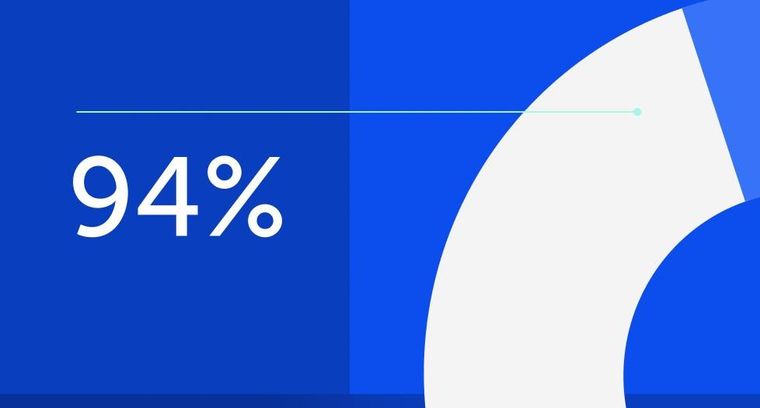
94% of researchers rate our articles as excellent or good
Learn more about the work of our research integrity team to safeguard the quality of each article we publish.
Find out more
ORIGINAL RESEARCH article
Front. Sustain. Food Syst., 31 July 2023
Sec. Sustainable Food Processing
Volume 7 - 2023 | https://doi.org/10.3389/fsufs.2023.1174597
This article is part of the Research TopicFermented-Based Foods As Sustainable Alternative Sources To Meet Future Demand For ProteinView all 8 articles
Introduction: Industrial applications of lentil (LP) and quinoa (QP) proteins are limited due to their relatively poor water solubility. In this study, a combination of protein-protein interaction (PPI) and fermentation was used to improve the functionality and nutritional value of LP by conjugating them with QP. The reaction conditions between LP and QP for producing these conjugates were established.
Methods: The ratio of LP to QP was equal (50:50), and complexation was carried out at 25°C for 60 min. Fermentation of the solubilized LP-QP complexes (1%, w/v) for 5 days at 25°C with water kefir (5%, v/v) was carried out to enhance the protein quality and functionality of the LP-QP complexes.
Results: The combined technique significantly enhanced protein digestibility, decreased the proportion of α-helices in the protein structure in favor of random coil components, and improved the phenolic content of the LP-QP complexes. Digestibility increased to 87%, up from 76% for unfermented LP-QP. Moreover, the LP-QP complexes produced using the combined technique generated a highly nutritional protein with a reduced saponin content.
Conclusion: This research revealed that a combination of PPI and water kefir fermentation significantly enhances the nutritional and functional quality of LP, creating new opportunities for leveraging the growing popularity of plant-based proteins into high-value industrial applications.
• - Lentil and quinoa protein complexes were prepared using a dual treatment approach.
• - A combined dual treatment (pH cycling and water kefir fermentation) was proposed.
• - The resulting protein complexes can be excellent alternatives to animal-based proteins.
• - Fermentation plays an important role in the modification of nutrient and non-nutrient compounds.
Plant-based proteins have gained steady popularity recently due to concerns about environmental, ethical, and health issues associated with animal-based proteins such as milk, eggs, and meat. Lentil (Lens culinaris) is the most common leguminous plant in the world and is extensively employed as a commercial source of food components (Jarpa-Parra et al., 2014). Lentil proteins (LP) are some of the promising plant-based proteins due to their low cost, low allergenicity, high environmental sustainability, and high nutritional value (Alrosan et al., 2022a). Another protein, the quinoa protein (QP), found in a pseudocereal from the Amaranthaceae family, is considered a trend in food applications due to its high nutritional value since QP contains all essential amino acids and is deemed non-allergenic (Lingiardi et al., 2022). However, several barriers exist to using LP as a primary protein source in diets, such as low protein digestibility (Alrosan et al., 2021) and low water solubility (Alrosan et al., 2022b). In addition, our understanding of the relationships between the basis of protein quality and the functional performance of LP and QP remains limited.
To address these issues, scientists have aimed to improve the functionality of plant proteins using different techniques involving enzymatic, chemical, and physical processes. Many researchers have successfully enhanced the functionality of plant proteins using protein–protein interactions (PPI), including the solubility of rice proteins (Wang et al., 2019) and LP (Alrosan et al., 2021, 2022b) and the emulsifying properties of wheat gluten proteins (He et al., 2020). These results stem from the altered secondary and tertiary protein structures caused by structural interactions and the molecular forces governing PPI, such as hydrogen bonds, hydrophobic interaction, and electrostatic interaction. A complex series of alterations to these molecular forces, which are initiated by the structural interactions between molecules on the surface of the proteins, lead to the modification of the protein higher order structure and secondary structure components (α-helix, β-turn, β-sheet, and random coil).
Furthermore, fermentation has been shown to significantly enhance the nutritional value and protein quality of plant proteins. For example, fermentation improved the digestibility of LP (Alrosan et al., 2021), reduced the levels of anti-nutritive factors present with soya-whey proteins (Alrosan et al., 2023), and enhanced the digestibility of pea proteins (Çabuk et al., 2018). Some fermenting microorganisms and their enzymes can degrade the ester linkages among carbohydrates, proteins, and naturally occurring non-nutrients such as phenolic compounds (Alrosan et al., 2022a). Among the various types of fermentation, water kefir is considered an excellent source of lactic acid bacteria (Leuconostoc mesenteroides ssp., Lacticaseibacillus casei, and Lentilactobacillus hilgardii), acetic acid bacteria (e.g., Acetobacter lovaniensis, Acetobacter tropicalis, and Gluconobacter liquefaciens), and yeasts (e.g., Saccharomyces cerevisiae) (Lynch et al., 2021).
Dual-treatment techniques, i.e., the combination of two different treatments, such as dehydration, enzyme hydrolysis, vacuum microwave, and ultrasonic treatments, have been reported to enhance the functional properties of plant-based proteins (Yan et al., 2021; Yen and Pratap-Singh, 2021). Zhao et al. (2022) showed that the combination of high-pressure treatment and ultrasound-assisted Maillard reaction increased the water solubility of pea protein isolates by over 80%. The objective of the present study was 2-fold: (i) to prepare a protein complex made of crude lentil proteins (CLP) and quinoa protein concentrate (QPC) using a combination of PPI (pH cycling technique) and water kefir fermentation and (ii) to investigate how this dual treatment may improve the protein quality and functionality of LP to boost its industrial applications as an alternative to animal-based proteins. The fermented LP-QP complexes were assessed for protein digestibility, protein secondary structure, phenolic profile, and total saponin content. Microorganism counts and pH were monitored to confirm the growth of yeasts, lactic acid, and acetic acid bacteria during fermentation.
Kefir grains, lentil seeds (Lens culinaris), quinoa seeds (Chenopodium quinoa), and brown sugar (one batch each) were purchased from a local food market in Malaysia. All the chemicals and reagents were purchased from Sigma-Aldrich Inc. (St. Louis, MO, USA), including pepsin, pancreatin, Folin–Ciocalteu reagent, fructose, glucose, sucrose, dextrose sorbitol mannitol (DSM) agar, potato dextrose agar, de Man, Rogosa and Sharpe (MRS) agar, oleanolic acid, phenolic compound standards, and HPLC grade solvents. The thermosensitive samples were transferred to the lab in a temperature-controlled container and stored at 4°C.
CLP was prepared in accordance with the technique described by Jarpa-Parra et al. (2014). Several batches were prepared, then pooled and mixed before further use. Lentil seeds were washed twice and left at room temperature at 25°C for 1 day to reduce the saponin content (Abugoch et al., 2008). A rotating mill (Retsch, ZM 300, Haan, Germany) was used to grind the lentil seeds into fine flour with a particle size of <0.5 mm. The resulting flour was collected in plastic bags that were sealed and stored at 4°C until protein extraction by sequential precipitation. Lentil flour and distilled water were combined at a ratio of 1:10 (w/v). The slurry was stirred using a digital magnetic stirrer (JoanLab, SH-4, Fristaden Lab, NV, USA) and its pH was adjusted to pH 9.5 using NaOH (0.1 M). Stirring (1,000 rpm) was continued for 2 h at 40°C with pH monitoring every 30 min, followed by centrifugation (Kubota, S700TR, Tokyo, Japan) at 8,500 ×g for 15 min at 23°C. The supernatant was obtained, acidified to pH 4.2 with HCl (0.1 M), then kept overnight before centrifugation at 1,590 ×g for 30 min. The precipitate was collected and lyophilized (Büchi, R-220, Flawil, Switzerland). The crude protein content of the lyophilized CLP (61.78% ± 2.10) was determined using the AOAC Method 930.29, N × 6.25 (AOAC, 2012). The lyophilisate was ground, placed in plastic bags, sealed, and stored at 4°C until complexation with QPC.
QPC was prepared as previously described (Alrosan et al., 2023). Several batches were prepared, then pooled and mixed before use. In brief, the quinoa seeds were washed multiple times with distilled water until no visible foam remained on the water. The seeds were dried (50°C) and ground using a ZM 300 rotating mill. Defatted quinoa flour was prepared by mixing the flour with hexane at a ratio of 10:1 (w/v) with continuous stirring for 24 h using an SH-4 digital magnetic stirrer, followed by air-drying at 21°C. To extract the proteins, distilled water was added to the defatted quinoa flour at a ratio of 10:1 (v/w) and the pH of the suspension was adjusted to pH 9.0 using 0.1 M NaOH with continuous stirring (SH-4 digital magnetic stirrer) at 40°C. The suspension was then centrifuged at 9,000 ×g for 15 min, the supernatant was collected, acidified to 5.0 using HCl (0.1 M), and kept overnight to allow the protein to precipitate before centrifugation at 9,000 ×g for 20 min. The precipitate was collected and lyophilized. The crude protein content (N × 6.25) of the lyophilized QPC (82.21% ± 1.43) was determined according to the AOAC Method 930.29 (AOAC, 2012). The lyophilisate was pulverized before being sealed in plastic bags and stored at 4°C until complexation with CLP.
Complexation of CLP and QPC was performed as reported by Alrosan et al. (2022b). Equal amounts of CLP and QPC were mixed in distilled water at 1% (v/w), and the pH of the mixture was adjusted to pH 12 with NaOH (0.05 M). The protein dispersion was stirred at 1,000 rpm with a magnetic stirrer at room temperature (25°C). After 1 h, the pH of the dispersion was readjusted to 7.0 with HCl (0.05 M), followed by centrifugation at 7,000 ×g for 10 min. The supernatant was collected and lyophilized. The lyophilisate was pulverized, sealed in plastic bags, and stored at 4°C until fermentation with water kefir.
Water kefir was produced by blending the kefir grains with brown sugar in distilled water at a ratio of 5:10:100 (w/w/v), respectively. The mixture was incubated for 3 days at 25°C in a chamber incubator (Corepoint, PRF122WWW/0CAD, Summerville, SC, USA) with shaking every 12 h to keep the kefir grains viable. At the end of the incubation period, a sterile sieve was utilized to separate the kefir grains. The filtrate (water kefir) was collected for fermentation. Water kefir fermentation of LP-QP complexes was carried out in triplicate. For each replicate, the samples were prepared by mixing the lyophilized LP-QP complexes with water kefir in distilled water at a ratio of (1:5:100, w/v/v), respectively, and incubated for 5 days at 25°C (Corepoint chamber incubator, PRF122WWW/0CAD) with shaking every 12 h. Aliquots were collected every 24 ± 1 h for further analyses (Section 2.5) which were performed in triplicate, except for microbial counts which were determined in duplicate. Samples collected on Day 0, immediately before fermentation, were labeled as unfermented LP-QP complexes.
The pH and total soluble solids (TSS, expressed as °Brix) of the unfermented and fermented samples were measured daily with a pH meter (MRC Laboratory-Instruments, INE-I500T, Essex, UK) and a digital refractometer (Rudolph Research Analytical, J257, Hackettstown, NJ, USA), respectively. Total saponin content was determined by absorbance using the perchloric acid-vanillin-glacial acetic acid method, as previously reported (Alrosan et al., 2023), and expressed as mg oleanolic acid equivalents (OAE) per 100 g.
Lactic acid bacteria (LAB), acetic acid bacteria (AAB), and yeast counts in both unfermented and fermented LP-QP samples were determined using the spread plate method. The microbial analyses were conducted in duplicate every 24 h throughout fermentation. Each sample was serially diluted and then plated by distributing 1 mL of each serial dilution in duplicate over the surface of the appropriate growth media. Yeasts, AAB, and LAB were plated on potato dextrose agar, DSM agar, and MRS agar, respectively.
The protein digestibility of the LP-QP complexes was determined as described by Almeida et al. (2015). Protein samples (0.25 g) were mixed in HCl (15 mL, 0.1 M) and pepsin (1.5 mg/mL), followed by incubation in a water bath (Munro, WBH-100, Essex, UK) at 37°C. After 180 min, 7.5 mL of 0.5 M NaOH containing 0.005 M sodium azide was added to the mixtures to prevent microbial growth. Then, 10 mg of pancreatin and 10 mL of 0.2 M phosphate buffer (pH 8.0) were added and mixed, and the suspensions were incubated at 37°C for 24 h (Corepoint chamber incubator) before centrifugation at 10,000 ×g for 20 min. The nitrogen content of the samples and the collected supernatants was determined using the Kjeldahl method (AOAC, 2012). Protein digestibility, expressed in %, was calculated according to Equation (1).
where NT and NS are the total nitrogen content of the LP-QP complexes before and after centrifugation, respectively, and NB is the nitrogen content in the supernatant of the blank.
Glucose, fructose, and sucrose concentrations in the suspensions of fermented and unfermented LP-QP complexes were determined by HPLC (Alrosan et al., 2021). The samples (1 mL) were diluted 2× with deionized water, vortexed for 10 min, and centrifuged at 15,000 ×g for 10 min. The supernatants were collected and filtered (0.45 μm Whatman membrane) before injection (20 μL) in an HPLC system equipped with a refractive index detector (Agilent, 1200 Series, East Brunswick, NJ, USA) and a Cosmosil Sugar-D HPLC column (4.6 mm × 250 mm) (Nacalai Tesque Inc., Kyoto, Japan) to separate the sugars at 40°C at a flow rate of 1.2 mL/min. The mobile phase was composed of water and acetonitrile in a 25:75 (v/v) ratio. The calibration curves of fructose, glucose, and sucrose were used to quantify each sugar in the samples.
The total phenolic content (TPC) of LP-QP complexes was measured using the Folin–Ciocalteu method as previously reported (Alrosan et al., 2021). Protein samples (100 μL) were combined with distilled water (8.4 mL) and Folin–Ciocalteu reagent (500 μL) in 50-mL test tubes followed by vortexing for 4 min. Sodium carbonate (1 mL, 5%) was added, and the mixtures were thoroughly mixed by vortexing and then stored in a dark environment for 1 h before reading the absorbance at 725 nm (Shimadzu UV-Vis spectrophotometer, UV-3600, Kyoto, Japan). TPC was expressed in mg gallic acid equivalents (GAE) per 100 g.
The individual phenolic compounds (caffeic acid, catechin, chlorogenic acid, epicatechin, ferulic acid, gallic acid, quercetin, rutin, sinapic acid, and syringic acid) in LP-QP complexes were quantified by HPLC according to Liu et al. (2021). In brief, 1 mL of the protein dispersions and 8 mL of methanol were mixed by vortexing before being subjected to ultrasonication at 35°C for 3 min or until the residues turned colorless. The supernatants were collected and filtered (0.45 μm) before being measured using an Agilent 1200 Series HPLC system equipped with a UV detector (Agilent, NJ, USA) and a Plus C18 column (4.6 × 250 mm). The injection volume and flow rate were set at 40 μL and 0.7 mL/min, respectively. The absorbance was read at 272 nm for caffeic acid, catechin, chlorogenic acid, epicatechin, ferulic acid, gallic acid, quercetin, and rutin, or 254 nm for sinapic acid and syringic acid.
The secondary structural components of the LP-QP complexes were quantified using Fourier transform infrared (FTIR) as described by Alrosan et al. (2021). Pre-weighed LP-QP lyophilisate (60 mg) was carefully inserted in the FTIR spectrometer (Malvern, MultiTectTM, Burlington, Canada) for scanning. The standardized data from the amide I area were subjected to a baseline correction. The analyzed FTIR spectra were measured between 1,600 and 1,700 cm−1 at an interval of 4 cm−1. The percentages of protein secondary components, specifically α-helix, β-turn, β-sheet, and random coil, were used to express the results (Zheng et al., 2017).
Data are reported as means of three independent replications unless otherwise indicated. Statistical analyses were conducted with SPSS software version 23.0 (IBM, Chicago, IL, USA). Duncan's multiple range test and one-way analysis of variance (ANOVA) were performed to identify statistically significant differences (P < 0.05) between the means.
As shown in Table 1, the pH of the LP-QP dispersions decreased significantly (P < 0.05) during fermentation with water kefir, from 6.61 initially to 3.73 on Day 5, which can be attributed to the release of organic acids, including lactic acid by LAB. The pH of the protein dispersions decreased rapidly over the first 24 h, reaching 3.90 on Day 2, which probably coincided with the exponential growth phase of the bacteria found in water kefir. Lynch et al. (2021) reported that LAB present in the water kefir significantly reduce the pH during the fermentation of protein substrates, while also breaking down proteins into smaller molecules, including short- and medium-sized peptides and amino acids. Similarly, Azi et al. (2020) and Alrosan et al. (2021) reported that LAB (Lactobacillus genus) led to increased acidity during water kefir fermentation. In agreement with our findings, the pH of water kefir is often below 4.0 after fermentation due to the production of both lactic and acetic acids (Laureys et al., 2019; Jia et al., 2021). Thus, the possibility of unfavorable microbial activity is limited (Fessard et al., 2017; Alrosan et al., 2022b). Protein degradation into short peptides, amino acids, and NH also plays a role in lowering the pH (Tepari et al., 2020).
Table 1. Changes in the pH, total soluble solids (TSS, °Brix), protein digestibility (%), total saponin content (TSC, mg OAE/100 g), and sugar profile (g/L) of unfermented (day 0) LP-QP complexes and water kefir fermented LP-QP complexes (days 1–5).
Similarly, the TSS of the fermented LP-QP dispersions were significantly decreased on Day 2, from 1.60 to 1.10 °Brix, and were further reduced until the final stage of the fermentation reached 0.90 °Brix on Day 5 (Table 1). These findings are consistent with the utilization of TSS (e.g., sugars and amino acids) by the fermenting microorganisms to support their growth. Indeed, the activity of bacterial enzymes is one of the main reasons for the drop in TSS during fermentation. Similarly, the TSS of fermented soy-whey proteins dropped from 9.20 to 4.43 °Brix during water kefir fermentation (Tu et al., 2019), while a reduction from 1.88 to 1.45 °Brix was found after water kefir fermentation of soymilk proteins (Dos Santos et al., 2019).
LAB (e.g., Liquorilactobacillus hordei, Lacticaseibacillus casei, Lentilactobacillus parafarraginis, and Liquorilactobacillus satsumensis), AAB (e.g., Acetobacter lovaniensis, Acetobacter fabarum, Acetobacter okenawensis, Gluconacetobacter liquefaciens, and Acetobacter orientalis), and yeasts (e.g., Saccharomyces cerevisiae, Dekkera bruxellensis, Candida ethanolica, and Saccharomyces cerevisiae) are considered the primary microbial constituents of water kefir (Lynch et al., 2021; Alrosan et al., 2022c). These microorganisms and their enzymes were likely responsible for the modifications in the fermented LP-QP complexes and other nutrient and non-nutrient profiles that resulted from fermentation. On Day 2, AAB and yeasts multiplied to over 7.2 log CFU/mL from <6 log CFU/mL on Day 0. In comparison, LAB reached 6.8 log CFU/mL on Day 2 (Figure 1). The LP-QP complexes and the sugar composition of water kefir are good sources of nutrients to sustain the growth of these fermenting microorganisms and the production of their enzymes. In water kefir multiculture fermentation, yeasts initiate the fermentation process and produce enzymes that play a key role in hydrolyzing simple sugars, including fructose, glucose, and sucrose, as well as in producing ethanol (Lynch et al., 2021). The primary sources of carbon sustaining the development of LAB are glucose and fructose, while ethanol is the primary substrate supporting AAB growth. The microbial diversity of water kefir and the synergic action of AAB, LAB, and yeasts enable the production of distinctively flavored and health-promoting beverages that are a popular alternative to sugary drinks (Gulitz et al., 2011).
Figure 1. Cell counts of lactic acid bacteria (LAB), acetic acid bacteria (AAB), and yeasts during water kefir fermentation of LP-QP complexes.
Water kefir fermentation significantly improved the digestibility of the LP-QP complexes from 76.43% before fermentation to 85.31% on Day 3 and then to 87.25% on Day 5 (Table 1). The fermenting microorganisms in water kefir produce a variety of enzymes, including proline-specific peptidases, exopeptidase, and intracellular peptidases, which can contribute to the breakdown of large protein assemblies into oligopeptides and then smaller peptides and amino acids (Tepari et al., 2020). Moreover, the linkages (e.g., amide, ether, and ester bonds) between nutrients (e.g., proteins, lipids, and carbohydrates) and non-nutrient components such as phenolic compounds, tannins, and saponins are also susceptible to cleavage by microbial enzymes (Chandra-Hioe et al., 2016; Emkani et al., 2022; Alrosan et al., 2023). Protein unfolding as the pH decreases promotes access of the enzymes to their cleavage sites. As illustrated in Figure 2, these enzymatic reactions decrease the molecular weight of the protein assemblies and modify the biological and physicochemical properties of fermented proteins (Siracusa, 2019; Alrosan et al., 2022c).
Figure 2. Schematic diagram of water kefir fermentation of LP-QP complexes highlighting the degraded bonds between nutrients and non-nutrient compounds together with the main outcomes.
Increased in vitro digestibility of the fermented LP-QP complexes is consistent with the wide variety of fermenting microorganisms found in water kefir (Rodrigues et al., 2016) and with earlier findings. Aguirre et al. (2008, 2014) showed that LAB (Lactobacillus delbrueckii subsp. lactis, Lactobacillus helveticus, Limosilactobacillus reuteri, Lapidilactobacillus dextrinicus, Lactiplantibacillus plantarum subsp. plantarum, Limosilactobacillus fermentum, and Lacticaseibacillus genus) and their enzymes can degrade the 7S β-conglycinin and 11S glycinin fractions of soy proteins. Improved protein digestibility from 70.5 to 77.2% was reported after faba bean and chickpea flour fermentation with Lactobacillus genus and Streptococcus bulgaricus from lyophilized yogurt cultures (Chandra-Hioe et al., 2016). Lactobacillus fermentation was also found to enhance the digestibility of amaranth and pea proteins (Çabuk et al., 2018; Ayala-Niño et al., 2019) as well as ovomucoid and ovalbumin (Jia et al., 2021). Recently, Alrosan et al. (2023) reported an increase in the digestibility of casein-LP complexes prepared by PPI and water kefir fermentation, from 79 to 86%.
The secondary structure components of the LP-QP complexes were assessed by FTIR. As shown in Table 2, all the secondary structure elements were present in appreciable proportions in the unfermented complexes, specifically β-turns (39.50%), β-sheets (33.58%), random coil (RC, 14.77%), and α-helices (12.13%). The contents of β-sheets and β-turns were not significantly modified during water kefir fermentation. This finding is consistent with improved protein digestibility because increased β-sheet content has been associated with impaired protein digestibility (Carbonaro et al., 2012; Bai et al., 2016). In contrast, the α-helix and RC contents of the unfermented and fermented LP-QP complexes differed significantly (P < 0.05), and the α-helix to β-sheet ratio dropped from 36.12 (Day 0) to 26.37 and 26.50 (Days 2 and 5). Positive correlations between the amount of α-helix, RC, and the α-helix to β-sheet ratio and the in vitro digestibility of feed proteins have been described in the literature (Peng et al., 2014; Bai et al., 2016). Thus, our findings of improved digestibility of fermented LP-QP complexes could be due to increased RC content (from 14.77% on Day 0 to 19.46% on Day 5) and unchanged β-sheet content. It is possible that the decreased content of α-helix and α-helix to β-sheet ratio may have been moderate enough so that no net reduction in protein digestibility was detected. Another plausible explanation is that the associations between the amount of these secondary structures and protein digestibility may be influenced by the food/feed matrix and composition, and/or by the experimental conditions used to determine protein digestibility. In line with our findings, Samadi and Yu (2011) showed that in situ protein digestibility and α-helix to β-sheet ratio varied in opposite directions after heat treatment of soybean seeds. Similar findings were reported with flaxseeds (Doiron et al., 2009).
Table 2. Changes in the percentage of secondary structure components [β-sheet, random coil (RC), α-helix, and β-turn] of unfermented LP-QP complexes (day 0) and water-kefir fermented LP-QP complexes (days 1–5) based on FTIR measurements.
While water solubility of the LP-QP complexes was not assessed in the present study, it is possible that the reduction in α-helix content and α-helix to β-sheet ratio achieved after fermentation might have improved the water solubility of these complexes. According to Alrosan et al. (2023), increasing the proportion of casein in LP-casein complexes significantly increased their water solubility from 68.6 to 91.4%. They further showed that subsequent fermentation with water kefir reduced the α-helix to β-sheet ratio and increased protein digestibility from 79.5 to 86.8%. Future studies will be needed to establish the water solubility and other important functional properties of LP-QP complexes.
Such structural changes could be explained by concomitant acidification and enzymatic action. The acidification caused by the production of lactic and acetic acids weakens the ionic interactions that stabilize the protein's secondary and tertiary structures, which promotes protein unfolding and changes in protein structures (Meinlschmidt et al., 2016). According to Wang et al. (2021), LAB and their enzymes are essential in fermented food applications and contribute to the hydrolysis of both sugars and proteins in plant proteins. LAB can secrete extracellular enzymes and hydrolyze carbohydrates and proteins into smaller fragments. Most sugar- and polysaccharide-hydrolyzing bacterial strains, such as Lactiplantabacillus plantarum, Levilactobacillus brevis, and Lactiplantibacillus plantarum (Gänzle and Zheng, 2019; Wang et al., 2021), can produce amylase to degrade starch into dextrin and ultimately glucose. Similarly, protein hydrolysis by LAB involves several steps, including protein breakdown, peptide transport and degradation, and amino acid catabolism (Kunji et al., 1996; Christensen et al., 1999). This process is initiated by cell envelope proteinases that hydrolyze proteins into oligopeptides, which are in turn converted into tri- and dipeptides and amino acids by the action of various peptidases enzymes, such as tri- and dipeptidases, aminopeptidases, endopeptidases, and proline-specific peptidases (Vesanto et al., 1996).
Our findings provide evidence of rearranging secondary structure components (α-helix and RC) of LP-QP complexes induced by fermentation. The α-helices content was significantly reduced (P < 0.05) to 7.63% at the end of water kefir fermentation, while RC increased to 19.46% (Table 2). The helix-coil conformational transition could explain these observations because the magnitude of change in α-helix and RC proportions was similar in absolute value (ca. −4 and +4 points, respectively), while the proportions of other structural elements remained stable. Similarly, fermentation with Aspergillus ficuum was found to alter the secondary protein structure of soy protein and to decrease the α-helix content from 19.16 to 14.48% after 24 h (Yasar et al., 2020). In Yasar et al.'s (2020) study, the α-helix component was completely degraded after 48 h, leading to a significant increase in RC and β-turn contents. Other types of fermentation were shown to impact these structural components. Alrosan et al. (2023) evidenced changes in the α-helix and RC contents of casein-LP complexes upon water kefir fermentation, while Yakubu et al. (2022) reported variations in β-sheets (55–48%), RC (12–15%), and β-turns (34–29%) after alkaline fermentation of locust bean flour.
As shown in Table 1, the concentrations of fructose, glucose, and sucrose evolved rapidly throughout water kefir fermentation. Sucrose concentration decreased from 4.85 g/L on Day 0 to 0.11 g/L on Day 2 and then to non-detectable levels on Days 4 and 5. In contrast, fructose and glucose concentrations peaked on Day 1 (2.07 g/L) and Day 2 (2.54 g/L), respectively, and steadily decreased subsequently. These findings are consistent with the rapid growth of LAB, AAB, and yeasts and the corresponding pH decline that occurred within the first 48 h of fermentation (Figure 1; Table 1). They concur with existing findings (Martínez-Torres et al., 2017; Tu et al., 2019; Alrosan et al., 2023). In Alrosan et al.'s (2023) study, fructose and glucose levels rose within the first 48 h of water kefir fermentation of casein-LP complexes, followed by a steady diminution of all soluble sugars until the end of fermentation.
Kefir yeasts not only metabolize these soluble sugars for growth and enzymatic activities, but they also release glucose and fructose that the commensal kefir microorganisms can use. Moreover, they play a crucial role by releasing peptides and amino acids to the LAB (e.g., Lentilactobacillus hilgardii) (Leroi and Pidoux, 1993; Rodriguez et al., 2022). Previous studies by Stadie et al. (2013) and Xu et al. (2019) have shown mutually beneficial outcomes between LAB and yeasts in water kefir, including enhanced microbial growth when LAB (e.g., Liquorilactobacillus hordei and Liquorilactobacillus nagelii) and yeasts (e.g., Saccharomyces cerevisiae and Zygotorulaspora florentina) are co-cultivated. Overall, our findings suggest that the water kefir fermentation process can be optimized to produce LP-QP complexes with low sugar content and desirable pH by adjusting the fermentation conditions and establishing adequate proportions of yeasts and LAB similar to those shown in Figure 1. Further studies would be required to optimize this fermentation process.
Plant-based proteins are associated with a wide range of health-promoting compounds, including phenolic compounds (Oomah et al., 2011). Phenolic compounds can form covalent bonds with proteins and other nutrients (Santos-Zea et al., 2018), which could influence protein digestibility and functional properties. In the present study, the TPC of the LP-QP complexes significantly increased after water kefir fermentation, from 364 mg GAE/100 g on Day 0 to 492 and 409 mg GAE/100 g on Days 2 and 5, respectively (Table 3). The main phenolic compounds identified were catechin, chlorogenic acid, epicatechin, quercetin, rutin, caffeic acid, ferulic acid, gallic acid, sinapic acid, and syringic acid (Table 3). The most abundant phenolics in the unfermented complexes were epicatechin, chlorogenic acid, and syringic acid (96.5, 48.8, and 29.5 mg/100 g, respectively). After fermentation for 5 days, catechin also reached a high level (31.5 mg/100 g). The levels of the individual phenolic compounds peaked on Days 2 or 3. A 2-fold increase was evidenced for catechin (Day 2), caffeic acid, gallic acid, and sinapic acid (Day 3). Other phenolics, including chlorogenic acid, epicatechin, and rutin, markedly increased on Day 2 or 3 (from 48.83 to 65.97, 96.49 to 134.69, and 7.39 to 12.05 mg/100 g, respectively).
Table 3. Changes in total phenolic content (TPC, mg GAE/100 g) and individual phenolic compounds (mg/100 g) of unfermented LP-QP complexes (day 0) and water kefir fermented LP-QP complexes (days 1–5).
The increased phenolic content of fermented LP-QP complexes could be due to the activities of the fermenting microorganisms found in water kefir, which may produce enzymes such as phenolic acid esterases and tannases that cleave the ester bonds that connect phenolic compounds to other compounds, most notably proteins (via hydroxyl groups) and carbohydrates (via carboxylic groups) (Bhanja et al., 2009; Dordević et al., 2010; Adebo and Medina-Meza, 2020; Shahidi and Dissanayaka, 2023), as illustrated in Figure 2. Such enzymatic action may not only increase the amounts of free phenolic compounds but may also release smaller or otherwise modified phenolic-derived metabolites with distinct biological activities (Liyana-Pathirana and Shahidi, 2006; Knockaert et al., 2012; Santos et al., 2018). Phenolic compounds and proteins also form complexes via non-covalent interactions through hydrophobic, electrostatic, van der Waals forces, and hydrogen bonding (Shahidi and Dissanayaka, 2023), which may be weakened or disrupted during fermentation as a result of the changing pH and ionic strength. Our findings are supported by earlier reports pointing to a rise in the phenolic compounds during the fermentation of plant-based proteins (Lai et al., 2013; Gunenc et al., 2017; Alrosan et al., 2023). Aiello et al. (2020) concluded that fermentation with water kefir is an effective strategy for enhancing the phenolic content and antioxidant capacity of plant-based materials.
We further found that after Days 2 or 3 of water kefir fermentation, the level of phenolic compounds started to decrease (P < 0.05). However, for most individual phenolics, except ferulic acid, gallic acid, and syringic acid, the levels achieved on Day 5 remained significantly greater than those in the unfermented complexes. This gradual reduction may be attributed to the hydrolysis of some phenolic compounds by some fermenting microorganisms, including Lactobacillus spp. (Adebo and Medina-Meza, 2020), which could result in the production of smaller catabolites. Further studies are needed to clarify the mechanisms underlying this phenomenon and to assess the bioactive properties of the resulting compounds.
Saponins are a diverse group of bioactive phytochemicals containing a carbohydrate moiety linked to a triterpenoid or steroid aglycone (Zhang et al., 2018). Many pharmacological properties have been reported for these compounds, including hypocholesterolemic, hypoglycemic, anti-inflammatory, and immunostimulant activities (Marrelli et al., 2016). However, saponins in cereals and legumes can confer a bitter taste resulting in reduced consumer acceptance (Han et al., 2018). According to Suárez-Estrella et al. (2021), high amounts of saponins (e.g., 860 mg/100 g in quinoa sprouts) can lead to poor consumer acceptability. The total saponin content of extracts from LP and QP was found to range from 0.19 to 10.63 g/100 g and 0.26 to 5.51 g/100 g, respectively, depending on the extraction solvent (del Hierro et al., 2018). The amount of saponins interacting with these proteins depends on the raw materials (e.g., variety, source, and origin) and on the preparation and extraction methods, especially solvent polarity which strongly influences the extraction and recovery yield.
We showed that the unfermented LP-QP complexes contained a relatively high amount of TSC (74.52 mg OAE/100 g) (Table 1). Upon fermentation by water kefir, TSC declined significantly (P < 0.05) to 66.63 mg OAE/100 g on Day 2 and ultimately to 60.50 mg OAE/100 g on Day 5. This drop may be attributed to weaker hydrogen bonding and electrostatic or hydrophobic interactions between saponins and proteins (Li et al., 2023) and/or to saponin hydrolysis by microbial enzymes during fermentation (Dajanta et al., 2011). Decreasing TSC is consistent with previous findings obtained with casein-LP complexes subjected to water kefir fermentation (Alrosan et al., 2023). Other studies indicated that fermentation with Aspergillus oryzae, Rhizopus stolonifer, and Bacillus can reduce saponin and phytate levels due to the action of their extracellular enzymes (Nout, 2009; Tsuji et al., 2015). Lactic fermentation by LAB was found to decrease the TSC in soymilk from 115.02 to 60.99 mg saponin equivalent/g (Lai et al., 2013). The reduced TSC contents achieved at the end of the fermentation could reduce the perceived bitterness while preserving the health benefits associated with dietary saponins.
This study provides a novel combination strategy for improving the functionality of LP and QP by forming soluble LP-QP complexes produced by protein–protein interactions, possibly including other nutritious compounds, and further modified by fermentation with water kefir. This combined (dual) treatment improved the quality of the fermented LP-QP complexes. The significant enhancement of protein digestibility in vitro may reflect the unique protein configurations and composition achieved through complexation and fermentation. Modified α-helix and random coil contents were evidenced over the course of fermentation, which were attributed to the activity of water kefir fermenting microorganisms and their enzymes. These improvements may lead to increased biological value and more widespread utilization of LP and QP, which could have important implications for overall health and nutrition. The increased phenolic content is also noteworthy, suggesting that these health-promoting compounds become more readily accessible upon fermentation with water kefir. Concomitantly, the reduced saponin content of the protein complexes could translate into reduced bitterness and thus greater acceptability of this novel multifunctional ingredient. Further research is needed to assess the sensory and functional properties of the fermented LP-QP complexes, their bioactive peptide content, and their bioavailability in vivo.
The original contributions presented in the study are included in the article/supplementary material. Further inquiries can be directed to the corresponding authors.
MAlr collected test data and drafted the manuscript. MAlr and T-CT designed the study, interpreted the results, and revised the manuscript. CCT contributed in conceptualization, visualization, interpreting the results, resources, and writing – review and editing. All authors contributed to the article and approved the submitted version.
This project was funded by the Fundamental Research Grant Scheme of Malaysia (FRGS/1/2019/STG01/USM/02/8), with additional funding from the Researchers Supporting Project (RSP2023R502) from King Saud University, Riyadh, Saudi Arabia.
The Fundamental Research Grant Scheme (FRGS/1/2019/STG01/USM/02/8) awarded by the Malaysian Ministry of Higher Education supported this project. The research was also partially funded by the Researchers Supporting Project number (RSP2023R502), King Saud University, Riyadh, Saudi Arabia. In addition, we gladly appreciate and thank the referees for their valuable remarks and recommendations for enhancing the manuscript.
The authors declare that the research was conducted in the absence of any commercial or financial relationships that could be construed as a potential conflict of interest.
All claims expressed in this article are solely those of the authors and do not necessarily represent those of their affiliated organizations, or those of the publisher, the editors and the reviewers. Any product that may be evaluated in this article, or claim that may be made by its manufacturer, is not guaranteed or endorsed by the publisher.
Abugoch, L. E., Romero, N., Tapia, C. A., Silva, J., and Rivera, M. (2008). Study of some physicochemical and functional properties of quinoa (Chenopodium quinoa Willd) protein isolates. J. Agric. Food Chem. 56, 4745–4750. doi: 10.1021/jf703689u
Adebo, O. A., and Medina-Meza, I. G. (2020). Impact of fermentation on the phenolic compounds and antioxidant activity of whole cereal grains: a mini review. Molecules 25, 927. doi: 10.3390/molecules25040927
Aguirre, L., Garro, M. S., and de Giori, G. S. (2008). Enzymatic hydrolysis of soybean protein using lactic acid bacteria. Food Chem. 111, 976–982. doi: 10.1016/j.foodchem.2008.05.018
Aguirre, L., Hebert, E. M., Garro, M. S., and de Giori, G. S. (2014). Proteolytic activity of Lactobacillus strains on soybean. LWT Food Sci. Technol. 59, 780–785. doi: 10.1016/j.lwt.2014.06.061
Aiello, F., Restuccia, D., Spizzirri, U. G., Carullo, G., Leporini, M., and Loizzo, M. R. (2020). Improving kefir bioactive properties by functional enrichment with plant and agro-food waste extracts. Fermentation 6, 83. doi: 10.3390/fermentation6030083
Almeida, C. C., Monteiro, M. L. G., da Costa-Lima, B. R. C., Alvares, T. S., and Conte-Junior, C. A. (2015). In vitro digestibility of commercial whey protein supplements. LWT Food Sci. Technol. 61, 7–11. doi: 10.1016/j.lwt.2014.11.038
Alrosan, M., Tan, T. C., Easa, A. M., Alu'datt, M. H., Tranchant, C. C., Almajwal, A. M., et al. (2023). Improving the functionality of lentil-casein protein complexes through structural interactions and water kefir-assisted fermentation. Fermentation 9, 194. doi: 10.3390/fermentation9020194
Alrosan, M., Tan, T. C., Easa, A. M., Gammoh, S., and Alu'datt, M. H. (2021). Effects of fermentation on the quality, structure, and non-nutritive contents of lentil (Lens culinaris) proteins. J. Food Qual. 2021, 5556450. doi: 10.1155/2021/5556450
Alrosan, M., Tan, T. C., Easa, A. M., Gammoh, S., and Alu'datt, M. H. (2022a). Recent updates on lentil and quinoa protein-based dairy protein alternatives: nutrition, technologies, and challenges. Food Chem. 383, 132386. doi: 10.1016/j.foodchem.2022.132386
Alrosan, M., Tan, T. C., Easa, A. M., Gammoh, S., Kubow, S., and Alu'datt, M. H. (2022b). Mechanisms of molecular and structural interactions between lentil and quinoa proteins in aqueous solutions induced by pH recycling. Int. J. Food Sci. Technol. 57, 2039–2050. doi: 10.1111/ijfs.15422
Alrosan, M., Tan, T. C., Koh, W. Y., Easa, A. M., Gammoh, S., and Alu'datt, M. H. (2022c). Overview of fermentation process: structure-function relationship on protein quality and non-nutritive compounds of plant-based proteins and carbohydrates. Crit. Rev. Food Sci. Nutr. 1–15. doi: 10.1080/10408398.2022.2049200
AOAC (2012). Official Methods of Analysis of AOAC, 19th Edn. Rockville, MD: Association of Official Analytical Chemists International.
Ayala-Niño, A., Rodríguez-Serrano, G. M., Jiménez-Alvarado, R., Bautista-Avila, M., Sánchez-Franco, J. A., González-Olivares, L. G., et al. (2019). Bioactivity of peptides released during lactic fermentation of amaranth proteins with potential cardiovascular protective effect: an in vitro study. J. Med. Food 22, 976–981. doi: 10.1089/jmf.2019.0039
Azi, F., Tu, C., Rasheed, H. A., and Dong, M. (2020). Comparative study of the phenolics, antioxidant and metagenomic composition of novel soy whey-based beverages produced using three different water kefir microbiota. Int. J. Food Sci. Technol. 55, 1689–1697. doi: 10.1111/ijfs.14439
Bai, M., Qin, G., Sun, Z., and Long, G. (2016). Relationship between molecular structure characteristics of feed proteins and protein in vitro digestibility and solubility. Asian Aust. J. Anim. Sci. 29, 1159–1165. doi: 10.5713/ajas.15.0701
Bhanja, T., Kumari, A., and Banerjee, R. (2009). Enrichment of phenolics and free radical scavenging property of wheat koji prepared with two filamentous fungi. Bioresour. Technol. 100, 2861–2866. doi: 10.1016/j.biortech.2008.12.055
Çabuk, B., Nosworthy, M. G., Stone, A. K., Korber, D. R., Tanaka, T., House, J. D., et al. (2018). Effect of fermentation on the protein digestibility and levels of non-nutritive compounds of pea protein concentrate. Food Technol. Biotechnol. 56, 257–264. doi: 10.17113/ftb.56.02.18.5450
Carbonaro, M., Maselli, P., and Nucara, A. (2012). Relationship between digestibility and secondary structure of raw and thermally treated legume proteins: a Fourier transform infrared (FT-IR) spectroscopic study. Amino Acids 43, 911–921. doi: 10.1007/s00726-011-1151-4
Chandra-Hioe, M. V., Wong, C. H., and Arcot, J. (2016). The potential use of fermented chickpea and faba bean flour as food ingredients. Plant Foods Hum. Nutr. 71, 90–95. doi: 10.1007/s11130-016-0532-y
Christensen, J. E., Dudley, E. G., Pederson, J. A., and Steele, J. L. (1999). Peptidases and amino acid catabolism in lactic acid bacteria. Antonie Van Leeuwenhoek 76, 217–246. doi: 10.1023/A:1002001919720
Dajanta, K., Apichartsrangkoon, A., Chukeatirote, E., and Frazier, R. A. (2011). Free-amino acid profiles of thua nao, a Thai fermented soybean. Food Chem. 125, 342–347. doi: 10.1016/j.foodchem.2010.09.002
del Hierro, J. N., Herrera, T., García-Risco, M. R., Fornari, T., Reglero, G., and Martin, D. (2018). Ultrasound-assisted extraction and bioaccessibility of saponins from edible seeds: quinoa, lentil, fenugreek, soybean and lupin. Food Res. Int. 109, 440–447. doi: 10.1016/j.foodres.2018.04.058
Doiron, K., Yu, P., McKinnon, J. J., and Christensen, D. A. (2009). Heat-induced protein structure and subfractions in relation to protein degradation kinetics and intestinal availability in dairy cattle. J. Dairy Sci. 92, 3319–3330. doi: 10.3168/jds.2008-1946
Dordević, T. M., Šiler-Marinković, S. S., and Dimitrijević-Branković, S. I. (2010). Effect of fermentation on antioxidant properties of some cereals and pseudo cereals. Food Chem. 119, 957–963. doi: 10.1016/j.foodchem.2009.07.049
Dos Santos, D. C., de Oliveira Filho, J. G., Santana, A. C. A., de Freitas, B. S. M., Silva, F. G., Takeuchi, K. P., et al. (2019). Optimization of soymilk fermentation with kefir and the addition of inulin: Physicochemical, sensory and technological characteristics. LWT Food Sci. Technol. 104, 30–37. doi: 10.1016/j.lwt.2019.01.030
Emkani, M., Oliete, B., and Saurel, R. (2022). Effect of lactic acid fermentation on legume protein properties, a review. Fermentation 8, 244. doi: 10.3390/fermentation8060244
Fessard, A., Kapoor, A., Patche, J., Assemat, S., Hoarau, M., Bourdon, E., et al. (2017). Lactic fermentation as an efficient tool to enhance the antioxidant activity of tropical fruit juices and teas. Microorganisms 5, 23. doi: 10.3390/microorganisms5020023
Gänzle, M. G., and Zheng, J. (2019). Lifestyles of sourdough lactobacilli–Do they matter for microbial ecology and bread quality? Int. J. Food Microbiol. 302, 15–23. doi: 10.1016/j.ijfoodmicro.2018.08.019
Gulitz, A., Stadie, J., Wenning, M., Ehrmann, M. A., and Vogel, R. F. (2011). The microbial diversity of water kefir. Int. J. Food Microbiol. 151, 284–288. doi: 10.1016/j.ijfoodmicro.2011.09.016
Gunenc, A., Yeung, M. H., Lavergne, C., Bertinato, J., and Hosseinian, F. (2017). Enhancements of antioxidant activity and mineral solubility of germinated wrinkled lentils during fermentation in kefir. J. Funct. Foods 32, 72–79. doi: 10.1016/j.jff.2017.02.016
Han, Z., Cai, M.-,j., Cheng, J.-H., and Sun, D.-W. (2018). Effects of electric fields and electromagnetic wave on food protein structure and functionality: a review. Trends Food Sci. Technol. 75, 1–9. doi: 10.1016/j.tifs.2018.02.017
He, C., Hu, Y., Liu, Z., Woo, M. W., Xiong, H., and Zhao, Q. (2020). Interaction between casein and rice glutelin: binding mechanisms and molecular assembly behaviours. Food Hydrocoll. 107, 105967. doi: 10.1016/j.foodhyd.2020.105967
Jarpa-Parra, M., Bamdad, F., Wang, Y., Tian, Z., Temelli, F., Han, J., et al. (2014). Optimization of lentil protein extraction and the influence of process pH on protein structure and functionality. LWT Food Sci. Technol. 57, 461–469. doi: 10.1016/j.lwt.2014.02.035
Jia, J., Ji, B., Tian, L., Li, M., Lu, M., Ding, L., et al. (2021). Mechanism study on enhanced foaming properties of individual albumen proteins by Lactobacillus fermentation. Food Hydrocoll. 111, 106218. doi: 10.1016/j.foodhyd.2020.106218
Knockaert, D., Van Camp, J., Struijs, K., Wille, C., and Raes, K. (2012). “Potential of lactic acid fermentation to produce health beneficial compounds from vegetable waste,” in The 23rd International ICFMH Symposium FoodMicro 2012: Global Issues in Food Microbiology (Istanbul).
Kunji, E. R., Mierau, I., Hagting, A., Poolman, B., and Konings, W. N. (1996). The proteotytic systems of lactic acid bacteria. Antonie Van Leeuwenhoek 70, 187–221. doi: 10.1007/BF00395933
Lai, L.-R., Hsieh, S.-C., Huang, H.-Y., and Chou, C.-C. (2013). Effect of lactic fermentation on the total phenolic, saponin and phytic acid contents as well as anti-colon cancer cell proliferation activity of soymilk. J. Biosci. Bioeng. 115, 552–556. doi: 10.1016/j.jbiosc.2012.11.022
Laureys, D., Aerts, M., Vandamme, P., and De Vuyst, L. (2019). The buffer capacity and calcium concentration of water influence the microbial species diversity, grain growth, and metabolite production during water kefir fermentation. Front. Microbiol. 10, 2876. doi: 10.3389/fmicb.2019.02876
Leroi, F., and Pidoux, M. (1993). Detection of interactions between yeasts and lactic acid bacteria isolated from sugary kefir grains. J. Appl. Bacteriol. 74, 48–53. doi: 10.1111/j.1365-2672.1993.tb02995.x
Li, Y., Liu, X., Liu, H., and Zhu, L. (2023). Interfacial adsorption behavior and interaction mechanism in saponin–protein composite systems: a review. Food Hydrocoll. 136, 108295. doi: 10.1016/j.foodhyd.2022.108295
Lingiardi, N., Galante, M., de Sanctis, M., and Spelzini, D. (2022). Are quinoa proteins a promising alternative to be applied in plant-based emulsion gel formulation? Food Chem. 394, 133485. doi: 10.1016/j.foodchem.2022.133485
Liu, C., Zhang, Z., Dang, Z., Xu, J., and Ren, X. (2021). New insights on phenolic compound metabolism in pomegranate fruit during storage. Sci. Hortic. 285, 110138. doi: 10.1016/j.scienta.2021.110138
Liyana-Pathirana, C. M., and Shahidi, F. (2006). Importance of insoluble-bound phenolics to antioxidant properties of wheat. J. Agric. Food Chem. 54, 1256–1264. doi: 10.1021/jf052556h
Lynch, K. M., Wilkinson, S., Daenen, L., and Arendt, E. K. (2021). An update on water kefir: microbiology, composition and production. Int. J. Food Microbiol. 345, 109128. doi: 10.1016/j.ijfoodmicro.2021.109128
Marrelli, M., Conforti, F., Araniti, F., and Statti, G. A. (2016). Effects of saponins on lipid metabolism: a review of potential health benefits in the treatment of obesity. Molecules 21, 1404. doi: 10.3390/molecules21101404
Martínez-Torres, A., Gutiérrez-Ambrocio, S., Heredia-del-Orbe, P., Villa-Tanaca, L., and Hernández-Rodríguez, C. (2017). Inferring the role of microorganisms in water kefir fermentations. Int. J. Food Sci. Technol. 52, 559–571. doi: 10.1111/ijfs.13312
Meinlschmidt, P., Ueberham, E., Lehmann, J., Schweiggert-Weisz, U., and Eisner, P. (2016). Immunoreactivity, sensory and physicochemical properties of fermented soy protein isolate. Food Chem. 205, 229–238. doi: 10.1016/j.foodchem.2016.03.016
Nout, M. R. (2009). Rich nutrition from the poorest–cereal fermentations in Africa and Asia. Food Microbiol. 26, 685–692. doi: 10.1016/j.fm.2009.07.002
Oomah, B. D., Caspar, F., Malcolmson, L. J., and Bellido, A.-S. (2011). Phenolics and antioxidant activity of lentil and pea hulls. Food Res. Int. 44, 436–441. doi: 10.1016/j.foodres.2010.09.027
Peng, Q., Khan, N. A., Wang, Z., and Yu, P. (2014). Relationship of feeds protein structural makeup in common Prairie feeds with protein solubility, in situ ruminal degradation and intestinal digestibility. Anim. Feed Sci. Technol. 194, 58–70. doi: 10.1016/j.anifeedsci.2014.05.004
Rodrigues, K. L., Araújo, T. H., Schneedorf, J. M., de Souza Ferreira, C., Moraes, G. d. O. I., et al. (2016). A novel beer fermented by kefir enhances anti-inflammatory and anti-ulcerogenic activities found isolated in its constituents. J. Funct. Foods 21, 58–69. doi: 10.1016/j.jff.2015.11.035
Rodriguez, M. A., Fernandez, L. A., Diaz, M. L., Perez, M., Corona, M., and Reynaldi, F. J. (2022). Microbiological and chemical characterization of water kefir: an innovative source of potential probiotics for bee nutrition. Rev. Argent. Microbiol. 55, 176–180. doi: 10.1016/j.ram.2022.09.003
Samadi, and Yu, P. (2011). Dry and moist heating-induced changes in protein molecular structure, protein subfraction, and nutrient profiles in soybeans. J. Dairy Sci. 94, 6092–6102. doi: 10.3168/jds.2011-4619
Santos, M. B., da Costa, A. R., and Garcia-Rojas, E. E. (2018). Heteroprotein complex coacervates of ovalbumin and lysozyme: formation and thermodynamic characterization. Int. J. Biol. Macromol. 106, 1323–1329. doi: 10.1016/j.ijbiomac.2017.08.132
Santos-Zea, L., Villela-Castrejon, J., and Gutierrez-Uribe, J. A. (2018). “Bound phenolics in foods,” in Bioactive Molecules in Food. Reference Series in Phytochemistry, eds J. M. Merillon, and K. Ramawat (Cham: Springer).
Shahidi, F., and Dissanayaka, C. S. (2023). Phenolic-protein interactions: insight from in-silico analyses – A review. Food Prod. Process. Nutr. 5, 2. doi: 10.1186/s43014-022-00121-0
Siracusa, V. (2019). Microbial degradation of synthetic biopolymers waste. Polymers 11, 1066. doi: 10.3390/polym11061066
Stadie, J., Gulitz, A., Ehrmann, M. A., and Vogel, R. F. (2013). Metabolic activity and symbiotic interactions of lactic acid bacteria and yeasts isolated from water kefir. Food Microbiol. 35, 92–98. doi: 10.1016/j.fm.2013.03.009
Suárez-Estrella, D., Borgonono, G., Buratti, S., Ferranti, P., Accardo, F., Pagani, M. A., et al. (2021). Sprouting of quinoa (Chenopodium quinoa Willd.): Effect on saponin content and relation to the taste and astringency assessed by electronic tongue. LWT Food Sci. Technol. 144, 111234. doi: 10.1016/j.lwt.2021.111234
Tepari, E. A., Nakhla, G., Haroun, B. M., and Hafez, H. (2020). Co-fermentation of carbohydrates and proteins for biohydrogen production: statistical optimization using response surface methodology. Int. J. Hydrog. Energy 45, 2640–2654. doi: 10.1016/j.ijhydene.2019.11.160
Tsuji, S., Tanaka, K., Takenaka, S., and Yoshida, K.-,i. (2015). Enhanced secretion of natto phytase by Bacillus subtilis. Biosci. Biotechnol. Biochem. 79, 1906–1914. doi: 10.1080/09168451.2015.1046366
Tu, C., Azi, F., Huang, J., Xu, X., Xing, G., and Dong, M. (2019). Quality and metagenomic evaluation of a novel functional beverage produced from soy whey using water kefir grains. LWT Food Sci. Technol. 113, 108258. doi: 10.1016/j.lwt.2019.108258
Vesanto, E., Peltoniemi, K., Purtsi, T., Steele, J. L., and Palva, A. (1996). Molecular characterization, over-expression and purification of a novel dipeptidase from Lactobacillus helveticus. Appl. Microbiol. Biotechnol. 45, 638–645. doi: 10.1007/s002530050741
Wang, R., Xu, P., Chen, Z., Zhou, X., and Wang, T. (2019). Complexation of rice proteins and whey protein isolates by structural interactions to prepare soluble protein composites. LWT Food Sci. Technol. 101, 207–213. doi: 10.1016/j.lwt.2018.11.006
Wang, Y., Wu, J., Lv, M., Shao, Z., Hungwe, M., Wang, J., et al. (2021). Metabolism characteristics of lactic acid bacteria and the expanding applications in food industry. Front. Bioeng. Biotechnol. 9, 612285. doi: 10.3389/fbioe.2021.612285
Xu, D., Bechtner, J., Behr, J., Eisenbach, L., Geißler, A. J., and Vogel, R. F. (2019). Lifestyle of Lactobacillus hordei isolated from water kefir based on genomic, proteomic and physiological characterization. Int. J. Food Microbiol. 290, 141–149. doi: 10.1016/j.ijfoodmicro.2018.10.004
Yakubu, C. M., Sharma, R., Sharma, S., and Singh, B. (2022). Influence of alkaline fermentation time on in vitro nutrient digestibility, bio-and techno-functionality, secondary protein structure and macromolecular morphology of locust bean (Parkia biglobosa) flour. LWT Food Sci. Technol. 161, 113295. doi: 10.1016/j.lwt.2022.113295
Yan, F., Cui, H., Zhang, Q., Hayat, K., Yu, J., Hussain, S., et al. (2021). Small peptides hydrolyzed from pea protein and their Maillard reaction products as taste modifiers: Saltiness, Umami, and Kokumi Enhancement. Food Bioprocess Technol. 14, 1132–1141. doi: 10.1007/s11947-021-02630-1
Yasar, S., Tosun, R., and Sonmez, Z. (2020). Fungal fermentation inducing improved nutritional qualities associated with altered secondary protein structure of soybean meal determined by FTIR spectroscopy. Measurement 161, 107895. doi: 10.1016/j.measurement.2020.107895
Yen, P. P. L., and Pratap-Singh, A. (2021). Vacuum microwave dehydration decreases volatile concentration and soluble protein content of pea (Pisum sativum L.) protein. J. Sci. Food Agric. 101, 167–178. doi: 10.1002/jsfa.10627
Zhang, B., Peng, H., Deng, Z., and Tsao, R. (2018). Phytochemicals of lentil (Lens culinaris) and their antioxidant and anti-inflammatory effects. J. Food Bioact. 1, 93–103. doi: 10.31665/JFB.2018.1128
Zhao, S., Huang, Y., McClements, D. J., Liu, X., Wang, P., and Liu, F. (2022). Improving pea protein functionality by combining high-pressure homogenization with an ultrasound-assisted Maillard reaction. Food Hydrocoll. 126, 107441. doi: 10.1016/j.foodhyd.2021.107441
Keywords: lentil protein, quinoa protein, protein digestibility, water kefir fermentation, phenolic compounds
Citation: Alrosan M, Tan T-C, Mat Easa A, Gammoh S, Alu'datt MH, Tranchant CC, Almajwal AM, Maghaydah S, Dheyab MA, Jameel MS, Al-Qaisi A and Al Qudsi FR (2023) Preparation of lentil and quinoa protein complexes through protein–protein interactions and water kefir–assisted fermentation to improve protein quality and functionality. Front. Sustain. Food Syst. 7:1174597. doi: 10.3389/fsufs.2023.1174597
Received: 26 February 2023; Accepted: 03 July 2023;
Published: 31 July 2023.
Edited by:
Gengjun Chen, Kansas State University, United StatesReviewed by:
Malgorzata Ziarno, Warsaw University of Life Sciences, PolandCopyright © 2023 Alrosan, Tan, Mat Easa, Gammoh, Alu'datt, Tranchant, Almajwal, Maghaydah, Dheyab, Jameel, Al-Qaisi and Al Qudsi. This is an open-access article distributed under the terms of the Creative Commons Attribution License (CC BY). The use, distribution or reproduction in other forums is permitted, provided the original author(s) and the copyright owner(s) are credited and that the original publication in this journal is cited, in accordance with accepted academic practice. No use, distribution or reproduction is permitted which does not comply with these terms.
*Correspondence: Mohammad Alrosan, bW9oYW1tYWQuYWxyb3NhbkBob3RtYWlsLmNvbQ==; Thuan-Chew Tan, dGh1YW5jaGV3QHVzbS5teQ==
†These authors have contributed equally to this work
‡ORCID: Carole C. Tranchant orcid.org/0000-0002-2026-819X
Disclaimer: All claims expressed in this article are solely those of the authors and do not necessarily represent those of their affiliated organizations, or those of the publisher, the editors and the reviewers. Any product that may be evaluated in this article or claim that may be made by its manufacturer is not guaranteed or endorsed by the publisher.
Research integrity at Frontiers
Learn more about the work of our research integrity team to safeguard the quality of each article we publish.