 2021).
4.3.1. Strengths
Carbon markets are an innovative iteration of market-based incentives, and may be cost-effective compared to strategies such as direct payments (OECD, 2013). In a properly functioning market, growers can diversify their revenue stream while businesses offset emissions that are otherwise difficult to curb. Continued market valuation could incentivize the sustained use of conservation practices. Furthermore, soil carbon offsets have already proved a driving force of innovation, as evidenced by the enormous investment in soil carbon research and quantification technologies, and the emerging markets for other ecosystem services (Reed, 2020).
4.3.2. Limitations
Poor quality standards for carbon trading can lead to a net increase in GHG emissions, if offset purchasers increase their emissions in exchange for soil carbon sequestration that may not be achieved, is difficult to verify, or is reversible. Highlighting this, a recent review ranked 17 protocols used in soil carbon offsets by their rigor, additionality, durability, and grower safeguards (Zelikova et al., 2021). Eight protocols, or nearly 50%, scored only 1 out of 5. Poor quality standards can erode trust between the public or grower communities, and scientists, governments, or NGOs, as observed during the 2010 collapse of the Chicago Climate Exchange (Gosnell et al., 2011). The continued emission of GHGs and other co-pollutants can have serious consequences for the environment, and for the socially or economically disadvantaged communities most likely to live near sources of fossil fuel pollution (Silva and Zhu, 2009; Cushing et al., 2018; Perera and Nadeau, 2022). Furthermore, carbon is frequently priced so low that markets fail to provide sufficient incentives for growers, act as a deterrent for emitters, or allow small operations and lessees to participate (Lundgren et al., 2015; Ervine, 2018; Wongpiyabovorn et al., 2022). Carbon markets also exclude early adopters, and may contribute to a siloed approach to soil protection that focuses only on CO2 drawdown.
4.3.3. Opportunities
Soil carbon is dynamic and heterogenous, its permanence is context-specific, and the science of how to build and measure it is evolving. As such, we suggest that soil carbon is not yet robustly quantifiable enough for contractual emissions trading. Soil carbon offsets have indeed been excluded in state-sponsored “cap and trade” carbon markets, such as in California and Washington. There, soil carbon is not traded in the market, but rather invested in by directing revenue from the sale of GHG emission allowances towards projects that can increase soil carbon. This “cap and invest” strategy promotes soil carbon sequestration as part of market-driven climate change mitigation, but does not depend on it. This approach minimizes potential externalities, keeps in place the pressure to reduce GHG emissions from other sectors, and promotes the formation and protection of soil carbon stocks.
4.4. Research
Research programs address uncertainty related to the impact of management on soil carbon by investigating region- and crop-specific contexts. Programs can take multiple forms. Long-term experiments investigate the impact of management practices on soil health, carbon sequestration, and farm profitability over time and under a changing climate. The USDA coordinates a network of 18 such sites in the US, while some states, universities, or community groups coordinate their own regional sites or networks. A common alternative approach are survey studies, such as those carried out by Cornell and the New York Soil Health Initiative (Amsili et al., 2020), Ohio State University (Culman et al., 2022), or the Soil Health Institute (Norris et al., 2020). These projects aggregate data from thousands of soil samples from a variety of real-world contexts, and use statistical analysis to link carbon storage potential to texture, climate, or management. Additional approaches include economic, life cycle, and behavioral studies, which can lead to a better understanding of barriers to adoption, environmental tradeoffs, and practice costs and benefits (Karlen et al., 2017; Stevens, 2018; Brown et al., 2021; Wade et al., 2021).
4.4.1. Strengths
Place-based, practice-specific research acknowledges that there is no one-size-fits-all solution, and can lead to science-informed recommendations specific to the climate, soils, and communities of a particular region. Long-term research helps overcome the challenges of soil dynamism and heterogeneity by investigating the impacts of management across time (Riar and Bhullar, 2020). Long-term studies also produce more robust conclusions than those restricted to a 1–5 year grant cycle. Survey studies, on the other hand, are more flexible options, as soil and management data can be obtained from real-world conditions across soil textures, climates, and cropping systems.
4.4.2. Limitations
Research is costly, time consuming, and may take multiple years to produce results. Long-term experiments require land to be set aside in perpetuity, which necessitates extraordinary levels of funding and coordination. Furthermore, these sites are geographically static, and can only make inferences about the soils and climates within their boundaries. Additionally, research plots are frequently smaller than typical production farms and may not represent real-world conditions. This can present obstacles to extrapolating conclusions to larger systems, and to disseminating relatable information to growers (Passioura, 2010). Survey studies address these limitations by investigating soils from actual farms across multiple environmental and social contexts. However, this approach also requires significant coordination and investment, as well as special care to reduce variability and maintain data quality across diverse soil sampling and laboratory practitioners.
4.4.3. Opportunities
Ideally, long-term research would be paired with survey studies and sociological investigations to produce site-specific knowledge and recommendations. Centrally coordinated research can ensure complementary scientific questions and results, cohesive data management and protocols, and effective public dissemination of results. The Washington Soil Health Initiative provides an example of a multi-agency collaboration with several research strategies in its portfolio. A successful research program integrates the needs, perspectives, and expertise of growers and community stakeholders from the onset (Warner and Watnick, 2021), and works to center practical, economic, and human health considerations. All research efforts should be translated into practice through simultaneous investment in technical assistance, direct incentive payments, and market development.
4.5. Model development and improvement efforts
Soil carbon modeling may be a strategy to overcome the challenges of resource-intensive soil sampling campaigns. Models such as Daycent, CropSyst, CQESTR, and COMET-Farm can estimate the carbon sequestration potential of a given practice in a given region, frequently without soil sampling. However, estimation accuracy hinges upon existing sample-based datasets. Therefore, model outputs vary by data quality and availability, as well as by computational differences; the inclusion or exclusion of factors such as future climate, crop type, microbial or mineral influence, plant litter inputs, or soil depth; and whether the user can input site-specific data such as initial measured SOC content (Carey et al., 2016; Crowther et al., 2016; Vereecken et al., 2016; Sulman et al., 2018; van Gestel et al., 2018). Extensive investigation into different models has revealed variable success in making accurate predictions (Vereecken et al., 2016; Sulman et al., 2018). Nevertheless, models can be used to make landscape-scale decisions (Bartkowski et al., 2021), and to enter agricultural operations into carbon markets or direct incentive programs (Oldfield et al., 2021).
4.5.1. Strengths
Accurate modeling is essential for any program aimed at building and protecting soil carbon. Models can provide swift, inexpensive estimates of the impact of management. Because many can be run without site-specific measurements, they spare technical assistance providers and producers from taking labor-intensive soil samples year after year, reducing monitoring and verification costs (Paustian et al., 2019). Models can also be used to compare multiple sites, which aids decision makers in prioritizing certain regions or practices to maximize climate change mitigation impacts with limited funding.
4.5.2. Limitations
Models have variable success in making accurate predictions, due to the dynamism and heterogeneity of soil carbon, and the numerous differences between models and available data (Vereecken et al., 2016; Sulman et al., 2018). Over-reliance on potentially inaccurate estimates can contribute to similar challenges described with carbon markets, including pollution trading and social inequities. Due to finite resources, difficult decisions must be made on whether to fund the development of new models, or instead improve existing models. This is exemplified by the widely used USDA NRCS COMET-Farm model. COMET-Farm has shown mixed ability to accurately estimate soil carbon changes, does not accommodate measured SOC data, and is difficult to parameterize for many crops and regions (Ball et al., 2023). Nevertheless, the USDA endorses its use, and allocates funding to improving COMET-Farm over models which incorporate emerging and promising technologies such as machine learning or multimodel ensembles. While COMET-Farm has limitations, however, its user-friendly interface enables use by practitioners of mixed technical ability (Paustian et al., 2018). This demonstrates a common tradeoff between ease-of-use and estimation accuracy, with simple models accessible to more practitioners, while more data-intensive and accurate models require advanced knowledge and skill to operate.
4.5.3. Opportunities
Increased research dollars from recent US legislation may mitigate the tradeoff between improving existing models or developing new models. Ideally, both could be pursued with a focus on incorporating the latest technologies and improving site-specific estimates. User interfaces and decision-support tools should accompany all models, to increase access for producers, decision makers, and technical assistance providers (Rose et al., 2016). Furthermore, models are only as strong as the datasets they are built from. With central coordination, in-depth literature reviews could be conducted to develop place-based (e.g., watershed, soil type, or contiguous cropping systems as feasible or appropriate) GHG coefficients for each conservation practice. Where literature does not exist, a grant program could be created to address the knowledge gap by funding primary research.
4.6. Technical assistance
Technical assistance, or practical support to growers in the form of resource assessment, project planning and implementation, and monitoring and evaluation, is necessary for translating research into practice (Piñeiro et al., 2020). Technical assistance providers include the NRCS, conservation districts, Cooperative Extension, and agricultural professionals such as Certified Crop or Pest Control Advisors. Technical assistance materials include videos, factsheets, and decision-support toolkits, like those available through the NRCS, Soil Health Nexus, and state soil health initiatives. Resources frequently feature growers who successfully adopted a conservation practice, with accompanying “why and how” materials. Technical support can also be tailored to specific growers or communities. The NRCS provides individualized carbon and conservation plans, whereas Utah Soil Health Program Specialists provide in-field assessments. “Train the trainer” programs like the Washington Conservation Commission’s Center for Technical Development can also provide additional education for existing practitioners. Most importantly, grower peer-to-peer networks including virtual forums, field days, grange hall meetings, and commodity conferences, are an effective provider of technical assistance. It is well established that other growers are a primary source of information for growers (Sutherland and Marchand, 2021). As such, peer-to-peer networks have been formalized by groups such as the National Association of Conservation Districts’ Soil Health Champions Network, or through the Ohio Soil Health Initiative’s Soil Health Ambassador Program.
4.6.1. Strengths
Technical assistance providers lower the barriers to entry for growers to practice conservation by filling knowledge gaps, aggregating relevant resources, and working through place-based challenges such as climate or access to resources (Piñeiro et al., 2020). Furthermore, growers better connected to early adopters are more likely to adopt conservation practices themselves. The mere presence of an early adopter in a given region can increase an entire community’s access to infrastructure, equipment, and knowledge (Carlisle, 2016).
4.6.2. Limitations
Technical assistance infrastructure can be time- and cost-intensive to establish and maintain, as it requires professional expertise, ongoing education, and community relationships established across time (Norton and Alwang, 2020).
4.6.3. Opportunities
Because technical assistance is essential for the success of all other strategies for building and protecting soil carbon, increasing technical assistance availability should be prioritized in all policies and programs. Increased funding and resources should be dedicated to continually training, employing, and equipping technical assistance providers and farmer support networks (Wick et al., 2019). These practitioners would ideally provide generalized toolkits, site-specific consultation, and foster peer-to-peer knowledge exchange, while working to develop long-term relationships built on trust.
4.7. Certification programs
One market-based strategy for promoting soil carbon sequestration adopts the “market segregation” approach, in which crops grown with specific practices are segregated from “business as usual” crops to create– or ensure access to– a market, or to elicit a higher price. The most high profile example is the certified organic program, in which consumers frequently pay a premium for crops grown without synthetic pesticides and fertilizers (Thøgersen et al., 2019). This model is increasingly employed for other farming practices, including those that protect wildlife or employ socially just labor practices. Certification schemes allow growers access to branding materials (e.g., signs and labels, or blockchain technology and smart tagging; Motta et al., 2020; Van Wassenaer et al., 2021) which help them negotiate higher prices with supply chain partners or directly with consumers. Examples with soil-specific components include the Michigan Agriculture Environmental Assurance Program (MAEAP) or Saving Tomorrow’s Agricultural Resources (STAR). Under these schemes, growers voluntarily provide information about practices such as the frequency of their tillage and soil testing, the source of their fertility, or how they manage crop residues. Responses are algorithmically converted into scores, and farms above a certain threshold can participate in branding initiatives with slogans such as “Environmentally Verified.” In the STAR program, the certification scheme is combined with an incentive program in which supply chain partners can provide per acre payments to growers with higher scores.
4.7.1. Strengths
Certification programs can improve the business case for soil health and soil carbon sequestration by generating market valuation for conservation practices. This is likely to lead to more sustained behavior change than incentive payments alone, as economic reward is continuous. A successful certification scheme can reward early adopters, improve farm profitability, allow industry partners to work towards sustainability goals, and provide an avenue for governmental or public interest groups to publicly recognize growers and conduct soil outreach and education.
4.7.2. Limitations
Certification programs can be resource-intensive to operate and are frequently supported by grant funding. Additionally, the popularity of this approach can lead to “certification fatigue,” in which growers choose not to enroll because of the multitude of options available, and the administrative resources required to participate (Stephenson et al., 2022). Like incentive programs, most contemporary certification programs reward the implementation of practices rather than the delivery of outcomes. Because conservation practices do not uniformly lead to soil carbon increases in all contexts, the outcome of increased soil carbon storage is not guaranteed.
4.7.3. Opportunities
Certifications should be paired with incentive programs, as in the case of STAR. An incentive or cost share payment can help initiate conservation, while market-based approaches can help sustain it. To generate a broadly recognized market signal and to minimize certification fatigue, programs could be scaled while remaining regionally customizable. As with incentives, certifications would ideally incorporate research partners to increase understanding of whether conservation practices are having the desired effect.
4.8. Agricultural finance tools
There is increasing recognition that agricultural finance institutions are impacted by—and have a role to play in mitigating—the effects of climate change (World Bank Group, 2016; Gauthier et al., 2022). This is especially timely as climate change increases uncertainty for farmers, and makes risk reduction and financing tools more essential than ever. Despite the necessity of these tools, however, access to capital remains one of the largest barriers farmers face when implementing conservation practices (Ranjan et al., 2019).
4.8.1. Strengths
To respond to these challenges, several innovative financial products have emerged that incentivize long-term stewardship rather than maximum yields. For example, revolving loan programs offer growers low interest long-term loans to access the capital required to implement conservation practices. Examples include Mad Capital and the AGRI3 Fund, a public-private partnership between the United Nations Environment Programme and Rabobank. Climate-smart tax credits can also be made available, as in Pennsylvania where the Resource Enhancement and Protection Program provides state tax credits to producers to implement conservation practices. Crop insurance reform is also underway, as current policies can disincentivize experimenting with new practices (Annan and Schlenker, 2015) or preclude practices such as cover cropping, crop intensification, or crop diversification (Natural Resources Defense Council, 2017). The USDA’s Pandemic Cover Crop Program (PCCP) was recently piloted to reduce insurance premiums by $5 per acre for participating growers that planted cover crops.
4.8.2. Limitations
The climate crisis is in large part due to market-driven resource consumption and growth imperatives (Cook et al., 2016). Incentivizing and valuing conservation practices within this system may hinder wider systemic reform efforts.
4.8.3. Opportunities
Within the current system, climate-smart financial tools and crop insurance programs are essential components to optimizing the climate change mitigation and adaptation potential of agricultural lands. As such, financial institutions, governmental agencies, and NGOs must continue working towards reform. Recommendations from advocacy groups such as the American Farmland Trust and Natural Resources Defense Council include eliminating fallow requirements, phasing out single-crop, yield-based coverage in lieu of whole farm revenue protection, destigmatizing the use of cover crops as a risky practice, and incentivizing the use of best management practices through insurance premium reductions (Natural Resources Defense Council, 2017; Beckie et al., 2019; van der Pol et al., 2021). USDA PCCP funding should be made permanent and expanded to include additional conservation practices, while climate-smart tax credit programs could be also expanded to a federal level. Agricultural finance tools are intended to provide a safety net for growers. This must increasingly include support for climate change adaptation.
4.9. Public outreach and education campaigns
Contemporary soil documentaries, books, websites, campaigns, and conferences are numerous (Amundson, 2022). For example, Chefs for Healthy Soil works through culinary communities to educate eaters about the importance of soil conservation. Soil Your Undies raises awareness about soil biodiversity and the role of soil microbes. Soil Life illustrates basic soil science concepts with simple and beautiful graphics.
4.9.1. Strengths
Public enthusiasm for soil carbon and soil health has been instrumental in garnering the political momentum necessary to allocate funds to diverse policies and programs. This illustrates how vital public outreach and education is to all other strategies for increasing soil carbon.
4.9.2. Limitations
The nuanced role of soils in climate change mitigation does not easily lend itself to slogans and sound bites. As such, catchy public interest campaigns run the risk of oversimplifying the science, overpromising the potential, and creating confusion in what is possible or practical to achieve.
4.9.3. Opportunities
Outreach and education can sustain political interest, generate market valuation, and clarify sources of confusion in soil carbon science. Successful campaigns should aim to accomplish all three.
4.10. Regulation and mandatory compliance
To our knowledge, there are no programs which regulate the formation and preservation of agricultural soil carbon via mandatory compliance. New Zealand may eventually regulate agricultural GHG emissions, though rules are currently limited to a GHG reporting requirement until emission reductions are more economically and technically viable (Prokopy et al., 2015). Other aspects of soil management are regulated in some regions in the US, including the quantity and timing of nitrogen-based fertilizers or the application of manure, the use of fumigants to treat soilborne disease, or tillage activities via air quality particulate matter thresholds. A small minority of growers may be subject to contractual soil carbon obligations if enrolled in voluntary carbon markets, or through corporate supply chain purchasing agreements.
4.10.1. Limitations and opportunities
Soil carbon is not easily integrated into regulatory and contractual frameworks, as previously discussed in the context of carbon markets. As such, the continued administration of voluntary rather than mandatory programs is appropriate, as well as the development of farm-specific recommendations rather than one-size-fits-all prescriptions.
5. Opportunities for increasing soil carbon storage
What emerges from a detailed review of strategies for building and preserving soil carbon is that a sound approach should drive innovation, engage stakeholders, address structural issues and lower barriers to adoption, increase market valuation, be system-specific, not place undue burden on producers, provide near-term benefits and lasting change, promote co-benefits, and minimize externalities. Given this extensive list, it is clear that no one strategy is sufficient. Table 1 qualitatively illustrates how diverse approaches are required to achieve these goals, while Figure 3 illustrates how diverse stakeholders are also required.
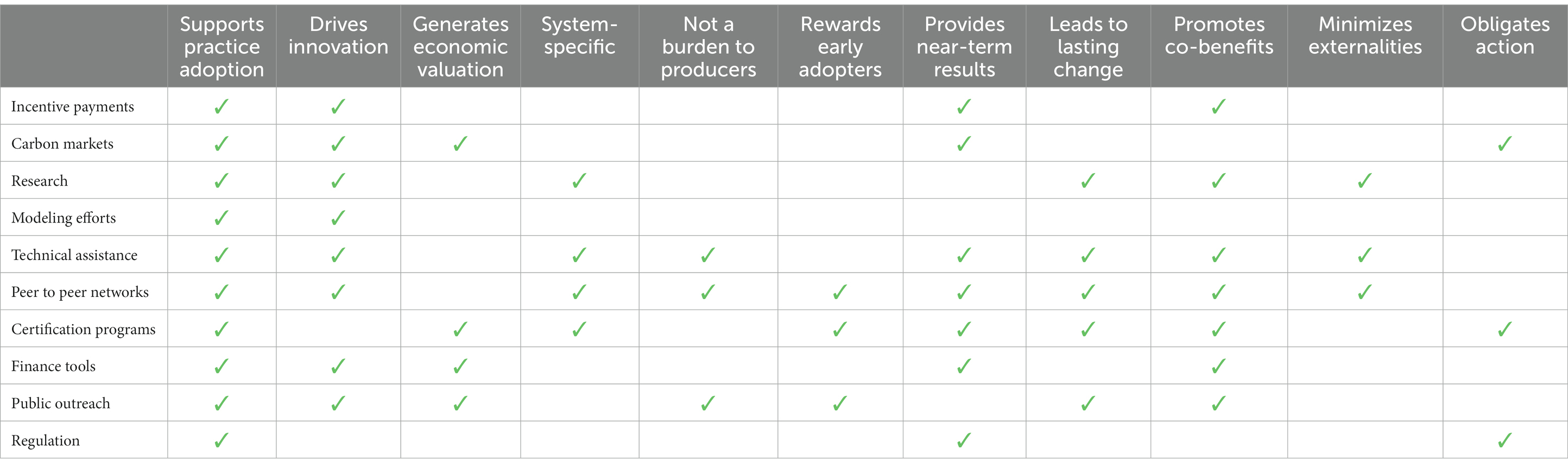
Table 1. Current United States strategies to building and preserving soil carbon, and their potential contributions to an effective, science-informed policy and program portfolio. This qualitative figure illustrates how diverse approaches are required to achieve multiple goals.
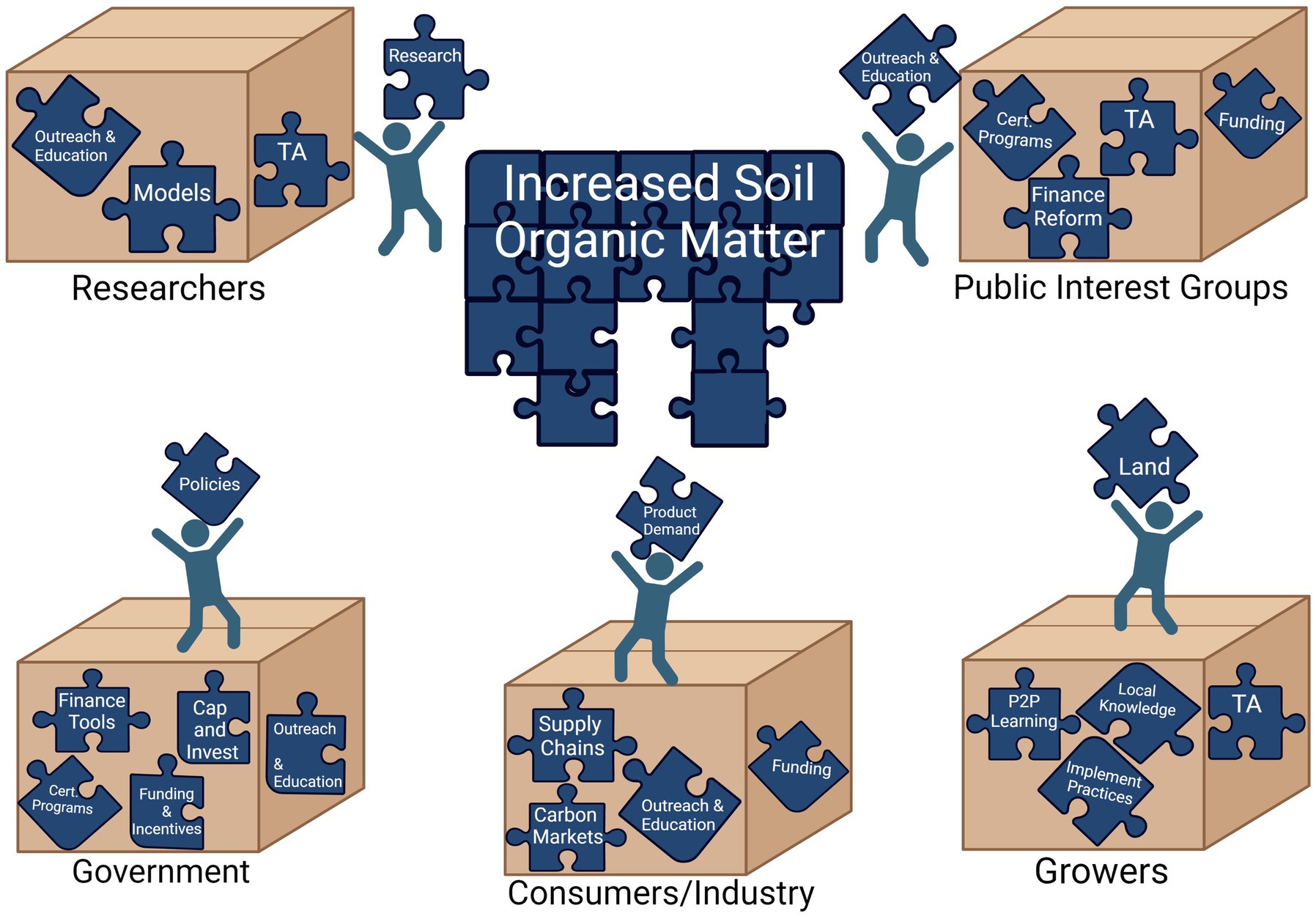
Figure 3. Conceptual diagram illustrating how diverse stakeholders contain the varied expertise required for the creation of an effective, science-informed policy and program portfolio in the United States.
We draw from the extensive scientific literature on soil carbon, and the strengths and limitations of current US approaches, to conclude by recommending that the below principles guide the creation of all future US policies and programs:
1. Natural climate solutions are only part of the solution. Climate change mitigation requires multiple strategies, including reducing current emissions (e.g., using less fertilizer per unit of production or driving a more fuel-efficient tractor), technological measures (e.g., geologic carbon capture and storage), and land management optimized for soil and vegetative carbon sequestration. Increased soil carbon cannot pick up the check for other emission sources, and will not solve the climate crisis in isolation.
2. Climate change adaptation must be prioritized alongside climate change mitigation. An exclusive policy focus on soil carbon for climate change mitigation misses opportunities to promote SOM co-benefits, and therefore climate adaptation and resilience. Policies and programs should protect soil and improve soil management, independent of the direct impact on carbon.
3. The science of soil carbon measurement is not yet sufficiently advanced to be responsibly integrated in the contractual frameworks proposed in cap-and-trade or regulatory schemes. Soil carbon formation and storage is complex, heterogenous, dynamic, and the science and technology are rapidly evolving. Measuring and modeling strategies must become more accurate, cost-effective, and scalable to be readily implemented.
4. Practice- and place-specific programs must be administered in lieu of one-size-fits-all prescriptions. The carbon sequestration potential of soil depends on a multitude of variables. Site specific programs are most likely to lead to science-informed recommendations, maximize the impact of conservation practices, minimize barriers to adoption, and avoid externalities.
5. Structural issues and barriers to adoption must be addressed as part of all programs and policies. This includes gaps in site- and practice-specific knowledge, lack of access to resources, lack of economic valuation for soil conservation, and inequities in how programs reach socially disadvantaged farmers. Significant investment in research, market development, technical assistance, outreach and education, and stakeholder engagement is required.
6. Effective and equitable soil carbon programs and policies require the collaboration of diverse stakeholders. Policymakers, governmental agencies, universities, growers, industry groups, public interest groups, environmental nonprofits and NGOs, consumers, and community members each have unique contributions to make to defensible, science-informed, and user-driven programs. All entities should be engaged early and often. Furthermore, collaboration between federal, state, and regional groups can lead to pooled resources and amplified impact.
7. Careful planning and investigation can minimize externalities. Environmental improvements should not be made at the expense of frontline communities, nor should a regional intervention have negative impacts elsewhere (e.g., reduced global crop yields, or reduced GHGs in one place in exchange for increased emissions elsewhere). Pollution trading, or exchanging one externality for another, should be carefully considered during the planning process of any policy or program.
Finally, and perhaps most importantly, is that the challenges in building and measuring soil carbon should not dissuade action. Soils are the foundation of our agricultural and social systems. The wholesale protection of soils and improvement of soil management is required to promote ecosystem services such as carbon sequestration, air and water filtration, crop production, and biodiversity support. The current popular and political momentum must be harnessed to address climate change, and to protect this invaluable terrestrial resource. Through collaboration, careful planning, and the acknowledgement that soil carbon storage is complex and nuanced, soils can remain a vital tool in working towards a more sustainable future.
Author contributions
DG wrote the first draft of the manuscript and designed Table 1. DR designed the Figures 1–3. All authors contributed equally to the conception of this manuscript, revising the manuscript, table, and figures, and approved the final submitted version.
Acknowledgments
The authors thank Kate M. Scow (UC Davis), Georgine G. Yorgey and Kirsten R. Ball (Washington State University), and Arohi Sharma, Lara Bryant, and Claire O’Connor (Natural Resources Defense Council) for their time and invaluable insight during the revision process of this manuscript.
Conflict of interest
The authors declare that the research was conducted in the absence of any commercial or financial relationships that could be construed as a potential conflict of interest.
Publisher’s note
All claims expressed in this article are solely those of the authors and do not necessarily represent those of their affiliated organizations, or those of the publisher, the editors and the reviewers. Any product that may be evaluated in this article, or claim that may be made by its manufacturer, is not guaranteed or endorsed by the publisher.
References
Amsili, J. P., van Es, H. M., Schindelbeck, K. S. M., Wolfe, D. W., and Barshad, G. (2020). Characterization of soil health in New York state: Summary. New York Soil Health Initiative. Cornell University: Ithica, NY.
Amundson, R. (2020). The policy challenges to managing global soil resources. Geoderma 379:114639. doi: 10.1016/j.geoderma.2020.114639
Amundson, R. (2022). Kiss the ground (and make a wish): soil science and Hollywood. Biogeochemistry 157, 127–130. doi: 10.1007/s10533-021-00857-w
Amundson, R., Buck, H., and Lajtha, K. (2022). Soil science in the time of climate mitigation. Biogeochemistry 161, 47–58. doi: 10.1007/s10533-022-00952-6
Angst, G., Mueller, K. E., Nierop, K. G. J., and Simpson, M. J. (2021). Plant- or microbial-derived? A review on the molecular composition of stabilized soil organic matter. Soil Biol. Biochem. 156:108189. doi: 10.1016/j.soilbio.2021.108189
Annan, F., and Schlenker, W. (2015). Federal Crop Insurance and the disincentive to adapt to extreme heat. Am. Econ. Rev. 105, 262–266. doi: 10.1257/aer.p20151031
Bai, X., Huang, Y., Ren, W., Coyne, M., Jacinthe, P.-A., Tao, B., et al. (2019). Responses of soil carbon sequestration to climate-smart agriculture practices: a meta-analysis. Glob. Chang. Biol. 25, 2591–2606. doi: 10.1111/gcb.14658
Baker, J. M., Ochsner, T. E., Venterea, R. T., and Griffis, T. J. (2007). Tillage and soil carbon sequestration—what do we really know? Agric. Ecosyst. Environ. 118, 1–5. doi: 10.1016/j.agee.2006.05.014
Ball, K. R., Baldock, J. A., Penfold, C., Power, S. A., Woodin, S. J., Smith, P., et al. (2020). Soil organic carbon and nitrogen pools are increased by mixed grass and legume cover crops in vineyard agroecosystems: detecting short-term management effects using infrared spectroscopy. Geoderma 379:114619. doi: 10.1016/j.geoderma.2020.114619
Ball, K. R., Brady, M. P., Burke, I. C., Collins, D. P., Hills, K. M., Kruger, C. E., et al. (2023). Evaluating compost application for soil carbon sequestration on agricultural land and compost buy-Back programs in Washington state. Center for Sustaining Agriculture & Natural Resources, Washington State University Available at: https://app.leg.wa.gov/ReportsToTheLegislature/Home/GetPDF?fileName=CSANR%20Organics%20Proviso_c588330e-636c-4db9-8844-2ea77c87073e.pdf (Accessed March 2, 2023).
Barker, J. R., Baumgardner, G. A., Turner, D. P., and Lee, J. J. (1996). Carbon dynamics of the conservation and wetland reserve programs. J. Soil Water Conserv. 51, 340–346.
Barthès, B. G., and Chotte, J.-L. (2021). Infrared spectroscopy approaches support soil organic carbon estimations to evaluate land degradation. Land Degrad. Dev. 32, 310–322. doi: 10.1002/ldr.3718
Barthès, B., and Roose, E. (2002). Aggregate stability as an indicator of soil susceptibility to runoff and erosion; validation at several levels. Catena 47, 133–149. doi: 10.1016/S0341-8162(01)00180-1
Bartkowski, B. (2021). Don’t throw efficiency out with the bathwater: a reply to Jeffery and Verheijen (2020). Environ. Sci. Pol. 122, 72–74. doi: 10.1016/j.envsci.2021.04.011
Bartkowski, B., Droste, N., Ließ, M., Sidemo-Holm, W., Weller, U., and Brady, M. V. (2021). Payments by modelled results: a novel design for Agri-environmental schemes. Land Use Policy 102:105230. doi: 10.1016/j.landusepol.2020.105230
Basile-Doelsch, I., Balesdent, J., and Pellerin, S. (2020). Reviews and syntheses: the mechanisms underlying carbon storage in soil. Biogeosciences 17, 5223–5242. doi: 10.5194/bg-17-5223-2020
Baveye, P. C. (2021a). Bypass and hyperbole in soil research: a personal view on plausible causes and possible remedies. Eur. J. Soil Sci. 72, 21–28. doi: 10.1111/ejss.12940
Baveye, P. C. (2021b). Soil health at a crossroad. Soil Use Manag. 37, 215–219. doi: 10.1111/sum.12703
Beckie, H. J., Smyth, S. J., Owen, M. D. K., and Gleim, S. (2019). Rewarding best Pest management practices via reduced crop insurance premiums. Int. J. Agron. 2019:e9390501, 1–11. doi: 10.1155/2019/9390501
Bell, A. R., Rakotonarivo, O. S., Bhargava, A., Duthie, A. B., Zhang, W., Sargent, R., et al. (2023). Financial incentives often fail to reconcile agricultural productivity and pro-conservation behavior. Commun. Earth Environ. 4, 1–12. doi: 10.1038/s43247-023-00689-6
Blanco-Canqui, H., Shapiro, C. A., Wortmann, C. S., Drijber, R. A., Mamo, M., Shaver, T. M., et al. (2013). Soil organic carbon: the value to soil properties. J. Soil Water Conserv. 68, 129A–134A. doi: 10.2489/jswc.68.5.129A
Bossio, D. A., Cook-Patton, S. C., Ellis, P. W., Fargione, J., Sanderman, J., Smith, P., et al. (2020). The role of soil carbon in natural climate solutions. Nat Sustain 3, 391–398. doi: 10.1038/s41893-020-0491-z
Bradford, M. A., Carey, C. J., Atwood, L., Bossio, D., Fenichel, E. P., Gennet, S., et al. (2019). Soil carbon science for policy and practice. Nat Sustain 2, 1070–1072. doi: 10.1038/s41893-019-0431-y
Brown, K., Schirmer, J., and Upton, P. (2021). Regenerative farming and human wellbeing: are subjective wellbeing measures useful indicators for sustainable farming systems? Environ. Sustain. Indicat. 11:100132. doi: 10.1016/j.indic.2021.100132
Buck, H. J., and Palumbo-Compton, A. (2022). Soil carbon sequestration as a climate strategy: what do farmers think? Biogeochemistry 161, 59–70. doi: 10.1007/s10533-022-00948-2
Buckeridge, K. M., Creamer, C., and Whitaker, J. (2022). Deconstructing the microbial necromass continuum to inform soil carbon sequestration. Funct. Ecol. 36, 1396–1410. doi: 10.1111/1365-2435.14014
Carey, J. C., Tang, J., Templer, P. H., Kroeger, K. D., Crowther, T. W., Burton, A. J., et al. (2016). Temperature response of soil respiration largely unaltered with experimental warming. Proc. Natl. Acad. Sci. 113, 13797–13802. doi: 10.1073/pnas.1605365113
Carlisle, L. (2016). Factors influencing farmer adoption of soil health practices in the United States: a narrative review. Agroecol. Sustain. Food Syst. 40, 583–613. doi: 10.1080/21683565.2016.1156596
Chenu, C., Angers, D. A., Barré, P., Derrien, D., Arrouays, D., and Balesdent, J. (2019). Increasing organic stocks in agricultural soils: knowledge gaps and potential innovations. Soil Tillage Res. 188, 41–52. doi: 10.1016/j.still.2018.04.011
Chowaniak, M., Głąb, T., Klima, K., Niemiec, M., Zaleski, T., and Zuzek, D. (2020). Effect of tillage and crop management on runoff, soil erosion and organic carbon loss. Soil Use Manag. 36, 581–593. doi: 10.1111/sum.12606
Collins, H. P., Rasmussen, P. E., and Douglas, C. L. Jr. (1992). Crop rotation and residue management effects on soil carbon and microbial dynamics. Soil Sci. Soc. Am. J. 56, 783–788. doi: 10.2136/sssaj1992.03615995005600030018x
Cook, J., Oreskes, N., Doran, P., Anderegg, W., Verheggen, B., Maibach, E., et al. (2016). Consensus on consensus: a synthesis of consensus estimates on human-caused global warming. Environ. Res. Lett. 11:048002. doi: 10.1088/1748-9326/11/4/048002
Cotrufo, M. F., and Lavallee, J. M. (2022). Chapter One - Soil organic matter formation, persistence, and functioning: A synthesis of current understanding to inform its conservation and regeneration. Advances Agronomy 172, 1–66. doi: 10.1016/bs.agron.2021.11.002
Crippa, M., Solazzo, E., Guizzardi, D., Monforti-Ferrario, F., Tubiello, F. N., and Leip, A. (2021). Food systems are responsible for a third of global anthropogenic GHG emissions. Nat. Food 2, 198–209. doi: 10.1038/s43016-021-00225-9
Croft, G. K., Hoover, K., Ramseur, J. L., and Stubbs, M. (2021). Agriculture and forestry offsets in carbon markets: background and selected issues. US: United States congressional research service, CRS. Available at: https://crsreports.congress.gov/product/pdf/R/R46956.
Crookston, B. S., Yost, M. A., Bowman, M., Veum, K., Cardon, G., and Norton, J. (2021). Soil health spatial-temporal variation influence soil security on Midwestern, U.S. farms. Soil Secur. 3:100005. doi: 10.1016/j.soisec.2021.100005
Crowther, T. W., Todd-Brown, K. E. O., Rowe, C. W., Wieder, W. R., Carey, J. C., Machmuller, M. B., et al. (2016). Quantifying global soil carbon losses in response to warming. Nature 540, 104–108. doi: 10.1038/nature20150
Culman, S., Deiss, L., Fortune, B., Gingery, M., Mann, M., Sprunger, C., et al. (2022). Baseline assessment of soil health in Ohio. Columbus, Ohio: The Ohio State University. Available at: https://soilhealth.osu.edu/sites/soilhealth/files/imce/WhitePapers/Baseline%20Ohio%20Soil%20Health.pdf
Cushing, L., Blaustein-Rejto, D., Wander, M., Pastor, M., Sadd, J., Zhu, A., et al. (2018). Carbon trading, co-pollutants, and environmental equity: evidence from California’s cap-and-trade program (2011–2015). PLoS Med. 15:e1002604. doi: 10.1371/journal.pmed.1002604
Delgado-Baquerizo, M., Reich, P. B., Trivedi, C., Eldridge, D. J., Abades, S., Alfaro, F. D., et al. (2020). Multiple elements of soil biodiversity drive ecosystem functions across biomes. Nat. Ecol. Evol. 4, 210–220. doi: 10.1038/s41559-019-1084-y
Derrien, D., Barré, P., Basile-Doelsch, I., Cécillon, L., Chabbi, A., Crème, A., et al. (2023). Current controversies on mechanisms controlling soil carbon storage: implications for interactions with practitioners and policy-makers. Rev. Agron. Sustain. Dev. 43:21. doi: 10.1007/s13593-023-00876-x
Dynarski, K. A., Bossio, D. A., and Scow, K. M. (2020). Dynamic stability of soil carbon: reassessing the “permanence” of soil carbon sequestration. Front. Environ. Sci. 8. doi: 10.3389/fenvs.2020.514701
Emerson, W. W. (1995). Water-retention, organic-C and soil texture. Soil Res. 33, 241–251. doi: 10.1071/sr9950241
Ervine, K. (2018). How low can it go? Analysing the political economy of carbon market design and low carbon prices. New Polit. Econ. 23, 690–710. doi: 10.1080/13563467.2018.1384454
Fageria, N. K. (2012). Role of soil organic matter in maintaining sustainability of cropping systems. Commun. Soil Sci. Plant Anal. 43, 2063–2113. doi: 10.1080/00103624.2012.697234
Feeney, C. J., Cosby, B. J., Robinson, D. A., Thomas, A., Emmett, B. A., and Henrys, P. (2022). Multiple soil map comparison highlights challenges for predicting topsoil organic carbon concentration at national scale. Sci. Rep. 12:1379. doi: 10.1038/s41598-022-05476-5
Feller, C., and Bernoux, M. (2008). Historical advances in the study of global terrestrial soil organic carbon sequestration. Waste Manag. 28, 734–740. doi: 10.1016/j.wasman.2007.09.022
Finke, P. A. (2012). On digital soil assessment with models and the Pedometrics agenda. Geoderma 171-172, 3–15. doi: 10.1016/j.geoderma.2011.01.001
Forster, P., Storevelmo, T., Armour, K., Collins, W., Dufresne, J. L., Frame, D., et al. (2021). “The Earth’s energy budget, climate feedbacks, and climate sensitivity” in Climate change 2021: The physical science basis. Contribution of working group I to the sixth assessment report of the intergovernmental panel on climate change. eds. R. Colman, H. D. Matthews and V. Ramaswamy (Cambridge, United Kingdom and New York, NY, USA: Intergovernmental Panel on Climate Change), 923–1054.
Gál, A., Vyn, T. J., Michéli, E., Kladivko, E. J., and McFee, W. W. (2007). Soil carbon and nitrogen accumulation with long-term no-till versus moldboard plowing overestimated with tilled-zone sampling depths. Soil Tillage Res. 96, 42–51. doi: 10.1016/j.still.2007.02.007
Gauthier, V., Monast, M., Watkins, S., Moik, K., and Chen, T. (2022). The impacts of climate change on agricultural finance. Environmental Defense Fund, Deloitte Consulting LLP. Available at: https://business.edf.org/files/impacts-climate-change-agricultural-finance-survey.pdf (Accessed December 12, 2022).
Gebhart, D. L., Johnson, H. B., Mayeux, H. S., and Polley, H. W. (1994). The CRP increases soil organic carbon. J. Soil Water Conserv. 49, 488–492.
Goll, D. S., Ciais, P., Amann, T., Buermann, W., Chang, J., Eker, S., et al. (2021). Potential CO2 removal from enhanced weathering by ecosystem responses to powdered rock. Nat. Geosci. 14, 545–549. doi: 10.1038/s41561-021-00798-x
Gosnell, H., Robinson-Maness, N., and Charnley, S. (2011). Engaging ranchers in market-based approaches to climate change mitigation: opportunities, challenges, and policy implications. Rangelands 33, 20–24. doi: 10.2111/1551-501X-33.5.20
Gross, C. D., and Harrison, R. B. (2019). The case for digging deeper: soil organic carbon storage, dynamics, and controls in our changing world. Soil Syst. 3:28. doi: 10.3390/soilsystems3020028
Guo, L. B., and Gifford, R. M. (2002). Soil carbon stocks and land use change: a meta analysis. Glob. Chang. Biol. 8, 345–360. doi: 10.1046/j.1354-1013.2002.00486.x
Happ, M. (2021). Closed out: how U.S. farmers are denied access to conservation programs. Institute for Agriculture and Trade Policy. Available at: https://www.iatp.org/documents/closed-out-how-us-farmers-are-denied-access-conservation-programs.
Huggins, D. R., and Reganold, J. P. (2008). No-till: the quiet revolution. Sci. Am. 299, 70–77. doi: 10.1038/scientificamerican0708-70
Jansson, C., Faiola, C., Wingler, A., Zhu, X.-G., Kravchenko, A., de Graaff, M.-A., et al. (2021). Crops for carbon farming. Front. Plant Sci. 12:636709. doi: 10.3389/fpls.2021.636709
Jansson, C., Wullschleger, S. D., Kalluri, U. C., and Tuskan, G. A. (2010). Phytosequestration: carbon biosequestration by plants and the prospects of genetic engineering. Bioscience 60, 685–696. doi: 10.1525/bio.2010.60.9.6
Janzen, H. H. (2006). The soil carbon dilemma: shall we hoard it or use it? Soil Biol. Biochem. 38, 419–424. doi: 10.1016/j.soilbio.2005.10.008
Janzen, H. H., Janzen, D. W., and Gregorich, E. G. (2021). The ‘soil health’ metaphor: illuminating or illusory? Soil Biol. Biochem. 159:108167. doi: 10.1016/j.soilbio.2021.108167
Jeffery, S., and Verheijen, F. G. A. (2020). A new soil health policy paradigm: pay for practice not performance! Environ. Sci. Pol. 112, 371–373. doi: 10.1016/j.envsci.2020.07.006
Jian, J., Du, X., Reiter, M. S., and Stewart, R. D. (2020). A meta-analysis of global cropland soil carbon changes due to cover cropping. Soil Biol. Biochem. 143:107735. doi: 10.1016/j.soilbio.2020.107735
Kantola, I. B., Masters, M. D., Beerling, D. J., Long, S. P., and DeLucia, E. H. (2017). Potential of global croplands and bioenergy crops for climate change mitigation through deployment for enhanced weathering. Biol. Lett. 13:20160714. doi: 10.1098/rsbl.2016.0714
Karlen, D. L., Goeser, N. J., Veum, K. S., and Yost, M. A. (2017). On-farm soil health evaluations: challenges and opportunities. J. Soil Water Conserv. 72, 26A–31A. doi: 10.2489/jswc.72.2.26A
Keskin, H., Grunwald, S., and Harris, W. G. (2019). Digital mapping of soil carbon fractions with machine learning. Geoderma 339, 40–58. doi: 10.1016/j.geoderma.2018.12.037
Kopittke, P. M., Berhe, A. A., Carrillo, Y., Cavagnaro, T. R., Chen, D., Chen, Q.-L., et al. (2022). Ensuring planetary survival: the centrality of organic carbon in balancing the multifunctional nature of soils. Crit. Rev. Environ. Sci. Technol. 52, 4308–4324. doi: 10.1080/10643389.2021.2024484
Kreibich, N., and Hermwille, L. (2021). Caught in between: credibility and feasibility of the voluntary carbon market post-2020. Clim. Pol. 21, 939–957. doi: 10.1080/14693062.2021.1948384
Lal, R. (2004). Soil carbon sequestration to mitigate climate change. Geoderma 123, 1–22. doi: 10.1016/j.geoderma.2004.01.032
Lavallee, J. M., Soong, J. L., and Cotrufo, M. F. (2020). Conceptualizing soil organic matter into particulate and mineral-associated forms to address global change in the 21st century. Glob. Chang. Biol. 26, 261–273. doi: 10.1111/gcb.14859
Lehmann, J., Bossio, D. A., Kögel-Knabner, I., and Rillig, M. C. (2020). The concept and future prospects of soil health. Nat. Rev. Earth Environ. 1, 544–553. doi: 10.1038/s43017-020-0080-8
Lundgren, T., Marklund, P.-O., Samakovlis, E., and Zhou, W. (2015). Carbon prices and incentives for technological development. J. Environ. Manag. 150, 393–403. doi: 10.1016/j.jenvman.2014.12.015
Luo, Z., Wang, E., and Sun, O. J. (2010). Can no-tillage stimulate carbon sequestration in agricultural soils? A meta-analysis of paired experiments. Agric. Ecosyst. Environ. 139, 224–231. doi: 10.1016/j.agee.2010.08.006
Marston, J. (2022). It’s time to hold agrifood corporates accountable for their climate commitments. This list may help. AgFunderNews. Available at: https://agfundernews.com/list-of-agrifood-corporate-climate-commitments-accountable (Accessed August 22, 2022).
McTiernan, K. B., Jarvis, S. C., Scholefield, D., and Hayes, M. H. B. (2001). Dissolved organic carbon losses from grazed grasslands under different management regimes. Water Res. 35, 2565–2569. doi: 10.1016/S0043-1354(00)00528-5
Miltner, A., Bombach, P., Schmidt-Brücken, B., and Kästner, M. (2012). SOM genesis: microbial biomass as a significant source. Biogeochemistry 111, 41–55. doi: 10.1007/s10533-011-9658-z
Monger, H. C., Kraimer, R. A., Khresat, S., Cole, D. R., Wang, X., and Wang, J. (2015). Sequestration of inorganic carbon in soil and groundwater. Geology 43, 375–378. doi: 10.1130/G36449.1
Moore, T. R. (1997). “Dissolved organic carbon: sources, sinks, and fluxes and role in the soil carbon cycle” in Soil processes and the carbon cycle. eds. R. Lal, J. M. Kimble, R. F. Follett and B. A. Stewart (Boca, Raton: CRC Press).
Motta, G. A., Tekinerdogan, B., and Athanasiadis, I. N. (2020). Blockchain applications in the Agri-food domain: the first wave. Front Blockchain 3:6. doi: 10.3389/fbloc.2020.00006
National Sustainable Agriculture Coalition (2021). Climate solutions for farmers: Invest in proven conservation programs not carbon markets. Washington, DC: National Sustainable Agriculture Coalition. Available at: https://sustainableagriculture.net/wp-content/uploads/2021/06/Climate-Solutions-for-Farmers_-Invest-in-Proven-Conservation-Programs-Not-Carbon-Markets-1.pdf (Accessed August 25, 2022).
Natural Resources Defense Council (2017). Covering crops: how federal crop insurance program reforms can reduce costs, empower farmers, and protect natural resources. Washington, DC: Resources Defense Council. Available at: https://www.nrdc.org/sites/default/files/federal-crop-insurance-program-reforms-ip.pdf (Accessed October 26, 2022)
Nguyen, T. T. (2021). Predicting agricultural soil carbon using machine learning. Nat Rev Earth Environ 2:825. doi: 10.1038/s43017-021-00243-y
Norris, C. E., Bean, G. M., Cappellazzi, S. B., Cope, M., Greub, K. L. H., Liptzin, D., et al. (2020). Introducing the north American project to evaluate soil health measurements. Agron. J. 112, 3195–3215. doi: 10.1002/agj2.20234
Norton, G. W., and Alwang, J. (2020). Changes in agricultural extension and implications for farmer adoption of new practices. Appl. Econ. Perspect. Policy 42, 8–20. doi: 10.1002/aepp.13008
Oades, J. M. (1984). Soil organic matter and structural stability: mechanisms and implications for management. Plant Soil 76, 319–337. doi: 10.1007/BF02205590
Oelkers, E. H., and Cole, D. R. (2008). Carbon dioxide sequestration a solution to a global problem. Elements 4, 305–310. doi: 10.2113/gselements.4.5.305
Ogle, S. M., Alsaker, C., Baldock, J., Bernoux, M., Breidt, F. J., McConkey, B., et al. (2019). Climate and soil characteristics determine where no-till Management can store carbon in soils and mitigate greenhouse gas emissions. Sci. Rep. 9:11665. doi: 10.1038/s41598-019-47861-7
Ogle, S. M., Swan, A., and Paustian, K. (2012). No-till management impacts on crop productivity, carbon input and soil carbon sequestration. Agric. Ecosyst. Environ. 149, 37–49. doi: 10.1016/j.agee.2011.12.010
Oldfield, E. E., Eagle, A. J., Rubin, R. L., Rudek, J., Sanderman, J., and Gordon, D. R. (2021). Agricultural soil carbon credits: making sense of protocols for carbon sequestration and net greenhouse gas removals. New York, New York: Environmental Defense Fund
Passioura, J. B. (2010). Scaling up: the essence of effective agricultural research. Functional Plant Biol. 37, 585–591. doi: 10.1071/FP10106
Patoine, G., Eisenhauer, N., Cesarz, S., Phillips, H. R. P., Xu, X., Zhang, L., et al. (2022). Drivers and trends of global soil microbial carbon over two decades. Nat. Commun. 13:4195. doi: 10.1038/s41467-022-31833-z
Paustian, K., Collier, S., Baldock, J., Burgess, R., Creque, J., DeLonge, M., et al. (2019). Quantifying carbon for agricultural soil management: from the current status toward a global soil information system. Carbon Manage 10, 567–587. doi: 10.1080/17583004.2019.1633231
Paustian, K., Easter, M., Brown, K., Chambers, A., Eve, M., Huber, A., et al. (2018). “Field- and farm-scale assessment of soil greenhouse gas mitigation using COMET-farm” in Agronomy Monographs. eds. J. A. Delgado, G. F. Sassenrath and T. Mueller (Madison, WI, USA: American Society of Agronomy, Crop Science Society of America, Soil Science Society of America), 341–359.
Perera, F., and Nadeau, K. (2022). Climate change, fossil-fuel pollution, and Children’s health. N. Engl. J. Med. 386, 2303–2314. doi: 10.1056/NEJMra2117706
Piñeiro, V., Arias, J., Dürr, J., Elverdin, P., Ibáñez, A. M., Kinengyere, A., et al. (2020). A scoping review on incentives for adoption of sustainable agricultural practices and their outcomes. Nat Sustain 3, 809–820. doi: 10.1038/s41893-020-00617-y
Pittelkow, C. M., Linquist, B. A., Lundy, M. E., Liang, X., van Groenigen, K. J., Lee, J., et al. (2015). When does no-till yield more? A global meta-analysis. Field Crop Res. 183, 156–168. doi: 10.1016/j.fcr.2015.07.020
Powlson, D. S. (2021). Is “soil health” meaningful as a scientific concept or as terminology? Soil Use Manage 37, 403–405. doi: 10.1111/sum.12721
Pretty, J., and Bharucha, Z. P. (2014). Sustainable intensification in agricultural systems. Ann. Bot. 114, 1571–1596. doi: 10.1093/aob/mcu205
Prokopy, L. S., Arbuckle, J. G., Barnes, A. P., Haden, V. R., Hogan, A., Niles, M. T., et al. (2015). Farmers and climate change: a cross-National Comparison of beliefs and risk perceptions in high-income countries. Environ. Manag. 56, 492–504. doi: 10.1007/s00267-015-0504-2
Ranjan, P., Church, S. P., Floress, K., and Prokopy, L. S. (2019). Synthesizing conservation motivations and barriers: what have we learned from qualitative studies of farmers’ behaviors in the United States? Soc. Nat. Resour. 32, 1171–1199. doi: 10.1080/08941920.2019.1648710
Reed, D. (2020). “Ecosystem services markets conceived and designed for US agriculture” in Soil and water conservation: A Celebration of 75 years. eds. J. A. Delgado, C. J. Gantzer, and G. F. Sassenrath (Ankeny, Iowa, USA: Soil and Water Conservation Society), 70–74.
Riar, A., and Bhullar, G. S. (2020). “Chapter I - Long-term experiments in agriculture: stages, challenges, and precautions” in Long-term farming systems research. eds. G. S. Bhullar and A. Riar (New York, NY: Academic Press), 3–12.
Rose, D. C., Sutherland, W. J., Parker, C., Lobley, M., Winter, M., Morris, C., et al. (2016). Decision support tools for agriculture: towards effective design and delivery. Agric. Syst. 149, 165–174. doi: 10.1016/j.agsy.2016.09.009
Ruark, M. D., Brouder, S. M., and Turco, R. F. (2009). Dissolved organic carbon losses from tile drained Agroecosystems. J. Environ. Qual. 38, 1205–1215. doi: 10.2134/jeq2008.0121
Sagar, L., and Singh, S. (2020). Impact of agronomic practices on soil organic carbon dynamics: a review. Int. J. Chem. Stud. 8, 2173–2178. doi: 10.22271/chemi.2020.v8.i4x.9951
Sanderman, J., Hengl, T., and Fiske, G. J. (2017). Soil carbon debt of 12,000 years of human land use. Proc. Natl. Acad. Sci. 114, 9575–9580. doi: 10.1073/pnas.1706103114
Scharlemann, J. P. W., Tanner, E. V. J., Hiederer, R., and Kapos, V. (2014). Global soil carbon: understanding and managing the largest terrestrial carbon pool. Carbon Manage 5, 81–91. doi: 10.4155/cmt.13.77
Schlatter, D., Kinkel, L., Thomashow, L., Weller, D., and Paulitz, T. (2017). Disease suppressive soils: new insights from the soil microbiome. Phytopathology 107, 1284–1297. doi: 10.1094/PHYTO-03-17-0111-RVW
Schlesinger, W. H. (2022). Biogeochemical constraints on climate change mitigation through regenerative farming. Biogeochemistry 161, 9–17. doi: 10.1007/s10533-022-00942-8
Schulte-Uebbing, L. F., Beusen, A. H. W., Bouwman, A. F., and de Vries, W. (2022). From planetary to regional boundaries for agricultural nitrogen pollution. Nature 610, 507–512. doi: 10.1038/s41586-022-05158-2
Seddon, N., Smith, A., Smith, P., Key, I., Chausson, A., Girardin, C., et al. (2021). Getting the message right on nature-based solutions to climate change. Glob. Chang. Biol. 27, 1518–1546. doi: 10.1111/gcb.15513
Shang, P., Lu, Y., Du, Y., Jaffé, R., Findlay, R. H., and Wynn, A. (2018). Climatic and watershed controls of dissolved organic matter variation in streams across a gradient of agricultural land use. Sci. Total Environ. 612, 1442–1453. doi: 10.1016/j.scitotenv.2017.08.322
Sharratt, B. S., Kennedy, A. C., Hansen, J. C., and Schillinger, W. F. (2018). Soil carbon loss by wind Erosion of summer fallow fields in Washington’s Dryland wheat region. Soil Sci. Soc. Am. J. 82, 1551–1558. doi: 10.2136/sssaj2018.06.0214
Silva, E. C. D., and Zhu, X. (2009). Emissions trading of global and local pollutants, pollution havens and free riding. J. Environ. Econ. Manag. 58, 169–182. doi: 10.1016/j.jeem.2009.04.001
Skiba, U. M., and Rees, R. M. (2014). Nitrous oxide, climate change and agriculture. CABI Rev. 2014, 1–7. doi: 10.1079/PAVSNNR20149010
Slessarev, E. W., Mayer, A., Kelly, C., Georgiou, K., Pett-Ridge, J., and Nuccio, E. E. (2023). Initial soil organic carbon stocks govern changes in soil carbon: reality or artifact? Glob. Chang. Biol. 29, 1239–1247. doi: 10.1111/gcb.16491
Smith, P. (2005). An overview of the permanence of soil organic carbon stocks: influence of direct human-induced, indirect and natural effects. Eur. J. Soil Sci. 56, 673–680. doi: 10.1111/j.1365-2389.2005.00708.x
Smith, P., Soussana, J.-F., Angers, D., Schipper, L., Chenu, C., Rasse, D. P., et al. (2020). How to measure, report and verify soil carbon change to realize the potential of soil carbon sequestration for atmospheric greenhouse gas removal. Glob. Chang. Biol. 26, 219–241. doi: 10.1111/gcb.14815
Stanley, P., Spertus, J., Chiartas, J., Stark, P. B., and Bowles, T. (2023). Valid inferences about soil carbon in heterogeneous landscapes. Geoderma 430:116323. doi: 10.1016/j.geoderma.2022.116323
State Healthy Soil Policy Map (2021). Nerds for earth. Available at: https://nerdsforearth.com/state-healthy-soils-policy/ (Accessed August 25, 2022).
Stella, T., Mouratiadou, I., Gaiser, T., Berg-Mohnicke, M., Wallor, E., Ewert, F., et al. (2019). Estimating the contribution of crop residues to soil organic carbon conservation. Environ. Res. Lett. 14:094008. doi: 10.1088/1748-9326/ab395c
Stephenson, G., Gwin, L., Schreiner, C., and Brown, S. (2022). Perspectives on organic transition from transitioning farmers and farmers who decided not to transition. Renew Agric Food Syst 37, 633–643. doi: 10.1017/S1742170521000119
Stevens, A. W. (2018). Review: the economics of soil health. Food Policy 80, 1–9. doi: 10.1016/j.foodpol.2018.08.005
Stewart, C. E., Plante, A. F., Paustian, K., Conant, R. T., and Six, J. (2008). Soil carbon saturation: linking concept and measurable carbon pools. Soil Sci. Soc. Am. J. 72, 379–392. doi: 10.2136/sssaj2007.0104
Stott, D. E. (2019). Recommended soil health indicators and associated laboratory procedures. USDA NRCS Soil Health Technical Note No. 450-03, 76.
Sulman, B. N., Moore, J. A. M., Abramoff, R., Averill, C., Kivlin, S., Georgiou, K., et al. (2018). Multiple models and experiments underscore large uncertainty in soil carbon dynamics. Biogeochemistry 141, 109–123. doi: 10.1007/s10533-018-0509-z
Sutherland, L.-A., and Marchand, F. (2021). On-farm demonstration: enabling peer-to-peer learning. J. Agric. Educ. Ext. 27, 573–590. doi: 10.1080/1389224X.2021.1959716
Teixeira, H. M., Vermue, A. J., Cardoso, I. M., Peña Claros, M., and Bianchi, F. J. J. A. (2018). Farmers show complex and contrasting perceptions on ecosystem services and their management. Ecosyst. Serv. 33, 44–58. doi: 10.1016/j.ecoser.2018.08.006
Thaler, E. A., Larsen, I. J., and Yu, Q. (2019). A new index for remote sensing of soil organic carbon based solely on visible wavelengths. Soil Sci. Soc. Am. J. 83, 1443–1450. doi: 10.2136/sssaj2018.09.0318
Thøgersen, J., Pedersen, S., and Aschemann-Witzel, J. (2019). The impact of organic certification and country of origin on consumer food choice in developed and emerging economies. Food Qual. Prefer. 72, 10–30. doi: 10.1016/j.foodqual.2018.09.003
Tiessen, H., Cuevas, E., and Chacon, P. (1994). The role of soil organic matter in sustaining soil fertility. Nature 371, 783–785. doi: 10.1038/371783a0
United States Department of Agriculture (2022). RCA Environmental Quality Incentive Program (EQIP) Report. Washington, DC: United States Department of Agriculture. Available at: https://www.nrcs.usda.gov/rca-conservation-program-reports
United States Department of Agriculture (n.d.). A brief history of NRCS. Natural Resources Conservation Service. Washington, DC: USDA. Available at: http://www.nrcs.usda.gov/about/history/brief-history-nrcs (Accessed March 2, 2023).
van der Pol, L. K., Tibbetts, C. A., and Lin Hunter, D. E. (2021). Removing barriers and creating opportunities for climate-resilient agriculture by optimizing Federal Crop Insurance. J. Sci. Policy Govern. 18. doi: 10.38126/JSPG180213
van Gestel, N., Shi, Z., van Groenigen, K. J., Osenberg, C. W., Andresen, L. C., Dukes, J. S., et al. (2018). Predicting soil carbon loss with warming. Nature 554, E4–E5. doi: 10.1038/nature25745
Van Wassenaer, L., van Hilten, M., van Ingen, E., and van Asseldonk, M. (2021). Applying blockchain for climate action in agriculture: State of play and outlook. Rome/Wageningen: FAO and WUR
Vereecken, H., Schnepf, A., Hopmans, J. W., Javaux, M., Or, D., Roose, T., et al. (2016). Modeling soil processes: review, key challenges, and new perspectives. Vadose Zone J. 15, 1–57. doi: 10.2136/vzj2015.09.0131
Vermeulen, S., Bossio, D., Lehmann, J., Luu, P., Paustian, K., Webb, C., et al. (2019). A global agenda for collective action on soil carbon. Nat Sustain 2, 2–4. doi: 10.1038/s41893-018-0212-z
Wade, J., Beetstra, M. A., Hamilton, M. L., Culman, S. W., and Margenot, A. J. (2021). Soil health conceptualization differs across key stakeholder groups in the Midwest. J. Soil Water Conserv. 76, 527–533. doi: 10.2489/jswc.2021.02158
Wade, J., Maltais-Landry, G., Lucas, D. E., Bongiorno, G., Bowles, T. M., Calderón, F. J., et al. (2020). Assessing the sensitivity and repeatability of permanganate oxidizable carbon as a soil health metric: an interlab comparison across soils. Geoderma 366:114235. doi: 10.1016/j.geoderma.2020.114235
Wallach, D., Martre, P., Liu, B., Asseng, S., Ewert, F., Thorburn, P. J., et al. (2018). Multimodel ensembles improve predictions of crop–environment–management interactions. Glob. Chang. Biol. 24, 5072–5083. doi: 10.1111/gcb.14411
Wallander, S., Smith, D., Bowman, M., and Claassen, R. (2021). Cover crop trends, programs, and practices in the United States. Washington, DC: United States Department of Agriculture Economic Research Service. Available at: https://www.ers.usda.gov/webdocs/publications/100551/eib-222.pdf?v=2824.5.
Warner, A., and Watnick, D. (2021). Soil health policy: developing community-driven state soil health policy and programs. New Haven, Connecticut, USA: Yale Center for Business and the Environment. Available at: https://cbey.yale.edu/research/soil-health-policy-developing-community-driven-state-soil-health-policy-and-programs.
Weinberg, M., and Claassen, R. (2006). Rewarding farm practices versus environmental performance. Washington, DC: United states department of agriculture economic research service. Available at: https://www.ers.usda.gov/webdocs/publications/42913/29515_eb5_002.pdf?v=56.7.
Wick, A. F., Haley, J., Gasch, C., Wehlander, T., Briese, L., and Samson-Liebig, S. (2019). Network-based approaches for soil health research and extension programming in North Dakota, USA. Soil Use Manag. 35, 177–184. doi: 10.1111/sum.12444
Wiesmeier, M., Urbanski, L., Hobley, E., Lang, B., von Lützow, M., Marin-Spiotta, E., et al. (2019). Soil organic carbon storage as a key function of soils - a review of drivers and indicators at various scales. Geoderma 333, 149–162. doi: 10.1016/j.geoderma.2018.07.026
Winiwarter, W., and Mohankumar, S. E. P. (2015). Reducing nitrous oxide emissions from agriculture: review on options and costs. International Institute for Applied Systems Analysis (IIASA). Available at: https://pure.iiasa.ac.at/id/eprint/13396/
Wongpiyabovorn, O., Plastina, A., and Crespi, J. M. (2022). Challenges to voluntary Ag carbon markets. Appl Econ Perspect Policy 45, 1154–1167. doi: 10.1002/aepp.13254
Wuest, S. (2014). Seasonal variation in soil organic carbon. Soil Sci. Soc. Am. J. 78, 1442–1447. doi: 10.2136/sssaj2013.10.0447
Yu, Z., Lu, C., Hennessy, D. A., Feng, H., and Tian, H. (2020). Impacts of tillage practices on soil carbon stocks in the US corn-soybean cropping system during 1998 to 2016. Environ. Res. Lett. 15:014008. doi: 10.1088/1748-9326/ab6393
Zelikova, J., Chay, F., Freeman, J., and Cullenward, D. (2021). A buyer’s guide to soil carbon offsets. Available at: https://carbonplan.org/blog/soil-protocols-added (Accessed August 31, 2022).
Keywords: carbon sequestration, policy, regenerative agriculture, climate smart agriculture, climate change, soil carbon
Citation: Gelardi DL, Rath D and Kruger CE (2023) Grounding United States policies and programs in soil carbon science: strengths, limitations, and opportunities. Front. Sustain. Food Syst. 7:1188133. doi: 10.3389/fsufs.2023.1188133
Edited by:
Stefan Kepinski, University of Leeds, United KingdomReviewed by:
Gurbir S. Bhullar, Bern University of Applied Sciences, SwitzerlandJulie Ingram, University of Gloucestershire, United Kingdom
Copyright © 2023 Gelardi, Rath and Kruger This is an open-access article distributed under the terms of the Creative Commons Attribution License (CC BY). The use, distribution or reproduction in other forums is permitted, provided the original author(s) and the copyright owner(s) are credited and that the original publication in this journal is cited, in accordance with accepted academic practice. No use, distribution or reproduction is permitted which does not comply with these terms.
*Correspondence: Danielle L. Gelardi, ZGdlbGFyZGlAYWdyLndhLmdvdg==