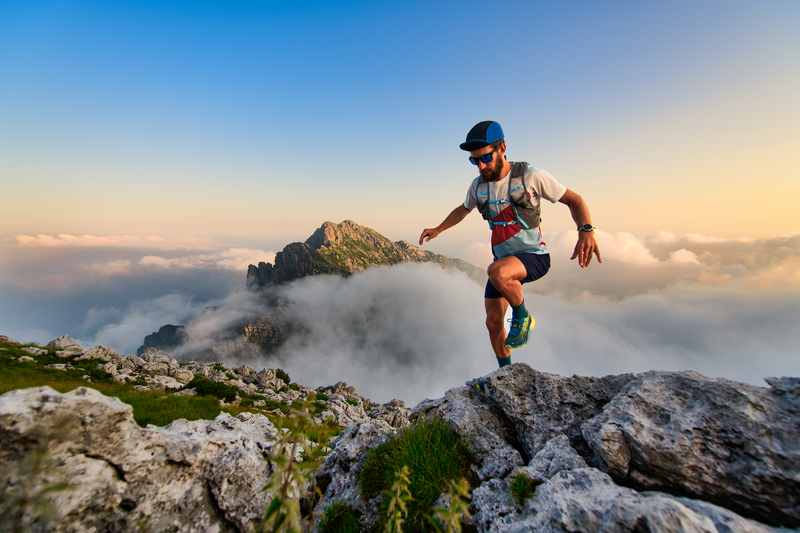
95% of researchers rate our articles as excellent or good
Learn more about the work of our research integrity team to safeguard the quality of each article we publish.
Find out more
ORIGINAL RESEARCH article
Front. Sustain. Food Syst. , 16 May 2023
Sec. Nutrition and Sustainable Diets
Volume 7 - 2023 | https://doi.org/10.3389/fsufs.2023.1182642
This article is part of the Research Topic Dietary Change Strategies for Sustainable Diets and their Impact on Human Health, volume II View all 11 articles
Background: Peas (Pisum sativum L.), the second largest edible bean in the world, have comprehensive and balanced nutrition. In China, peas are mainly used in the processing of starch and related products, during which a large amount of processing by-products—pea dregs—is produced. Because of its large particle size, coarse texture, and difficulty in storage, it is mostly discarded or used as feed, resulting in unnecessary waste.
Materials and methods: The preparation and simulated moving bed chromatographic purification process conditions of pea-resistant dextrin were optimized using pea production waste-pea residue as raw material, and structural characterization and functional properties of pea residue-resistant dextrin were analyzed.
Results: The results showed that the optimal preparation process conditions for pea-resistant dextrin were as follows: acid concentration 1.0%, acid addition 7.3%, treatment temperature 178.8°C, and treatment time 92.5 min. Subsequently, the pea-resistant dextrin content of 42.15 ± 0.16% was obtained. The optimal SSMB purification conditions were as follows: feed volume 455 g/h, feed volume 682 g/h, circulation volume 346 mL, outlet concentration 24.8 ± 0.2%, purity 99.35 ± 0.17%, and yield 91.08 ± 0.42%. The structural characterization revealed that pea-resistant dextrin had large and variable particle size and amorphous structure; the chemical bond or functional group differences between pea-resistant dextrin and pea starch were not significant; pea-resistant dextrin was a glucose-based dextran with a monosaccharide composition of 2.6% arabinose, 1.5% xylose, and 95.9% glucose, and its molecular weight was (601.1 ± 8.5) × 103 u. Functional characterization revealed that the RS content of pea-resistant dextrin was 92.35%, which had significantly slow digestive properties as well as hypoglycemic and hypolipidemic effects.
Conclusions: Using pea dregs to produce pea dregs resistant dextrin has low production cost and significant functional characteristics, which can be widely applied in the food industry.
Peas (Pisum sativum L.), the second largest edible bean in the world, have comprehensive and balanced nutrition (Huang et al., 2020). They have a high nutritional value, containing 33.4–47.5% starch, 24.3–30.4% protein, and 14.4–19.5% crude fiber (Gomes et al., 2018). In China, peas are mainly used in the processing of starch and related products, such as vermicelli, during which a large amount of processing by-products—pea dregs—is produced. Because of its large particle size, coarse texture, and difficulty in storage, it is mostly discarded or used as feed, resulting in unnecessary waste (Wu et al., 2020). The starch content of pea dregs is about 20% (Zhou et al., 2016), and if the starch in it can be processed to make a high-quality, high-value-added resistant dextrin, the utilization rate and added value of the peas can be improved significantly.
Resistant dextrin is a short-chain glucose polymer formed by high-temperature cleavage and condensation of starch as a white or pale-yellow powder, a non-sweet, low-calorie indigestible dextrin (Hobden et al., 2015). Resistant dextrin reduces the secretion of digestive tract hormones that regulate the activity of digestive enzymes in the intestine to alter and maintain the balance of the metabolic activity of the body, which promotes human health (Xie et al., 2017). It has the physiological functions of regulating blood sugar (Shigeru et al., 1999; Aliasgharzadeh et al., 2015) and fat metabolism (Basu and Ooraikul, 2010; Dong et al., 2015), improving the composition of human intestinal colonies (Le et al., 2003; Barczynska et al., 2010; Lanubile et al., 2012), and being a laxative (Emilien et al., 2018; Pérez-Calahorra et al., 2020). Also, as a major water-soluble dietary fiber with good processing characteristics, it can be widely used in dairy products, baby food, pasta products, meat products, and other fields and has a broad application prospect. The preparation of resistant dextrin is similar, and the structural characteristics, physiological functions, and other properties of resistant dextrin are related to the raw materials and their starch composition; therefore, the studies on the preparation of resistant dextrin are focused on the selection of raw materials. Shigeru et al. (1991) made resistant dextrin from potato starch. Kapusniak and Jane (2007) prepared maize-resistant dextrin from maize starch. Kamonrat et al. (2019) prepared cassava-resistant dextrin from cassava starch; Liu et al. (2022) prepared mung bean resistant-dextrin from mung bean starch. Zhang et al. (2020) prepared buckwheat resistant-dextrin from sorghum starch. Hitherto, the preparation of pea-resistant dextrin has not been reported. Thus, the present study was carried out to prepare pea dregs-resistant dextrin by acid thermal enzymatic method using pea dregs as raw material. Then, the dextrin was purified by simulated moving bed chromatography, and its physicochemical structure was analyzed by scanning electron microscopy, infrared spectroscopy, X-ray diffraction, gel chromatography-multi-angle laser light scattering method, and gas chromatography-mass spectrometry coupling, and it's in vitro digestibility, in vitro hypoglycemic effect, in vitro lipid-lowering, and other functional properties were also evaluated. Gas scattering and gas chromatography-mass spectrometry analyzed the physical and chemical structure. Therefore, the present study aimed to explore the methods of deep processing of bean starch waste and lay the foundation for the comprehensive utilization and industrialized production of bean residue.
Pea okara was supplied by Shuangta Food Co., L2.2.1td Yantai, Shandong, China. Pea okara was dried at 40°C, crushed, and screened (200 μm).
After impurity removal, the pea dregs were degreased with petroleum ether, extracted with 0.1 mol/L NaOH solution for 3 h, centrifuged at 3,550 × g for 10 min, and the supernatant was used to prepare protein. The precipitate was repeatedly washed with deionized water to remove the yellowish-brown substances in the upper layer of the precipitate until the starch slurry is white, then mixed with 1 mol/L hydrochloric acid to pH 7.0, filtered, dried at 30°C, crushed through 80 mesh sieve, and pea starch was prepared (Kou et al., 2017). Pea starch was dried at 90°C for 1 h and reduce the water content to below 5%, then spray with hydrochloric acid and pyrolysis at 160–200°C for 1–2 h; After completion, taken it out for natural cooling, add water to the pyrolysis product (pyrodextrin) to make a solution, used 1 mol/L NaOH to adjust it to pH 5.5–6.5, 0.5% high temperature resistance α-amylase reacted at 95°C for 1 h, and the 0.4% saccharifying enzyme reacted at 60°C, pH 4.0–4.5 for 2 h, and then concentrated in vacuum to obtain the crude pea resistant dextrin. The resistant dextrin was purified by simulated moving bed chromatography and its structure and function were determined (Li et al., 2020).
Weighed pea starch 1,000 g, added hydrochloric acid at the concentration of 0.6, 0.8, 1.0, 1.2, and 1.4%, respectively, add hydrochloric acid at the level of 4, 5, 6, 7, 8, 9, and 10% of raw material, pyrolysis temperature at 150, 160, 170, 180, and 190°C, and treatment time at the level of 80, 90, 100, 110, and 120 min, respectively, high temperature resistance α-amylase added was 0.5%, and the reaction was conducted at 95°C for 1 h. The saccharifying enzyme added 0.4%, reacted at 60°C for 2 h, and concentrated in vacuum to obtain crude resistant dextrin. Taking the content of resistant dextrin in pea as an index, the relationship diagram was drawn by using sigma plot to determine the best rotation test center level. On the basis of single factor test, the response surface method was used to optimize the extraction process. The content of pea resistant dextrin was Y, the acid concentration (%) was X1, the amount of acid added (%) was X2, the treatment temperature (°C) was X3, the treatment time (min) was X4, and the test factor level coding in Table 1.
Weighed 99K+310 resin (Dow Chemical Company, Midland, MI, U.S.A.) 200 mL(structural framework: styrene diethylene benzene, functional Group:-, homogeneous particle 310 μm), washed it repeatedly with deionized water to remove the damaged resin and impurities in the process, and soak it overnight with deionized water. The self-made preparation chromatography device (10 × 1,200 mm with thermal insulation function, equipped with six-way sample injection valve) was used for the preparation chromatography experiment. The resin was filled into the preparation chromatography column. After filling, the resin was continuously flushed with deionized water until the conductivity of the effluent was consistent with that of deionized water. During this period, the temperature was continuously increased, and the temperature is maintained to 60°C. The resin was ready for use after filling. The preparation chromatographic separation test was carried out with the feed concentration of resistant dextrin of 60%, the feed concentration of 10 mL, the column temperature of 60°C, and the elution flow rate of 1 BV/h. The concentration was determined by the saccharometer, and the purity of the sample was analyzed by HPLC. The single-column elution curve of pea resistant dextrin was drawn according to the test results (Li et al., 2016b).
The SSMB-6Z6L device (National Coarse Chemical Engineering Technology Research Center, Daqing, Heilongjiang, China) was used for the separation of SSMB purified resistant dextrin. The device has 6 chromatographic columns, which are connected in series. Each chromatographic column has to go through three steps of full in and full out (S1), large cycle (S2) and small cycle (S3) in one operation cycle day (Figure 1). Step 1: injected desorber D into the upper end of the first chromatographic column, and release AD (purity) at the lower end, and F (raw material) at the upper end of the third column at the interval, and release BD (resistant dextrin component) at the lower end of the fourth chromatographic column at the interval; Step 2: The material does not enter or exit the system, but only carries out a large cycle; Step 3: injected desorber D into the upper end of the first chromatographic column, and release BD (resistant dextrin component) at the lower end of the fourth chromatographic column in the interval Then switch to the next chromatographic column, carry out three steps in turn, and then cycle until the end of the test (Li et al., 2016a).
The molecular weight distribution was determined by liquid chromatography using the method of Wu et al. (2010) with slight modifications and a highly efficient size exclusion chromatography (SEC)-18-angle laser light scatter-differential refractive index detector coupled system, (Wyatt Company, Santa Barbara, CA, USA) with a size exclusion chromatography column (TSK G5000 PW 7.5 × 600 mm; Tosoh Company, Tokyo, Japan). The prepared sample (100 μL) was analyzed using ASTRA 6.1 software. The instrument was calibrated with bovine serum albumin, the mobile phase was 0.1 mol/L NaNO3 (containing 0.02% NaN3), the flow rate was 0.4 mL/min, the column temperature was 60°C, and the loading volume was 100 μL (Wu et al., 2010).
Refered to the method of Cao et al. (2021) and make some changes. The whole process of sample treatment requires nitrogen protection, reduction and acetylation, and GC-MS analysis. The chromatographic column is HP-5MS quartz capillary column (30 m × 0.25 mm, 0.25 μm). The monosaccharide composition of pea resistant dextrin was determined by qualitative analysis of monosaccharide according to the peak time of gas spectrum and the ion peak of mass spectrum.
A Ultra-High-Resolution Schottky Scanning Electron Microscope SU7000 (Hitachi High-Technologies, Tokyo, Japan) was used for analyzing and observing morphology of pea resistance dextrin. resistance dextrin granules homogeneously dispersed were fixed with electrically conductive adhesive, coated with gold using an ion sputter coater, observed using SEM, and representative photographs were taken (Wei et al., 2022).
The infrared spectrum was measured with a FTIR-1500 Fourier transform infrared spectrometer (Thermo Fisher Scientific, Madison, WI, USA). The sample and KBr were mixed and ground, and the vacuum tablet press was used for tablet pressing, and the infrared spectrum scanning was conducted in the range of 4,000 400 cm−1.
The prepared pea starch and purified pea resistant dextrin were dried and crushed, and then measured by Empyrean X-ray diffraction (XRD) (PANalytical B.V. Almelo, Eindhoven, Netherlands). The voltage of the instrument is 40 kV, the current is 40 mA, and the scanning range is diffraction angle (2θ)5~40°, step is 10.16 steps/s (Sankhon et al., 2013).
Refered to the methods of Li et al. (2015) for the determination of the concentration, purity, yield and resolution of resistant dextrin.
Refered to the method of Li et al. (2019) and make some changes. Determined the absorbance of the digestive solution at 540 nm, calculate the maltose content in the digestive system according to the standard curve of maltose, and then analyze the content of digestible starch (RDS), slow digestible starch (SDS), and resistant starch (RS) of pea starch and pea resistant dextrin.
Refered to the method of Ramkumar et al. (2010) and make some changes. Taken acarbose as control, determined the α-inhibition amylase activity and α-inhibition glucosidase activity of resistant dextrin.
The oil-holding capacity were determined by reference to Guo et al. (2018) with a slight modifications.
The adsorption of cholesterol was determined according to Peerajit et al. (2012) with slight modifications.
Microsoft Excel (Redmond, WA, USA) and SPSS software (IBM Corp, Armonk, NY, USA) were used for statistical analysis. SigmaPlot (Systat Corporation, Palo Alto, CA, USA) was used for image processing. All data were collected in triplicate and the average value was used for analysis.
The results showed that hydrochloric acid concentration, hydrochloric acid addition, treatment temperature, and treatment time had significant effects on the yield of resistant dextrin (P ≤ 0.05) (Figure 2). The yield of resistant dextrin in peas increased with the addition of hydrochloric acid; the yield of resistant dextrin was equivalent to the addition of hydrochloric acid >7%, the subsequent increase was not significant, and the yield was maximum with the addition of hydrochloric acid at 7%. Subsequently, the yield of resistant dextrin increased with rising treatment temperature, the increase of yield slowed down and tended to equilibrate with the treatment temperature >170°C, and the yield was maximum with the treatment temperature of 170°C. The yield of resistant dextrin increased with increasing treatment time, and the yield was maximum with the treatment time >100 min. The yield of resistant dextrin increased with increasing treatment time, and the yield of resistant dextrin increased slowly and equilibrated at >100 min. The maximum yield was obtained at 100 min of treatment time. Therefore, a response surface test was conducted with a concentration of 1% hydrochloric acid, 7% hydrochloric acid addition, a treatment temperature of 170°C, and a treatment time of 100 min as the center point.
Figure 2. Effect of different preparation conditions on the yield of pea resistant dextrin. (A) HCl concentration; (B) HCl addition; (C) Preparation temperature; (D) Preparation time.
Based on the results of the single-factor test, a response surface test was conducted using X1 (acid concentration), X2 (amount of acid added), X3 (treatment temperature), and X4 (treatment time) as independent variables and pea-resistant dextrin content as Y. The statistical analysis of the test results showed that the regression model R2 = 0.95, P < 0.01, and the F-value of the out-of-fit term was 0.21, P > 0.05, indicating a good fit for the model. The F-value of the primary term was 12.41, P < 0.01; the F-value of the interaction term was 4.07, P < 0.05, and the overall
F-value was 12.39, P < 0.01, indicating that each term affected the pea-resistant dextrin content to different degrees. Based on the experimental results, the regression equation Y = −4559.53 + 641.46X1 + 99.36X2 + 32.13X3 + 22.39X4 - 481.15 - 35.25X1X2 + 2.8X1X3 + 1.1 X1X4 - 4.7 + 0.03X2X3 - 0.07 - 0.1X3X4 - 0.03 was obtained using X1 (acid concentration), X2 (amount of acid added), X3 (treatment temperature), and X4 (treatment time) as independent variables and pea-resistant dextrin content as Y.
Based on the analysis of the regression equation, the standardized values for X1, X2, X3, and X4 were 0.126, 0.302, 0.886, and−0.747, respectively, and the parameters after conversion to non-standardized values were as follows: acid concentration (X1) 1.03%, acid addition (X2) 7.3%, treatment temperature (X3) 178.86°C, treatment time ah (X4) 92.53 min, the theoretical maximum value was 42.38%, and the content of pea-resistant dextrin was 42.15 ± 0.16% obtained from the validation test. The prepared pea-resistant dextrin mainly consisted of five peaks with three main substances: resistant dextrin (peak time 9.974 min), disaccharide (peak time 10.962 min), and glucose (peak time 12.585 min), in which the content of resistant dextrin was 42.1%.
The results of preparative chromatography of pea-resistant dextrin showed (Figure 3) that the retention time of resistant dextrin and heterosaccharides differed greatly, and the separation was calculated to reach 0.49. Although some overlapping parts could not be separated completely, the separation distance and time could be prolonged by simulated mobile chromatography, the amount of elution feed water could be increased, and a good separation effect could be achieved entirely through the optimization of the experiment.
Based on the results of the preparative chromatographic evaluation tests, the feed refraction of SSMB was determined to be 60%, and the temperature was 60°C. The technical parameters of the purification of resistant dextrin by the SSMB method were optimized (Table 2). Considering the feed volume, feed-water ratio, exit refraction, purity, and yield, the sixth group of tests showed better results than the other six groups. Hence, the best separation conditions were determined as follows: the feed volume was 455 g/h, the feed water volume was 682 g/h, and the circulation volume was 346 mL. At this time point, the exit refraction was 24.8 ± 0.2%, the purity reached 99.35 ± 0.17%, and the yield reached 91.08 ± 0.42%. The liquid chromatogram of the resistant dextrin fraction after separation by SSMB is shown in Figure 4.
The results of molecular weight determination (Figure 5) showed only a single peak on the DPF detection curves of both pea starch and pea-resistant dextrin, indicating that the molecular weights of both molecules were concentrated. The molecular weights (Mw) of pea starch and pea-resistant dextrin were (1465.4 ± 53.2) × 103 u and (601.1 ± 8.5) × 103 u, respectively.
Figure 5. Differential refraction detection spectrum of pea starch and peas resistance dextrin. (A) Pea starch; (B) peas resistance dextrin.
The monosaccharide composition of pea starch was more diverse than that of pea-resistant dextrin, containing six monosaccharides, including rhamnose 0.6%, arabinose 6.1%, xylose 4.3%, mannose 1.5%, glucose 82.3%, and galactose 5.2%. Conversely, the monosaccharide composition of resistant dextrin consisted of only three components: glucose as the main ingredient and a small amount of arabinose and xylose. The monosaccharide composition was 2.6% arabinose, 1.5% xylose, and 95.9% glucose. This might be due to the degradation of pea starch under acid heat conditions with glucose as the main body for repolymerization. Also, arabinose and xylose participate in this process, forming a pea-resistant dextrin polymer, while rhamnose, mannose, and galactose may not be involved in and are not detected in the pea-resistant dextrin.
The scanning electron microscope results of pea-resistant dextrin showed (Figure 6) that pea starch and purified pea-resistant dextrin had completely different morphological structures at the same magnification. The pea starch granules were small, with a slight particle difference, regular oval-shaped granules, and smooth granule surface, while the purified pea-resistant dextrin had larger and different-sized granules and irregular fragments, and the granule surface became rough due to an amorphous structure. This phenomenon could be due to the effect of acid and heat that breaks down the starch molecules, which are reassembled irregularly with different sizes of polymerization. This results in varied sizes and rough surfaces of particles of purified pea-resistant dextrin, favorable for the entry of water molecules, reflecting better solubility and smaller viscosity of pea-resistant dextrin than pea starch.
Figure 6. SEM images of pea starch and pea resistance dextrin. (a) Pea starch (×500); (b) pea starch (×5,000); (c) pea resistance dextrin (×500); (d) pea resistance dextrin (×5,000).
The results of the infrared spectra of pea-resistant dextrin (Figure 7) showed that pea starch and pea-resistant dextrin had absorption peaks near 2,900, 2,300, 2,000, and 1,600 cm−1. The peak shapes and positions of the characteristic peaks of both infrared spectra did not change significantly, only the size of some absorption peaks differed, which was related to the content of the corresponding chemical bonds. This finding indicated that the chemical bonds or functional groups of pea starch and pea-resistant dextrin had not changed significantly.
Figure 7. Fourier transform infrared spectra of pea starch and peas resistance dextrin. (A) Pea starch; (B) peas resistance dextrin.
The results of pea starch X-ray diffraction test showed (Figure 8A) that the crystalline type of pea starch was C-crystalline with 2θ at 15.40, 17.32, and 23.38°, respectively, and the crystallinity was 34.37% by fitting analysis. The X-ray diffractogram of pea-resistant dextrin showed (Figure 8B) that the diffraction peaks of pea-resistant dextrin are broad and not highly crystalline when viewed directly, and its 2θ is about 19°, which is typical of the amorphous spectrum, indicating that pea-resistant dextrin belongs to the amorphous state. This is due to the degradation of pea starch under acid heat conditions, following which the original crystalline structure of pea starch is destroyed; thus, the pea-resistant dextrin is a glucose-based dextran in the amorphous state.
Figure 8. The X-ray diffraction spectra of pea starch and peas resistance dextrin. (A) Pea starch; (B) peas resistance dextrin.
The content of RDS, SDS, and RS of pea starch was determined by simulated gastrointestinal fluid digestion: 81.77, 12.36, and 5.87%, respectively. The content of RDS, SDS and RS of pea-resistant dextrin was 5.37, 2.28, and 92.35%, respectively. The starch content of the resistant dextrins was significantly higher (P < 0.05) than that of the untreated starch.
The in vitro hypoglycemic function test results with pea-resistant dextrin (Figure 9) showed that the inhibition rate of α-glucosidase and α-amylase showed a significant increase in pea-resistant dextrin-dependent manner. This phenomenon indicated a positive linear correlation with the inhibition rate of α-glucosidase and α-amylase. The inhibition rates of α-glucosidase and α-amylase of pea-resistant dextrins were significantly lower than the corresponding inhibition capacity of acarbose (P < 0.05).
Figure 9. The inhibition of α-amylase and α-glucosidase. The black line represents α- Inhibition of amylase activity; The red line represents α- Inhibitory effect of glucosidase.
The in vitro lipid-lowering function of pea-resistant dextrin (Table 3) showed that pea-resistant dextrin had a marked lipid-lowering function. The cholesterol adsorption capacity of pea-resistant dextrin was 28.12 ± 1.58 mg/g at pH 7.0 and 22.50 ± 1.16 mg/g at pH 2.0. The cholesterol adsorption capacity of pea-resistant dextrin at pH 7.0 was significantly greater than that of pea-resistant dextrin at pH 2.0 (P < 0.05). The adsorption capacity of pea starch for lard and soybean oil was determined as 1.32 g/g and 0.76 g/g, respectively, the adsorption capacity of pea-resistant dextrin for both was 1.69 and 0.88 g/g, respectively, and the adsorption effect of pea-resistant dextrin for oil and grease was better than that of pea starch (P < 0.05).
Currently, the purity of the prepared resistant dextrin is about 40–50%, and purification is essential to reach the international standards of purity >95%. The common purification methods include microbial fermentation, enzymatic methods, and ethanol precipitation. Aboubacar et al. (2006) studied the effect of Brewer's yeast on the purification of resistant dextrin, and the dietary fiber content of purified resistant dextrin reached 90%; however, the microbial fermentation method has several by-products, complex operation, and high production costs, and is not suitable for industrial production. Zhang et al. (2020) purified sorghum-resistant dextrin by enzymatic method and achieved 88.23% purity. Liu et al. (2022) and Su et al. (2022) used the ethanol method for the purification of resistant dextrin, which showed that ethanol precipitation is still the most commonly used and effective method (Liu et al., 2022; Su et al., 2022). The purity of purified resistant dextrin by ethanol precipitation method reaches >95%, but requires several consecutive washes and complicated operation; the ethanol needs to be recycled, the production cost is high, and also serious pollution and safety risks are recorded. The current methods have problems of low product purity, high consumption, and high purification costs. In this study, SSMB chromatography was used for the purification of resistant dextrin, and the purity of the purified resistant dextrin was >99%, and the yield was >90%. This technique uses deionized water as the eluent, with high inlet and outlet concentrations and low production costs, the purity and yield of the product are high, and the industrial mass production can be automated and continuous (Ha et al., 2016).
Structural characterization revealed that the particles of pea-resistant dextrin were large and of different particle sizes and irregular fragments; the surfaces of the particles became rough and different as amorphous structures. The chemical bonds or functional groups of pea-resistant dextrin did not change significantly after acid heat treatment; the monosaccharide composition of pea-resistant dextrin was dominated by glucose and differed significantly (P < 0.05) from that of starch monosaccharides. Also, the molecular weight of pea starch pea-resistant dextrin decreased significantly (P < 0.05) after acid heat reaction, the glycosidic bonds were reorganized by heat breakage, and the corresponding chemical bond content changed, and the chemical bond content of anti-digestive enzyme hydrolysis increased, resulting in significant changes in physicochemical properties and the molecular weight and monosaccharide composition of resistant dextrin. In addition, the resistant starch content in the resistant dextrin was significantly higher (P < 0.05) compared to pea starch-resistant dextrin and exhibited in vitro hypoglycemic and hypolipidemic functions, which is consistent with the current findings (Liu et al., 2022; Su et al., 2022). The overall condensation and polymerization processes of resistant dextrin are stochastic, and its cleavage process is fixed. The α-1,4 glycosidic bond of starch is broken by amylase hydrolysis to form small molecules of glucose and disaccharide analogs, which are reconnected and polymerized by α-1,6 glycosidic bond to form resistant dextrin (Li W. et al., 2016). Digestive enzymes and human gastrointestinal fluids can act only on the α-1,4 glycosidic bond and not on this newly formed bond (Kong et al., 2020), leading to a significant decrease in the ratio of fast- and slow-digesting starch in resistant dextrin and a significant increase in the proportion of resistant starch. Moreover, pea-resistant dextrin exhibited inhibitory effects on α-glucosidase and α-amylase. Resistant dextrin is a glucan with a more complex branching structure than starch. Thus, the presence of these irregular structures may provide the resistant dextrin with an effect similar to acarbose in binding to α-glucosidase, inhibiting the activity of α-glucosidase and α-amylase (Jiang et al., 2022).
The present study was conducted to investigate the preparation, structural characterization, and functional properties of pea residue-resistant dextrin using pea production waste-pea residue as raw material. Based on a single factor, the processing conditions for the preparation of pea-resistant dextrin by acid thermal treatment were optimized by response surface methodology, and pea-resistant dextrin with purity >99% was obtained by preparative chromatography and simulated moving bed chromatography purification. The structural characterization revealed that the resistant dextrins have satisfactory processing properties and can be widely used in the dairy, beverage, noodle products, health food, meat products and other food industry. The functional properties of in vitro digestibility, hypoglycemia, and hypolipidemia were analyzed, and we found that pea-resistant dextrin has significantly slow digestibility as well as hypoglycemic and hypolipidemic effects. This study provided novel ideas for the comprehensive utilization of pea dregs, which significantly increased the added value of pea dregs processing and promoted the development of pea cultivation, production, deep processing, and other related industries.
The original contributions presented in the study are included in the article/supplementary material, further inquiries can be directed to the corresponding author.
LL and R-aC were responsible for the design, overall management of the entire study, and writing review and editing. YL, TH, XL, CS, and CL provided the validation and formal analysis. YL, TH, and CL analyzed the data. R-aC supervised, writing—review and editing, and funding acquisition. All authors have read, agreed to the publishing of the current version of the manuscript, contributed to the article, and approved the submitted version.
The present study was supported by the Scientific Research Initiation Fund for Completion and Introduction of Talents (XDB202213) of Heilongjiang BaYi Agricultural University.
The authors declare that the research was conducted in the absence of any commercial or financial relationships that could be construed as a potential conflict of interest.
All claims expressed in this article are solely those of the authors and do not necessarily represent those of their affiliated organizations, or those of the publisher, the editors and the reviewers. Any product that may be evaluated in this article, or claim that may be made by its manufacturer, is not guaranteed or endorsed by the publisher.
Aboubacar, O. B., Tang, J., and Qian, H. (2006). Yeast application for de-salting fibersol-2. Int. J. Food Sci. Technol. 41, 997–1001. doi: 10.1111/j.1365-2621.2006.01064.x
Aliasgharzadeh, A., Dehghan, P., Gargari, B., and Asghari-Jafarabadi, M. (2015). Resistant dextrin, as a prebiotic, improves insulin resistance and inflammation in women with type 2 diabetes: a randomised controlled clinical trial. Br. J. Nutr.113, 321–330. doi: 10.1017/S0007114514003675
Barczynska, R. K., Jochym, K., Slizewska, K., Kapusniak, K., and Libudzisz, Z. (2010). The effect of citric acid-modified enzyme-resistant dextrin on growth and metabolism of selected strains of probiotic and other intestinal bacteria. J. Funct. Foods. 2, 126–133. doi: 10.1016/j.jff.2010.03.002
Basu, T. K., and Ooraikul, B. (2010). Lipid-lowering effects of dietary fiber. J.Clin.Biochem.Nutr. 18, 1–9. doi: 10.3164/jcbn.18.1
Cao, R. A., Ji, R. X., Tabarsa, M., Zhang, J. Q., and You, S. G. (2021). Purification, characterization and immunostimulatory effects of polysaccharides from Anemarrhena asphodeloides rhizomes. Int. J. Biol. Macromol.172, 550–559. doi: 10.1016/j.ijbiomac.2021.01.088
Dong, J. L., Zhu, Y. Y., Li, L., Lin, J., and Shen, R. L. (2015). Anti-obesity effect of oat dietary fiber on high-fat diet-induced obese mice. J. Chin. Cereals Oils Assoc. 30, 24–29. doi: 10.3969/j.issn.1003-0174.2015.09.005
Emilien, C. H., Zhu, Y., Hsu, W. H., Williamson, P., and Hollis, J. H. (2018). The effect of soluble fiber dextrin on postprandial appetite and subsequent food intake in healthy adults. Nutrition. 47, 6–12. doi: 10.1016/j.nut.2017.08.016
Gomes, D. D. S., Cordoba, L. D. P., Rosa, L. S., Spier, M. R., Schnitzler, E., Waszczynskyj, N., et al. (2018). Thermal, pasting properties and morphological characterization of pea starch (Pisum sativum L.), rice starch (Oryza sativa) and arracacha starch (Arracacia xanthorrhiza) blends, established by simplex-centroid design. Thermochim. Acta. 662, 90–99. doi: 10.1016/j.tca.2018.02.011
Guo, Z. W., Ma, P., Diao, J. J., Li, C. Y., Quan, Z. G., Man, Y. G., et al. (2018). Physicochemical and adsorption properties of ultramicro insoluble dietary fiber from soybean hull. Food Sci. 32, 106–112. doi: 10.7506/spkx1002-6630-201805017
Ha, Z. R., Ma, W. Y., Shen, C. J., Shen, Q., and Ma, J. Y. (2016). The application of L-tryptophan separation by SSMB technology. Bull. Ferment. Sci. Technol. 45, 165–169. doi: 10.16774/j.cnki.issn.1674-2214.2016.03.008
Hobden, M. R., Guérin-Deremaux, L., Rowland, I., Gibson, G. R., and Kennedy, O. B. (2015). Potential anti-obesogenic properties of non-digestible carbohydrates: specific focus on resistant dextrin. Proceedings of the Nutrition Society. 74, 258–267. doi: 10.1017/S0029665115000087
Huang, Y. Y., Wu, S. H., Li, F., Liu, B. S., Wang, X. Y., Ma, C. G., et al. (2020). Effect of extrusion on swelling and water holding capacity of insoluble dietary fiber from pea residue. J. Shandong Univ. Technol. 34, 59–64. doi: 10.13367/j.cnki.sdgc.2020.06.012
Jiang, C. X., Wang, R., Liu, X. L., Wang, J. T., Zheng, X. Q., and Zuo, F. (2022). Effect of particle size on physicochemical properties and in vitro hypoglycemic ability of insoluble dietary fiber from corn bran. Front. Nutr. 9:951821. doi: 10.3389/fnut.2022.951821
Kamonrat, T., Kuakarun, K., and Kanitha, T. (2019). In-depth study of the changes in properties and molecular structure of cassava starch during resistant dextrin preparation. Food Chem. 297, 1–7. doi: 10.1016/j.foodchem.2019.124996
Kapusniak, J., and Jane, J. L. (2007). Preparation and characteristics of enzyme-resistant pyrodextrins from corn starch. Polish J. Food Nutr. Ences.57, 261–265. doi: 10.1093/jmedent/22.2.130
Kong, H., Yu, L., Gu, Z., Li, C., and Li, Z. (2020). An innovative short-clustered maltodextrin as starch substitute for ameliorating postprandial glucose homeostasis. J. Agric. Food Chem. 69, 354–367. doi: 10.1021/acs.jafc.0c02828
Kou, F., Kang, L. J., Ning, D. X., Xia, T. T., Shen, M., Wang, W. H., et al. (2017). Effects of fermentation on molecular structure and pasting properties of millet starch. Food Sci.38, 36–42. doi: 10.7506/spkx1002-6630-201712006
Lanubile, A., Bernardi, J., Marocco, A., Logrieco, A., and Paciolla, C. (2012). Differential activation of defense genes and enzymes in maize genotypes with contrasting levels of resistance to Fusarium verticillioides. Environ. Exp. Bot. 35, 39–46. doi: 10.1016/j.envexpbot.2011.12.006
Le, B. G., Michel, C. D., Blottière, H. M., and Cherbut, C. J. (2003). Raw potato starch and short-chain fructo-oligosaccharides affect the composition and metabolic activity of rat intestinal microbiota differently depending on the caecocolonic segment involved. J. Appl. Microbiol. 94, 312–320. doi: 10.1046/j.1365-2672.2003.01836.x
Li, L. Y., Jia, P. Y., Li, C. Y., and Zhang, L. P. (2016a). Efficient purification technique of galacto-oligosaccharides using simulated moving bed chromatography. J. Chin. Inst. Food Sci. Technol. 16, 138–145. doi: 10.16429/j.1009-7848.2016.03.019
Li, L. Y., Li, C. Y., Yu, W., Jia, P. Y., Cao, L. K., Zhang, L. P., et al. (2016b). Studies on adsorption thermodynamics and kinetics of fructose syrup separated by ZG106Ca2+ resin. J. Chin. Inst. Food Sci. Technol. 16, 62–69. doi: 10.16429/j.1009-7848.2016.11.009
Li, L. Y., Liu, W. X., Li, C. Y., and Niu, G. C. (2020). High efficient purification technique and molecular characteristics of the phaseolus radiates L. Resistant Dextrin. J. Chin. Inst. Food Sci. Technol. 10, 134–141. doi: 10.16429/j.1009-7848.2020.10.016
Li, L. Y., Song, D. W., Sun, R., Li, C. Y., Li, H. F., Wang, X. Q., et al. (2015). Research on simulated moving bed chromatography purification of dextrose mother liquid. J. Nuclear Agric. Sci. 29, 1970–1978. doi: 10.11869/j.issn.100-8551.10,1970.
Li, W. W., Li, C. M., Gu, Z. B., Qiu, Y. J., Cheng, L., Hong, Y., et al. (2016). Retrogradation behavior of corn starch treated with 1,4-α-glucan branching enzym. Food Chem. 203, 308–313. doi: 10.1016/j.foodchem.2016.02.059
Li, Z., Ai, L. Z., Ding, W. Y., Zhang, X. D., and Zhang, H. (2019). Inhibitory effects of soluble dietary fibers on the in vitro digestion of corn starch. Sci. Technol. Food Industry. 40, 1–6. doi: 10.13386/j.issn1002-0306.2019.19.001
Liu, D. Z., Wang, W. H., Quan, Z. G., Zhao, S. T., Wu, Y. J., Wang, Y. F., et al. (2022). Study on structure characterization and anti digestion properties of mung bean resistant dextrin. Sci. Technol. Food Industry. 43, 119–125. doi: 10.13386/j.issn1002-0306.2021090154
Peerajit, P., Chiewchan, N., and Devahastin, S. (2012). Effects of pretreatment methods on health-related functional properties of high dietary fibre powder from lime okaras. Food Chem. 132, 1891–1898. doi: 10.1016/j.foodchem.2011.12.022
Pérez-Calahorra, S., Lamiquiz-Moneo, I., Marco-Bened,í, V., Bea, A. M., Fumanal, A. J., Prieto-Martín, A., et al. (2020). Effect of an alcohol-free beer enriched with isomaltulose and a resistant dextrin on insulin resistance in diabetic patients with overweight or obesity. Clin. Nutr. 39, 475–483. doi: 10.1016/j.clnu.2019.02.025
Ramkumar, K. M., Thayumanavan, B., Palvannan, T., and Rajaguru, P. (2010). Inhibitory effect of Gymnema Montanum leaves on α-glucosidase activity and α-amylase activity and their relationship with polyphenolic content. Med. Chem. Res. 19, 948–961. doi: 10.1007/s00044-009-9241-5
Sankhon, A., Yao, W. R., Amadou, I., Wang, H., Qian, H., and Sangare, M. (2013). Effect of pyrodextrinization, crosslinking and heat- moisture treatment on in vitro formation and digestibility of resistant starch from african locust bean (Parkia biglobosa). Trop. J. Pharm. Res. 12, 173–179. doi: 10.4314/tjpr.v12i2.7
Shigeru, W., Mitsuko, S., Yoshiki, N., Ohkuma, K., and Matsuoka, A. (1991). Effect of indigestible dextrin on cholesterol metabolism in rat. J. Japanese Soc. Nutr. Food Sci. 44, 471–478. doi: 10.4327/jsnfs.44.471
Shigeru, W., Yuka, K., Seiki, N., and Akira, M. (1999). Effects of indigestible dextrin on postprandial rise in blood glucose levels in man. Japan. Assoc. Dietary Fiber Res. 3, 13–19.
Su, Y. T., Wang, W. H., Wei, C. H., Wu, Y. J., Wang, Y. F., Zhao, S. T., et al. (2022). Optimization of preparation conditions and structure characterization of buckwheat resistant dextrin. J. Chin. Cereals Oils Assoc. 37, 85–91. doi: 10.3969/j.issn.1003-0174.2022.07.013
Wei, C. H., Ge, Y. F., Zhao, S. T., Liu, D. Z., Jiliu, J. L., Wu, Y. J., et al. (2022). Effect of fermentation time on molecular structure and physicochemical properties of corn ballast starch. Front. Nutr. 9:885662. doi: 10.3389/fnut.2022.885662
Wu, H. B., Yu, J. W., Wu, C. L., Li, Y., Jiang, L. Z., Teng, Y., et al. (2020). Cavitation microjet effects on structural and functional properties of okara dietary fiber. Food Sci. 41, 94–99. doi: 10.7506/spkx1002-6630-20181119-215
Wu, M. Y., Xu, S.m, Zhao, J.h, Kang, H., et al. (2010). Physicochemical characteristics and anticoagulant activities of low molecular weight fractions by free-radical depolymerization of a fucosylated chondroitin sulphate from sea cucumber Thelenata ananas. Food Chem. 122, 716–723. doi: 10.1016/j.foodchem.2010.03.042
Xie, F., Li, M., Lan, X. H., Zhang, W., and Gong, S. X. (2017). Modification of dietary fibers from purple-fleshed potatoes (Heimeiren) with high hydrostatic pressure and high pressure homogenization processing: a comparative study. Innov. Food Sci. Emerg. Technol. 42, 157–164. doi: 10.1016/j.ifset.2017.05.012
Zhang, T., Li, J. Y., An, S. S., Dong, J. L., and Shen, R. L. (2020). Optimization of sorghum resistant dextrin preparation process and structure characterization. Food Sci. Technol. 45, 232–237. doi: 10.13684/j.cnki.spkj.2020.04.041
Keywords: pea pomace, resistant dextrin, preparation, purification, simulated moving bed chromatography, structural characterization, functional properties
Citation: Li L, He T, Ling Y, Li X, Sui C, Cao R-a and Li C (2023) Preparation, structure characterization and functional properties of pea dregs resistant dextrin. Front. Sustain. Food Syst. 7:1182642. doi: 10.3389/fsufs.2023.1182642
Received: 09 March 2023; Accepted: 24 April 2023;
Published: 16 May 2023.
Edited by:
Fatih Ozogul, Çukurova University, TürkiyeReviewed by:
Sunil C. K., Indian Institute of Food Processing Technology, IndiaCopyright © 2023 Li, He, Ling, Li, Sui, Cao and Li. This is an open-access article distributed under the terms of the Creative Commons Attribution License (CC BY). The use, distribution or reproduction in other forums is permitted, provided the original author(s) and the copyright owner(s) are credited and that the original publication in this journal is cited, in accordance with accepted academic practice. No use, distribution or reproduction is permitted which does not comply with these terms.
*Correspondence: Chaoyang Li, bHp5ODg1NDAwQDE2My5jb20=
Disclaimer: All claims expressed in this article are solely those of the authors and do not necessarily represent those of their affiliated organizations, or those of the publisher, the editors and the reviewers. Any product that may be evaluated in this article or claim that may be made by its manufacturer is not guaranteed or endorsed by the publisher.
Research integrity at Frontiers
Learn more about the work of our research integrity team to safeguard the quality of each article we publish.